- Department of Materials Science and Engineering, University of Sheffield, Sheffield, United Kingdom
Gastropods, such as snails and slugs, can excrete mucus to aid in movement and adhesion. However, very few studies have examined the physical relationship between mucus composition and function. Here, we explore the role of mucus polymers (specifically their proteins) and their influence on the material properties of locomotive mucus. Using a range of spectroscopic, thermal, and rheological analytical tools, we characterised locomotive mucus from six gastropod species across four families. We report that all mucus tested consisted of 97%–99% water, and the remaining 1%–3% solid content contained a range of proteins (41–377 kDa, 18 of which are previously undocumented), which we propose contribute to its weak gel behaviour (1.58–36.33 Pa•s at 1 rad/s). Our results indicate that mucus properties are also grouped at the family level, suggesting that niche-specific adaptation occurs in these materials. We expect our study to offer a broader approach to how a correlation between properties is crucial for understanding the stability and functionality of snail mucus.
Introduction
Most natural materials remain inside or close to the body, allowing for repair and reconfiguration. However, some species have evolved the ability to produce and use materials outside their bodies. Termed ecto-secretions, these are a remarkably overlooked yet important class of materials that are selected to perform in extreme environments and facilitate a range of biological functions, from structural (silks) to chemical (venoms) (Casewell et al., 2013; Flórez et al., 2015; Avella et al., 2021). Gastropod mucus is a prime example of such an ecto-secretion that when excreted as a thin layer (10–20 µm) aids locomotion, adhesion, and defence; prevents desiccation and infection; and in some cases, even serves as a substrate for microbial “farming” (Chase et al., 1980; Luchtel et al., 1991; Peck et al., 1993; Kim et al., 1996; Perez-vilar and Hill, 1999; Smith and Morin, 2002; Thornton, 2004; Artacho and Nespolo, 2009; Lai et al., 2009; He et al., 2016; Dhanisha et al., 2018). However, despite its natural ubiquity and utility, only a handful of studies have specifically focused on gastropod mucus alone (Denny, 1980; Denny, 1984; Denny and Gosline, 1980; Bretz and Dimock, 1983; Deyrup-Olsen et al., 1983; Hawkins, 1992; Cottrell et al., 1994; Davies and Hutchinson, 1995; Davies and Hatcher, 1999; Smith et al., 1999, Smith et al., 2009; Skingsley et al., 2000; Smith and Morin, 2002; Struthers et al., 2002; Pawlicki et al., 2004; Ewoldt et al., 2007; Werneke et al., 2007; Ewoldt et al., 2009; Braun et al., 2013; Newar and Ghatak, 2015; Zhong et al., 2018; Fung, Gallego Lazo, and Smith, 2019; O’Hanlon et al., 2019). This limited amount of knowledge surrounding the composition and structure of gastropod mucus is further compounded when considering its material and mechanical properties.
Although gastropods have captivated researchers for centuries, it was not until the 1970s that Denny (1973) and Denny (1984) systematised the study of gastropod mucus with a broader vision and through a combination of experimental and theoretical studies related a range of mucus’ physical properties to the animal’s biology and habitat (Denny, 1984). Denny was the first to study the mechanical (rheological) properties of gastropod locomotive mucus, demonstrating that in the slug Ariolimax columbianus, its shear stiffness is indirectly proportional to its water content (degree of hydration) (Denny, 1984). He proposed that the mucus polymers in gastropod locomotive mucus contributed to these properties by responding to their degree of hydration. For example, as the water content of mucus is reduced, the preferential interactions of the mucus polymers with water begin to switch to interacting with other mucus polymers, increasing the number of intermolecular associations and, therefore, stiffness.
Twenty years after Denny’s studies, Ewoldt et al. (2007) extended this line of research and compared the non-linear rheological properties of locomotive mucus from a snail (Helix aspersa) and a slug (Limax maximus). Their significant findings suggested that the timescale by which mucus is deformed determines whether it behaves as an adhesive or a lubricant. Using a novel rheological fingerprinting technique, they categorised mucus as having viscoelastic properties and behaving as a non-Newtonian gel (Ewoldt et al., 2007). More recently, Fung, Gallego Lazo, and Smith (2019) attributed the rheological properties of Arion subfuscus adhesive mucus to a double network of protein chains with sacrificial bonds and carbohydrates interacting with metal ions, both of which can readily reform if broken.
In addition to the degree of hydration or concentration, from a polymer science perspective, molecular weight (i.e., polymer chain length) could be equally, if not more, influential in determining flow properties (Ferry, 1980). However, this link has been somewhat overlooked to date. Most mucus compositional studies have tended to use the simplicity of SDS-PAGE to identify the molecular weight of proteins in gastropod mucus, for example, characterising the marine snails Lottia limatula and Haliotis diversicolor; terrestrial slugs Arion subfuscus and Arion ater; and the garden snail Helix aspersa (Cottrell et al., 1994; Smith et al., 1999, Smith et al., 2009; Smith and Morin, 2002; Pawlicki et al., 2004; Ewoldt et al., 2007; Werneke et al., 2007; Guo et al., 2009; Wilks et al., 2015). However, more specialised mass spectroscopy and chromatography have also been used for determining the molecular weight of components in the marine snails Patella vulgata and Dendropoma maxima; terrestrial slug A. subfuscus; and terrestrial snails H. aspersa, Eobania vermiculata, Thebe pisana, and Monacha obstructa (Davies and Hatcher, 1999; Pawlicki et al., 2004; Werneke et al., 2007; Smith et al., 2009; Sallam and El-Wakeil, 2012; Klöppel et al., 2013). However, the link between mucus polymer morphology and its influence on mucus performance remains to be determined.
Hence, a cohesive understanding, within an appropriate evolutionary context, between mucus’ molecular components and flow behaviour across a range of species is currently missing. Therefore, to differentiate between the factors that could influence mucus rheology, we propose using UV-vis spectroscopy to determine protein concentration or hydration level between samples and SDS-PAGE to identify proteins and their molecular weight. The approach proposed here has not been explored previously, as most studies incorporating SDS-PAGE with UV-vis tend to focus on a specific protein of interest, not probing the entire composition of mucus. In addition, to the authors’ knowledge, something as seemingly trivial as mucus protein concentration has surprisingly not been reported.
Our hypothesis is that mucus polymers influence the mucus phenotype, and in line with our wider classification of these materials as ecto-secretions, mucus proteins will be a key component in helping deliver functionality for the required timescale of use by the animal. Hence, this work presents an initial foray into this area through a combination of thermal (thermogravimetric analysis (TGA) and rheological ramp temperature tests), compositional (UV-vis and SDS-PAGE), and functional (rheology) techniques to characterise and compare locomotive mucus across six different terrestrial gastropod species: Achatina fulica (Lissachatina fulica), Cornu aspersum, Cepaea nemoralis, Arion ater, Arion hortensis, and Limax flavus.
Materials and Methods
Materials
Three species of terrestrial snails (A. fulica, C. aspersum, and C. nemoralis) and three terrestrial slugs (A. ater, A. hortensis, and L. flavus) were included in this study. Apart from the snail A. fulica, which is native to Africa, all other species are found in Europe. A. hortensis, A. ater, C. nemoralis, C. aspersum, and L. flavus were collected in Hillsborough Park, Hillsborough, Sheffield (53.4080° N, 1.5015° W). A. fulica snails were purchased as juveniles and reared in house. All species were kept in plastic containers (39 × 48 × 20 cm) with ∼6 cm of vermiculite layering the bottom of the box at 22°C ± 1°C and high humidity. Animals were fed ad libitum twice weekly with cucumber, lettuce, and sweet potatoes.
For each species, three specimens were removed from the containment area, cleaned using type II water, and placed in an empty and clean plastic container. Animals were then allowed to move freely across a clean sheet of glass for 5 min to avoid collecting adhesive mucus. After that, locomotive mucus was collected from the glass surface using two razor blades (cleaned using ethanol and then type II water), kept in a 2.0-ml polypropylene-graduated centrifuge tube with a cap, stored at room temperature, and subjected to analysis on the day of collection.
Methods
Thermogravimetric analysis
TGA tests were conducted using an MX-50 (A&D Instruments, United Kingdom) moisture content analyser. An alumina crucible (9.5 mm diameter and 14 mm high, Almath Crucibles Ltd., United Kingdom) with 1 ml of fresh native mucus collected as described previously was used for all experiments. The heating rate was 1°C/min, from 25°C to 120°C, with a data interval of 15 s. All experiments were repeated three times per species, with separate samples collected for each test from the same group of individuals at the same time.
UV-vis spectroscopy
With concentrations obtained from TGA analysis, dilutions were prepared for all species, and samples were set to the same concentration of ∼1 mg/ml. Type II water was used as a solvent, and native mucus was collected as described in Materials and Methods. Then, all samples were analysed at room temperature (22°C ± 1°C) using a UV-300 Spectronic Unicam spectrometer (Thermo, United Kingdom) in 1 cm path-length polystyrene cuvettes. Type II water was used as a blank, and mucus samples were manipulated using a 1,000-μL micro-pipette. Scans were performed from 200 to 500 nm at a scan speed of 240 nm/min and 300 steps in total.
We deliberately chose to use offset absorbance wavelength values to determine protein concentration in order to analyse the peak maximum in the UV range because A. fulica mucus shows a shift to lower wavenumbers of its protein absorption peaks, most likely due to differences in phenylalanine (Phe) chromophores. Therefore, concentrations can be estimated for each sample, using maximum absorbance at 215, 225–230, and 260 nm, as follows (Walker, 2002; Liu et al., 2009):
The use of Eqs 1, 2 depends on the presence of peaks at 215 nm with absorbance values <2.0 (Walker, 2002). To corroborate the use of Eqs 1, 2 for each species based on maximum absorption peaks, deconvolution of UV-vis spectra and curve fitting were performed between 200 and 350 nm, and a 10-point multipeak Gaussian fitting (every 10 nm) was performed using Origin software, v2020 (Supplementary Figure S1). Eq. 1 was used for C. aspersum, C. nemoralis, A. ater, A. hortensis, and L. flavus (snails and slugs native from the United Kingdom), whereas Eq. 2 was used for A. fulica.
SDS-PAGE
Gastropod locomotive mucus was collected as described in Materials and Methods. Samples were weighed, placed in 2-ml polypropylene-graduated centrifuge tubes, and diluted with type I water to 5.92 mg/ml, based on the lowest dry weight measured previously by TGA. Aliquots of 20 µL were taken and mixed with an equal volume of a solution containing sodium dodecyl sulphate and β-mercaptoethanol, according to the method of Laemmli (Walker, 2002). Aliquots of 20 µL of the resulting solutions were resolved on 4%–20% Tris-glycine gel under reducing conditions, using a mini gel tank Invitrogen (Thermo Fisher Scientific, United States) and power supply PS 250 (Hybaid Ltd., United Kingdom), over 100 min at 120 V. Protein bands were visualised by staining with 0.25% Coomassie Brilliant Blue R-250 (VWR Chemicals, United States) and imaged using a Scanjet G2710 scanner (HP Inc., United States). A HiMark Pre-Stained Protein Standard (Thermo Fisher Scientific, United States) was used as a ladder.
Rheology
Rheological measurements (frequency sweep and temperature ramp tests) were performed using an AR-2000 (TA Instruments, Delaware, United States) rheometer equipped with a Peltier temperature-controlled bottom plate and cone-plate upper geometry (diameter 40 mm, cone angle 2°, and 55 μm truncation) or a cross-hatched parallel plates for validation of non-slip conditions (20 mm diameter, 0.7 mm gap). Native mucus, sufficient to completely fill the geometry gap, was placed onto the bottom plate, and the geometry was lowered to the truncation gap at the slowest speed to avoid any undue shearing of the sample. An isolated environmental chamber using a water trap and moistened tissue was used to avoid the native mucus drying out and minimise temperature variations during the tests. Oscillatory frequency sweep tests were performed from 0.6 to 62.8 rad/s (0.1–10 Hz) at 10% strain at 25°C (chosen to be within the linear viscoelastic region of the materials; Supplementary Figure S2). Single-frequency oscillatory tests were then conducted at 0.63 rad/s 10% strain between 15°C and 80°C using a ramp temperature of 5°C/min.
Statistical analysis
Statistical analysis was performed using Student’s t-test and p < 0.05 significance level (Origin software, v2020, United States).
Results and discussion
The results from a comparative study of gastropod locomotive mucus relating compositional, thermal, and functional properties are given in the following sections.
Compositional properties
TGA, UV-vis, and SDS-PAGE were performed to determine the compositional properties of gastropod locomotive mucus. In addition, total solids and protein concentrations were determined using TGA and UV-vis, respectively, to gain an initial and broad appreciation of composition (Table 1).
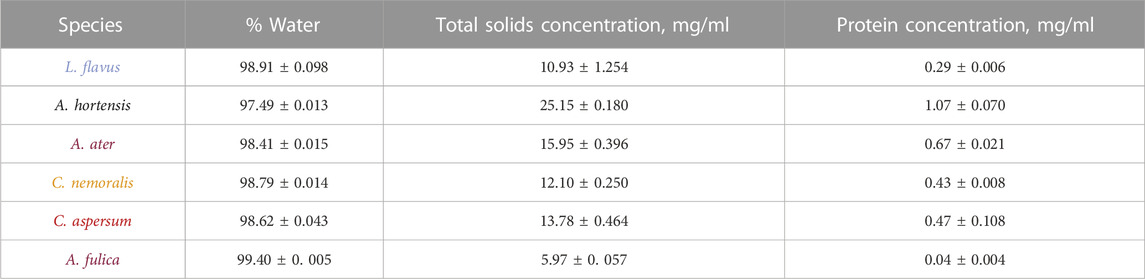
TABLE 1. Table showing % total water and gravimetric total solids concentration; and UV-vis protein concentration measurements for locomotive mucus collected from A. fulica, C. aspersum, C. nemoralis, A. ater, A. hortensis and L. flavus.
Our findings agree with previous studies where pedal mucus in terrestrial snails and slugs has been shown to consist of approximately 91%–98% water, with the remaining mass corresponding to inorganic material and high-molecular-weight organic compounds, mucus polymers, such as proteins and carbohydrates (Denny, 1973, 1984; Hausdorf, 2001; Smith, 2002). For all species tested here, water composition in gastropod mucus lay between 97.4% ± 0.013% in the slug A. hortensis and 99.4% ± 0.005% in the snail A. fulica. The snails C. aspersum and C. nemoralis have the closest percentages of water, 98.62% ± 0.043% and 98.79% ± 0.014, respectively, and notably, they are from the same family Helicidae. Simply inverting the TGA data from % water to solids concentration, the lowest total solids value corresponds to the African snail (A. fulica) with 5.97 mg/ml, whereas the highest value to the slug A. hortensis is 25.15 mg/ml.
UV-vis was used to determine the total protein concentration to determine the constituents of the total solids proportion of mucus. From Figure 1A, concerning the protein-to-total solids ratio, it is apparent that proteins only account for <5% of the total solids present. The remaining solids are assumed to be primarily carbohydrates, as previous studies have found <1% of mucus dry mass to be inorganic (Liudmyla et al., 2022).
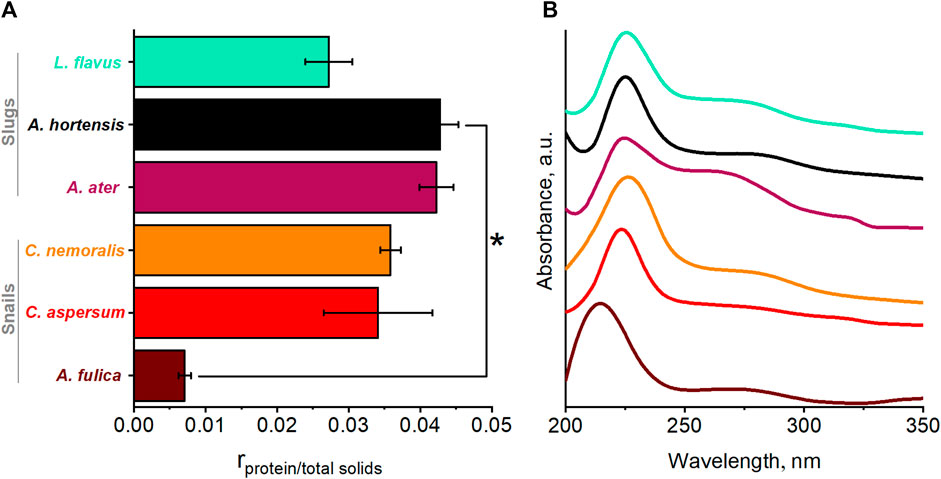
FIGURE 1. (A) Protein-to-total solid concentration ratio and significant differences between A. fulica and A. hortensis (p < 0.05) are indicated with black asterisk brackets. Standard deviation error bars correspond to a series of three experiments per species. (B) UV-vis spectra of native locomotive mucus.
Comparing species, if we first investigate the three slugs, we immediately identify a lower solids-to-protein ratio in the slug L. flavus from the Limacidae family, compared to the other two slugs, A. ater and A. hortensis, both of the Arionidae family. In terms of the three snails, a similar pattern is observed, which overall suggests that members of the same family show similar solid-to-protein ratios. With a value of 0.007, the African snail A. fulica has the lowest ratio, whereas the highest one is for the slug A. hortensis, with 0.042, which represents a significant difference (p = 0.0030) and one we attribute to the amount of aforementioned carbohydrates present in the mucus samples.
Upon closer inspection of UV-vis spectra, it is possible to observe some finer details. Figure 1B depicts a consistent main band at 225–230 nm associated with the presence of proteins for all species. However, there appears to be a shift in this band in the snail A. fulica to 215 nm (assigned to
We analysed our samples using SDS-PAGE to further compare these species and determine the molecular weight of proteins in their locomotive mucus (Figure 2).
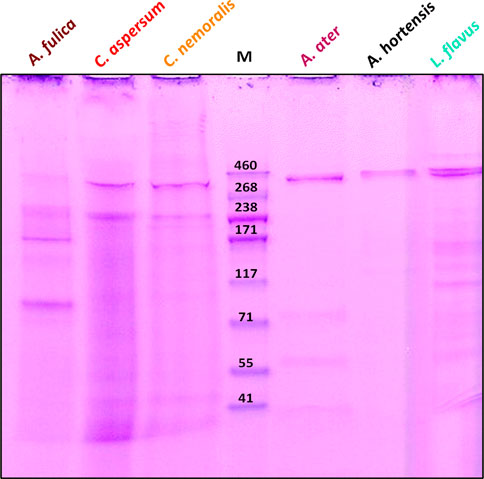
FIGURE 2. Electropherogram of gastropod locomotive mucus. Lines correspond to A. fulica, C. aspersum, C. nemoralis, A. hortensis, A. ater, and L. flavus mucus. Molecular weights corresponding to ladder bands are indicated.
Overall, most bands observed are unreported to date, and there appears to be little consistency across species. However, in general, proteins with molecular weights ranging from 41 to 377 kDa were seen and could be separated into 2–9 main bands (Table 2). Where assignments of protein bands were possible based on previous research, they are named within the table (Ulagesan and Kim, 2018).
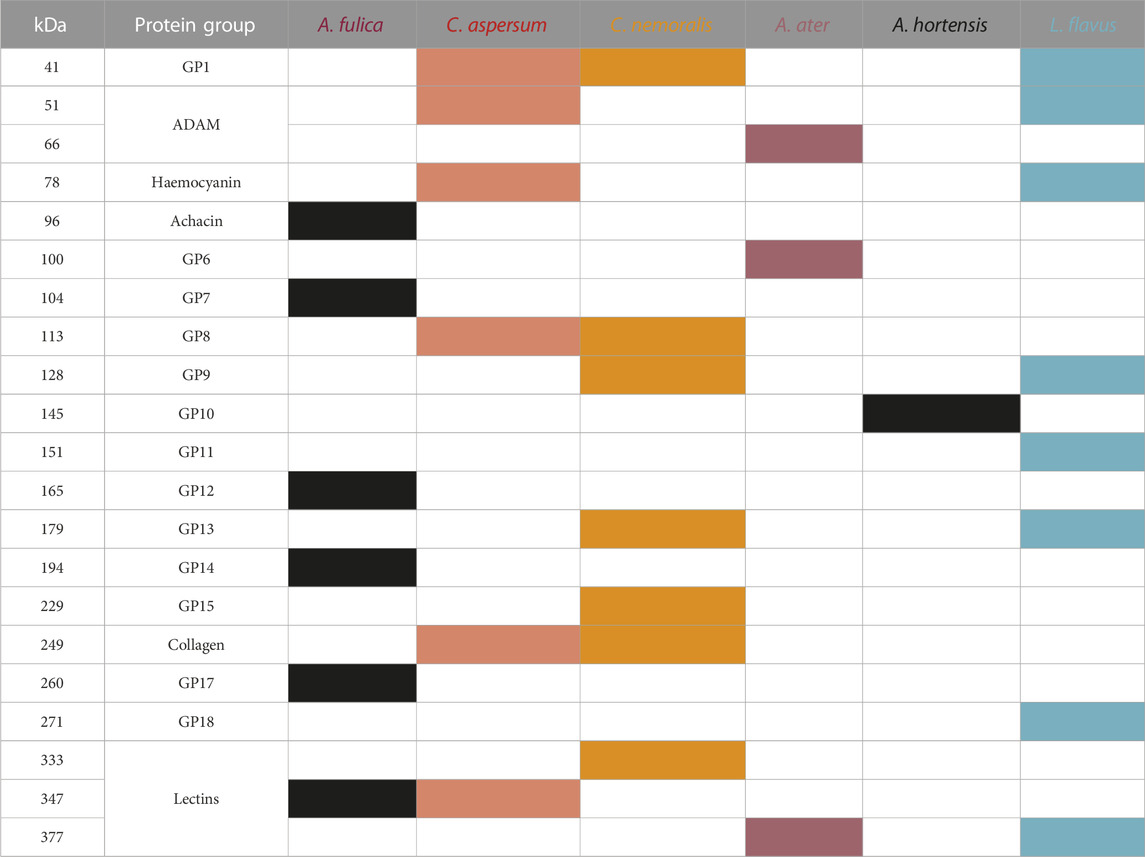
TABLE 2. Identities of specimens in each lane and main bands observed. Coloured cells indicate the presence of that protein group (GP). GP1, GP6–GP15, GP17 and GP18 are unknown protein groups of bands not identified before in gastropod mucus.
Lower-molecular-weight proteins identified include haemocyanin at 78 kDa, normally present in the haemolymph (Huang et al., 2008) and reported for C. aspersum, which well ties our findings (Ballard et al., 2021). Achacin at 96 kDa, which is the glycoprotein associated with the antimicrobial activity of the African snail mucus and only seen in our A. fulica sample (Ogawa et al., 1999; Cilia and Fratini, 2018), and the metalloproteinase-like ADAM family of proteins between 66–67 and 52–51 kDa, which play a key role in protein degradation (Edwards et al., 2009; Ballard et al., 2021), which were evident in A. ater and C. aspersum. Interestingly, collagen (∼250 kDa) was observed for C. aspersum and C. nemoralis and is most likely to be variants II, III, and XI, which are mostly related to locomotive mucus. However, it could be collagens IV, IX, and X, which have been found in the locomotive, adhesive, and defensive mucus (Sripriya and Kumar, 2015; Ballard et al., 2021; Tachapuripunya et al., 2021; Cerullo et al., 2022).
Finally, a ∼350-kDa high-molecular-weight protein band was present in all our samples. Previously, this has been attributed to lectins (proteins that bind to carbohydrates) (Ito et al., 2011) but could readily be an unidentified mucus protein (McDermott et al., 2021). We believe that this protein could be a primary contributor to the rheological properties of mucus, given its length and possible carbohydrate-binding interactions (Fung, Gallego Lazo, and Smith, 2019).
Functional properties
To relate mucus composition to function (i.e., flow properties and stability), we conducted a range of rheological tests. First, a rheological “fingerprint” was captured for each species where linear viscoelastic moduli were measured across a range of timescales of deformation (obtaining the storage modulus G′ and loss modulus G′′, Figure 3) (detailed information is included in Supplementary Figure S3). Before venturing into a discussion of these results, it is important to note that we have accounted for experimental artefacts that can arise in rheological measurements when undertaking analysis on low-viscosity samples as a result of instrument and sample inertia and low-torque limits (Ewoldt et al., 2015) (Supplementary Figure S4 and Figure 3).
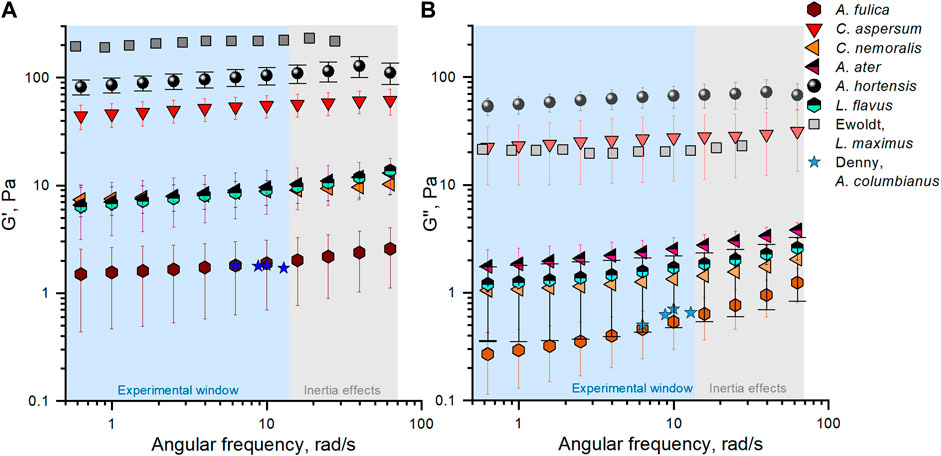
FIGURE 3. Average linear viscoelastic moduli, (A) G′ and (B) G′′, of locomotive mucus from A. fulica (brown hexagon), C. aspersum (red down triangle), C. nemoralis (orange left triangle), A. ater (magenta half up left triangle), A. hortensis (black sphere), and L. flavus (cyan half up hexagon), including digitised data reported previously by Ewoldt et al. (2007) and Denny and Gosline (1980), corresponding to L. maximus (grey square), and A. columbianus (blue star) for comparison purposes. Standard deviation error bars correspond to a series of three experiments per species. Two main regions are indicated in blue and grey, corresponding to the experimental window (reliable data) and inertia effects.
Our analysis indicates that all mucus samples behave as viscoelastic gels over the range of angular frequencies tested, with G′ > G′′ (Peters et al., 2021), a finding similar to mammalian mucus (Innes et al., 2009; Schuster et al., 2013; Murgia et al., 2016; Huck et al., 2019; Peters et al., 2021). The results from the previous two studies analysing mucus from slugs L. maximus and A. columbianus are included in Figure 3 for further reference (Denny and Gosline, 1980; Ewoldt et al., 2007). Both pioneering works reported modulus values within the overall range we report for our species, although these studies collected and tested their mucus samples under conditions different from ours (see Methods). For example, Ewoldt et al. (2007) tested slug mucus using a 2 cm plate with sandpaper, 200 µm gap, and at 22°C. Denny and Gosline (1980) collected slug mucus using a glass rod, and tests were performed at temperatures between 21°C and 24°C. In summary, we note that the overall spread of rheological properties reported for locomotive mucus to date is considerable, with a two-order-of-magnitude variation in modulus, but this is not unsurprising given the infancy of this field (Holland et al., 2006).
It is also possible to extract a complex viscosity (resistance to flow) from these fingerprinting tests (Figure 4). Akin to many polymeric materials, gastropod mucus clearly exhibits non-Newtonian shear thinning fluid properties (Perez-vilar and Hill, 1999), with viscosity dependent on the deformation rate. This was also the case when synthetic mucus was tested and used as a viscoelastic biofluid (Aghakhani et al., 2022). Our results show that A. fulica exhibits the lowest overall complex viscosity, whereas A. hortensis represents the highest. To corroborate if slippage occurred in our measurements due to the use of a smooth cone plate geometry, we tested C. aspersum locomotive mucus using a 20 mm cross-hatched geometry (Ewoldt et al., 2015). Results indicated values in similar ranges when comparing cone-plate geometry measurements with those performed under a rough surface (Supplementary Figure S5).
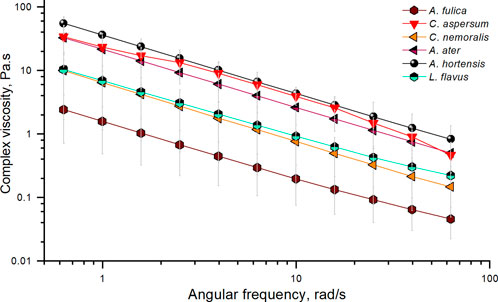
FIGURE 4. Complex viscosity vs. angular frequency of A. fulica (brown hexagon), C. aspersum (red down triangle), C. nemoralis (orange left triangle), A. ater (magenta half up left triangle), A. hortensis (black sphere), and L. flavus (cyan half up hexagon). Standard deviation error bars correspond to a series of three experiments per species.
Figure 5 attempts to establish whether this rheological diversity is related to the total solids or protein content in gastropod mucus. There is a positive relationship between solid concentration and viscosity (Figure 5A). However, the same is seen for protein concentration (Figure 5B), indicating that proteins are most likely linked to mucus rheology.
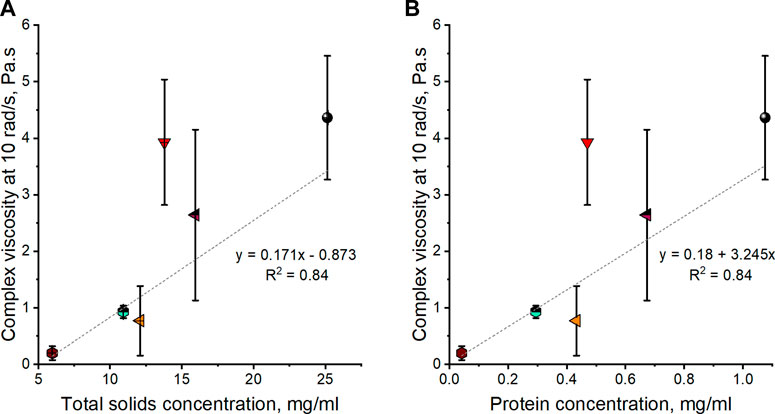
FIGURE 5. (A) Average complex viscosity at 10 rad/s vs. total solid concentration. (B) Average complex viscosity at 10 rad/s vs. protein concentration of A. fulica (brown hexagon), C. aspersum (red down triangle), C. nemoralis (orange left triangle), A. ater (magenta half up left triangle), A. hortensis (black sphere), and L. flavus (cyan half up hexagon). Standard deviation error bars correspond to a series of three experiments per species.
If previous assumptions and observations on different kinds of mucus are valid, and mucus polymers and their protein constituents (e.g., lectins and their carbohydrate-binding ability) are the main factors governing the rheological behaviour of mucus (Bansil and Turner, 2006; Hill et al., 2014), then an examination of viscosity as a function of temperature could add valuable information. The hypothesis is that thermal transitions, such as protein glass transition and, ultimately, denaturation, disrupt overall gel strength and subsequent flow properties. Figure 6 shows the average complex viscosity versus temperature. As a precaution, Lauger et al. (2002) described the importance of obtaining reliable rheological data when performing tests as a function of temperature. Therefore, we complemented our temperature ramp tests with experiments performed at a lower heating rate, using a cross-hatched geometry and the same environmentally isolated chamber to account for any temperature gradients that may arise from our tests. Reassuringly, our findings showed no major differences compared with the data in Figure 6 (Supplementary Figure S6).
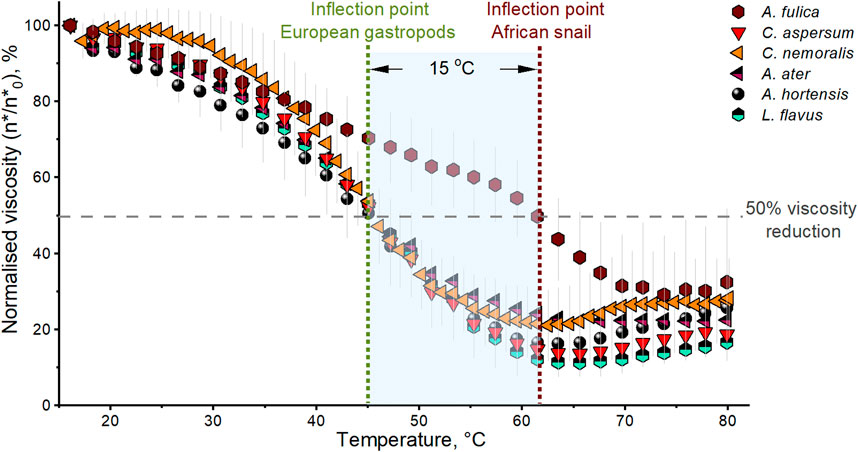
FIGURE 6. Normalised complex viscosity vs. temperature of native locomotive mucus from A. fulica (brown hexagon), C. aspersum (red down triangle), C. nemoralis (orange left triangle), A. ater (magenta half up left triangle), A. hortensis (black sphere), and L. flavus (cyan half up hexagon). Standard deviation error bars correspond to a series of three experiments (each normalised to its first value of complex viscosity taken at 0.63 rad/s, η*0) for all the species.
Figure 6 shows that mucus across all species retains up to 80% of its original viscosity from 15°C to 30°C presumably to maintain consistency in flow behaviour during the natural habitat temperature ranges these animals encounter. Between 45°C and 48°C, a 50% reduction in viscosity is observed for A. hortensis, A. ater, C. aspersum, and L. flavus. A. fulica is an exception; compared to the other European species, it continues a steady decline in viscosity until 60°C before quickly dropping. Once viscosity has fallen for all species, potentially as a result of the change in protein conformation, it appears to plateau before beginning to rise again slightly. This lower plateau represents the minimum level of viscosity and, thus, minimal mucus polymer molecular interactions in the mucus. We attribute the subsequent rise to one of two scenarios: the unlikely possibility that the samples are slightly drying out and forming a skin at the edges of the gap, or there may be protein structures undergoing denaturation, leading to dehydration and aggregation, resulting in the reformation of a newer, phase-separated, and stronger gel structure, consistent with recent FTIR observations (Barajas-Ledesma and Holland, 2023).
There is a clear difference between A. fulica and the other five species. Mucus from this snail exhibits a higher stability in viscosity when the temperature increases, and the drastic viscosity drop occurs at 15°C higher than the other samples. Here, it is also important to remember that Figure 6 depicts relative changes based on the original viscosity of the mucus, and A. fulica already has the lowest viscosity tested (Figure 4). Hence, this variation could result from A. fulica having the highest water content of all mucus tested and the lowest ratio of proteins to total solids. In this instance, the other mucus polymer components, such as carbohydrates, could contribute more to the locomotive mucus viscosity of A. fulica and hence the different thermal response or the smaller amount of proteins present could have a higher thermal tolerance by comparison (Towns, 1995; Petrou and Crouzier, 2018).
This can be rationalised through an evolutionary context, as whilst terrestrial snails and slugs stem from the same common ancestor in the Stylommatophora clade (Wade et al., 2006), it is highly likely that specific adaptations of mucus to certain habitats have arisen. This is evidenced in a recent FTIR study of mucus biodiversity, showing that species identification can be made based on the unique chemical fingerprint of locomotive mucus alone (Barajas-Ledesma and Holland, 2023). In our results, A. fulica has a more stable mucus under thermal stress, implying its mucus polymers maintain their function over a wider range of temperatures, which is consistent with the observation that this African species is native to a region with higher average temperature compared to the other five European species included in this study (Albuquerque et al., 2008). Furthermore, FTIR spectra of A. fulica indicates that its Amide III region differs from the other species tested, suggesting the specialisation of the proteins present in the mucus (Barajas-Ledesma and Holland, 2023).
Conclusion
This study takes some initial steps toward the broad characterisation of a range of compositional and functional properties of gastropod locomotive mucus. It reports and discusses for the first-time fundamental aspects of the material, such as concentration and protein content, and uses this information to account for differences in the mechanical or flow properties of the mucus. The different compositional and rheological tests presented here support our hypothesis that mucus may have been adapted to specific environments. In particular, the marked differences between the African snail A. fulica and the European snails and slugs suggest that not only can the morphology or genotype of gastropods be used to infer evolutionary relationships but also the materials they produce can be used.
Data availability statement
The datasets presented in this study can be found in online repositories. The names of the repository/repositories and accession number(s) can be found in the article/Supplementary Material.
Author contributions
EB-L conducted the experimental work and data analysis. The manuscript was written through contributions of all authors. All authors contributed to the article and approved the submitted version.
Funding
This work was supported by the Consejo Nacional de Ciencia y Tecnología (CONACYT), Mexico (Grant No. 472130), and the UKRI EPSRC (EP/K005693/1).
Conflict of interest
The authors declare that the research was conducted in the absence of any commercial or financial relationships that could be construed as a potential conflict of interest.
Publisher’s note
All claims expressed in this article are solely those of the authors and do not necessarily represent those of their affiliated organizations, or those of the publisher, the editors, and the reviewers. Any product that may be evaluated in this article, or claim that may be made by its manufacturer, is not guaranteed or endorsed by the publisher.
Supplementary material
The Supplementary Material for this article can be found online at: https://www.frontiersin.org/articles/10.3389/frsfm.2023.1201511/full#supplementary-material
References
Aghakhani, A., Pena-Francesch, A., Bozuyuk, U., Cetin, H., Wrede, P., and Sitti, M. (2022). High shear rate propulsion of acoustic microrobots in complex biological fluids. Sci. Adv. 8 (10), 1–12. doi:10.1126/sciadv.abm5126
Albuquerque, F. S., Peso-Aguiar, M., and Assunção-Albuquerque, M. (2008). Distribution, feeding behavior and control strategies of the exotic land snail Achatina fulica (Gastropoda: pulmonata) in the northeast of Brazil. Braz. J. Biol. 68 (4), 837–842. doi:10.1590/s1519-69842008000400020
Antosiewicz, J. M., and Shugar, D. (2016). UV–Vis spectroscopy of tyrosine side-groups in studies of protein structure. Part 2: selected applications. Biophys. Rev. 8 (2), 163–177. doi:10.1007/s12551-016-0197-7
Artacho, P., and Nespolo, R. F. (2009). Natural selection reduces energy metabolism in the garden snail, helix aspersa (cornu aspersum). Hoboken, New Jerseyn: Wiley, 1044–1050. doi:10.1111/j.1558-5646.2008.00603.x
Avella, I., Barajas-Ledesma, E., Casewell, N. R., Harrison, R. A., Rowley, P. D., Crittenden, E., et al. (2021). Unexpected lack of specialisation in the flow properties of spitting cobra venom. J. Exp. Biol. 224, 1–11. doi:10.1242/jeb.229229
Ballard, K. R., Klein, A. H., Hayes, R. A., Wang, T., and Cummins, S. F. (2021). The protein and volatile components of trail mucus in the Common Garden Snail, Cornu aspersum. PLoS ONE 16 (5), 02515655–e251620. doi:10.1371/journal.pone.0251565
Bansil, R., and Turner, B. S. (2006). Mucin structure, aggregation, physiological functions and biomedical applications. Curr. Opin. Colloid Interface Sci. 11 (2–3), 164–170. doi:10.1016/j.cocis.2005.11.001
Bansil, R., and Turner, B. S. (2018). The biology of mucus: composition, synthesis and organization. Adv. Drug Deliv. Rev. 124, 3–15. doi:10.1016/j.addr.2017.09.023
Barajas-Ledesma, E., and Holland, C. (2023). Analysis, classification and identification of gastropod locomotive mucus by Fourier Transform Infrared Spectroscopy.
Braun, M., Menges, M., Opoku, F., and Smith, A. M. (2013). The relative contribution of calcium, zinc and oxidation-based cross-links to the stiffness of Arion subfuscus glue. J. Exp. Biol. 216 (8), 1475–1483. doi:10.1242/jeb.077149
Bretz, D. D., and Dimock, R. V. (1983). Behaviorally important characteristics of the mucous trail of the marine gastropod Ilyanassa Obsoleta (Say). J. Exp. Mar. Biol. Ecol. 71 (2), 181–191. doi:10.1016/0022-0981(93)90072-V
Butnarasu, C., Barbero, N., Pacheco, D., Petrini, P., and Visentin, S. (2019). Mucin binding to therapeutic molecules: the case of antimicrobial agents used in cystic fibrosis. Int. J. Pharm. 564, 136–144. doi:10.1016/j.ijpharm.2019.04.032
Casewell, N. R., Wüster, W., Vonk, F. J., Harrison, R. A., and Fry, B. G. (2013). Complex cocktails: the evolutionary novelty of venoms. Trends Ecol. Evol. 28 (4), 219–229. doi:10.1016/j.tree.2012.10.020
Cerullo, A. R., McDermott, M. B., Pepi, L. E., Liu, Z-L., Barry, D., Zhang, S., et al. (2022). Comparative mucomic analysis of three functionally distinct cornu aspersum secretions. bioRxiv 11 (16), 516827. doi:10.1101/2022.11.16.516827v1
Chase, R., Croll, R. P., and Zeichner, L. L. (1980). Aggregation in snails, Achatina fulica. Behav. neural Biol. 30 (2), 218–230. doi:10.1016/S0163-1047(80)91101-2
Cilia, G., and Fratini, F. (2018). Antimicrobial properties of terrestrial snail and slug mucus. J. Complementary Integr. Med. 15, 1–10. doi:10.1515/jcim-2017-0168
Cottrell, J. M., Henderson, I. F., and Wright, D. J. (1994). Studies on the glycosaminoglycan component of trail mucus from the terrestrial slug, Arion ater L. Comp. Biochem. Physiology -- Part B Biochem.107 (2), 285–296. doi:10.1016/0305-0491(94)90051-5
Crammer, J. L., and Neuberger, A. (1943). The state of tyrosine in egg albumin and in insulin as determined by spectrophotometric titration. Biochem. J. 37 (2), 302–310. doi:10.1042/bj0370302
Davies, M. S., and Hatcher, A. M. (1999). Limpet mucus as a depuration route and potential biomonitor. Ecotoxicology 8 (3), 177–187. doi:10.1023/A:1026492230488
Davies, M. S., and Hutchinson, S. J. (1995). Crystalline calcium in littorinid mucus trails. Hydrobiologia 309 (1–3), 117–121. doi:10.1007/BF00014478
Davies, M. S., Jones, H. D., and Hawkins, S. J. (1992). Physical factors affecting the fate of pedal mucus produced by the common limpet patella vulgata. J. Mar. Biol. Assoc. U. K. 72 (3), 633–643. doi:10.1017/S0025315400059403
Denny, M. W., and Gosline, J. M. (1980). The physical properties of the pedal mucus of the terrestrial slug, ariolimax columbianus. J. Exp. Biol. 88 (1), 375–394. doi:10.1242/jeb.88.1.375
Denny, M. W. (1984). Mechanical properties of pedal mucus and their consequences for gastropod structure and performance. Integr. Comp. Biol. 24 (1), 23–36. doi:10.1093/icb/24.1.23
Denny, M. W. (1980). The role of gastropod pedal mucus in locomotion. Nature 285, 160–161. doi:10.1038/285160a0
Denny, M. W. (1973). “The role of mucus in the locomotion and adhesion of the pulmonate slug, ariolimax columbianus,”. Ph. D. thesis (Durham, North Carolina: Duke University).
Deyrup-Olsen, I., Luchtel, D. L., and Martin, a. W. (1983). Components of mucus of terrestrial slugs (Gastropoda). Am. J. physiology 245 (3), R448–R452. doi:10.1152/ajpregu.1983.245.3.r448
Dhanisha, S. S., Guruvayoorappan, C., Drishya, S., and Abeesh, P. (2018). Mucins: structural diversity, biosynthesis, its role in pathogenesis and as possible therapeutic targets. Crit. Rev. Oncology/Hematology 122, 98–122. doi:10.1016/j.critrevonc.2017.12.006
Edwards, D. R., Handsley, M. M., and Pennington, C. J. (2009). The ADAM metalloproteinases. Mol. Aspects Med. 29 (5), 258–289. doi:10.1016/j.mam.2008.08.001
Ewoldt, R. H., Clasen, C., Hosoi, A. E., and McKinley, G. H. (2007). Rheological fingerprinting of gastropod pedal mucus and synthetic complex fluids for biomimicking adhesive locomotion. Soft Matter 3 (5), 634–643. doi:10.1039/b615546d
Ewoldt, R. H., Johnston, H., Michael, T., and Caretta, L. M. (2015). “Experimental challenges of shear rheology: how to avoid bad data,” in Complex fluids in biological systems. Editor S. Spagnolie (Berlin, Germany: Springer Biological Engineering Series), 207–241. doi:10.1007/978-1-4939-2065-5_6
Ewoldt, R. H., Hosoi, A. E., and McKinley, G. H. (2009). Nonlinear viscoelastic biomaterials: meaningful characterization and engineering inspiration. Integr. Comp. Biol. 49 (1), 40–50. doi:10.1093/icb/icp010
Flórez, L. V., Biedermann, P. H. W., Engl, T., and Kaltenpoth, M. (2015). Defensive symbioses of animals with prokaryotic and eukaryotic microorganisms. Nat. Product. Rep. 32 (7), 904–936. doi:10.1039/c5np00010f
Fung, T. M., Gallego Lazo, C., and Smith, A. M. (2019). Elasticity and energy dissipation in the double network hydrogel adhesive of the slug Arion subfuscus. Philosophical Trans. R. Soc. B Biol. Sci. 374 (1784), 1–8. doi:10.1098/rstb.2019.0201
Guo, F., Huang, Z. b., Huang, M. q., Zhao, J., and Ke, C. h. (2009). Effects of small abalone, Haliotis diversicolor, pedal mucus on bacterial growth, attachment, biofilm formation and community structure. Aquaculture 293 (1–2), 35–41. doi:10.1016/j.aquaculture.2009.03.033
Hausdorf, B. (2001). Macroevolution in progress: competition between semislugs and slugs resulting in ecological displacement and ecological release. Biol. J. Linn. Soc. 74 (3), 387–395. doi:10.1006/bijl.2001.0591
He, Z. P., Dai, X. B., Zhang, S., Zhi, T. T., Lun, Z. R., Wu, Z. D., et al. (2016). Complete mitochondrial genome of the giant african snail, achatina fulica (Mollusca: achatinidae): A novel location of putative control regions (cr) in the mitogenome within pulmonate species. Mitochondrial DNA 27 (2), 1084–1085. doi:10.3109/19401736.2014.930833
Hill, D. B., Vasquez, P. A., Mellnik, J., McKinley, S. A., Vose, A., Mu, F., et al. (2014). A biophysical basis for mucus solids concentration as a candidate biomarker for airways disease. PLoS ONE 9 (2), 876811–e87711. doi:10.1371/journal.pone.0087681
Holland, C., Terry, A. E., Porter, D., and Vollrath, F. (2006). Comparing the rheology of native spider and silkworm spinning dope. Nat. Mater. 5 (11), 870–874. doi:10.1038/nmat1762
Huang, B., Zhang, J., and Xiang, J. (2008). Purification and primary identification of haemocyanin in the Chinese shrimp, fenneropenaeus chinensis (Decapoda, penaeoidea). Crustaceana 81 (7), 769–780. doi:10.1163/156854008784771630
Huck, B. C., Hartwig, O., Biehl, A., Schwarzkopf, K., Wagner, C., Loretz, B., et al. (2019). Macro- and microrheological properties of mucus surrogates in comparison to native intestinal and pulmonary mucus. Biomacromolecules 20 (9), 3504–3512. doi:10.1021/acs.biomac.9b00780
Innes, A. L., Carrington, S. D., Thornton, D. J., Kirkham, S., Rousseau, K., Dougherty, R. H., et al. (2009). Ex vivo sputum analysis reveals impairment of protease-dependent mucus degradation by plasma proteins in acute asthma. Am. J. Respir. Crit. Care Med. 180 (3), 203–210. doi:10.1164/rccm.200807-1056OC
Ito, S., Shimizu, M., Nagatsuka, M., Kitajima, S., Honda, M., Tsuchiya, T., et al. (2011). High molecular weight lectin isolated from the mucus of the giant african snail achatina fulica. Biosci. Biotechnol. Biochem. 75 (1), 20–25. doi:10.1271/bbb.100389
Kim, Y. S., Jo, Y. Y., Chang, I. M., Toida, T., Park, Y., and Linhardt, R. J. (1996). A new glycosaminoglycan from the giant african snail Achatina fulica. J. Biol. Chem. 271 (20), 11750–11755. doi:10.1074/jbc.271.20.11750
Klöppel, A., Brümmer, F., Schwabe, D., and Morlock, G. (2013) ‘Detection of bioactive compounds in the mucus nets of dendropoma maxima, sowerby 1825 (prosobranch gastropod vermetidae, Mollusca)’, J. Mar. Biol., 2013, pp. 1–9. doi:10.1155/2013/283506
Lai, S. K., Wang, Y. Y., Cone, R., Wirtz, D., and Hanes, J. (2009). Altering mucus rheology to “solidify” human mucus at the nanoscale. PLoS ONE 4 (1), e4294–e4296. doi:10.1371/journal.pone.0004294
Lauger, J., Wollny, K., Bernzen, M., and Raffer, G. (2002). New developments for temperature control of rotational rheometers. Annu. Trans. Nordic Soc. Rheology 10, 147–154. doi:10.1165/96606
Lesniak, W. G., Jyoti, A., Mishra, M. K., Louissaint, N., Romero, R., Chugani, D. C., et al. (2013). Concurrent quantification of tryptophan and its major metabolites. Anal. Biochem. 443 (2), 222–231. doi:10.1016/j.ab.2013.09.001
Liu, P. F., Avramova, L. V., and Park, C. (2009). Revisiting absorbance at 230 nm as a protein unfolding probe. Anal. Biochem. 389 (2), 165–170. doi:10.1016/j.ab.2009.03.028
Liudmyla, K., Olena, C., and Nadiia, S. (2022). Chemical properties of Helix aspersa mucus as a component of cosmetics and pharmaceutical products. Mater. Today Proc. 62 (P15), 7650–7653. doi:10.1016/j.matpr.2022.02.217
Luchtel, D. L., Deyrup-Olsen, I., and Martin, A. W. (1991). Ultrastructure and lysis of mucin-containing granules in epidermal secretions of the terrestrial slug Ariolimax columbianus (Mollusca: gastropoda:Pulmonata). Cell. Tissue Res. 266 (2), 375–383. doi:10.1007/BF00318193
McDermott, M., Cerullo, A. R., Parziale, J., Achrak, E., Sultana, S., Ferd, J., et al. (2021). Advancing discovery of snail mucins function and application. Front. Bioeng. Biotechnol. 9, 1–9. doi:10.3389/fbioe.2021.734023
Moran, A., Gonzalez, R., and Leon, O. (1983). Studies of the effects of phenylalanine and pH on pyruvate kinase from the muscle of the sea mollusc Concholepas concholepas. Comp. Biochem. Physiology 75 (4), 603–605. doi:10.1016/0305-0491(83)90103-7
Murgia, X., Pawelzyk, P., Schaefer, U. F., Wagner, C., Willenbacher, N., and Lehr, C. M. (2016). Size-limited penetration of nanoparticles into porcine respiratory mucus after aerosol deposition. Biomacromolecules 17 (4), 1536–1542. doi:10.1021/acs.biomac.6b00164
Newar, J., and Ghatak, A. (2015). Studies on the adhesive property of snail adhesive mucus. Langmuir 31, 12155–12160. doi:10.1021/acs.langmuir.5b03498
Ogawa, M., Nakamura, S., Atsuchi, T., Tamiya, T., Tsuchiya, T., and Nakai, S. (1999). Macromolecular antimicrobial glycoprotein, achacin, expressed in a methylotrophic yeast Pichia pastoris. FEBS Lett. 448 (1), 41–44. doi:10.1016/S0014-5793(99)00327-0
O’Hanlon, A., Williams, C. D., and Gormally, M. J. (2019). Terrestrial slugs (Mollusca: gastropoda) share common anti-predator defence mechanisms but their expression differs among species. J. Zoology 307 (3), 203–214. doi:10.1111/jzo.12635
Pakay, J. L., Withers, P. C., Hobbs, A. A., and Guppy, M. (2002). In vivo downregulation of protein synthesis in the snail Helix apersa during estivation. Am. J. Physiology - Regul. Integr. Comp. Physiology 283 (1 52-1), 197–204. doi:10.1152/ajpregu.00636.2001
Pawlicki, J. M., Pease, L. B., Pierce, C. M., Startz, T. P., Zhang, Y., and Smith, A. M. (2004). The effect of molluscan glue proteins on gel mechanics. J. Exp. Biol. 207 (7), 1127–1135. doi:10.1242/jeb.00859
Peck, L. S., Prothero-Thomas, E., and Hough, N. (1993). Pedal mucus production by the Antarctic limpet Nacella concinna (Strebel, 1908). J. Exp. Mar. Biol. Ecol. 174 (2), 177–192. doi:10.1016/0022-0981(93)90016-H
Perez-vilar, J., and Hill, R. L. (1999). The structure and assembly of secreted mucins. J. Biol. Chem. 274 (45), 31751–31754. doi:10.1074/jbc.274.45.31751
Peters, G., Wendler, O., Böhringer, D., Gostian, A. O., Müller, S. K., Canziani, H., et al. (2021). Human laryngeal mucus from the vocal folds: rheological characterization by particle tracking microrheology and oscillatory shear rheology. Appl. Sci. Switz. 11 (7), 3011–3015. doi:10.3390/app11073011
Petrou, G., and Crouzier, T. (2018). Mucins as multifunctional building blocks of biomaterials. Biomaterials Sci. 6 (9), 2282–2297. doi:10.1039/c8bm00471d
Radicioni, G., Cao, R., Carpenter, J., Ford, A., Wang, T., Li, Y., et al. (2016).,The innate immune properties of airway mucosal surfaces are regulated by dynamic interactions between mucins and interacting proteins: the mucin interactome Mucosal Immunol, 9(6), 1442–1454. doi:10.1038/mi.2016.27
Sallam, A., and El-Wakeil, N. (2012). “Biological and ecological studies on land snails and their control,” in Integrated pest management and pest control - current and future tactics (London, UK: Intechopen). doi:10.5772/29701
Schmid, F.-X. (2001). Biological macromolecules: UV-Visible spectrophotometry. Hoboken, New Jerseyn: Wiley, 1–4. doi:10.1038/npg.els.0003142
Schuster, B. S., Suk, J. S., Woodworth, G. F., and Hanes, J. (2013). Nanoparticle diffusion in respiratory mucus from humans without lung disease. Biomaterials 34 (13), 3439–3446. doi:10.1016/j.biomaterials.2013.01.064
Skingsley, D. R., White, A. J., and Weston, A. (2000). Analysis of pulmonate mucus by infrared spectroscopy. J. Molluscan Stud. 66 (3), 363–372. doi:10.1093/mollus/66.3.363
Smith, A. M., and Morin, M. C. (2002). Biochemical differences between trail mucus and adhesive mucus from marsh periwinkle snails. Biol. Bull. 203 (3), 338–346. doi:10.2307/1543576
Smith, A. M., Quick, T. J., and St. Peter, R. L. (1999). Differences in the composition of adhesive and non-adhesive mucus from the limpet Lottia limatula. Biol. Bull. 196 (1), 34–44. doi:10.2307/1543164
Smith, A. M., Robinson, T., Salt, M., Hamilton, K., Silvia, B., and Blasiak, R. (2009). Robust cross-links in molluscan adhesive gels: testing for contributions from hydrophobic and electrostatic interactions. Comp. Biochem. Physiol. B Biochem. Mol. Biol. 152 (2), 110–117. doi:10.1016/j.cbpb.2008.10.004
Smith, A. M. (2002). The structure and function of adhesive gels from invertebrates. Integr. Comp. Biol. 42 (6), 1164–1171. doi:10.1093/icb/42.6.1164
Sripriya, R., and Kumar, R. (2015). A novel enzymatic method for preparation and characterization of collagen film from swim bladder of fish rohu (Labeo rohita). Food Nutr. Sci. 06 (15), 1468–1478. doi:10.4236/fns.2015.615151
Struthers, M., Rosair, G., Buckman, J., and Viney, C. (2002). The physical and chemical microstructure of the Achatina fulica epiphragm. J. Molluscan. Stud. 68, 165–171.
Tachapuripunya, V., Roytrakul, S., Chumnanpuen, P., and E-kobon, T. (2021). Unveiling putative functions of mucus proteins and their tryptic peptides in seven gastropod species using comparative proteomics and machine learning-based bioinformatics predictions. Molecules 26 (11), 3475–3518. doi:10.3390/molecules26113475
Thornton, D. J. (2004). From mucins to mucus: toward a more coherent understanding of this essential barrier. Proc. Am. Thorac. Soc. 1 (1), 54–61. doi:10.1513/pats.2306016
Towns, J. K. (1995). Moisture content in proteins: tts effects and measurement. J. Chromatogr. A 705 (1), 115–127. doi:10.1016/0021-9673(94)01257-F
Ulagesan, S., and Kim, H. (2018). Antibacterial and antifungal activities of proteins extracted from seven different snails. Appl. Sci. 8 (8), 1362. doi:10.3390/app8081362
Wade, C. M., Mordan, P. B., and Naggs, F. (2006). Evolutionary relationships among the Pulmonate land snails and slugs (Pulmonata, Stylommatophora). Biol. J. Linn. Soc. 87 (4), 593–610. doi:10.1111/j.1095-8312.2006.00596.x
Walker, J. M. (2002). Protein protocols the handbook. Humana Totowa, NJ: Springer. doi:10.1385/1592591698
Watz, J., and Nyqvist, D. (2022). Interspecific competition among terrestrial slugs. J. Molluscan Stud. 88 (2). doi:10.1093/mollus/eyac007
Werneke, S. W., Swann, C., Farquharson, L. A., Hamilton, K. S., and Smith, A. M. (2007). The role of metals in molluscan adhesive gels. J. Exp. Biol. 210 (12), 2137–2145. doi:10.1242/jeb.006098
Wilks, A. M., Rabice, S. R., Garbacz, H. S., Harro, C. C., and Smith, A. M. (2015). Double-network gels and the toughness of terrestrial slug glue. J. Exp. Biol. 218 (19), 3128–3137. doi:10.1242/jeb.128991
Yoo, H., Widhalm, J. R., Qian, Y., Maeda, H., Cooper, B. R., Jannasch, A. S., et al. (2013). An alternative pathway contributes to phenylalanine biosynthesis in plants via a cytosolic tyrosine:phenylpyruvate aminotransferase. Nat. Commun. 4, 2833. doi:10.1038/ncomms3833
Keywords: ecto-secretion, gastropods, mucus polymers, protein–carbohydrate interactions, function
Citation: Barajas-Ledesma E and Holland C (2023) Probing the compositional and rheological properties of gastropod locomotive mucus. Front. Soft Matter 3:1201511. doi: 10.3389/frsfm.2023.1201511
Received: 06 April 2023; Accepted: 01 August 2023;
Published: 14 September 2023.
Edited by:
Rama Bansil, Boston University, United StatesReviewed by:
Yu Ogawa, UPR5301 Centre de Recherches sur les Macromolecules Végétales (CERMAV), FranceSuvi Arola, VTT Technical Research Centre of Finland Ltd, Finland
Sean J. Blamires, University of New South Wales, Australia
Copyright © 2023 Barajas-Ledesma and Holland. This is an open-access article distributed under the terms of the Creative Commons Attribution License (CC BY). The use, distribution or reproduction in other forums is permitted, provided the original author(s) and the copyright owner(s) are credited and that the original publication in this journal is cited, in accordance with accepted academic practice. No use, distribution or reproduction is permitted which does not comply with these terms.
*Correspondence: Edgar Barajas-Ledesma, ZS5iYXJhamFzLmxlZGVzbWFAc2hlZmZpZWxkLmFjLnVr; Chris Holland, Q2hyaXN0b3BoZXIuaG9sbGFuZEBzaGVmZmllbGQuYWMudWs=