- Redefine Meat Ltd., Rehovot, Israel
The escalating environmental impact of traditional livestock farming, particularly beef production, has spurred the search for sustainable meat alternatives. This study introduces a novel Plant-Based Tissue Engineering (PBTE) approach, to replicate the complex structure and sensory experience of whole-muscle cuts of meat using plant-based ingredients. Leveraging principles of tissue engineering and advanced food manufacturing technologies, PBTE deconstructs meat into its fundamental components: muscle, fat, and connective tissue, and reconstructs them using a combination of plant proteins, fats and polysaccharide materials. The muscle component is reassembled to mimic the anisotropic fibrous structure of beef, while the fat component is engineered through lipid encapsulation within a hydrocolloid matrix. Advanced manufacturing techniques, including additive manufacturing and robotics, are utilized for precise spatial configuration and assembly of these components. Our findings demonstrate that PBTE can effectively replicate the mechanical integrity, texture, and sensory attributes of traditional meat, presenting a promising alternative that could significantly reduce the environmental footprint of meat production. This approach aligns with the principles of Soft Matter in the manipulation of artificial structures and materials for mimicking naturally occurring designs, such as whole cut meat foods. It also holds substantial potential for revolutionizing the alternative protein industry by catering to a broader consumer base, including flexitarians and meat-eaters.
1 Introduction
Beef production is an especially resource-intensive industry. There is mounting evidence linking livestock farming to high greenhouse gas (GHG) emissions and to climate change (Steinfeld, 2006; Garnett, 2009; Heng et al., 2022). The beef industry’s high ecological footprint underscores the urgent need for environmentally sound alternatives to traditional beef products, including whole-muscle cuts, which comprise a substantial portion of market sales (Close, 2014). As a result, the technological and scientific communities are those working on creative solutions to make meat production more sustainable (Kumar et al., 2022).
Meat substitutes have been an active part of the modern food industry, beginning with Kellogg’s Protose (Protose, 1900). But despite advancements in the past century, alternative meat products have often been marketed mainly to vegetarian and vegan consumers, excluding broader segments of the population such as flexitarians. One reason for this is the apparent dissatisfaction of the market with the flavor and texture of meat alternatives (Appiani et al., 2023). Another challenge is the limited structural sophistication, which has prioritized the development of high volume, low value products such as minced meat and chicken nuggets and excluded the complex nuances of premium products such as whole-muscle cuts of meat (Schreuders et al., 2021; Bushnell et al., 2022).
New approaches, such as better protein texturization methods (Dekkers et al., 2018), are improving the sensory appeal of plant-based meat. Still, the market is missing a systemic approach that addresses the multifaceted traits of meat. This article proposes a pioneering methodology that draws from the principles of tissue engineering and repurposes them using plant-based components, supported by advanced manufacturing technologies. The Plant-Based Tissue Engineering (PBTE) approach originated by Redefine Meat (Ben-Shitrit et al., 2020; redefinemeat) not only addresses the structural and sensory challenges of legacy plant-based products, but has the potential to mimic the full spectrum of animal meat products. We claim that PBTE has could significantly alter the landscape of meat consumption by addressing the expectations of quality, range and versatility of the broader base of consumers that, unlike the captive market segments of vegans and vegetarians, consume animal meat today (Broad, 2020).
2 Meat through the perspective of tissue engineering
2.1 Introduction to tissue engineering and meat structure
Tissue engineering, a discipline established in the 1960s, depicts living organisms as assemblages of various tissues, each characterized by complex architectures that involves diverse cell types and extracellular matrices that serve distinct biological and biomechanical functions (Ikada, 2006; Vacanti, 2006). Although they focus mainly on biomedical applications for repairing living tissues, these principles can also offer a new lens through which to study and replicate the structure of livestock meat, a “product” comprising muscle tissue, connective tissue, adipose tissue, and bone (Ben-Arye and Levenberg, 2019) (Figure 1).
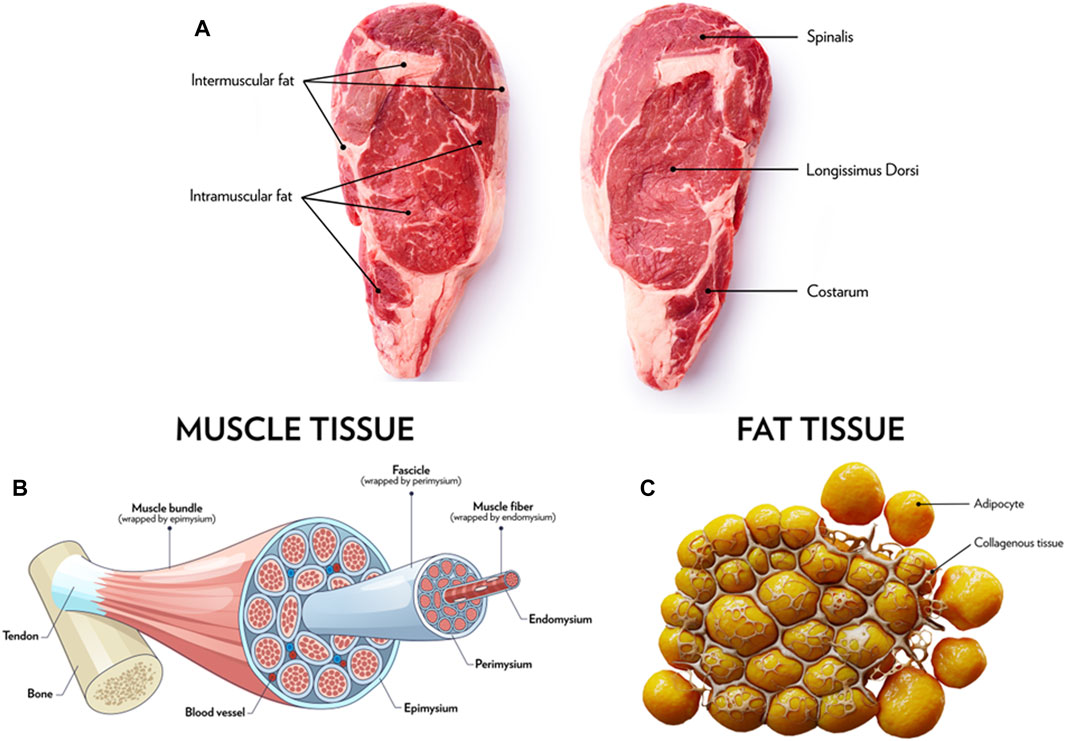
FIGURE 1. Photograph of a typical ribeye steak (A) and illustrations of the anatomical features it comprises of–muscle tissue (B) and fat tissue (C). It depicts the complexity of one of the most common premium cuts in the market and underlines the challenge of reverse engineering it. The PBTE approach is capable of mimicking the various muscle tissue and fat tissue structures via manipulation of protein, lipid and connective tissue components at various scales and assembly methods. Reproduced with permission from Redefine Meat Ltd.
2.2 Understanding the complexity of meat
In its processed form, the elements of meat are intricately organized to form familiar elements or “products” such as steaks, which offer a unique culinary and organoleptic experience. Animal meat, with its inherent heterogeneous structure (such as the varied distribution of fat and muscle) and anisotropic characteristics (owing to muscle fibers and the orientation of connective tissue), presents a sophisticated challenge in food technology. The complex thermal transition during cooking, which is driven by the reactions of proteins and other biomolecules to heat, significantly influences its perceived quality and consumer experience (Tornberg, 2005; Yu et al., 2017). Previous attempts to replicate whole-muscle cuts with plant-based ingredients (Dekkers et al., 2018) often fell short of expectations, resulting in products with limited appeal to omnivores and minimal penetration beyond the vegetarian market (Szenderák et al., 2022).
2.3 The PBTE approach to meat replication
The Plant-Based Tissue Engineering (PBTE) approach addresses these complexities by deconstructing meat into simpler elements, and then mimicking them using accessible food manufacturing technologies, before reassembling them using innovative fabrication methods inspired by Additive Manufacturing (Hertafeld et al., 2019) and robotics. This section describes each of the main tissue elements and its relevant characteristics, highlighting the aspects that are crucial to replicating the meat-eating experience. It outlines the sufficient structural elements and behaviors that are required for successful imitation of the organoleptic qualities of meat, but at the same time strives to limit the complexity of the reverse-engineered tissue components and their spatial resolution accuracy to avoid unnecessary development and production costs (GFI, 2022).
2.3.1 Muscle tissue
The skeletal muscle tissue (Figure 1B) relevant to meat is composed of approximately 65%–75% water and 18%–23% protein, forming a hierarchical structure of muscle fibers interconnected by a network of connective tissue (Purslow, 2023). These fibers, organized into sarcomeres, myofibrils, fascicles, and complete muscles, are further interlinked by connective tissue elements such as endomysium, perimysium, and epimysium (Nishimura, 2010a; Purslow, 2020). Typically, whole muscle cuts are butchered to orient muscle fibers consistently, contributing to the meat’s anisotropic characteristics, such as varying tensile, compression, and shear behaviors (Lepetit and Culioli, 1994), resulting in substantially different values when measured along vs. across fiber direction. The fibrous morphology and orientation are evident during manual or oral breakdown of meat (LILLFORD, 2001), impacting the organoleptic experience of its consumption. The physical properties of these fibers also influence the behavior of meat in raw and cooked form. Upon thermal processing, for example, muscle fibers contract (Purslow et al., 2016), expelling meat exudate rich in peptides and metabolites that are crucial for the Maillard reaction and flavor development (Mottram, 1998), altering simultaneously the aroma, juiciness, and appearance of meat (Flores, 2023).
2.3.2 Adipose tissue (fat)
Beef cuts typically contain between 10% and 25% of fat, which varies with the type of cut and the breed. This tissue is composed of about 90% lipids, 8% water and 2% proteins and is distributed as large intermuscular and subcutaneous inclusions or thinner intramuscular layers (Figure 1A), significantly influencing meat quality and culinary experience (Wood et al., 2008). Fat tissue consists of lipid-rich adipose cells encapsulated in a collagenous connective tissue matrix (Figure 1C), with cells about 0.1 mm in diameter and its surrounding collagenous tissue less than 0.01 mm thick (Tordjman, 2012). The lipid component of beef fat, known as tallow, has a melting point around 40°C–45°C (Grompone, 1989). During cooking lipids melt, altering the appearance and texture of the fat, but leaving the overall structure intact due to the thermally stable connective tissue matrix, which releases most lipids during chewing rather than cooking and contributes to the characteristic mouthfeel of fat (Frank et al., 2016).
2.3.3 Connective tissue
In muscle tissue, connective tissue (CT) structures such as endomysium, perimysium, and epimysium (Figure 1B) play vital roles in texture and integrity (Purslow, 2002; Nishimura, 2010a; Purslow, 2018). The perimysium, particularly significant due to its encasement of fascicle structures, is a thin and strong crossed-ply structure composed of partially cross-linked collagen fibers, constituting about 10% of muscle’s dry mass (Bendall, 1967). Its properties, including significant strength and flexibility, are major contributors to meat toughness, with higher CT fractions typically leading to lower tenderness (Roy and Bruce, 2023). When cooked, collagen partially melts, but the cross-linked fraction remains, maintaining interconnectivity of muscle fibers. Extended cooking can degrade CT, affecting the meat’s textural properties (Weston et al., 2002).
2.3.4 The organization of whole-muscle cuts
The diversity of whole muscle cuts in beef reflects varied arrangements of muscles and fat (Figure 1A), contributing to the unique characteristics of different steak types. These variations are influenced by multiple factors, including the animal’s breed, growth conditions, feed, age, and butchery techniques, such as the specific area of the cut, meat aging, and processing conditions (Chriki et al., 2013). Each steak can be analyzed in terms of protein and fat composition, the amount of connective tissue, fat distribution, and the presence of bones–all of which contribute to the culinary classification, perceived quality, and market value (Dransfield, 1977).
The gastronomic qualities of meat are not solely determined by intrinsic properties but are also significantly influenced by the culinary process. Cooking methods, temperatures, and durations can drastically alter the texture, flavor, and nutritional value of the meat. For example, the Maillard reaction, which occurs during certain types of cooking, greatly enhances flavor and aroma, contributing to the overall appeal of the dish (Bailey, 1994; Mottram and Elmore, 2005).
This intricate balance of biological structure and culinary science underscores the challenge in replicating these characteristics in plant-based alternatives. It is crucial to consider these parameters when attempting to recreate the complex architecture of whole-muscle cuts. The PBTE approach aims to address these challenges by meticulously analyzing and mimicking these properties using plant-based ingredients, assembling them in a manner that replicates the nuanced structure and sensory experience of traditional meat cuts. The subsequent sections will detail how each tissue type is analyzed, its key structural and physical parameters identified, and the methods used to reconstruct these elements with plant-based materials before assembling them into a cohesive, steak-like structure.
3 Reconstructing whole-muscle cuts of meat using the PBTE approach
3.1 Muscle component: Generating fascicles
One of the guiding principles of our development process was that it would need to address a set of key characteristics to successfully imitate muscle components:
a. High protein content: This is essential if we aim to match the nutritional profile of meat.
b. Anisotropic fibrous structure: Visible muscle strands (1–5 mm thick) that are densely packed and separable, mirroring the jagged-shaped interface morphology of natural meat. Resistance to shear should be higher across the fibers than along the fibers, aligning with values observed in livestock meat.
c. Texture and Hardness: Defined by typical Texture Profile Analysis (TPA) tests, 20 mm cubic specimens at 70% strain should meet the load resistance values of raw (about 20–40N) and cooked (about 50–80N) livestock meat.
d. Manufacturing ability: Allowing to form into steak-relevant form factor, say having dimensions of at least 150 × 80 × 15 mm.
e. Liquids: Containing at least 60% water and usually some lipids as well. These liquid components are responsible for the experience of juiciness and for the development and delivery of taste and aroma during cooking and consumption.
f. Color: Purple-red when raw, transitioning to grey-brown after cooking.
It is important to mention, that this is a minimal set of characteristics providing the entry-point to steak-relevant texture. Further analysis of tensile behavior, shear behavior and sensory analysis is crucial for generating a product that will be accepted by consumers.
Current alternative meat production methods, such as protein extrusion (Areas, 1992; Dekkers et al., 2018), are inadequate to meet all the above requirements. TVP (Textured Vegetable Protein) (Emin and Schuchmann, 2017) and HME (High Moisture Extrusion) (Lin et al., 2000) techniques fall short in mimicking all the above requirements to replicate the specific texture and structural integrity of meat. TVP is too porous and HME is too dense and impermeable, and neither can be formed into a steak form factor (e.g., at least 150 × 80 × 15 mm), while providing relevant orientation of fibers. Methods like shear cell (Krintiras et al., 2016) address the dimensional requirements yet fall short in providing beef-relevant texture. Other techniques, such as spinning (Mattis and Marangoni, 2020), fail to provide sufficient throughput at an acceptable cost. Bioengineering approaches, such as mycelium (Kyoungju et al., 2011) or animal tissue culture (Post, 2012) still lack the required texture demands - and require supplementing them with plant-based texture elements (Ben-Arye et al., 2020), but even then, they fail to deliver the relevant meat performance operating in industrial environments (Post et al., 2020).
To meet the requirements of the muscle component, our research proposes disintegrating the tough and chewy TVP material into separate fibers (0.2–2 mm in diameter, about 2–20 mm in length), blending them with a proteinous dough made from soy or pea isolates (Ben-Shitrit et al., 2021). A typical TVP product suiting the toughness criteria typically comprises about 50% wheat protein and soy protein and has relatively low (<250% w/w) water holding ability, such as DuPont Danisco SUPRO® MAX 5050. This dough, when extruded using a progressive cavity pump (PCP, PCM Ecomoineau™ C) through a 3 mm nozzle, delivers strands that imitate the hierarchical structure of fibrous livestock meat, namely, stacked fascicles (Ben-Shitrit et al., 2021). To form a macroscopic muscle section, the deposition is made according to typical 3D-printing protocols, in a self-supporting manner, as the dough viscosity is sufficiently high (>300 P) to prevent gravitational flow or sagging at the fabrication temperature that is set to about 4°C to meet food safety requirements. Unidirectional deposition of such strands results in structure having prominent anisotropic behavior and its resistance to shear being comparable to that of animal meat. This approach allows the projection of the meat-like texture of TVP, but in a flexible manner, and in a scalable manner. At the same time, this muscle-like structure alone is insufficient in meeting livestock meat’s tensile behavior values and fully mimic its inter-fascicle failure mechanisms. This deficiency required the development and introduction of a component that would act as connective tissue and reinforce the composite structure of the meat alternative and upgrade its toughness and behavior during cooking and eating (such as the disintegration of fibers) in a way that matches the behavior of animal meat.
3.2 From fascicles to muscle tissue
Developing a connective tissue (CT) component posed a few significant challenges:
a. Obtaining Food-Grade Ingredients and Processes: Using ingredients and formulations that are safe and compliant for consumption, while meeting the structural requirements listed below. Most plant-based edible materials lack the strength that is characteristic of animal tissues.
b. Formation into thin laminates less than 0.05 mm thick, to mimic naturally occurring connective tissue. Conventional food technologies do not require high spatial accuracy and are limited in providing such level of structural fidelity.
c. Strength and Durability: Minimum tensile strength of 1 MPa in a 70% water environment when raw and retaining at least 0.2 MPa strength after heating to 75°C. This humidity level corresponds to hydrogels that are typically weak and brittle and require obtaining formulation with exceptional molecular bonding for delivering such strength.
We found that carrageenan-based materials with melting temperatures around 90°C fulfil these requirements and could be cast into films, which are then introduced in-between the fascicles during layer-wise fabrication (Figures 2A,B), while setting one step interlace between the strands (Dikovsky and Hausner, 2022). Post-hydration and thermal processing result in fusion of the carrageenan films and formation of continuous comb-shaped network (Figure 2C), with tensile strengths mimicking that of animal meat. Table 1 compares the tensile strength of meat measured along and across the direction of the fibers. It shows that the addition of the CT component substantially improves the performance of PB meat, matching its strength to that of a tenderloin (Psoas Major) at 90% at longitudinal direction and 85% at transverse direction. Moreover, the plant-based muscle with CT demonstrates a different failure mode upon stretching (Figure 3A), that is comparable to livestock meat (Figure 3B) and substantially different from plant-based muscle without CT (Figure 3C). Internal sensory panel tests (n = 30) indicated 82% preference for alternative meat samples that contained CT compared to those without CT, with specific feedback from participants noting improvements in texture and appearance. The characteristic fibrous texture of such meat is shown after dissection in Figure 2G.
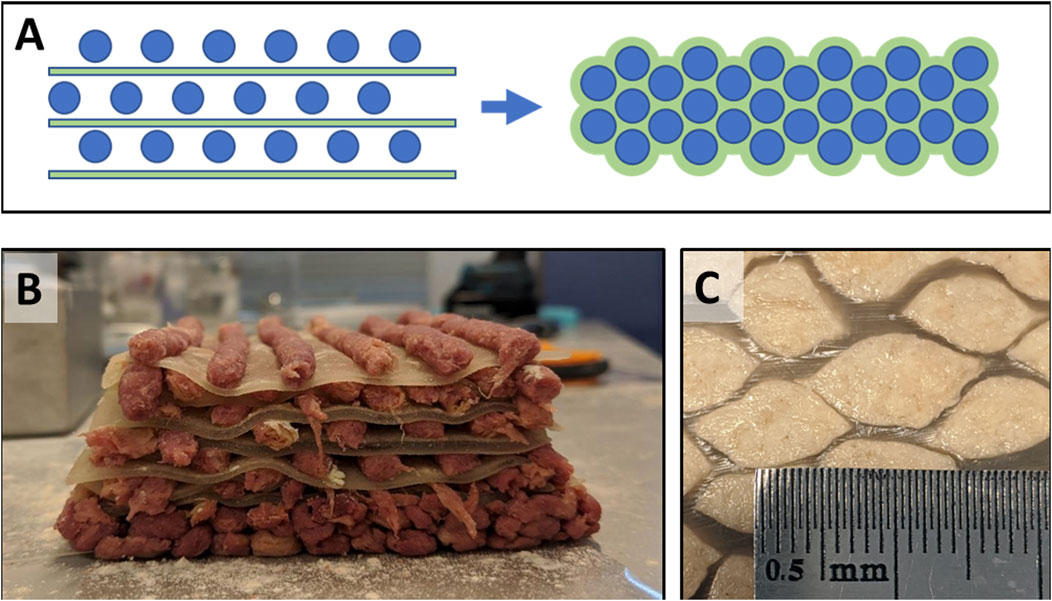
FIGURE 2. Demonstrating the importance of connective tissue for mimicking the complex mechanical behavior of livestock meat. Schematic illustration of muscle tissue fabrication process presented in cross-sectional view, where the circles represent extruded PB muscle strands and the lines represent sheets of edible material that laid in between to imitate CT component. The deposition sequence (A) and the resulting muscle tissue structure after compactization and thermal post-processing cycle that fuses the CT layers together into a continuous comb-shaped matrix. Photographs showing the composite structure of PB muscle tissue before compactization (B) and after (C). Reproduced with permission from Redefine Meat Ltd.
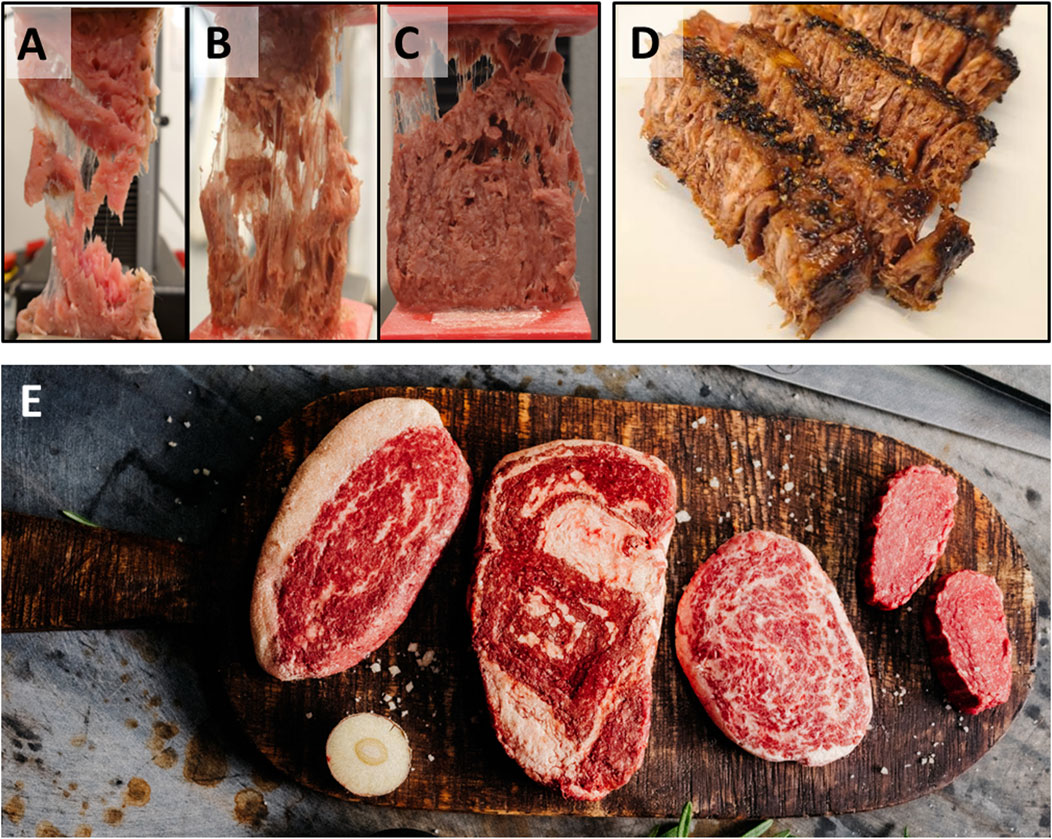
FIGURE 3. Demonstrating the structure and behavior of complete alt-meat whole-muscle cuts made via PBTE approach. Photographs of 3 types of raw meat subjected to tensile extension: livestock Psoas Major muscle (A), PB muscle with CT (B) and PB muscle without CT (C). Photograph of sliced cooked steak made via the PBTE approach (D). Photograph of raw PB steaks, each of which was created using advanced manufacturing that used the same set of muscle, fat and connective tissue components, but different fabrication protocols (E). Reproduced with permission from Redefine Meat Ltd.
The muscle component and connective tissue component are integrated into a system that employs additive manufacturing methods accompanied by robotic film deposition, altogether digitally controlled, to deliver about a variety of alternative meat muscle tissue structures. The following parameters can easily be adapted to control the toughness, fibrousity, chewiness and cooking behavior of the alternative meat:
• Type of TVP
• Fraction of TVP in the muscle component
• The composition of the Proteinous dough as well as its water percentage
• Thickness of muscle strands
• CT film composition, e.g., carrageenan ratio
• CT film thickness
• Additional adhesive layers between CT and muscle strands
The final last component in the list of components is fat, and fat distribution and marbling.
3.3 Fat
The fat component, crucial to achieve the desired texture and mouthfeel, involves encapsulating a lipid formulation (melting point >35°C, using primarily cacao fat) in a hydrocolloid matrix. This matrix, composed of methylcellulose, stabilizes the fat tissue during cooking and prevents it from melting out. The high lipid content (≥75% w/w) ensures a substantial liquid oily phase at serving temperature (50°C), contributing to a fatty mouthfeel. The different types of fat, such as intermuscular fat and intramuscular fat, can be recreated, for example, via the variation of overall lipid content and the concentration methylcellulose in the matrix. This fat component is compatible with the additive manufacturing process, allowing precise deposition to recreate specific marbling patterns. Here too, the viscosity of the fat component paste is maintained high enough to allow self-supporting deposition using typical PCP pumps. Due to the nature of marbling in beef steaks, the fat deposition resolution may require thinner nozzles, down to 1 mm. The interconnection between the muscle component and the fat component is facilitated by the presence of the CT component that holds together the unidirectional elements that form the muscle and fat tissue regions.
3.4 Advanced food manufacturing
Breaking meat down into components and addressing each component separately allows more accurate mimicking using plant-based ingredients. However, the assembly of these components into a complete meat product requires new approaches to food manufacturing. Inspired by Additive Manufacturing (AM) and robotics, we developed a process that uses high-pressure extrusion devices and a static multi-nozzle array, delivering high-viscosity component pastes onto a digitally controlled XYZ platform (Ben-Shitrit et al., 2020). Other elements of the plant-based meat, such as the CT laminates, are dispensed via dedicated robotic modules. The use of this advanced manufacturing method enables the creation of any spatial configuration of muscle and fat, supporting the production of relevant whole-muscle cut analogs.
The Additive Manufacturing (AM) process facilitates:
• Spatial configuration control of muscle and fat and realistic marbling.
• Mass customization of steaks and cuts.
• Inducing orientation of TVP fibers in plant-based muscle strands.
• The fabrication of composite structures, such as muscle with integrated CT.
• Unidirectional deposition of muscle strands, resulting in a culinary-relevant grain when the steaks are cut (Figure 1).
While successfully exploiting the benefits of AM, it was important to address its major weakness points, namely, cost and throughput, that frequently limit its implementation in industrial applications and could impact the market potential of the current solution. This was achieved through a design-for-function approach, where the fabrication system was optimized solely for beef whole muscle cuts application, while maximizing throughput. In practice this resulted in a system operating at relatively coarse spatial resolution, depositing about 3 mm strands, with no need for support material or curing, with all strands being laid in the same orientation forming long slabs, approximately 1-m in length. Altogether, this resulted in minimal tray idle time, maximized travel velocity and low waste. Once combined with a 12-nozzle plate simultaneously depositing material, the system generated a throughput of more than 10 kg/h, an equivalent of about cow a day of alt-meat products (Mandelik et al., 2021).
Figure 3E shows a set of animal-free whole muscle cuts made using the PBTE concept and matching some of the common premium cuts, such as tenderloin, sirloin, ribeye and wagyu. All of these were cut from slabs made with the same set of muscle, fat and connective tissue components and digitally manipulated to form the desired form factor and marbling patterns.
4 Discussion and conclusion
This paper presents a novel Plant-Based Tissue Engineering (PBTE) approach, leveraging principles of tissue engineering and advanced food manufacturing to recreate the complex structure of whole-muscle meat cuts. Our findings demonstrate that the PBTE method effectively addresses the multifaceted challenges inherent in replicating the texture, structure, and sensory attributes of traditional meat products.
4.1 Significance in the field of Soft Matter
The PBTE approach represents a significant contribution to the field of Soft Matter, particularly in food science. It showcases how the manipulation of plant-based materials at the microstructural level can lead to the development of complex, multi-component food systems. By mimicking the hierarchical structure of muscle, adipose, and connective tissues in meat, we can bridge a crucial gap in the alternative protein industry, aligning with the Soft Matter principles of understanding and engineering complex structures and materials.
4.2 Key findings
• Muscle Tissue Replication: Through disintegration of TVP into fibers blended with proteinous dough, we successfully mimicked the anisotropic and fibrous structure of muscle tissue. The introduction of a connective tissue component further enhanced the mechanical integrity and sensory attributes of the muscle analog, closely resembling those of animal meat.
• Fat Tissue Engineering: The encapsulation of lipid formulation within a hydrocolloid matrix effectively recreated the mouthfeel and texture of intramuscular fat, a key element in the sensory profile of meat.
• Advanced Manufacturing Techniques: Our adoption of additive manufacturing and robotic systems enabled precise spatial configuration of muscle and fat components, offering a versatile platform for producing a wide range of meat analogs. This aspect is particularly groundbreaking, showcasing the potential of multi-material 3D-printing and robotics in revolutionizing food manufacturing.
4.3 Implications and future directions
• Environmental Impact: Given the resource-intensive nature of traditional meat production, the PBTE method offers a more sustainable alternative to meat, potentially reducing greenhouse gas emissions and the use of resources like water, land, and energy.
• Market Potential: The consumption of meat around the world continues to climb, despite a growing awareness of the negative impact of animal agriculture. This is also despite the introduction of plant-based meat analogues to the market, which have so far mostly focused on imitating burgers, chicken nuggets or other meat products on the low-end of the value chain. We believe that this technology opens new avenues for the alternative meat industry to cater to a broader consumer base, including flexitarians and meat-eaters, by offering products that closely replicate the sensory experience of animal meat, including whole-muscle cuts.
• Relevance to other meats: The PBTE approach can be extended to mimic other types of meat, besides beef. The reconstruction of naturally-occurring sophisticated structures through their breakdown into a set of simpler components and their independent reconstruction with a different set of materials, followed by a digital assembly for resembling the complex architecture of the original object can be applied to other foods. Naturally, this requires dedicated analysis of the target meat to determine the characteristics of its tissues and their organization. Then the plant-based components analogs need to be fine-tuned to match these characteristics. However, we are confident that the set of the plant-based ingredients, formulation methods and advanced manufacturing methods described here, can address a wide range of meat products.
• Future Research: We believe that the versatility of our approach together with its market potential and its expected environmental impact can attract further interest and research potential within both industrial and academical domains, and help accelerate its expansion to additional markets and product types. We emphasize the importance of further progress in developing plant-based components that have tougher textures, good processability, low levels of off-flavors, good nutritional profiles, competitive cost and availability at scale, while having low environmental footprint. In parallel, we expect further acceleration of this domain through the development and adaptation of advanced manufacturing techniques to food production environments.
In conclusion, the PBTE approach marks a pivotal step in the evolution of meat alternatives. By intricately replicating the structure and texture of animal meat using plant-based ingredients, we not only address environmental and ethical concerns but also cater to the growing demand for sustainable and diverse protein sources that meet consumer expectations for taste, texture and other parameters of meat. The principles and methodologies developed in this study hold significant potential for future innovations in the Soft Matter field, particularly in helping to develop sustainable food systems.
Author’s note
This perspective manuscript was prepared by the sole author, based on a presentation at the 1st Soft Matter Conference in 2023 and it summarizes major work done by Redefine Meat Ltd. research groups between 2019 and 2023, with specific contribution by: Daniel Mandelik, Sagee Schechter, Or Sabbah, Alexey Tomsov, Nissim David, Inbar Haimovich, Hay Shaino, Yael Prigat Goldfinger, Moran Cohen, Jon Hausner, Nina Bochner, Tal Shimony-Cohen, Eshchar Ben Shitrit and Adam Lahav. The photography presented in Figure 3E was made by Roscoe Raz and May Siri and reproduced with permission from Redefine Meat Ltd.
Data availability statement
The original contributions presented in the study are included in the article/Supplementary material, further inquiries can be directed to the corresponding author.
Author contributions
DD: Writing–original draft, Writing–review and editing.
Funding
The author declares financial support was received for the research, authorship, and/or publication of this article. The work was funded by Redefine Meat Ltd. and partially by Israeli Innovation Authority (IIA). The grant number for IIA is #71520. Redefine Meat Ltd. was not involved in the study design, collection, analysis, interpretation of data, the writing of this article, or the decision to submit it for publication.
Acknowledgments
The author would like to thank prof. Peter Purslow for his advisory on this project and the organizers of the first Soft Matter Conference: prof. Alejandro Marangoni, prof. Raffaele Mezzenga, prof. Ali Miserez and prof. Frank Alexis.
Conflict of interest
Author DD was employed by Redefine Meat Ltd.
Publisher’s note
All claims expressed in this article are solely those of the authors and do not necessarily represent those of their affiliated organizations, or those of the publisher, the editors and the reviewers. Any product that may be evaluated in this article, or claim that may be made by its manufacturer, is not guaranteed or endorsed by the publisher.
References
Appiani, M., Cattaneo, C., and Laureati, M. (2023). Sensory properties and consumer acceptance of plant-based meat, dairy, fish and eggs analogs: a systematic review. Front. Sustain. Food Syst. 7, 1–23. doi:10.3389/fsufs.2023.1268068
Areas, J. (1992). Extrusion of food proteins. Crit. Rev. Food Sci. Nutr. 32 (4), 365–392. doi:10.1080/10408399209527604
Bailey, M. E. (1994). “Maillard reactions and meat flavour development,” in Flavor of meat and meat products (Boston, MA: Springer US), 153–173.
Ben-Arye, T., and Levenberg, S. (2019). Tissue engineering for clean meat production. Front. Sustain. Food Syst. 3, 46. doi:10.3389/fsufs.2019.00046
Ben-Arye, T., Shandalov, Y., Ben-Shaul, S., Landau, S., Zagury, Y., Ianovici, I., et al. (2020). Textured soy protein scaffolds enable the generation of three-dimensional bovine skeletal muscle tissue for cell-based meat. Nat. Food 1, 210–220. doi:10.1038/s43016-020-0046-5
Bendall, J. R. (1967). The elastin content of various muscles of beef animals. J. Sci. Food Agric. 18 (12), 553–558. doi:10.1002/jsfa.2740181201
Ben-Shitrit, E., Tomsov, A., Mandelik, D., Dikovsky, D., and Silberstein, S. (2020). Meat analogues and methods of producing the same.
Ben-Shitrit, E., Tomsov, A., Mandelik, D., Hazan, N., Bochner, N., Dikovsky, D., et al. (2021). Whole muscle meat substitute and methods of obtaining the same. WO2021095034A1.
Broad, G. M. (2020). Making meat, better: the metaphors of plant-based and cell-based meat innovation. Environ. Commun. 14 (7), 919–932. doi:10.1080/17524032.2020.1725085
Bushnell, C., Specht, L., and Almy, J. (2022). State of the Industry Report | Plant-based meat, seafood, eggs, and dairy.
Chriki, S., Renand, G., Picard, B., Micol, D., Journaux, L., and Hocquette, J. F. (2013). Meta-analysis of the relationships between beef tenderness and muscle characteristics. Livest. Sci. 155 (2-3), 424–434. doi:10.1016/j.livsci.2013.04.009
Close, D. (2014). Ground Beef Nation: the effect of changing consumer tastes and preferences on the US cattle industry.
Dekkers, B. L., Boom, R. M., and van der Goot, A. J. (2018). Structuring processes for meat analogues. Trends Food Sci. Technol. 81, 25–36. doi:10.1016/j.tifs.2018.08.011
Dransfield, E. (1977). Intramuscular composition and texture of beef muscles. J. Sci. Food Agric. 28 (9), 833–842. doi:10.1002/jsfa.2740280910
Emin, M. A., and Schuchmann, H. P. (2017). A mechanistic approach to analyze extrusion processing of biopolymers by numerical, rheological, and optical methods. Trends Food Sci. Technol. 60, 88–95. doi:10.1016/j.tifs.2016.10.003
Flores, M. (2023). The eating quality of meat: III—flavor. Lawrie’s Meat Sci., 421–455. doi:10.1016/b978-0-323-85408-5.00014-5
Frank, D., Joo, S. T., and Warner, R. (2016). Consumer acceptability of intramuscular fat. Korean J. food Sci. animal Resour. 36 (6), 699–708. doi:10.5851/kosfa.2016.36.6.699
Garnett, T. (2009). Livestock-related greenhouse gas emissions: impacts and options for policy makers. Environ. Sci. policy 12 (4), 491–503. doi:10.1016/j.envsci.2009.01.006
Grompone, M. A. (1989). Physicochemical properties of fractionated beef tallows. J. Am. Oil Chem. Soc. 66 (2), 253–255. doi:10.1007/bf02546070
Heng, M., McCarl, B., and Fei, C. (2022). Climate change and livestock production: a literature review. Atmosphere 13 (1), 140. doi:10.3390/atmos13010140
Hertafeld, E., Zhang, C., Jin, Z., Jakub, A., Russell, K., Lakehal, Y., et al. (2019). Multi-material three-dimensional food printing with simultaneous infrared cooking. 3D Print. Addit. Manuf. 6 (1), 13–19. doi:10.1089/3dp.2018.0042
Ikada, Y. (2006). Challenges in tissue engineering. J. R. Soc. Interface 3 (10), 589–601. doi:10.1098/rsif.2006.0124
Krintiras, G. A., Gadea Diaz, J., van der Goot, A. J., Stankiewicz, A. I., and Stefanidis, G. D. (2016). On the use of the Couette Cell technology for large scale production of textured soy-based meat replacers. J. Food Eng. 169, 205–213. doi:10.1016/j.jfoodeng.2015.08.021
Kumar, P., Abubakar, A. A., Verma, A. K., Umaraw, P., Adewale Ahmed, M., Mehta, N., et al. (2022). New insights in improving sustainability in meat production: opportunities and challenges. Crit. Rev. Food Sci. Nutr. 63, 11830–11858. doi:10.1080/10408398.2022.2096562
Kyoungju, K., Byungsun, C., Inhee, L., Hyeyoung, L., Soonhyang, K., Kyoungyoung, O., et al. (2011). Bioproduction of mushroom mycelium of Agaricus bisporus by commercial submerged fermentation for the production of meat analogue. J. Sci. Food Agric. 91 (9), 1561–1568. doi:10.1002/jsfa.4348
Lepetit, J., and Culioli, J. (1994). Mechanical properties of meat. Mech. Prop. meat 36 (1-2), 203–237. doi:10.1016/0309-1740(94)90042-6
Lillford, P. J. (2001). Mechanisms of fracture in foods. J. Texture Stud. 32 (5-6), 397–417. doi:10.1111/j.1745-4603.2001.tb01244.x
Lin, S., Huff, H. E., and Hsieh, F. (2000). Texture and chemical characteristics of soy protein meat analog extruded at high moisture. J. Food Sci. 65 (2), 264–269. doi:10.1111/j.1365-2621.2000.tb15991.x
Mandelik, D., Comforti, E., Schachter, S., Shapira, G., and Dikovsky, D. (2021). System and method for fabrication of a three-dimensional edible product. WO2022079718.
Mattis, K. D., and Marangoni, A. G. (2020). Comparing methods to produce fibrous material from zein. Food Res. Int. 128, 108804. doi:10.1016/j.foodres.2019.108804
Mottram, D. S. (1998). Flavour formation in meat and meat products: a review. Food Chem. 62 (4), 415–424. doi:10.1016/s0308-8146(98)00076-4
Mottram, D. S., and Elmore, J. S. (2005). “The interaction of lipid-derived aldehydes with the Maillard reaction in meat systems,” in The maillard reaction in foods and medicine (USA: Woodhead Publishing), 198–203.
Nishimura, T. (2010a). The role of intramuscular connective tissue in meat texture. Animal Sci. J. 81 (1), 21–27. doi:10.1111/j.1740-0929.2009.00696.x
Post, M. J. (2012). Cultured meat from stem cells: challenges and prospects. Meat Sci. 92 (3), 297–301. doi:10.1016/j.meatsci.2012.04.008
Post, M. J., Levenberg, S., Kaplan, D. L., Genovese, N., Fu, J., Bryant, C. J., et al. (2020). Scientific, sustainability and regulatory challenges of cultured meat. Nat. Food 1, 403–415. doi:10.1038/s43016-020-0112-z
Purslow, P. P. (2002). The structure and functional significance of variations in the connective tissue within muscle. Comp. Biochem. Physiology Part A Mol. Integr. Physiology 133 (4), 947–966. doi:10.1016/s1095-6433(02)00141-1
Purslow, P. P. (2018). Contribution of collagen and connective tissue to cooked meat toughness; some paradigms reviewed. Meat Sci. 144, 127–134. doi:10.1016/j.meatsci.2018.03.026
Purslow, P. P. (2020). The structure and role of intramuscular connective tissue in muscle function. Front. Physiology 11, 495. doi:10.3389/fphys.2020.00495
Purslow, P. P. (2023). “The structure and growth of muscle,” in Lawrie’s meat science (China: Woodhead Publishing), 51–103.
Purslow, P. P., Oiseth, S., Hughes, J., and Warner, R. D. (2016). The structural basis of cooking loss in beef: variations with temperature and ageing. Food Res. Int. 89, 739–748. doi:10.1016/j.foodres.2016.09.010
redefinemeat. Available at: https://www.redefinemeat.com/technology.
Roy, B. C., and Bruce, H. L. (2023). Contribution of intramuscular connective tissue and its structural components on meat tenderness-revisited: a review. Crit. Rev. Food Sci. Nutr., 1–31. doi:10.1080/10408398.2023.2211671
Schreuders, F., Schlangen, M., Kyriakopoulou, K., Boom, R., and van der Goot, A. J. (2021). Texture methods for evaluating meat and meat analogue structures: a review. Food control. 127, 108103. doi:10.1016/j.foodcont.2021.108103
Steinfeld, H. (2006). Livestock’s long shadow: environmental issues and options. Food and Agric. Org.
Szenderák, J., Fróna, D., and Rákos, M. (2022). Consumer acceptance of plant-based meat substitutes: a narrative review. Foods 11 (9), 1274. doi:10.3390/foods11091274
Tordjman, J. (2012). “Histology of adipose tissue,” in Physiology and physiopathology of adipose tissue (Paris: Springer Paris), 67–75.
Tornberg, E. V. A. (2005). Effects of heat on meat proteins–Implications on structure and quality of meat products. Meat Sci. 70 (3), 493–508. doi:10.1016/j.meatsci.2004.11.021
Vacanti, C. A. (2006). The history of tissue engineering. J. Cell. Mol. Med. 10 (3), 569–576. doi:10.2755/jcmm010.003.20
Weston, A. R., Rogers, R. W., and Althen, T. G. (2002). Review: the role of collagen in meat tenderness. Prof. Animal Sci. 18 (2), 107–111. doi:10.15232/s1080-7446(15)31497-2
Wood, J. D., Enser, M., Fisher, A. V., Nute, G. R., Sheard, P. R., Richardson, R. I., et al. (2008). Fat deposition, fatty acid composition and meat quality: a review. Meat Sci. 78 (4), 343–358. doi:10.1016/j.meatsci.2007.07.019
Keywords: meat analog, beef, connective tissue, additive manufacturing, tissue engineering, plant-based meat
Citation: Dikovsky D (2024) Addressing the structural sophistication of meat via plant-based tissue engineering. Front. Soft Matter 4:1343906. doi: 10.3389/frsfm.2024.1343906
Received: 24 November 2023; Accepted: 22 January 2024;
Published: 06 February 2024.
Edited by:
Raffaele Mezzenga, ETH Zürich, SwitzerlandReviewed by:
Mike Boland, Massey University, New ZealandLilia Ahrné, University of Copenhagen, Denmark
Copyright © 2024 Dikovsky. This is an open-access article distributed under the terms of the Creative Commons Attribution License (CC BY). The use, distribution or reproduction in other forums is permitted, provided the original author(s) and the copyright owner(s) are credited and that the original publication in this journal is cited, in accordance with accepted academic practice. No use, distribution or reproduction is permitted which does not comply with these terms.
*Correspondence: Daniel Dikovsky, ZGFuaWVsLmRpa292c2t5QHJlZGVmaW5lbWVhdC5jb20=