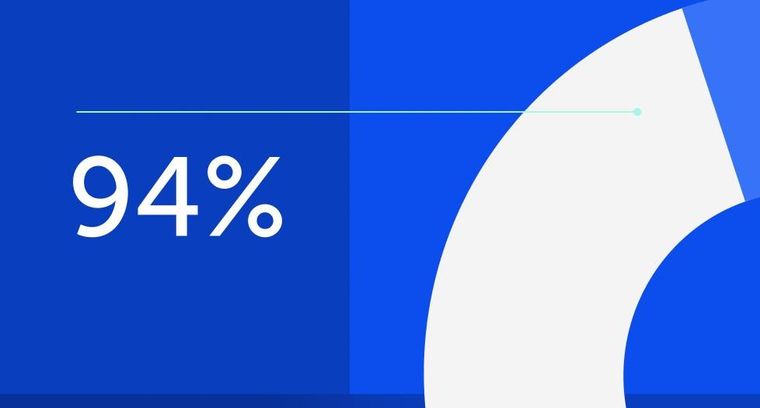
94% of researchers rate our articles as excellent or good
Learn more about the work of our research integrity team to safeguard the quality of each article we publish.
Find out more
ORIGINAL RESEARCH article
Front. Soft Matter, 26 April 2024
Sec. Food and Soft Materials
Volume 4 - 2024 | https://doi.org/10.3389/frsfm.2024.1363898
This article is part of the Research TopicCelebrating 3 Years of Frontiers in Soft MatterView all 5 articles
Introduction: Fluid gels exhibit a unique flow behaviour. Their pronounced viscoelastic behaviour arises from irregular microgel particles, leading to plasticity and yielding, as well as a characteristic transition from a solid to a fluid-like state. This is defined by both the core and the surface structures of the particles. Adding co-solutes such as sucrose alters the network properties at the molecular scale, affecting texture and lubrication. This study investigates how the microscopic changes due to sucrose addition correlate with macroscopic changes.
Material and methods: Agarose fluid gels with varying sucrose concentrations were prepared and studied using the rheometer. Temperature dependent viscosity behaviour during gelation under shear was investigated in situ. Light microscopy and particle size measurements were examined, and complemented by amplitude, frequency and flow sweeps as well as tribological studies. These tests allow us to understand the influence of sucrose on the particle network formation.
Results and discussion: Sucrose concentration influenced the sizes, shapes, and interconnected network structure of the microgel particles. These microstructural changes are closely related to the dynamic competition between gelation and disruption of the agarose chains during shear, which directly influences the rheological and tribological properties of the resulting fluid gels. Additionally, the association of the agarose chains and their gelation process is also influenced depending on whether the sucrose or agarose was first dissolved in water. The experimental observations suggest specific molecular mechanisms, explaining the role of sucrose in structure formation of agarose-based fluid gels. These results have the potential to expand the applications of fluid gels, which play a crucial role in modifying the texture and flow behaviour of foods and beverages, particularly in addressing challenges such as dysphagia.
Fluid gels are based on gelling polysaccharides such as agarose, where gelling occurs at defined shear rates, resulting in systems with controlled microstructures consisting of particulate gel suspensions (Frith et al., 2002; Gabriele et al., 2010; Farrés et al., 2014; Norton et al., 1999). The particles have an elastic core, are irregularly shaped and immersed in a continuous water phase. Due to this, fluid gels provide unique flow and textural properties, which makes them of considerable interest in various applications, including food, cosmetics, and pharmaceuticals. They offer a pleasant mouthfeel for gastronomic applications. Their cohesion is important for geriatric applications and medically induced swallowing disorders. Fluid gels exhibit the properties of shape-retaining elastic materials but have a fluid-like flow behaviour under sufficiently high stress.
This combination contributes to the special mouthfeel during oral processing. However, the texture and lubrication felt in the oral cavity, can be strongly influenced by the presence of low molecular weight co-solutes, indicating a strong effect of local (atomistic) scales with macroscopic structural changes. For a systematic illustration sucrose has been chosen as an example. As shown earlier, the addition of sugars significantly affects the properties of hydrocolloids systems by interacting with each other and with the water molecules (Nishinari et al., 1992; Watase, Kohyama, and Nishinari, 1992; Deszczynski et al., 2003a; Maurer, Junghans, and Vilgis, 2012; Russ, Zielbauer, and Vilgis, 2014). The main objective here is the investigation of the underlying physicochemical effects, the molecular interaction during gelation under shear, and the resulting fluid gel properties. In particular, this study focuses on the influences of sucrose on the resulting particle structure and the rheological and tribological behaviour of the fluid gel formation.
Adding co-solutes causes physical changes on local length scales. Sucrose has a strong binding affinity for water, causing the “bound” water to become no longer available to the agarose for unobstructed gel formation. Sufficiently higher concentrations of sucrose weaken the ability of agarose to form a gel. The mechanism promoting gel formation is not entirely clear, but is suggested to involve hydrogen bonding between sugar and agarose, leading to helix formation and aggregation (Watase et al., 1990; Watase, Kohyama, and Nishinari, 1992). However, this remains a topic of ongoing debate. Higher sucrose concentrations strongly influence the mobility of agarose chains, affecting both structure formation and changes in elasticity. The altered chain dynamics effects the structure formation, for example, via the shear rate, and the water binding of the co-solute modifies the elasticity of the resulting gel particles.
Agarose, isolated from red algae (Rhodophyceae), is a linear polar, water soluble polymer composed of units of (1–3)-linked agarobiose, which in turn consists of β-D-galactopyranose (1–4)-linked to 3,6-anhydro-α-L-galactopyranose (Imeson, 2009). The gelation mechanism involves a “two-step” mechanism during cooling (Valéry Normand et al., 2000), starting with the association of polymer chains into double helices, which then further aggregate to form a continuously denser meshed three-dimensional network and immobilize the water as investigated experimentally and emphasised earlier (Nordqvist and Vilgis, 2011; Russ et al., 2013; Russ et al., 2014). It should be emphasised that this sol-gel transition appears to be much more complex, involving different transition states. Within these two steps, described by Nordqvist et al. as major steps, the agarose chains undergo a coil-helix transition that exist in a variety of hierarchical structures.
Different chain associations for agarose by forming helical structures at the molecular level have been suggested to influence mechanical properties, as reported by Matinez-Sanza et al. (2020). These authors proposed that agarose chains in some of the samples do not only exist in a coil structure at a temperature of 75°C, but also in some form of molecular association, indicating a variety of hierarchical structures (Martínez-Sanz et al., 2020). Thus, some agarose did not show true solution behaviour (at 75°C) but rather the behaviour typical of a transient (entangled) network. They reported that although it is known that agarose chains associate by forming ordered helical structures, this observation does not necessarily mean that these helixes are already formed, but it does imply the existence of some kind of molecular association (Martínez-Sanz et al., 2020).
Recent studies using methods such as AFM and computer simulations have demonstrated that charged polysaccharides undergoing coil-helix transitions exist in a multitude of hierarchical structures (Schefer, Adamcik, and Mezzenga, 2014; Schefer et al., 2015a; Schefer, Usov, and Mezzenga, 2015b; Diener et al., 2019; 2020; Tavagnacco et al., 2023). By studying negatively charged carrageenan as a model polysaccharide for ion-mediated gelation, the authors demonstrated the presence of several structural levels determined by statistical analysis of AFM images. These works refined the understanding of ion-induced structural hierarchies, revealing coil, single helix, coiled-coiled helices, and double and multiple supercoiled helices, corresponding to primary, secondary, tertiary, and quaternary structures, respectively (Diener et al., 2019; 2020). However, these findings on ion-induced structural changes and gelation mechanism cannot be readily applied to agarose. Controlled ion-mediated gelation, which enables a targeted modification and observation of chain association, may be challenging due the lack of charge of agarose and its strong gelling properties without the addition of ions.
Thus, the investigation of intramolecular conformational changes at the secondary structure level and their influence on structural properties, particularly in response to the addition of counterions and charge screening, remains unexplored for agarose.
In contrast, Agarose, is an uncharged polysaccharide and was therefore specifically chosen by us due to its special model system for polar systems. These short ranged polar interactions make them ideal for studying the nature of biopolymer gelation (Xiong et al., 2005).
Under certain temperatures, disordered agarose chains form helical structures with tetrahedral cavities occupied by water molecules (Tako et al., 2021). The left-handed threefold helix structure of agarose, featuring a specific axial displacement and pitch of 1.90 nm, allows for the formation of a sterically acceptable parallel double helix. This structure contains a small inner cavity with inward-directed C-H bonds, lined with hydroxyl groups that promote hydrogen bonding, involving specific oxygen atoms (Ablett et al., 1978; Nussinovitch, 1997). Hydrophobic C-H bonds contribute to the cavity stability and enable higher hierarchical structures, such as multi-helical assemblies (Tako et al., 2021). The precise positioning of the water molecules within the tetrahedral cavity, determined by their specific interaction with certain oxygen atoms reinforces the double helix structure (Arnott et al., 1974; Deszczynski, Kasapis, and Mitchell, 2003b; Tako et al., 2021). Thus, hydrophobic interactions contribute to the formation of the cavity structure, while hydrogen bonds contribute to its stabilization (Fittolani, Seeberger, and Delbianco, 2020). However, this underlines the understanding that agarose gels rely solely on water as the solvent to promote helix aggregation and the formation of junction zones (Mineo Watase, Nishinari, and Hatakeyama, 1988; Vilgis, 2015). Therefore, adding sucrose to the hydrated agarose system induces strongly competing dipolar interactions and hydrogen bonding, which destabilize the helix formations and hinder their aggregation (Deszczynski, Kasapis, and Mitchell, 2003b).
Russ et al. (2014) attributed a decrease in elastic modulus with increasing sucrose concentration to the limited mobility and diffusion of the agarose polymer chains, resulting in fewer associations into double helices and their aggregation during cooling. This restriction results in a weaker three-dimensional network with larger meshes, reducing elasticity and stability (Russ, Zielbauer, and Vilgis, 2014; Vilgis, 2015). At sucrose concentrations above 40 wt%, competition for water prevents stable hydrogen bonds with agarose molecules, disrupting thermodynamically stable helix formation and depriving agarose chains of the hydration layer necessary for network formation (Deszczynski et al., 2003a; Kasapis et al., 2003; Maurer, Junghans, and Vilgis, 2012). Therefore, when a lower sucrose concentration is added, an increase in elastic modulus is explained by a reduction in free water and an increase in apparent local concentration (Deszczynski et al., 2003a; Kasapis et al., 2003).
Shimazu et al. (2014) further investigated co-solvent enhanced gelation of agarose. The authors suggested that co-solvents control the sol-gel transition by either restructuring water around the biopolymer or excluding co-solvents from the biopolymer surface. The driving force for gelation is attributed to co-solvent exclusion, followed by biopolymer aggregation due to increased compactness. This finding is relevant to the fluid gels in this study, where sucrose is dissolved in water before agarose, and supports the conclusion that co-solvent exclusion, rather than biopolymer hydration, i.e., the change in water structure around the biopolymer, drives gelation, as discussed below (Shimizu and Matubayasi, 2014). Another hypothesis suggests co-induced hydration changes, where sugar indirectly affects the sol-gel equilibrium through a change in biopolymer hydration that is influenced by competitive hydration between biopolymer and co-solvent (Watase et al., 1990; Williams et al., 1991; Watase, Kohyama, and Nishinari, 1992; Shimizu and Matubayasi, 2014). However, this concept is relevant to fluid gels, which in the current work were mainly prepared by dissolving agarose in heated water before adding sucrose. Sucrose addition to hydrocolloids, alters water structure through hydration shells (Vilgis, 2015). The dissolution of sucrose changes water structure, affecting its tendency to bind agarose polymers via hydrogen bonds. This challenges the notion of water as an inert solvent, leading to changes in gelling properties when sucrose is added (Vilgis, 2015).
Sugar significantly affects hydration water dynamics, by forming more stable hydrogen bonds with water than those between water molecules (Lee, Debenedetti, and Errington, 2005). This cumulative impact extends to the surrounding water (Zhao, Ma, and Yang, 2015) and hinders agarose polymer helix formation by removing water molecules from the helix cavity. Sucrose reduce the flexibility of agarose at high temperatures by hydrogen bonding with the hydration water of agarose molecules, causing a decrease in conformational entropy (Hédoux et al., 2006; Adrien Lerbret et al., 2007). Furthermore, a decrease in the hydration number, linked to increased sugar concentration, was observed due to overlapping of distance outer layers within the dynamic hydration shells of sugar molecules (Gharsallaoui et al., 2008). This correlation affects agarose hydration and indirect interact with sucrose.
Studies on the effect of the sugar addition before or after agar dissolution, found that gels were weaker and more inhomogeneous when more than 40 wt% of sucrose was dissolved before agar addition (Yang et al., 2015). The order of addition affected fracture stress, strain and sucrose release ratio. Other studies have also opted to add sugar after agar dissolution (Ellis, Mills, and Norton, 2019). However, for the present paper, we considered it essential to prepare the samples by first dissolving agarose in water, as we did in previous studies, in order to obtain the same initial state of the agarose-water system and thus determine the effect of sucrose on this specific system (Ghebremedhin, Seiffert, and Vilgis, 2021).
However, despite previous studies on the effect of sucrose on biopolymer fluid gels and the resulting mechanical properties, the exact mechanism and interaction during gelation under shear at the molecular level are not yet understood. Therefore, the present study investigates the effect of sucrose on the gelling mechanism of agarose during the preparation of fluid gels. The paper is organized as follow: First, we investigated the behaviour of the temperature dependent shear viscosity of the prepared fluid gels in the rheometer using the in situ method. This “in situ” method for producing fluid gels has been utilized in our previous work as well (Ghebremedhin, Seiffert, and Vilgis, 2021; 2022). It offers the advantage inducing gelation at an imposed steady shear with controlled shear and temperature rates (De Carvalho and Djabourov, 1997; Ghebremedhin, Seiffert, and Vilgis, 2021). Supported by light microscopy and particle size measurements, we examined the resulting particle sizes and shapes to understand their impact on the textural and lubricating properties in relation to sucrose concentration. Rheological studies, including amplitude sweep, frequency sweep and flow sweep were performed to gain insight into the influence of sucrose on the particulate network formation. Finally, we employed tribology to support the previous assessment results of the microgel particles by evaluating the frictional behaviour of the different fluid gels. Consequently, based on the aforementioned characterization and our earlier studies, we have proposed a schematic model that describes the mechanism involving the interplay between sucrose, agarose and water during gelation under shear, along with its effects on the rheological and tribological properties of agarose gels with added co-solutes.
The agarose [CAS: 9012–36-6] used in this study was purchased from Fisher Scientific GmbH (Schwerte, Germany) having a number-average molar mass Mn ≈ 74,557.8 g/mol, a weight-average molar mass Mw ≈ 213,949 g/mol, and a polydispersity index of 2.87 (Ghebremedhin, Seiffert, and Vilgis, 2022). The substance reaches a gel strength of >100 g/cm2, and the manufacturer specifies a gelation temperature range of 34°C–45°C. A maximum sulphate content of 0.15% was also specified. Sucrose [CAS: 57–50-1] was purchased from Sigma Aldrich (St. Louis, MO, United States of America).
In order to investigate the influence of disaccharides such as sucrose on the mechanical, viscoelastic, and tribological properties of agarose fluid gels, it is first interesting to understand their effect on the formation of the fluid gels during gelation under shear. For this purpose, agarose fluid gels with different sucrose concentrations were prepared.
Fluid gels were prepared using the Discovery HR-3 Rheometer (TA Instruments New Castle, Delaware United States of America) with a cup (diameter = 30.37 mm) and vane (diameter = 28 mm, length = 42 mm) tool geometry used together with an electrically heatable concentric cylinder Peltier Jacket. For preparation of the 1 wt% agarose fluid gels with the respective sucrose concentration (0 wt%, 10 wt%, 20 wt%, 30 wt%), agarose was slowly added to Milli-Q water while stirring (500 rpm) and heated to 85 °C for about 20 min in the sealed beaker to ensure complete dissolution. The required sucrose concentration was then added with stirring. Subsequently, the hot agarose respectively agarose-sucrose solution was poured into the cup preheated to 85 °C and allowed to equilibrate for 5 minutes more before starting the measurements. While cooling from 85°C to 25°C at a temperature rate of 1 K/min, gelation under shear was carried out at a constant shear rate of 400 s−1. This was followed by an additional 15 min of shearing at 25°C. As a result, pourable but set, spoonable smooth gels were produced with the characteristics of an elastic material that could hold its shape but also flow like liquid under sufficient stress. These fluid gel samples were then stored at 4°C for 24 h before being subjected to further characterization investigations.
It should be noted that for the fluid gel preparation mainly used in this study, the agarose concentration was kept constant at 1 wt%, while the sucrose content ranged from 0 wt% to 30 wt% and was added after dissolving agarose. Additionally, an alternative preparation method was employed for comparison purposes, where 20 wt% sucrose was initially dissolved in Milli-Q water, followed by the addition of agarose to the sucrose solution. This approach aimed to investigate and discuss the effect of the order of sucrose and agarose addition. The results and comparisons are provided in the Supplementary Material.
Dynamic viscoelastic measurements of the produced fluid gels were carried out on a Discovery HR- Rheometer (TA Instruments) with a 40 mm diameter parallel plate with a pre-set distance of 500 µm and a solvent trap. All rheological measurements were conducted in triplicate.
Amplitude sweep measurement was performed to investigate the viscoelastic properties of the different fluid gels. Using this method, oscillatory deformation was applied and storage G′ and loss G″ moduli were measured to describe the deformation behaviour of the gel samples in the non-destructive range, which is defined as the linear-viscoelastic (LVE) range. In addition, the characterization of the behaviour in the non-linear viscoelastic regime was of interest. All amplitude sweeps were carried out at a constant frequency ƒ = 1 Hz, and storage (G′) and loss (G″) moduli were measured as a function of strain
The frequency sweeps of the fluid gels were carried out in a range of 0.1–100 rad/s (0.0159–15.9 Hz) at a constant strain of 0.05%, i.e., within the LVE range of their amplitude sweep measurements.
To investigate the flow behaviour of the fluid gels and the viscosity as a function of the shear rate, rotating steady shear was performed for the flow sweep test. The shear rate was increased from 0.001 to 1,000 s−1 and then decreased from 1,000 to 0.001 s−1. The two-step flow was conducted to determine how much the hysteresis varies between the two steps, indicating a change in viscosity.
Microstructure examinations of the gel particles were performed by light microscopy using a Carl Zeiss Axio Scope.A1 microscope (Carl Zeiss AG, Oberkochen, Germany). Transmission bright field microscopy was used to capture the images, giving total magnifications of ×100 at objective magnifications of ×10. The scale bar was inserted using ImageJ software (National Institutes of Health (NIH), Maryland United States of America). Fluid gel samples were carefully transferred onto a microscope slide and then diluted with Milli-Q water (1:5). To enhance contrast, the diluted sample was stained with toluidine blue (0.1 wt%).
Static light scattering experiments were performed to determine the particle size distributions using the Beckmann Coulter LS 13320 laser diffraction particle size analyser (Beckmann Coulter, CA United States of America), which can determine particle sizes between 0.040–2,000 μm. The diffraction data were analysed using the Fraunhofer model provided by the supplier’s software. No additional data such as refractive index or absorption coefficient were required for this calculation. To avoid multiple scattering effects, samples were diluted by pipette into a measuring cell (universal liquid module) filled with distilled water until an obscuration of about 10% was attained, following the recommendation of Beckman Coulter. Size distribution measurements were conducted in triplicate.
The lubricating properties of the fluid gels with the different sucrose concentrations were determined using a tribo-rheometry accessory obtainable for the Discovery HR-3 (TA Instruments New Castle, Delaware United States of America). A three balls on a plate set up was used, consisting of an upper part with three hemispheres (diameter of 7.9375 mm or 5/16″) screwed to a plate (diameter of 30 mm) and a lower plate attached with a silicone rubber (diameter of 40 mm and thickness of 1 mm). Hence, the contact surface of the tribopairs consisted of a stainless-steel ball and a silicone surface maintaining a point contact. To ensure a uniform solid-solid contact and axial force distribution, e.g., an even distribution of the normal force between the surfaces during rotation, a helical-spring-like aluminium beam coupling was used (specified by Trios manual 2019). The lower plate geometry is attached to the Peltier plate of the DHR-3 Rheometer for stable temperature control, and measurements were performed at 25°C. A normal force of 3 N was applied, which is considered to be similar to the normal forces acting during oral processing (Laiho et al., 2017; Nguyen et al., 2017; He et al., 2018). The sliding speed was varied from 100 to 1,000,000 μm/s by rotating the upper three-ball geometry, while the lower plate geometry was held stationary. The friction force FF and friction coefficient μ were calculated for the three balls on plate set up using the trios software, with the torque M as an independent variable. As a result, the friction coefficient μ was determined as the ratio between the measured frictional force FF and the normal force FN and is displayed as a function of sliding speed μm/s for each test. The tribology tests were performed in triplicate.
Figure 1 illustrates the change in viscosity for the 1 wt% agarose fluid gels with the different sucrose concentrations (0 wt%, 10 wt%, 20 wt% and 30 wt%) as a function of time and temperature. All fluid gel samples exhibit a gelation controlled viscosity increase with decreasing temperature, with similar trends: (i) first, a gradually increasing viscosity (see Table 1) as temperature decreases (85°C–40°C) involving the onset of gelation and the Arrhenius behaviour (between 85°C and 55°C), (ii) followed by a rapid viscosity increase (40°C–25°C), (iii) and finally a plateau region (at 25°C) (Ghebremedhin, Seiffert, and Vilgis, 2021). A small step is observed within the gradual increase in (i) (indicated by arrows), shifting to higher temperatures with higher sucrose concentrations. However, no clear difference can be observed for the fluid gels with 0 wt% and 10 wt% sucrose concentration. Comparing the sharp increase in viscosity in (ii), a barely perceptible earlier increase can be observed for the fluid gel with the highest concentration of 30 wt%. All other samples show a similar steep viscosity increase around 31°C (Ghebremedhin, Seiffert, and Vilgis, 2021). This temperature, the gelation temperature of the agarose used, can be assigned to the aggregation of the associated double helices of the agarose chains into microgel cluster (Norton, Jarvis, and Foster, 1999).
Figure 1. Viscosity profile during the production of 1 wt% agarose fluid gels with varying sucrose concentrations (0 wt%, 10 wt%, 20 wt%, 30 wt%). Samples were subjected to a constant applied shear rate of 400 s−1, cooled from 85°C to 25°C at 1°C/min and then held at 25°C for 15 min. The arrows indicate the step-like increase in viscosity behaviour, which shifts to lower temperature with decreasing sucrose concentration. This shift is attributed to the onset of agarose double helix aggregation, influenced by sucrose interaction with water molecules through hydrogen bonding during the fluid gel formation process.
Table 1. Slope of the gradual increase in viscosity in region (i) with time (refer Figure 1) for 1 wt% agarose with varying sucrose content.
This viscosity range indicates a sol-gel transition of agarose under shear, illustrating a dynamic interplay between gelation through physical cross-linking and shear force-induced limitation of gel growth (De Carvalho and Djabourov, 1997). This process, including of agarose concentration dependence and local microstructure, was comprehensively explored in our previous work (Ghebremedhin, Seiffert, and Vilgis, 2021).
However, a change is expected in the preparation of fluid gels as sugar affects the water of hydration by forming stable hydrogen bonds with water and inhibits helix formation in agarose polymers by removing water molecules from helix cavity (Lee, Debenedetti, and Errington, 2005; Zhao, Ma, and Yang, 2015).
The hydration number of sucrose decreases with increasing sugar concentration and temperature (Gharsallaoui et al., 2008), which in turn affects the hydration of agarose polymers. Due to this indirect interaction, we propose, that when sucrose is added to heated agarose solutions, it competes with fully hydrated agarose polymers for H-bonds with water. This competition decreases with temperature, allowing more non-hydrated sucrose molecules to interact with agarose polymers, restricting their helix formation and reducing water activity. As the temperature decreases, the surrounding sucrose impedes the ordered conformation of the agarose into double helices and their further association. In Figure 1, the transition between the range of expected Arrhenius behaviour and the onset of initial gelation (between 60°C and 50°C) systematically shifts towards higher temperatures with rising sucrose concentration. This step-like increase in shear viscosity (indicated by arrows in Figure 1), which occurs with increasing concentration at higher temperatures, might be attributed to the initiation of the aggregation of agarose double helices. However, this change in viscosity indicates that the strong hydrogen bonding between sucrose and water molecules may influences the process of fluid gel formation. The viscosity increase caused by the addition of co-solutes impedes agarose polymer mobility and diffusion (Maurer, Junghans, and Vilgis, 2012; Russ, Zielbauer, and Vilgis, 2014), which alters the relation between the time scales given by shear rate and molecular relaxation times. A number of studies on the interaction between sucrose and water, using a variety of methods, support the above conclusions (Lee, Debenedetti, and Errington, 2005; Lerbret et al., 2011; Zhao, Ma, and Yang, 2015; Olsson and Swenson, 2020; Tas et al., 2022). The competitive formation of hydrogen bonds with water molecules and agarose chains seems to lead to earlier gel formation at a higher temperature with higher sucrose concentration.
Consequently, the addition of sucrose disturbs the sol-gel transition strongly. Gelation does not proceed properly and as mentioned above, the hydrophobic C-H interactions contribute to the formation of the cavity, allowing higher hierarchical structures, while hydrogen bonds contribute to the stabilization of the cavity. This is likely to result in a more random ordering and double helix formation between the agarose chains and spontaneous multi-helical assemblies, as indicated by the earlier increase in viscosity with increasing sucrose concentration at higher temperatures. This reduced hydration may alter the coil-helix transition, resulting in larger, softer, and weaker particles due to the dominance of randomly arranged cross-links over the shear rates (Fernández Farrés and Norton, 2015).
As shown in previous work (Ghebremedhin, Seiffert, and Vilgis, 2021), the size and structure of the gel particles determines the flow properties of fluid gels and demonstrate the importance of taking this into account. Figure 2 displays microscopic images of the microgel particles of 1 wt% agarose fluid gels with varying sucrose concentrations (0 wt%, 10 wt%, 20 wt% and 30 wt%), revealing irregular microstructures with denser cores and disordered part towards the periphery. It can also be seen that as the sucrose concentration increases, the particle size decreases, whereas the disordered surfaces become less irregular. Observations of the microscope images in our previous studies have already demonstrated that an increase in agarose concentration, accompanied by an increase in the viscosity of the solution, results in a decrease in both particle size and the number of disordered trunks on the surface, referred to as “hairy” parts (Ghebremedhin, Seiffert, and Vilgis, 2021). Therefore, higher viscosity solutions due to sucrose provide more resistance, resulting in reduced shear and the formation of particles with a less hairy structure on the particle surface. Indeed, the trend in particle size and the reduction of disordered parts on the surface with increasing agarose concentration and, consequently, viscosity in sucrose-free systems were discussed in detail using Atomic Force Microscopy, AFM (Ghebremedhin, Seiffert, and Vilgis, 2022).
Figure 2. Optical microscopy images of 1 wt% agarose fluid gel particles with (A–C) 0 wt% sucrose, (D–F) 10 wt% sucrose, (G–I) 20 wt% sucrose and (J–L) 30 wt% sucrose.
Figure 3 shows the concentration dependent particle size distribution at different sucrose concentrations. With increasing concentration, a slight decrease in the median particle size is observed, starting at about 150 μm for the sample without sucrose, and shifting to the smallest size of 100 μm for 30 wt% sucrose. This is in consistent with the observation of the microscope images. However, higher sucrose concentrations result in a broader particle size distribution and the appearance of additional larger particle aggregates. The larger aggregates are most pronounced in the sample with the highest sucrose concentration, clarifying the impact of sucrose on particle formation in fluid gels.
Figure 3. Particle size distribution of 1 wt% agarose fluid gels with different sucrose concentrations.
Additionally, the appearance of a shoulder at larger particle sizes is most likely caused by larger aggregates being caught between the blades of the vane geometry during gelation (Moakes, Sullo, and Norton, 2015).
The mechanism of fluid gel formation involves initial nucleation and growth into microgel particles due to agglomeration of the nucleating particles as they are pushed together during shear flow. The shear forces counteract particle growth, restrict the molecular cross-linking of agarose double helices and prevent further aggregation within the particles (Norton, Jarvis, and Foster, 1999).
However, these findings complement our previous studies on concentration dependence of fluid gel properties. Increased viscosity alters the competition between the imposed shear rate and the molecular diffusion of agarose chains, a competing time scale during the gelation under shear discussed earlier for agarose fluid gels (Ghebremedhin, Seiffert, and Vilgis, 2021). Due to the highly non-equilibrium gelation process of agarose, the competition between the shear rate time scales and the broad spectrum of polymer time scales becomes crucial. The chains diffuse to the lowest approximation according to the Rouse-Zimm model before gelation onset, with the longest relaxation time determined by the center-of-mass diffusion constant DCM in good solvent. DCM and rotational relaxation time are influenced by the molecular weight, which, in turn, is related to the average chain lengths, but also to the viscosity of the solvent (Doi and Edwards, 1988). Therefore, changes in viscosity and diffusion due to co-solvents affect particle size and shape, as evidenced by the variation in particle size with sucrose concentration (see Figure 2).
Additionally, the increase in shoulder and decrease in particle size with increasing sucrose concentration can be further attributed to the reduction in available water required to hydrate the agarose chains and stabilize their tetrahedral arrangement. Consequently, the interplay between hydrogen bond formation involving water, agarose polymers and sucrose molecules, plays a pivotal role in influencing the diversity of particle size, shape, and gel formation. Higher sucrose concentration increases solution viscosity, reducing agarose chain mobility (Valery Normand et al., 2003; Ellis, Mills, and Norton, 2019). This hinders helix nucleation and limits the growth process into bundles, thereby slowing down the gelation kinetics. This viscosity driven effect explains the trends in Figures 2, 3: smaller particle size with wider distribution, and the appearance of a shoulder with increasing sucrose concentration.
The impact of sucrose on the viscoelastic and flow characteristics of agarose fluid gels was investigated using rheological techniques. In Figure 4 amplitude sweep measurements show the storage (G′) and loss (G″) moduli plotted as a function of strain, representing the linear viscoelastic range followed by a nonlinear range.
Figure 4. Amplitude sweep measurement of 1 wt% agarose fluid gels with different sucrose concentrations. Dependence of G′ (filled symbols) and G″ (empty symbols) on strain at constant frequency (ƒ = 1 Hz) and temperature (T = 25°C).
The different samples appear to have similar G′ values within the LVE range. Although not clearly evident in Figure 4, a slight decrease in the storage moduli can be observed with increasing sucrose concentration of the fluid gels. Nevertheless, all samples show elastic and solid-like behaviour in the linear viscoelastic range, with the storage moduli significantly higher than the loss moduli.
The decrease in modulus can be attributed to the competition between agarose and sucrose for H-bond formation with water, reducing local water availability for agarose during cooling, which is crucial for helix formation. At the same time hydrated sucrose may form H-bonds with agarose, i.e., its hydration water, which also limits double helix formation. Therefore, if agarose is dissolved first and sucrose is added, the competitive reaction displaces water as sucrose interacts with agarose during cooling. This inhibits proper subsequent agarose association and aggregation, supported by faster binding of sucrose molecules. Ultimately, entropy plays a crucial role. The gelation process decreases the agarose coils transition into helices, thereby reducing helix aggregation. All these combined effects result in fewer and weaker cross-links, leading to a decrease in G′ moduli. Consequently, sucrose increases viscosity and prevents agarose from forming double helices and aggregation into a dense network. The result is larger aggregates with weaker networks rather than particles with a discrete and narrow size distribution.
To illustrate the forthcoming interpretation, a schematic model of the different fluid gel particles is shown in Figure 5. As examined from the above findings, with increasing sucrose concentration, the particles become smaller with additional aggregate clusters containing fewer and weaker networks, which can be seen in Figures 5A–D.
Figure 5. As sucrose concentration increases the 1 wt% agarose fluid gels, discrete particle sizes show an increase from 0 wt% sucrose (A), 10 wt% sucrose (B), 20 wt% sucrose (C) and 30 wt% sucrose (D). Larger aggregates, forming a weaker network, increase in size from 20 wt% sucrose and 30 wt% sucrose.
In Figure 4, all samples show a local maximum in loss modulus G″ at larger deformation (approximately 0.2%–2% oscillation strain). This overshoot of the loss modulus is typical for soft materials that exhibit yielding behaviour with the characteristic ability to change from solid to liquid-like behaviour at sufficiently high deformation (Donley et al., 2020). The sucrose-free fluid gel displays this local maximum at lower strain compared to those with sucrose, which is addressed to the size of the particles. Higher sucrose concentrations result in softer and larger clusters (see Figure 2L and Figure 3), shifting the overshoot to higher strains required to deform and shear the largest particles. This implies an increased deformation dissipation before segments of the internal structure are deformed and the connectivity between particles is finally disturbed. In addition, the relative motion of regions with free dangling hairy-like structured parts and chain ends, explains the appearance of the G″ overshoot (see Ghebremedhin, Seiffert, and Vilgis, 2021; 2022 for more details). These observations are in agreement with other publications, for example, on soft hydrogel particles, which state that the overshoot results from viscous dissipation due to microstructure destruction and cluster rearrangement (Parthasarathy and Klingenberg, 1999; Sim, Ahn, and Lee, 2003; Wyss et al., 2007).
These ideas are consistent with the different LVE range. The sucrose-free fluid gel exhibits the lowest LVE range, followed by samples with increasing sucrose concentration, aligned with yield points and G″ maxima. Larger aggregate clusters at higher sucrose concentration account for the LVE limits and G″ overshoots at higher strain rates in sucrose-added fluid gels. This is because larger deformations are required to deform and slide the clusters against each other, before they reform, align, and finally break. As the oscillatory strain increases, steric interparticle interactions experience greater deformation and subsequent separation. Simultaneously, the rearrangement of percolation connectivity of the gel particles decreases, disturbing the equilibrium between both processes and causing a decline in interparticle bridges of densely packed gel particles. This indicates a critical region where the particle-particle interactions can no longer reform, causing irreversible damage to the interconnected network of the fluid gel particles.
The nonlinear behaviour of the fluid gels offers further structural insight. At sufficiently large strain amplitudes (
The shear rate dependent viscosity profiles are shown in Figure 6 and confirm the results and ideas of the previous amplitude sweeps and temperature dependent viscosity measurements.
Figure 6. Flow behaviour (Viscosity profile) of 1 wt% agarose fluid gels with different sucrose concentrations, showing (A) ramp up and (B) rump down.
Rotational steady shear experiments cover a wide shear rate range, from high to low rates. Results primarily reflect viscosity properties with prominent shear thinning attributed to particle structure, encompassing the dense core, hairy component, and viscosity of the sugar solution between gel particles. This correlation enhances understanding of the structural response to shear, offering deeper insights as elaborated below.
Furthermore, the extent of hysteresis is assessed using a two-step flow sweep to measure changes in viscosity or its decrease between the two sequences. A rotational flow run with increasing (Figure 6A) and decreasing (Figure 6B) shear rates is conducted, covering shear rates from 0.001 s−1 to 1,000 s−1. The results indicate a shear thinning behaviour in all fluid gel samples. While at first glance, Figure 6A shows no significant differences in the curve profile at low shear rates for sucrose-containing fluid gels, Figure 6B shows an even greater similarity among all samples.
The viscosity curves initially decline gradually at lower shear rates for all samples, due to the increasing alignment of the gel particles. However, once the elastic gel particles align in the shear direction, further orientation is hindered, resulting in a plateau-like curve between 5 s−1–50 s−1. This behaviour is attributed to the prevention of additional particle orientation due to shear-induced alignment (Wolf et al., 2001). Previous studies have investigated the rheological behaviour of particulate gel suspensions based on particle properties such as shape and aggregate state (Tsenoglou, 1990; Zhou, Uhlherr, and Luo, 1995; Wolf et al., 2001). At higher shear rates, the viscosity curves of the various samples remain comparable and exhibit minor deviations. However, a distinct difference can be seen between the shear rates of 1 s−1 and 100 s−1. This plateau-like region, where viscosity changes minimally within the profile, displays an increase in viscosity with rising sucrose content, for both flow steps: rump-up (Figure 6A) and rump-down (Figure 6B). This rise in viscosity due to increased sucrose concentration is noticeable at even lower shear rates, but only during the ramp-up phase (see Figure 6A).
The difference in viscosity between ramp-up and ramp-down at low shear rates is attributed to the increase in larger aggregates with higher sucrose concentration and the impact of a more viscous solution, with the latter being particularly relevant during ramp-up. This effect of the viscous solution is no longer relevant during the ramp-down, as the structural changes that the aggregate particles have undergone due to the previous high shear rates prevail over the influence of the viscous solution. The viscosity trend at low shear rate during ramp-down (Figure 6B) is explained by considering the particle structure and size distribution shown in Figures 2, 3. Hence, the 30 wt% sucrose fluid gel exhibits the highest viscosity, followed by the 20 wt% sucrose fluid gels, consistent with the ramp-up phase and explained by the larger aggregates. In addition, the highly viscous solution of the continuous phase may impede the diffusion of the aggregates, thus preventing the disruption they have previously experienced at high shear rates. In contrast, the higher viscosity of the sucrose-free fluid gel compared to the 10 wt% sucrose fluid gel is ascribed to the larger particle size and higher proportion of protruding tails on the particle surface in the sucrose-free fluid gels. The entanglement and penetration of the tails of the hairy parts leads to increased friction and higher viscosity at very low shear rates (Ghebremedhin, Seiffert, and Vilgis, 2021). For the 0 wt% and 10 wt% sucrose fluid gels, only the effect of the discrete particles with the hairy structures is relevant, as these samples do not contain any larger aggregates. It is also striking that during the first flow step, the 10 wt% and 20 wt% sucrose fluid gel samples run alike viscosity profiles, whereas during the second flow step, the viscosity profiles of the 0 wt% and 10 wt% fluid gel samples run more similarly. This similarity for the downward curves can again be attributed to the absence of larger aggregates and the fact that the gel particles in the 0 wt% and 10 wt% fluid gel samples have gel cores with comparable elasticities.
However, at elevated shear rates, the particle clusters undergo significant deformation as they move past each other, most pronounced at higher steer rates. This is particularly true for the agarose gels prepared with higher sucrose concentrations, which, as the particle size measurements in Figure 3 show, yield larger particle clusters and thus an increase in shear viscosity. Despite variations in aggregate cluster formation, the identical viscosity values of all fluid gel samples at the highest shear rate can be explained by the breakdown of superstructures into their primary microgel particle aggregates. This is expected since the agarose concentration is the same for all samples.
The viscosity curve in Figure 6 remains flattened over a wider shear rate range for the sucrose-free fluid gels, suggesting discrete particles with a narrow size distribution and less aggregation. In Figure 6B, the flatter viscosity curves compared to Figure 6A result from the prior exposure to high shear rates during the initial ramp-up, causing persistent alignment of aggregate particles that influences the downward viscosity curves. However, the significant magnitude of the hysteresis indicates irreparable damage to the aggregates after the first sweep, suggesting that the system did not recover within the specified range before the second sweep. This reconfirms that sucrose-containing fluid gels form larger but weaker aggregates, resulting in higher viscosity with lower connectivity. The hysteresis between the upward and downward curves of viscosity, indicating reduced thixotropic behaviour, implies that these connections cannot be re-established during the downward shear rate (Moakes, Sullo, and Norton, 2015).
The corresponding frequency sweep results are shown in Figure 7. The graph displays the storage (G′) and loss (G″) moduli as a function of frequency at a constant strain (γ = 0.05%). This was performed to further characterize the mechanical response under small oscillation strain, which covers the range of non-destructive deformations, and to describe their time-dependent behaviour.
Figure 7. Frequency dependence of storage moduli G′ (filled symbols) and loss moduli G″ (empty symbols) at a constant strain (γ = 0.05%) and temperature (T = 25°C) for 1 wt% agarose fluid gels with different sucrose concentrations. Dashed lines indicate the angular frequency (ωc) where G″ starts to increase for the respective concentrations.
The moduli of the fluid gels show a slight rise with increasing frequency, linked to faster particle movement at higher frequencies (Ghebremedhin, Seiffert, and Vilgis, 2021). As densely packed gel particles are confined in a cage, the linear rise in both moduli is attributed to particle motion, taking into account difference in structure and size distribution (Figures 2, 3). The rise in storage modulus with increasing frequency is less pronounced when the sucrose concentration is reduced. This indicates that the sucrose free sample is less frequency dependent, followed by the fluid gels with 10 wt%, 20 wt%, and finally 30 wt% sucrose. The lower frequency dependence can be explained by the discrete gel particles with a narrow size distribution, leading to their dense packing and higher percolation. This applies in particular to the 0 wt% but also to the 10 wt% sucrose fluid gels. Conversely, this is not the case for the fluid gels containing 20 wt% and 30 wt% sucrose, where the larger aggregates have less continuous connectivity and a weaker network, indicating that the cross-links are incoherently weaker and less ordered. In addition, the contribution of the viscosity of the sugar solution between the particles becomes more significant at higher concentrations, limiting the movement of the particles with the dense core and the hairy parts at the periphery. Therefore, this effect is less relevant when the sucrose concentration is reduced.
The increase in loss modulus (G″) with the frequency can be attributed to the specific structure of the particle surface. As seen in the micrographs in Figure 2, the “hairy” structural components decrease with increasing sucrose concentration. This results in a systematically more pronounced G″ increases starting at the angular frequency (ωc) (as indicated by the dashed lines) for the fluid gels with 0 wt% sucrose concentration, followed by 10 wt% sucrose fluid gel and so on (Ghebremedhin, Seiffert, and Vilgis, 2021; 2022). The delayed rise in loss modulus with increasing sucrose concentration can also be attributed to the larger aggregates in fluid gel particles, which move slower due to molecular weight and respond on slower time scales, requiring a higher frequency to respond properly. The observed increase in loss modulus (G″) at very low frequencies is attributed to the incoherently weak network of larger aggregate clusters, which are more prone to rupture. This aligns with the decrease in storage modulus (G′) with increasing sucrose concentration.
All rheological measurements confirmed that increasing sucrose content in fluid gels leads to the formation of larger but weaker aggregate clusters, as evidenced by the data in Figure 7 and the decreased storage (G′) moduli. The flow sweep exhibits the highest viscosity for the 30 wt% sucrose fluid gel, particularly pronounced at medium shear rates (5 s−1–50 s−1), suggesting the presence of large particle aggregates sliding against each other. This is further supported by the humps at large oscillations in the amplitude sweeps (Figure 4). Although it might be assumed that the fluid gel containing 30 wt% sucrose would show the highest moduli in the amplitude sweep and frequency sweep measurements due to the largest aggregates, our observations contradict this. Fluid gels are formed with a less discrete particle size distribution and a less densely packed core with loosely connected aggregate domains. Consequently, larger cluster of aggregate are expected to form after the formation of gel particles with a size of approximately 100 μm size is complete.
Lubrication measurements were carried out to investigate the coefficient of friction of the agarose fluid gels as a function of sucrose content. Here, the lubrication between interacting surfaces and the behaviour of the fluid gels in a thin layer were investigated using tribology tests (Stokes, Boehm, and Baier, 2013). Our findings support those obtained from previous bulk examinations. Figure 8 shows the Stribeck curves with the friction coefficient as a function of the sliding speed conducted from 100 to 1,000,000 μm/s.
Figure 8. Stribeck curves at a normal load of 3 N for 1 wt% agarose fluid gels with different sucrose concentrations.
While the fluid gels show different overall curves, they share some common characteristics that can be related to their microstructure. All samples show an increased coefficient of friction at lower sliding speeds in the boundary regime, except for the 10 wt% sucrose sample. This is generally due to exclusion of large particles from the narrow gap between the contact surfaces, which reduces lubrication and increases contact between the plate and spheres, thereby raising friction (Baier et al., 2009; Gabriele, Spyropoulos, and Norton, 2010; Fernández Farrés and Norton, 2015). However, the peak in the friction coefficient between 2 × 102 and 4 × 102 μm/s can be ascribed to the entrainment of a few particles small enough to enter the small gap between the two surfaces (Gabriele et al., 2010).
At sliding speeds above 3 × 102 μm/s, the coefficient of friction decreases for all samples in the mixed regime. In this regime, a thin layer of fluid gel particles separates the surfaces, thickened by bulk entrainment, leading to minimal surface contact. This improves lubrication, resulting in lower friction coefficients. However, as the sliding speed continues to increase, a point is reached where the decrease in friction stops, and for the fluid gel containing 30 wt% sucrose, friction increases slightly. In this hydrodynamic regime, the friction coefficient and the separation distance between surfaces increases as the entrainment speed of the fluid gel lubrication rises. This is due to the hydrodynamic pressure generated by the flow of the lubricant (Baier et al., 2009; Shewan, Pradal, and Stokes, 2019).
Notably, the fluid gel containing 10 wt% sucrose exhibits the lowest coefficient of friction in the boundary regime due to the smaller particles and weaker, less hairy surfaces compared to the 0 wt% sucrose fluid gel (see Figures 2, 3, 5). The higher friction of the sucrose-free samples can be attributed to larger particles containing more pronounced hairy surfaces (see Figures 3, 5A), which increase the interaction between the particles and result in higher friction coefficients. The increase and maximum friction in the boundary regime are influenced by the micro particle entrainment in the gap between the surfaces (as illustrated in Figure 9). These larger particles also contribute to a lower coefficient of friction in the mixed regime of the 0 wt% sucrose fluid gel (Figure 9A) compared to the 10 wt% sucrose fluid gel (Figure 9B). This is because larger particles increase the separation between the two surfaces, resulting in reduced contact between them (Rudge et al., 2020). This effect is especially evident at the higher sliding speed when the lubrication flow starts and the thin layer samples thickened by entrainment, causing minimal surface contact by separating them from each other.
Figure 9. Schematic illustration of the proposed behaviour of 1 wt% agarose fluid gels with different sucrose concentration (A) 0 wt%, (B) 10 wt%, (C) 20 wt% and (D) 30 wt% sucrose, in the boundary regime of a Stribeck curve between the two surfaces (stainless steel ball and silicon sheet).
The highest coefficient of friction in the boundary regime is observed for the agarose fluid gels with 30 wt% sucrose, attributed to the presence of additional larger aggregates (Figure 3). The exclusion of these aggregates from the narrow gap between the two contact surfaces results in an accumulation of particles around the contact region, preventing smaller particles from being entrained (illustrated in Figure 9D). This leads to high friction due to poor lubrication and nearly dry contact. The extended boundary regime may also be due to the build-up of large aggregates, requiring faster sliding speeds for these larger aggregates to enter the gap. However, the steep drop in friction could be explained by the rupture of the weaker larger clusters at increasing sliding speed. These smaller ruptured fragments are able to enter the gap with the lubricant flow. Furthermore, Fernandez et al. (2015) found that agar fluid gels with co-solutes have larger and softer particles that are unable to separate the two surfaces due to their low elasticity, resulting in a higher friction coefficient in the boundary regime (Fernandez et al., 2015). This is consistent with the present result, indicating larger but weaker network structures at higher sucrose concentrations. The friction coefficient decreases sharply in the mixed regime as more larger aggregates enter the gap with higher sliding speeds, resulting in the lower friction at a sliding speed of around 4 × 104 and 2 × 105 μm/s.
In the case of the 20 wt% sucrose fluid gel, a friction peak appears in the boundary region at the same particle entrainment speed as the other fluid gels. Particle size measurements revealed larger aggregates, although smaller than those in the 30 wt% sucrose fluid gels, resulting in lower friction coefficients (illustrated in Figure 9C). In addition, the friction gradually decreases as the sliding speed increases. Although the reduction is less pronounced compared to the 30 wt% sample, it remains steeper than for the other fluid gels. This behaviour is attributed to the presence of intermediate larger aggregates that can enter the gap and keep the surfaces apart.
Using an alternative preparation method, 20 wt% sucrose was first dissolved in Milli-Q water, followed by the addition of agarose. This approach aims to compare how the order of dissolution of sucrose and agarose influences the behaviour of fluid gels and their preparation. The results are discussed in this chapter and corresponding graphs are provided in the Supplementary Material.
The viscosity profile of the 1 wt% agarose fluid gel with 20 wt% sucrose, prepared using the alternative method, is shown in Supplementary Figure S1. Similar trend can be observed, but with an earlier viscosity increase in both temperature regimes, 85°C–40°C (i) and 40°C–25°C (ii). Microscopic images in Supplementary Figure S2 show that the alternative preparation (Supplementary Figure S2B) results in particles with homogenously smaller sizes compared to the standard preparation (Supplementary Figure S2A). This was corroborated by particle size measurements (see Supplementary Figure S3), which revealed a decrease in mean particle size for the alternatively prepared fluid gel. Amplitude sweep measurements showed an increase in G′ for the alternatively preparation (shown in Supplementary Figure S4). This can be explained by enhanced particle interaction between smaller particles, resulting in more interconnected network due to the increased packing density (Ghebremedhin, Seiffert, and Vilgis, 2021). Frequency sweep tests (Supplementary Figure S6) reveal similar trends for storage (G′) and loss (G″) moduli, with the alternative preparation showing higher storage modulus (G′) values. The homogenously smaller microgel particles result in a slight increase in shear viscosity (shown in Supplementary Figure S5A, B). This observation align with the explanation for the increase in the storage (G′) modulus in the amplitude sweep measurements (see Supplementary Figure S4). Moreover, the Stribeck curves of the tribological measurements (see. Supplementary Figure S7) display a lower coefficient of friction for the alternatively prepared fluid gel in the boundary and mixed regime. This is due to the higher particle elasticity and the fact that smaller gel particles result in a more interconnected network, denser packing and higher percolation.
A molecular explanation for the increase in storage modulus (G′) relies on the formation of strong and stable hydrogen bonds between sucrose and water molecules. This reduced availability of water for agarose chains leads to the formation of a closed-meshed network. The prior complete dissolution of sucrose, and thus its fully formed hydration shell, results in the absence of sucrose molecules that would otherwise interfere with the helix formation and aggregation of agarose chains. Consequently, the storage modulus increases due to the exclusion of completely hydrated sucrose, leading to an increased local concentration of agarose within its phase.
As the hydration number of sucrose decreases with temperature, this temperature dependence may also be relevant to the various preparation methods and the resulting fluid gels (Gharsallaoui et al., 2008). This is because higher temperatures weaken the hydrogen bonds between water and sucrose molecules, leading to a reduction in the hydration number (Starzak, Peacock, and Mathlouthi, 2000; Starzak and Mathlouthi, 2006; Zhao, Ma, and Yang, 2015). The weakening of the hydrogen bond network at higher temperatures accounts for the expected changes (Zhao, Ma, and Yang, 2015).
This suggests that for the alternative fluid gel preparation when sucrose is first dissolved at ambient temperature, the subsequent high temperature used to initiate gelation has a lesser impact on its hydration number compared to the preparation when sucrose is added to the heated biopolymer solution. In the former, agarose is added to a system with less available free water, which increases the elasticity through local concentration. Consequently, depending on whether sucrose is first dissolved in water or agarose, it changes their interaction and influences the association of the agarose chains and, thus their gelation process during fluid gel preparation. To illustrate the different dissolution orders, a schematic model is shown in Figure 10. However, as mentioned in the introduction, the sol-gel transition, and thus the transition from coil to helix of the agarose chains, exists in multiple of hierarchical structures and appears to be complex. Recent studies on agarose-based fluid gels have successfully revealed thick bundles with helical assembly at the periphery of these particle surfaces using atomic force microscopy, and were discussed in detail (Ghebremedhin, Seiffert, and Vilgis, 2022). It was clearly shown that the associated helices assemble into a multitude of helices showing thick strands that change with agarose concentration. The height profile allowed clear visualisation and distinction of individual strands, showing a higher ordered aggregate at higher agarose concentrations. Therefore, based on these findings and our results on macroscopic rheological properties, supported by publications on microscopic length scales discussed throughout this work, Figure 10 is a simplified representation. However, this model provides crucial insights into the effect of sucrose on the resulting fluid gels.
Figure 10. Schematic model illustrating the effect of the different order of dissolution of agarose and sucrose on the network structure of the fluid gels. Fig (A) shows the preparation in which agarose is dissolved first, followed by the addition of sucrose, which leads to competition between agarose and sucrose for H-bond formation with water (B). This results in a lack of water for the cavity, crucial for double helix formation and their aggregation. Consequently, fewer cross-links are formed, resulting in a weak network structure (C). When sucrose is first dissolved and fully hydrated (D), this reduces the availability of water for the agarose chain during cooling. The exclusion of fully hydrated sucrose leads to an increased local concentration of agarose within its phase (E) and the formation of a closed mesh network due to increased cross-linking (F).
Figure 10 summarizes the initial high entropic coil state and the final gel state according to the present literature. In contrast to charged systems, as discussed in Schefer, Adamcik, and Mezzenga (2014), Schefer et al. (2015a), Schefer, Usov, and Mezzenga (2015b), Diener et al. (2019), (2020), Tavagnacco et al. (2023) for carrageenan and gellan, where different intermediate states are possible during gelation as long as ions (salts) are added, for uncharged, polar agarose, such intermediate states as single helices have not been reported in the literature so far. The gelation process is likely initiated through double helices. A reason for this could be that highly flexible agarose chains in good solvent (water) are entropy and excluded volume dominated, and the polar and hydrogen bonding interactions are too weak to overcome the required entropy loss for complex secondary structures. The addition of sucrose mainly changes the apparent water concentration to first order. Still, the short-range dipolar and hydrogen bonds are seemingly not strong enough to reduce the entropy of single chains to fold into an intramolecular double helix with a hairpin-like structure.
In this work, we have investigated the effect of sucrose addition on the physical-chemical properties of agarose fluid gels, with the aim of relating the resulting structural changes to the rheological and tribological properties.
Based on the current findings, it appears that the presence of sucrose affects the interplay between gelation and breakup during shear. As a result, gelation is hindered by the impaired diffusion of agarose chains, leading to less defined and discrete gel particles with a broader size distribution and larger aggregates, characterized by weaker and less stable network structures. Roughly speaking, the addition of sucrose has two main effects: First, the “hairy” particles become slightly smaller with increasing viscosity as the sucrose concentration increases. Second, the reduced availability of water to stabilise the agarose double helix allows for the formation of larger and less stable gel particles with increasing sucrose concentration. These changes in network structure could be related to rheological and lubrication properties. It was found that increasing the sucrose content, which leads to reduced connectivity between the larger aggregate particles, results in a decrease in storage (G′) moduli but an increase in viscosity, as shown by the flow sweep test. These larger aggregates were responsible for the higher coefficient of friction in the boundary regime as they were excluded from the narrow gap preventing the smaller particles from being entrained between the surfaces up to a certain sliding speed.
Furthermore, the interaction between sugar and water molecules in hydrocolloid solutions is also primarily entropy-driven. Whether this effect is particularly pronounced when sucrose or agarose is dissolved in advance requires clarification. Therefore, aspects influencing entropic effects, including molecular flexibility, conformational changes, and solvent effects, need to be considered. The impact of dissolving sucrose and agarose in different orders on the changes in the molecular conformation of agarose was discussed in detail. The binding of sugar molecules with water in their hydration shell reduces the availability of free water surrounding the agarose polymers. This, in turn, leads to stronger interactions between the agarose chains when sucrose is first dissolved and may promote the formation of helical structures, due to locally increased concentration. However, it can be concluded that the interaction between the agarose and sucrose molecules is primarily indirect, involving hydrogen bonding, which has a noticeable impact on the fluid gel network. It can be suggested that the addition of sucrose influences the rheological and mechanical properties by affecting the hydration shell surrounding the agarose molecules during the gelation process. This consequent change in the hydration environment is considered to be a key factor in the observed changes in gel properties. Overall, the findings of this study demonstrate the ability to modify edible soft matter systems to alter textural properties for further potential applications. Additionally, they provide a deeper understanding of the complex interactions within fluid hydrogels.
The raw data supporting the conclusion of this article will be made available by the authors, without undue reservation.
MG: Conceptualization, Investigation, Methodology, Validation, Visualization, Writing–original draft, Writing–review and editing. SS: Conceptualization, Supervision, Writing–review and editing. TV: Conceptualization, Supervision, Writing–review and editing, Writing–original draft, Visualization.
The author(s) declare that no financial support was received for the research, authorship, and/or publication of this article.
The authors would like to thank Dr. Kaloian Koynov and especially Andreas Hanewald for the technical support during rheology and tribology experiments. Furthermore, we thank the members of the MPIP soft matter food science group for fruitful discussion and proofreading the manuscript.
The authors declare that the research was conducted in the absence of any commercial or financial relationships that could be construed as a potential conflict of interest.
The author(s) declared that they were an editorial board member of Frontiers, at the time of submission. This had no impact on the peer review process and the final decision.
All claims expressed in this article are solely those of the authors and do not necessarily represent those of their affiliated organizations, or those of the publisher, the editors and the reviewers. Any product that may be evaluated in this article, or claim that may be made by its manufacturer, is not guaranteed or endorsed by the publisher.
The Supplementary Material for this article can be found online at: https://www.frontiersin.org/articles/10.3389/frsfm.2024.1363898/full#supplementary-material
Ablett, S., Lillford, P. J., Baghdadi, S. M. A., and Derbyshire, W. (1978). Nuclear magnetic resonance investigations of polysaccharide films, sols, and gels. J. Colloid Interface Sci. 67 (2), 355–377. doi:10.1016/0021-9797(78)90020-6
Arnott, S., Fulmer, A., Scott, W., Dea, I., Moorhouse, R., and Rees, D. (1974). The agarose double helix and its function in agarose gel structure. J. Mol. Biol. 90 (2), 269–284. doi:10.1016/0022-2836(74)90372-6
Baier, S., Elmore, D., Guthrie, B., Lindgren, T., Smith, S., Steinbach, A., et al. (2009). “A new tribology device for assessing mouthfeel attributes of foods,” in 5th International Symposium on Food Structure and Rheology, 432–435. (ETH Zurich: Switzerland).
De Carvalho, W., and Djabourov, M. (1997). Physical gelation under shear for gelatin gels. Rheol. Acta 36 (6), 591–609. doi:10.1007/s003970050074
Deszczynski, M., Kasapis, S., MacNaughton, W., and Mitchell, J. R. (2003a). Effect of sugars on the mechanical and thermal properties of agarose gels. Food Hydrocoll. 17 (6), 793–799. doi:10.1016/s0268-005x(03)00100-0
Deszczynski, M., Kasapis, S., and Mitchell, J. R. (2003b). Rheological investigation of the structural properties and aging effects in the agarose/Co-solute mixture. Carbohydr. Polym. 53, 85–93. doi:10.1016/s0144-8617(02)00327-2
Diener, M., Adamcik, J., Bergfreund, J., Catalini, S., Fischer, P., and Mezzenga, R. (2020). Rigid, fibrillar quaternary structures induced by divalent ions in a carboxylated linear polysaccharide. ACS Macro Lett. 9 (1), 115–121. doi:10.1021/acsmacrolett.9b00824
Diener, M., Adamcik, J., Sánchez-Ferrer, A., Jaedig, F., Schefer, L., and Mezzenga, R. (2019). Primary, secondary, tertiary and quaternary structure levels in linear polysaccharides: from random coil, to single helix to supramolecular assembly. Biomacromolecules 20 (4), 1731–1739. doi:10.1021/acs.biomac.9b00087
Doi, M., and Edwards, S. F. (1988). The theory of polymer dynamics. Vol. 73. Oxford, United Kingdom: Oxford University Press.
Donley, G. J., Singh, P. K., Shetty, A., and Rogers, S. A. (2020). Elucidating the G? Overshoot in soft materials with a yield transition via a time-resolved experimental strain decomposition. Proc. Natl. Acad. Sci. U. S. A. 117 (36), 21945–21952. doi:10.1073/pnas.2003869117
Ellis, A. L., Mills, T. B., Norton, I. T., and Norton-Welch, A. (2019). The effect of sugars on agar fluid gels and the stabilisation of their foams. Food Hydrocoll. 87, 371–381. August 2018. doi:10.1016/j.foodhyd.2018.08.027
Farrés, , Fernández, I., Moakes, R. J. A., and Norton, I. T. (2014). Food hydrocolloids designing biopolymer fluid gels: a microstructural approach. Food Hydrocoll. 42, 362–372. doi:10.1016/j.foodhyd.2014.03.014
Fernández Farrés, I., and Norton, I. T. (2015). The influence of Co-solutes on tribology of agar fluid gels. Food Hydrocoll. 45, 186–195. doi:10.1016/j.foodhyd.2014.11.014
Fittolani, G., Seeberger, P. H., and Delbianco, M. (2020). Helical polysaccharides. Peptide Sci. 112 (1). doi:10.1002/pep2.24124
Frith, W. J., Garijo, X., Foster, T. J., and Norton, I. T. (2002). Microstructural origins of the rheology of fluid gels. Special publication-royal society of chemistry 278, 95–103.
Gabriele, A., Spyropoulos, F., and Norton, I. T. (2010). A conceptual model for fluid gel lubrication. Soft Matter 6 (17), 4205–4213. doi:10.1039/c001907k
Gharsallaoui, A., Rogé, B., Génotelle, J., and Mohamed, M. (2008). Relationships between hydration number, water activity and density of aqueous sugar solutions. Food Chem. 106, 1443–1453. 4 SPEC. ISS. doi:10.1016/j.foodchem.2007.02.047
Ghebremedhin, M., Seiffert, S., and Vilgis, T. A. (2021). Physics of agarose fluid gels: rheological properties and microstructure. Curr. Res. Food Sci. 4, 436–448. December 2020. doi:10.1016/j.crfs.2021.06.003
Ghebremedhin, M., Seiffert, S., and Vilgis, T. A. (2022). Molecular behavior of fluid gels - the crucial role of edges and particle surface in macroscopic properties. Food Funct. 13 (13), 6902–6922. doi:10.1039/d2fo00102k
He, Qi, Bramante, F., Davies, A., Elleman, C., Fourtouni, K., and Wolf, B. (2018). Material properties of ex vivo milk chocolate boluses examined in relation to texture perception. Food Funct. 9 (6), 3532–3546. doi:10.1039/c8fo00548f
Hédoux, A., Willart, J. F., Ionov, R., Affouard, F., Guinet, Y., Paccou, L., et al. (2006). Analysis of sugar bioprotective mechanisms on the thermal denaturation of lysozyme from Raman scattering and differential scanning calorimetry investigations. J. Phys. Chem. B 110 (45), 22886–22893. doi:10.1021/jp061568i
Kasapis, S., Al-Marhoobi, I. M., Deszczynski, M., Mitchell, J. R., and Abeysekera, R. (2003). Gelatin vs polysaccharide in mixture with sugar. Biomacromolecules 4 (5), 1142–1149. doi:10.1021/bm0201237
Laiho, S., Williams, R. P., Poelman, A., Appelqvist, I., and Logan, A. (2017). Effect of whey protein phase volume on the tribology, rheology and sensory properties of fat-free stirred yoghurts. Food Hydrocoll. 67, 166–177. doi:10.1016/j.foodhyd.2017.01.017
Lee, S. L., Debenedetti, P. G., and Errington, J. R. (2005). A computational study of hydration, solution structure, and dynamics in dilute carbohydrate solutions. J. Chem. Phys. 122 (20), 204511. doi:10.1063/1.1917745
Lerbret, A., Affouard, F., Bordat, P., Hédoux, A., Guinet, Y., and Descamps, M. (2011). Slowing down of water dynamics in disaccharide aqueous solutions. J. Non-Crystalline Solids 357 (2), 695–699. doi:10.1016/j.jnoncrysol.2010.05.092
Lerbret, A., Bordat, P., Affouard, F., Hédoux, A., Guinet, Y., and Descamps, M. (2007). How do trehalose, maltose, and sucrose influence some structural and dynamical properties of lysozyme? Insight from molecular dynamics simulations. J. Phys. Chem. B 111 (31), 9410–9420. doi:10.1021/jp071946z
Martínez-Sanz, M., Ström, A., Lopez-Sanchez, P., Knutsen, S. H., Ballance, S., Zobel, H. K., et al. (2020). Advanced structural characterisation of agar-based hydrogels: rheological and small angle scattering studies. Carbohydr. Polym. 236, 115655. February. doi:10.1016/j.carbpol.2019.115655
Maurer, S., Junghans, A., and Vilgis, T. A. (2012). Impact of xanthan gum, sucrose and fructose on the viscoelastic properties of agarose hydrogels. Food Hydrocoll. 29 (2), 298–307. doi:10.1016/j.foodhyd.2012.03.002
Moakes, R. J. A., Sullo, A., and Norton, I. T. (2015). Preparation and characterisation of whey protein fluid gels: the effects of shear and thermal history. Food Hydrocoll. 45, 227–235. doi:10.1016/j.foodhyd.2014.11.024
Nguyen, P. T. M., Kravchuk, O., Bhandari, B., and Prakash, S. (2017). Effect of different hydrocolloids on texture, rheology, tribology and sensory perception of texture and mouthfeel of low-fat pot-set yoghurt. Food Hydrocoll. 72, 90–104. doi:10.1016/j.foodhyd.2017.05.035
Nishinari, K., Watase, M., Kohyama, K., Nishinari, N., Oakenfull, D., Koide, S., et al. (1992). The effect of sucrose on the thermo-reversible gel-sol transition in agarose and gelatin. Polym. J. 24 (9), 871–877. doi:10.1295/polymj.24.871
Nordqvist, D., and Vilgis, T. A. (2011). Rheological study of the gelation process of agarose-based solutions. Food Biophys. 6, 450–460. doi:10.1007/s11483-011-9225-0
Normand, V., Aymard, P., Lootens, D. L., Amici, E., Plucknett, K. P., and Frith, W. J. (2003). Effect of sucrose on agarose gels mechanical behaviour. Carbohydr. Polym. 54 (1), 83–95. doi:10.1016/s0144-8617(03)00153-x
Normand, V., Lootens, D. L., Amici, E., Plucknett, K. P., and Aymard, P. (2000). New insight into agarose gel mechanical properties. Biomacromolecules 1 (4), 730–738. doi:10.1021/bm005583j
Norton, I. T., Jarvis, D. A., and Foster, T. J. (1999). A molecular model for the formation and properties of fluid gels. Int. J. Biol. Macromol. 26 (4), 255–261. doi:10.1016/s0141-8130(99)00091-4
Nussinovitch, A. (1997). Hydrocolloid applications: gum technology in the food and other industries. Springer.
Olsson, C., and Jan, S. (2020). Structural comparison between sucrose and trehalose in aqueous solution. J. Phys. Chem. B 124 (15), 3074–3082. doi:10.1021/acs.jpcb.9b09701
Parthasarathy, M., and Klingenberg, D. J. (1999). Large amplitude oscillatory shear of ER suspensions. J. Newt. Fluid Mech. 81 (1–2), 83–104. doi:10.1016/s0377-0257(98)00096-2
Rudge, R. E. D., Jesse, P. M. V.De S., Dijksman, J. A., and Scholten, E. (2020). Uncovering friction dynamics using hydrogel particles as soft ball bearings. Soft Matter 16 (15), 3821–3831. doi:10.1039/D0SM00080A
Russ, N., Zielbauer, B. I., Koynov, K., and Vilgis, T. A. (2013). Influence of nongelling hydrocolloids on the gelation of agarose. Biomacromolecules 14 (11), 4116–4124. doi:10.1021/bm4012776
Russ, N., Zielbauer, B. I., and Vilgis, T. A. (2014). Impact of sucrose and trehalose on different agarose-hydrocolloid systems. Food Hydrocoll. 41, 44–52. doi:10.1016/j.foodhyd.2014.03.020
Schefer, L., Adamcik, J., Diener, M., and Mezzenga, R. (2015a). Supramolecular chiral self-assembly and supercoiling behavior of carrageenans at varying salt conditions. Nanoscale 7 (39), 16182–16188. doi:10.1039/c5nr04525h
Schefer, L., Adamcik, J., and Mezzenga, R. (2014). Unravelling secondary structure changes on individual anionic polysaccharide chains by atomic force microscopy. Angew. Chem. - Int. Ed. 53 (21), 5376–5379. doi:10.1002/anie.201402855
Schefer, L., Usov, I., and Mezzenga, R. (2015b). Anomalous stiffening and ion-induced coil-helix transition of carrageenans under monovalent salt conditions. Biomacromolecules 16 (3), 985–991. doi:10.1021/bm501874k
Shewan, H. M., Pradal, C., and Stokes, J. R. (2019). Tribology and its growing use toward the study of food oral processing and sensory perception. J. Texture Stud. 51, 7–22. May. doi:10.1111/jtxs.12452
Shimizu, S., and Matubayasi, N. (2014). Gelation: the role of sugars and polyols on gelatin and agarose.
Sim, H. G., Ahn, K. H., and Lee, S. J. (2003). Three-dimensional dynamics simulation of electrorheological fluids under large amplitude oscillatory shear flow. J. Rheology 47 (4), 879–895. doi:10.1122/1.1582854
Starzak, M., and Mohamed, M. (2006). Temperature dependence of water activity in aqueous solutions of sucrose. Food Chem. 96 (3), 346–370. doi:10.1016/j.foodchem.2005.02.052
Starzak, M., Peacock, S. D., and Mathlouthi, M. (2000). Hydration number and water activity models for the sucrose-water system: a critical review. Crit. Rev. Food Sci. Nutr. 40 (4), 327–367. doi:10.1080/10408690091189185
Stokes, J. R., Boehm, M. W., and Baier, S. K. (2013). Oral processing, texture and mouthfeel: from rheology to tribology and beyond. Curr. Opin. Colloid Interface Sci. 18 (4), 349–359. doi:10.1016/j.cocis.2013.04.010
Tako, M., Teruya, T., Tamaki, Y., Uechi, K., and Konishi, T. (2021). Molecular origin for strong agarose gels: multi-stranded hydrogen bonding molecular origin for strong agarose gels: multi-stranded hydrogen bonding. J. Polym. Biopolymer Phys. Chem. 9 (1), 13–19. doi:10.12691/jpbpc-9-1-2
Tas, O., Ertugrul, U., Grunin, L., and Oztop, M. H. (2022). Investigation of the hydration behavior of different sugars by time domain-NMR. Foods 11 (8), 1148. doi:10.3390/foods11081148
Tavagnacco, L., Chiessi, E., Severini, L., Franco, S., Buratti, E., Capocefalo, A., et al. (2023). Molecular origin of the two-step mechanism of gellan aggregation. Sci. Adv. 9 (10), 1–11. doi:10.1126/sciadv.adg4392
Tsenoglou, C. (1990). Scaling concepts in suspension rheology. J. Rheology 34 (1), 15–24. doi:10.1122/1.550120
Vilgis, T. A. (2015a). Gels: model systems for soft matter food physics. Curr. Opin. Food Sci. 3, 71–84. doi:10.1016/j.cofs.2015.05.009
Watase, M., Kohyama, K., and Nishinari, I. L. (1992). Effects of sugars and polyols on the gel-sol transition of agarose by differential scanning calorimetry. Adv. Exp. Med. Biol. 206, 163–173. doi:10.1016/0040-6031(92)85294-6
Watase, M., Nishinari, K., and Hatakeyama, T. (1988). DSC study on properties of water in concentrated agarose gels. Food Hydrocoll. 2 (6), 427–438. doi:10.1016/s0268-005x(88)80043-2
Watase, M., Nishinari, K., Williams, P. A., and Phillips, G. O. (1990). Agarose gels: effect of sucrose, glucose, urea, and guanidine hydrochloride on the rheological and thermal properties *. J. Agric. Food Chem. 38, 1181–1187. doi:10.1021/jf00095a005
Williams, P. A., Day, D., Langdon, M., Phillips, G., and Nishinari, K. (1991). Synergistic interaction of xanthan gum with glucomannans and galactomannans. Top. Catal. 4 (6), 489–493. doi:10.1016/s0268-005x(09)80199-9
Wolf, B., Frith, W. J., Singleton, S., Tassieri, M., and Norton, I. T. (2001). Shear behaviour of biopolymer suspensions with spheroidal and cylindrical particles. Rheol. Acta 40 (3), 238–247. doi:10.1007/s003970000133
Wyss, H. M., Miyazaki, K., Mattsson, J., Hu, Z., Reichman, D. R., and Weitz, D. A. (2007). Strain-rate frequency superposition: a rheological probe of structural relaxation in soft materials. Phys. Rev. Lett. 98 (23), 238303. doi:10.1103/physrevlett.98.238303
Xiong, J. Y., Narayanan, J., Liu, X. Y., Chong, T. K., Chen, S. B., and Chung, T. S. (2005). Topology evolution and gelation mechanism of agarose gel. J. Phys. Chem. B 109 (12), 5638–5643. doi:10.1021/jp044473u
Yang, K., Wang, Z., Brenner, T., Kikuzaki, H., Fang, Y., and Nishinari, K. (2015). Sucrose release from agar gels: effects of dissolution order and the network inhomogeneity. Food Hydrocoll. 43, 100–106. doi:10.1016/j.foodhyd.2014.05.005
Zhao, L., Ma, K., and Yang, Zi (2015). Changes of water hydrogen bond network with different externalities. Int. J. Mol. Sci. 16 (4), 8454–8489. doi:10.3390/ijms16048454
Keywords: agarose, fluid gels, microgel particles, physical gelation, rheology, tribology, sucrose, microstructure
Citation: Ghebremedhin M, Seiffert S and Vilgis TA (2024) Effects of sugar molecules on the rheological and tribological properties and on the microstructure of agarose-based fluid gels. Front. Soft Matter 4:1363898. doi: 10.3389/frsfm.2024.1363898
Received: 31 December 2023; Accepted: 08 April 2024;
Published: 26 April 2024.
Edited by:
Lester Geonzon, The University of Tokyo, JapanReviewed by:
Luben Arnaudov, Unilever’s Foods Innovation Centre, NetherlandsCopyright © 2024 Ghebremedhin, Seiffert and Vilgis. This is an open-access article distributed under the terms of the Creative Commons Attribution License (CC BY). The use, distribution or reproduction in other forums is permitted, provided the original author(s) and the copyright owner(s) are credited and that the original publication in this journal is cited, in accordance with accepted academic practice. No use, distribution or reproduction is permitted which does not comply with these terms.
*Correspondence: Marta Ghebremedhin, Z2hlYnJlQG1waXAtbWFpbnoubXBnLmRl; Thomas A. Vilgis, dmlsZ2lzQG1waXAtbWFpbnoubXBnLmRl
Disclaimer: All claims expressed in this article are solely those of the authors and do not necessarily represent those of their affiliated organizations, or those of the publisher, the editors and the reviewers. Any product that may be evaluated in this article or claim that may be made by its manufacturer is not guaranteed or endorsed by the publisher.
Research integrity at Frontiers
Learn more about the work of our research integrity team to safeguard the quality of each article we publish.