- INRAE, Biologie du Fruit et Pathologie, UMR 1332, University Bordeaux, Villenaved’Ornon, France
We report the stabilization of all-aqueous droplets upon addition of ethanol, which were further transformed into robust capsules. An all-aqueous emulsion consisting of dextran (Dex)-rich droplets in a polyethylene glycol (PEG)-rich continuous phase was used as templates. Addition of ethanol induced gelation of dextran, forming aggregated pellets of poor interest. However, this feature was prevented by initially adding bovine serum albumin (BSA) or positively charged silica particles so that in this case, stable droplets formed upon solvent addition, which no longer coalesced with time. An alternative method consisting of pouring concentrated Dex solution in a mixture of PEG, ethanol and BSA or particles, also afforded stable droplets. These stable droplets were further converted into robust capsules, using carbodiimide chemistry or silylation. We expect this method for preparing capsules to be of interest for various applications in the field of microencapsulation.
1 Introduction
Capsules are of particular interest for microencapsulation applications (Caruso, 2000; Li and Shi, 2014), and in synthetic biology, as compartments or bioreactors (Huang et al., 2013). Many methods are available to produce capsules using sacrificial templates, which include solid templates but also, oil in water (or water in oil) emulsion droplets (Caruso, 2000). This includes colloidosomes (Dinsmore et al., 2002; Thompson et al., 2015), which were initially made in bulk using colloidal particles or microgels reticulated to form a solid shell around the template. Capsules having a protein shell have already been developed, yielding so called proteinosomes (Huang et al., 2013), which use water in oil emulsion droplets as templates. Most other ways to produce capsules are based on polyelectrolyte (PE) shells that are sequentially deposited on templates using the layer by layer technique (Donath et al., 1998; Caruso, 2000; Dai et al., 2002; Discher and Eisenberg, 2002). Here again, the template can be a polymer gel, a solid bead or an oil droplet in water that is further sacrificed, yielding formation of hollow structures. Recently, such capsules were successfully produced by microfluidics, or electrospray device. (Zhu and Hayward, 2008; Liu et al., 2010; Windbergs et al., 2013; Kim et al., 2015; Zhang et al., 2016; Duan et al., 2017; Ma et al., 2018; Chao and Shum, 2020). Methods to produce such capsules in large amounts without using oil or strong solvents used to remove the sacrificial template are still desirable (Douliez et al., 2019; Perro et al., 2022). This has already been achieved in the past by using the solvent shifting process, also known as the ‘Ouzo’ effect (Ganachaud and Katz, 2005; Aubry et al., 2009; Botet, 2011; Lepeltier et al., 2013; Sciortino et al., 2017; Sciortino et al., 2018; Goubault et al., 2020). It consists of mixing solvents such as tetrahydrofurane and water that are macroscopically miscible but form nanodroplets to be used as templates. Nanoparticles were successfully deposited on the surface of such droplets to form hybrid hollow capsules (Sciortino et al., 2017; Sciortino et al., 2018). Immiscible aqueous polymer dispersions, also named aqueous two phase systems (ATPS) or all-in-water emulsions, which includes coacervates, are also promising tools for producing capsules since water-in-water droplets can be used as templates, which can be easily sacrificed (Esquena, 2016; Perro et al., 2022). ATPS form from a large variety of chemicals, including charged or neutral polymers (Keating, 2012), surfactants (Wang and Wang, 2014; Garenne et al., 2016; Douliez et al., 2017) and also mixtures of polyelectrolytes and polymers (Koga et al., 2011; Dzieciol and Mann, 2012). These systems are of increasing interest in synthetic biology as protocells or bioreactors because the droplets that form in solution can spontaneously uptake payloads (Dzieciol and Mann, 2012; Keating, 2012; Buddingh and van Hest, 2017; Chao and Shum, 2020; Abbas et al., 2021). ATPS droplets may coalesce with time yielding macroscopic phase separation. Then, strong efforts have been developed to stabilize droplets and prevent coalescence by adding chemicals that position at the droplet interface (Nguyen et al., 2013; Dewey et al., 2014; Dora Tang et al., 2014; de Freitas et al., 2016; Gonzalez-Jordan et al., 2016; Ma et al., 2016; Peddireddy et al., 2016; Song et al., 2016; Hann et al., 2017a; Hann et al., 2017b; Mason et al., 2017; Ma et al., 2018; Douliez et al., 2019; Tea et al., 2019; Abbas et al., 2021; Coudon et al., 2022; Perro et al., 2022). In most cases, the material forming the shell of droplets (preventing coalescence) may need to be gelled or cross-linked to yield robust capsules otherwise they break upon dilution in water, due to the variation of osmotic pressure. This is known as the demulsification control experiment (DCE) (Zhang et al., 2019), which allows determining whether robust capsules are formed or not and if chemicals that were added only to the interface allow to stabilize droplets. ATPS have already been successfully used to produce such robust capsules by converting coacervates into membranous hollow spheres (Williams David et al., 2014), colloidosomes using latex beads (Douliez et al., 2018a) or dopamine-based particles (Zhang et al., 2019) and also capsules having a gelatin shell (Douliez et al., 2018b). Recently, polyelectrolyte-based microcapsules were produced by using ATPS by microfluidics or electrospray, preventing the use of oil as sacrificial templates and/or strong solvents (Wu et al., 2010; Cheung Shum et al., 2012; Song et al., 2013; Bakry et al., 2015; Kim et al., 2015; Song et al., 2015; Kim et al., 2016; Zhang et al., 2016; Hann et al., 2017a; Hann et al., 2017b; Duan et al., 2017; Deng et al., 2019; Chao and Shum, 2020).
Here, we report the production of robust capsules by combining the solvent shifting process and all-in-water emulsions. As shown in Scheme 1, dextran-enriched droplets in a PEG-enriched continuous phase are used as templates whereas the presence of BSA or positively charged silica particles prevents aggregation of Dex upon solvent shifting, further affording robust capsules. We also developed an alternative method based on mixing a Dex-rich solution in PEG/EtOH containing proteins or particles, which also yielded stable droplets.
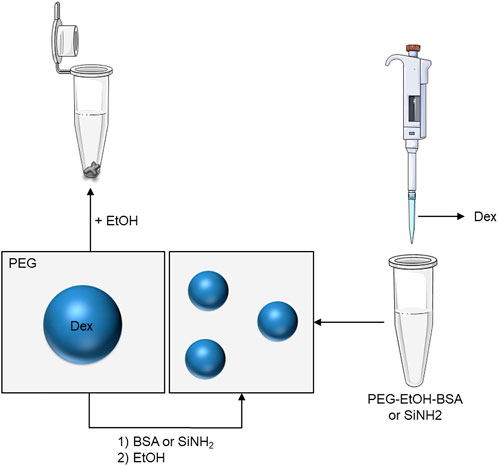
SCHEME 1. Schematic representation of the production of stable droplets from water-in-water emulsions upon solvent shifting. 1st method (left): ethanol is poured in the emulsion containing proteins or particles, 2nd method (right): A Dex-rich dispersion is poured into aqueous PEG that also contain ethanol, proteins or particles. In both cases, stable droplets that no longer coalesce form.
2 Results and discussion
2.1 Solvent shifting process
We start with an ATPS made of dextran (Dex, MW: 500 kDa, 32.5 mg/mL)-enriched droplets in a polyethylene glycol (PEG, 20 kDa, 70 mg/mL)-rich continuous phase. After vigorous mixing, the slightly turbid dispersion had a pH of about 6.5 and contained droplets (∼10–50 µm) that coalesced upon resting, yielding macroscopic phase separation (Figure 1A). When diluted by a factor of 4 with pure water, this yielded limpid solutions (demulsification control experiment, DCE), the concentration of both Dex and PEG being too low to form an aqueous emulsion (not shown). Addition of equal volume of ethanol to the initial ATPS yielded a pellet composed of gelled dextran floating in aqueous PEG (Figure 1B). This feature has already been observed in pure solutions of Dex and is used for fractionating this polymer in protein free dispersions (Neuchl and Mersmann, 1995), ethanol acting as a bad solvent (Antoniou et al., 2010). We expect that the hydroxyl group of ethanol makes hydrogen bonds with the hydroxyl groups of this polysaccharide, so that the ethylene moiety of the bonded ethanol molecules yields hydrophobic interactions that condense the polymer chains to form a gel.
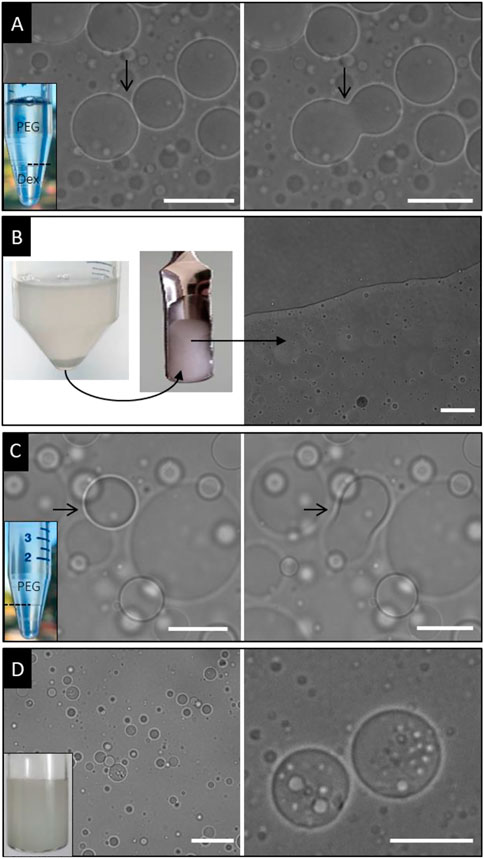
FIGURE 1. Addition of ethanol to ATPS (PEG/Dex). Panel (A) solvent free system showing macroscopic phase separation (left) of samples due to coalescence of droplets as observed by bright field microscopy (the arrow points at a coalescence event). Scale bar is for 50 µm. Panel (B) sample tube photo (left) and spatula (middle) showing formation of gel Dex-rich pellet upon solvent shifting onto ATPS. The sample is also observed by bright field microscopy showing no droplet (right). Scale bar is for 20 µm. Panel (C) sample tube photo (left) after macroscopic phase separation and solvent shifting onto ATPS-containing BSA using only half amount of ethanol (the arrow points at a coalescence event). Scale bar is for 20 µm. Panel (D) sample tube photo (left) after solvent shifting using the same volume of ethanol as that of ATPS (-containing BSA). The sample is constituted by small stable droplets that can entrap other droplets (the scale bar is for 20 µm).
To the stock solution of ATPS, Bovine Serum Albumin (BSA: 100 mg/mL, 1 mL/5 mL ATPS) was added and we checked that the protein was fully dispersed in this ATPS. Here, the final concentration of BSA is lower than 2 wt%, a value too low to produce a Dex/BSA biphasic mixture (Antonov et al., 2015) and eventually to form a PEG/Dex/BSA aqueous three-phase system. Moreover, the pH of the solution was about 6.5, higher than the isoeletric point of the protein, which is then still charged, allowing its full dispersion in the ATPS. This is further demonstrated when using BSA-FITC to study the partitioning of the protein in Dex and PEG phases (see below). Then, the solvent shifting process was applied using various volumes of ethanol. For low amounts of that solvent (2.5 mL ethanol for 5 mL ATPS), this yielded turbid, milky dispersions composed of droplets that still coalesced with time, still yielding macroscopic phase separation after few hours (Figure 1C).
By contrast, for an equal volume of ATPS and ethanol, stable droplets that no longer coalesced were observed (Figure 1D). They exhibited a size much lower than in the absence of solvent, being lower than 20 µm. No pellet of gelled dextran formed at the bottom of the sample tube as in the case of BSA-free samples. Clearly, BSA allowed stabilization of droplets upon solvent shifting with ethanol. More precisely, double all-aqueous emulsions formed upon addition of ethanol. Small bright patches were observed within Dex-rich droplets (Figure 1D), indicating that small PEG droplets were entrapped. Again, we further checked (see below) using BSA-FITC that these internal droplets are not BSA-rich droplets, eventually forming an aqueous three phase system in the presence of the solvent. The capture of internal PEG-rich droplets probably occurs upon mixing, because of shearing forces and gelation of dextran that could retain small PEG droplets. A similar feature has been observed in capsules produced by other ways, but because of osmosis induced-diffusion of water (Song et al., 2015; Hann et al., 2017b). We believe that the core of droplets is probably gelled as this occurs for pellets in the absence of proteins but we did not investigate this aspect. Obviously, these capsules are not made of a gelled droplet of Dex that would be surrounded by a BSA shell, but rather, the protein is homogeneously embedded within the Dex gelified droplet and allows for a more compact structure that further prevent coalescence of droplets.
Using some fluorescently labelled dextran (Dex-FITC) allowed checking that the core of droplets was indeed composed of this polymer (Figure 2A). Again, it also clearly revealed the presence of entrapped PEG droplets since patches lacking fluorescence were observed within main droplets. We also used fluorescently labelled BSA (BSA-FITC) that was first shown to partition slightly preferentially into the PEG phase since that phase was more colored. This was evidenced after macroscopic phase separation by visual inspection but also by epifluorescence microscopy showing higher fluorescence intensity in the continuous PEG-rich outer phase, than in droplets (Figure 2B). BSA (FITC-free) was also initially added in samples allowing confirming that no aqueous three phase systems formed as commented above. Then, solvent shifting was applied to these samples also containing BSA-FITC. Clearly, fluorescence was mainly observed within droplets showing that BSA was entrapped within them. In other words, the proteins not only lie at the droplet interface as could be expected to form a shell around Dex-rich droplets, but are fully mixed with the polysaccharide to prevent its aggregation upon addition of ethanol. This also allows confirming that the entrapped aqueous droplets lacking fluorescence are not protein-rich droplets that would have formed in the case of an aqueous three phase system occurring in the presence of solvent as commented above.
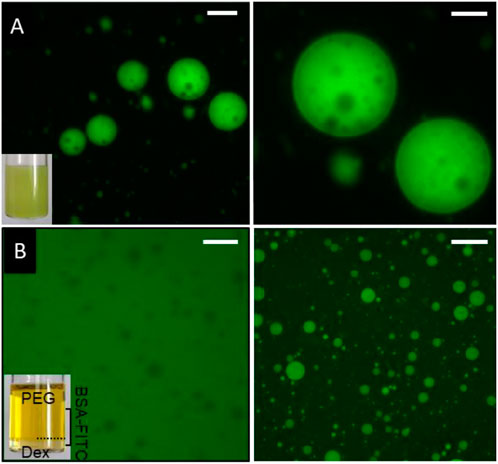
FIGURE 2. Panel (A) solvent shifting applied to ATPS-containing Dex- FITC. Sample tube photo (left) shows homogeneous stable emulsion and epifluorescence microscopy (left: scale bar is for 20 µm and right for 10 µm) allows visualizing FITC labelled Dex only, confirming the presence of small PEG droplets lacking fluorescence within main droplets. Panel (B) solvent shifting applied to ATPS-containing BSA-FITC. Sample tube photo (left) after macroscopic phase separation in the solvent-free sample allowing visualizing the presence of protein preferentially in the PEG phase (not quantitatively). This is confirmed by epifluorescence microscopy showing higher fluorescence intensity out of droplets (left, black patches, scale bar is for 50 µm). Epifluorescence image of a sample after solvent shifting (right) showing that fluorescent proteins were fully incorporated within stable Dex-rich droplets (the scale bar is for 50 µm).
Instead of BSA, we also used commercially available positively charged silica nanoparticles (SiNH2, <50 nm dispersed in water). These NP (100 µL) were added in the ATPS (1 mL) and were shown to be mainly present in the PEG-rich phase by epifluorescence after labelling with FITC, and dark field microscopy (Figure 3A). In the first case, high fluorescence was observed out of Dex-rich droplets while white small patches were clearly visible out of droplets in the second case. This contrasts with positively charged latex beads but also with polydopamine-based particles that were observed to lie at the droplet interface in similar ATPS (Douliez et al., 2018a; Zhang et al., 2019).
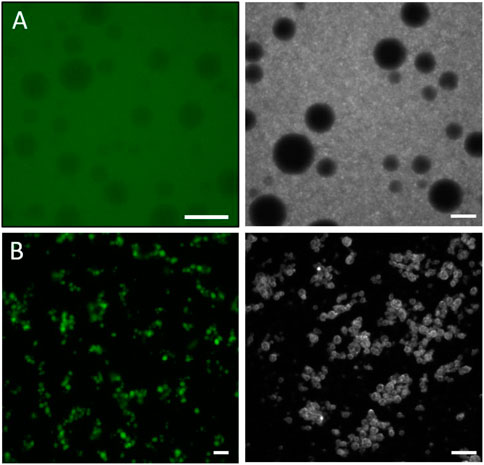
FIGURE 3. Panel (A) spatial localization of amino-silica based particles within solvent-free ATPS showing high fluorescence (left epifluorescence microscopy) mainly out of Dex-rich droplets, i.e., in the outer PEG-rich continuous phase and white patches (right, dark field microscopy). Panel (B) stabilization of ATPS upon solvent shifting using amino-silica based particles, showing smaller droplets than in the solvent-free ATPS. The inner core of droplets being fluorescent (epifluorescence image, left) attests that particles are localized not only at the interface but also within Dex-rich droplets. These stable droplets can also be observed by dark field microscopy (right). (Scale bars are for 20 µm).
Then, ethanol was added affording stable droplets of much smaller diameter (than in the absence of ethanol) that no longer coalesced with time (Figure 3B). They were however slightly aggregated. Again, no pellet was observed at the bottom of the sample tube. The use of fluorescently labelled particles allowed observing fluorescence within droplets, as in the case of samples containing BSA-FITC. Then, in the same way as before, droplets are stabilized not only because of the presence of particles at the interface but also because they are embedded within the Dex gelified droplets, reinforcing the structure and preventing coalescence of droplets.
2.2 Alternative method for producing droplets upon solvent shifting
We then varied the protocol to produce such droplets. We took advantage that as classical oil and water emulsions, for which one phase is poured in the other one upon mixing, water-in-water emulsions can also be prepared in this way. Then, instead of adding ethanol in the ATPS, ethanol was mixed with a slightly more concentrated aqueous solution of PEG (PEG 20 kDa, 8%, 4.0 mL/5 mL ethanol). Then, a concentrated solution of dextran (500 kDa, 21%, 1 mL) was added under vigorous stirring (see Scheme 1). In the absence of BSA, this yielded gelled aggregated pellets of dextran as observed using ATPS in the experiments described above (not shown). In the presence of BSA (100 mg/mL, 1 mL in PEG (4.0 mL)-Ethanol (5 mL), addition of Dex induced turbid dispersions as in the case of previous experiments starting with an ATPS and addition of ethanol. The experimental conditions (final concentrations of polymers and BSA) were slightly different than those used above; however, stable dextran-rich droplets (that did not coalesce) also formed using this protocol (Figure 4A). We also investigated the case for which BSA was co-added with Dex, instead of mixing proteins with PEG/ethanol. For this, we also employed BSA-FITC allowing to confirm that proteins were entrapped within Dex-rich droplets (Figure 4B). When BSA (FITC-free) was initially mixed with PEG and ethanol, still using some BSA-FITC co-added with dextran, fluorescent proteins were again observed mainly within Dex-rich stabilized droplets (Figure 4C). In contrast, when BSA-FITC was also mixed initially with BSA (FITC-free) in PEG/ethanol, fluorescence was indeed observed within droplets but also clearly at the droplet interface (Figure 4D).
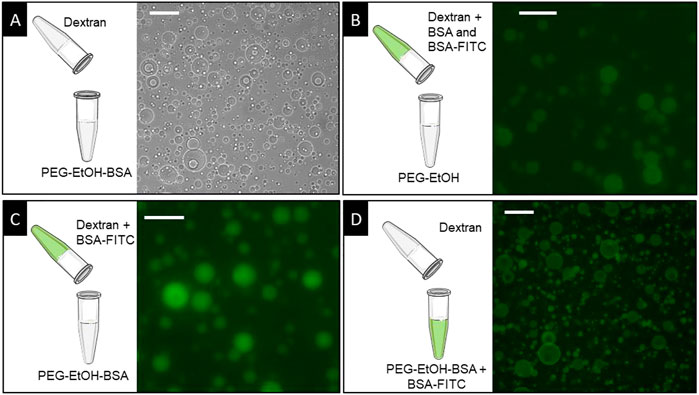
FIGURE 4. Schemes and microscopy images showing the formation of stable droplets using the second method (see main text). (A) Stable droplets observed using bright field microscopy after pouring Dex-rich solution within a dispersion of PEG-ethanol-BSA. (B–D) Stable droplets observed using BSA-FITC via epifluorescence microscopy, by co-adding BSA-FITC and BSA (FITC-free) in Dex (B) or in PEG-Ethanol (D), or co-adding BSA-FITC in Dex while BSA (FITC-free) was co-added in PEG/ethanol. Schemes allow describing the localization of BSA-FITC in each case. The scale bars stand for 50 µm.
Similar experiments were performed when adding silica particles instead of BSA. Particles were added with PEG-EtOH and the Dex-rich phase was further incorporated. This also yielded stable droplets as in the case described above when solvent shifting was applied on the ATPS that contained particles (not shown).
2.3 Stability of capsules
Immediate dilution with pure water (diluted by a factor of 4, dilution control experiment, DCE) of such samples forming stable droplets upon solvent shifting yielded limpid solutions (as in the case of stock ATPS solution), showing that no robust capsules formed immediately (not shown). As commented above and elsewhere (Perro et al., 2022), chemicals or particles present at the droplet interface need to be cross-linked to form robust capsules that could further survive dilution. Robust capsules were indeed formed in the present work using carbodiimide chemistry and upon mineralization by adding tetraethoxysilane (TEOS). Although TEOS is not soluble in pure water, it was advantageously well dispersed in samples under these conditions because of the presence of ethanol. Here, the proteins or even particles serve as a scaffold for the condensation of TEOS as has been observed in other similar cases (Erni et al., 2013). In both cases, the use of Dex-FITC allowed visualizing fluorescent droplets after dilution of samples, attesting that the fluorescent polymer remained entrapped within these droplets that survive variation of the osmotic pressure (Figure 5).
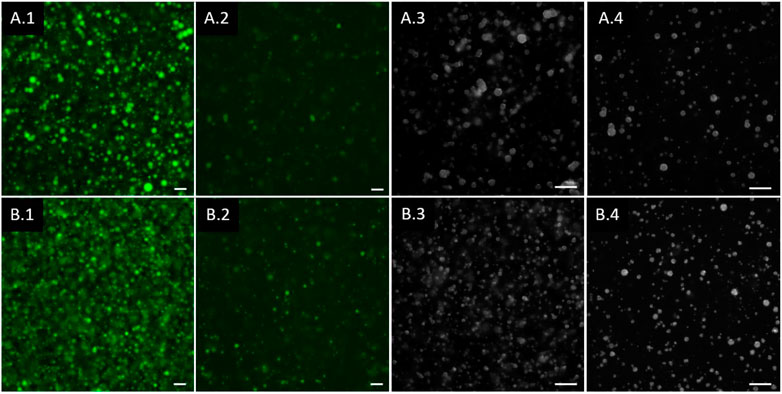
FIGURE 5. Epifluorescence (A.1, A.2 and B.1, B.2) and dark field (A.3, A.4 and B.3, B.4) images using Dex-FITC within samples, in stable droplets prepared after solvent shifting (A.1, A.3 and B.1, B.3) and after dilution (A.2, A.4 and B.2, B.4), when mixed with TEOS (A.1–4) and 1-Ethyl-3-carbodiimide (EdC) (B.1–4). (Scale bars are for 20 µm).
3 Conclusion
In summary, within the framework of strong efforts aimed at developing methods for stabilizing ATPS and producing capsules from such systems (Douliez, 2023), we report the formation of stable droplets and capsules using ATPS upon solvent shifting. These are prepared in bulk, without solid templates or oil, but from all-in-water emulsions. The template is an aqueous polymer-rich droplet that requires stabilization with proteins or particles when solvent is added to form droplets that no longer coalesce with time. The solvent shifting process can be applied on the pre-prepared ATPS but also alternatively, by mixing one polymer-rich phase with the solvent and further adding the second polymer-rich dispersion. We also expect that it could be applied in the case of other ATPS or even coacervates that are made of counter-charged polymers and/or polyelectrolytes. Unexpectedly, here hollow capsules do not form but rather, gelified droplets made of mixed proteins or particles and dextran. However, the use of other ATPS and/or proteins, particles or other chemicals used for the stabilization of such aqueous droplets could allow formation of hollow capsules upon solvent shifting. Our capsules allow retaining entrapped fluorescent dextran and then, we believe that this new method for generating capsules should find applications in domains that concern microencapsulation.
4 Materials and methods
All chemicals were from Sigma-Aldrich, except dextran from Pharmacia.
The ATPS was prepared as follows: 80 mL PEG/dextran stock mixture (70 mg/mL PEG, 32.5 mg/mL dextran) was prepared by dissolving 5.6 g of PEG and 2.6 g of dextran in 80 mL of Milli-Q water in a glass container, and mixed under magnetic stirring for 24 h at room temperature (range: 17°C–24°C) to ensure complete dissolution of the polymers. This stock solution was aliquoted in 5 mL tubes and stored at 4°C. Proteins were dispersed in water and particles were used as well (they are already dispersed in water) at concentrations described in the main text. To the stock solution of ATPS, Bovine Serum Albumin (BSA: 100 mg/mL, 1 mL/5 mL ATPS) was added, and then, the solvent shifting process was applied using various volumes of ethanol. We also used positively charged silica nanoparticles (Sigma-Aldrich, ref: 791342 SiNH2, <50 nm dispersed in water). They were added at a concentration of 100 µL (particles) for 1 mL ATPS.
Aqueous stock solutions of BSA-FITC and dextran-FITC were prepared at a concentration of 1 mg/mL and 5 mg/mL, respectively. Then, 200 µL of one or the other were added to 5 mL ATPS.
For the alternative method, samples were prepared as follows: more concentrated aqueous solution of PEG (PEG 20 kDa, 8%, and dextran (500 kDa, 21%) were prepared separately. 4 mL of the PEG solution were mixed with 5 mL ethanol and BSA (100 mg/mL, 1 mL). Then, 1 mL of the Dex solution was added under vigorous stirring.
The formation of robust capsules was done at room temperature as follows: either 1-Ethyl-3-carbodiimide (EdC) or tetraethoxysilane (TEOS) were used. EdC was prepared in water at a concentration of 200 mg/mL. In typical experiments, either 200 µL EdC or 1 mL TEOS were added in samples after solvent shifting (5 mL ATPS, 1 mL BSA or 500 µL particles, 5 mL ethanol) under vigorous shaking and allowed to react for 3 h under mild agitation.
Samples were observed either by dark field or epifluorescence microscopies: in the first case, an Eclipse Ni (Nikon) microscope working in reflection and equipped with a dark field condenser was used. The Nikon oil immersion microscope objective was ×40 with a numerical aperture (N. A.) of 0.80. Images were acquired with an Iris 9™ Scientific CMOS camera (2,960 × 2,960 pixels) and further treated with ImageJ. Epifluorescence imaging was performed on a Leica DMI 4000B inverted microscope equipped with a ×40 lens (HCX PL APO, 1.4 NA) and a CoolLED light source combined with appropriate filter cubes to select the excited and emitted fluorescence wavelength range. Images were acquired using MicroManager and processed with ImageJ.
Data availability statement
The original contributions presented in the study are included in the article/supplementary material, further inquiries can be directed to the corresponding author.
Author contributions
All authors listed have made a substantial, direct, and intellectual contribution to the work and approved it for publication.
Conflict of interest
The authors declare that the research was conducted in the absence of any commercial or financial relationships that could be construed as a potential conflict of interest.
Publisher’s note
All claims expressed in this article are solely those of the authors and do not necessarily represent those of their affiliated organizations, or those of the publisher, the editors, and the reviewers. Any product that may be evaluated in this article, or claim that may be made by its manufacturer, is not guaranteed or endorsed by the publisher.
References
Abbas, M., Lipiski, W. P., Wang, J., and Spruijt, E. (2021). Peptide-based coacervates as biomimetic protocells. Chem. Soc. Rev. 50, 3690–3705. doi:10.1039/d0cs00307g
Antoniou, E., Themistou, E., Sarkar, B., Tsianou, M., and Alexandridis, P. (2010). Structure and dynamics of dextran in binary mixtures of a good and a bad solvent. Colloid Polym. Sci. 288, 1301–1312. doi:10.1007/s00396-010-2259-x
Antonov, Y. A., Wolf, B. A., and Moldenaers, P. (2015). Inducing mixing of water in water BSA/Dextran emulsion by a strong polyelectrolyte. Food Hydrocoll. 43, 243–251. doi:10.1016/j.foodhyd.2014.05.029
Aubry, J., Ganachaud, F., Cohen Addad, J.-P., and Cabane, B. (2009). Nanoprecipitation of polymethylmethacrylate by solvent shifting:1. Boundaries. Langmuir 25, 1970–1979. doi:10.1021/la803000e
Bakry, A. M., Abbas, S., Ali, B., Majeed, H., Abouelwafa, M. Y., Mousa, A., et al. (2015). Microencapsulation of oils: A comprehensive review of benefits, techniques, and applications. Compr. Rev. Food Sci. Food Saf. 15, 143–182. doi:10.1111/1541-4337.12179
Botet, R. (2011). The "ouzo effect", recent developments and application to therapeutic drug carrying. J. Phys. Conf. Ser. 352, 012047. doi:10.1088/1742-6596/352/1/012047
Buddingh, B. C., and van Hest, J. C. M. (2017). Artificial cells: Synthetic compartments with life-like functionality and adaptivity. Accounts Chem. Res. 50, 769–777. doi:10.1021/acs.accounts.6b00512
Caruso, F. (2000). Hollow capsule processing through colloidal templating and self-assembly. Chem. – A Eur. J. 6, 413–419. doi:10.1002/(sici)1521-3765(20000204)6:3<413::aid-chem413>3.0.co;2-9
Chao, Y., and Shum, H. C. (2020). Emerging aqueous two-phase systems: From fundamentals of interfaces to biomedical applications. Chem. Soc. Rev. 49, 114–142. doi:10.1039/c9cs00466a
Cheung Shum, H., Varnell, J., and Weitz, D. A. (2012). Microfluidic fabrication of water-in-water (w/w) jets and emulsions. Biomicrofluidics 6, 012808. doi:10.1063/1.3670365
Coudon, N., Navailles, L., Nallet, F., Ly, I., Bentaleb, A., Chapel, J.-P., et al. (2022). Stabilization of all-aqueous droplets by interfacial self-assembly of fatty acids bilayers. J. Coll. Int. Sci. 617, 257–266. doi:10.1016/j.jcis.2022.02.138
Dai, Z., Dahne, L., Mohwald, H., and Tiersch, B. (2002). Novel capsules with high stability and controlled permeability by hierarchic templating. Angew. Chem. Int. Ed. Engl. 114, 4019–4022. doi:10.1002/1521-3773(20021104)41:21<4019::aid-anie4019>3.0.co;2-z
de Freitas, R. A., Nicolai, T., Chassenieux, C., and Benyahia, L. (2016). Stabilization of water-in-water emulsions by polysaccharide-coated protein particles. Langmuir 32, 1227–1232. doi:10.1021/acs.langmuir.5b03761
Deng, Y., Ma, Q., Yuan, H., Lum, G. C., and Shum, H. C. (2019). Development of dual-component protein microparticles in all-aqueous systems for biomedical applications. J. Mater. Chem. B 7, 3059–3065. doi:10.1039/c8tb03074j
Dewey, D. C., Strulson, C. A., Cacace, D. N., Bevilacqua, P. C., and Keating, C. D. (2014). Bioreactor droplets from liposome-stabilized all-aqueous emulsions. Nat. Commun. 5, 4670. doi:10.1038/ncomms5670
Dinsmore, A. D., Hsu, M. F., Nikolaides, M. G., Marquez, M., Bausch, A. R., and Weitz, D. A. (2002). Colloidosomes: Selectively permeable capsules composed of colloidal particles. Science 298, 1006–1009. doi:10.1126/science.1074868
Discher, D. E., and Eisenberg, A. (2002). Polymer vesicles. Science 297, 967–973. doi:10.1126/science.1074972
Donath, E., Sukhorukov, G., Caruso, B. F., Davis, S., and Möhwald, A. H. (1998). Novel hollow polymer shells by colloid-templated assembly of polyelectrolytes. Angew. Chem. Int. Ed. Engl. 37, 2201–2205. doi:10.1002/(sici)1521-3773(19980904)37:16<2201::aid-anie2201>3.0.co;2-e
Dora Tang, T. Y., Rohaida Che Hak, C., Thompson, A. J., Kuimova, M. K., Williams, D. S., Perriman, A. W., et al. (2014). Fatty acid membrane assembly on coacervate microdroplets as a step towards a hybrid protocell model. Nat. Chem. 6, 527–533. doi:10.1038/nchem.1921
Douliez, J.-P., Martin, N., Beneyton, T., Eloi, J.-C., Chapel, J.-P., Navailles, L., et al. (2018). Preparation of swellable hydrogel-containing colloidosomes from aqueous two-phase pickering emulsion droplets. Angew. Chem. Int. Ed. Engl. 57, 7906–7910. doi:10.1002/ange.201802929
Douliez, J.-P., Martin, N., Gaillard, C., Beneyton, T., Baret, J.-C., Mann, S., et al. (2017). Catanionic coacervate droplets as a surfactant-based membrane-free protocell model. Angew. Chem. Int. Ed. 56, 13877–13881. doi:10.1002/ange.201707139
Douliez, J.-P., Perro, A., and Béven, l. (2019). Stabilization of all-in-water emulsions to form capsules as artificial cells. Chem. Bio. Chem. 20, 2546–2552. doi:10.1002/cbic.201900196
Douliez, J.-P., Perro, A., Chapel, J.-P., Goudeau, B., and Béven, L. (2018). Preparation of template-free robust yolk-shell gelled particles from controllably evolved all-in-water emulsions. All-in-Water Emuls. 14, 1803042. doi:10.1002/smll.201803042
Douliez, J. P. (2023). Stabilizing an all-aqueous two-phase system using oil-in-water droplets to mimic double emulsions. ChemRXiv 2023. doi:10.26434/chemrxiv-2023-fcgcx
Duan, G., Haase, M. F., Stebe, K. J., and Lee, D. (2017). One-Step generation of salt-responsive polyelectrolyte microcapsules via surfactant-organized nanoscale interfacial complexation in emulsions (SO NICE). Langmuir 34, 847–853. doi:10.1021/acs.langmuir.7b01526
Dzieciol, A. J., and Mann, S. (2012). Designs for life: Protocell models in the laboratory. Chem. Soc. Rev. 41, 79–85. doi:10.1039/c1cs15211d
Erni, P., Dardelle, G., Sillick, M., Wong, K., Beaussoubre, P., and Fieber, W. (2013). Turning coacervates into biohybrid glass: Core/shell capsules formed by silica precipitation in protein/polysaccharide scaffolds. Angew. Chem. Int. Ed. Engl. 52, 10334–10338. doi:10.1002/anie.201303489
Esquena, J. (2016). Water-in-water (W/W) emulsions. Curr. Op. Coll. Inter. Sci. 25, 109–119. doi:10.1016/j.cocis.2016.09.010
Ganachaud, F., and Katz, J. L. (2005). Nanoparticles and nanocapsules created using the ouzo effect: Spontaneous emulsification as an alternative to ultrasonic and high-shear devices. ChemPhysChem 6, 209–216. doi:10.1002/cphc.200400527
Garenne, D., Navailles, L., Nallet, F., Grélard, A., Dufourc, E. J., and Douliez, J.-P. (2016). Clouding in fatty acid dispersions for charge-dependent dye extraction. J. Coll. Int. Sci. 468, 95–102. doi:10.1016/j.jcis.2016.01.049
Gonzalez-Jordan, A., Nicolai, T., and Benyahia, L. (2016). Influence of the protein particle morphology and partitioning on the behavior of particle-stabilized water-in-water emulsions. Langmuir 32, 7189–7197. doi:10.1021/acs.langmuir.6b01993
Goubault, C., Sciortino, F., Mongin, O., Jarry, U., Boston, M., Jakobczyk, H., et al. (2020). The ouzo effect: A tool to elaborate high-payload nanocapsules. J. Control. Release 324, 430–439. doi:10.1016/j.jconrel.2020.05.023
Hann, S. D., Lee, D., and Stebe, K. J. (2017). Tuning interfacial complexation in aqueous two phase systems with polyelectrolytes and nanoparticles for compound all water emulsion bodies (AWE-somes). Phys. Chem. Chem. Phys. 19, 23825–23831. doi:10.1039/c7cp02809a
Hann, S. D., Stebe, K. J., and Lee, D. (2017). AWE-somes: All water emulsion bodies with permeable shells and selective compartments. ACS Appl. Mater. Interfaces 9, 25023–25028. doi:10.1021/acsami.7b05800
Huang, X., Li, M., Green, D. C., Williams, D. S., Patil, A. J., and Mann, S. (2013). Interfacial assembly of protein-polymer nano-conjugates into stimulus-responsive biomimetic protocells. Nat. Commun. 4, 2239. doi:10.1038/ncomms3239
Keating, C. D. (2012). Aqueous phase separation as a possible route to compartmentalization of biological molecules. Accounts Chem. Res. 45, 2114–2124. doi:10.1021/ar200294y
Kim, M., Doh, J., and Lee, D. (2016). pH-induced softening of polyelectrolyte microcapsules without apparent swelling. ACS Macro Lett. 5, 487–492. doi:10.1021/acsmacrolett.6b00124
Kim, M., Yeo, S. J., Highley, C. B., Burdick, J. A., Yoo, P. J., Doh, J., et al. (2015). One-Step generation of multifunctional polyelectrolyte microcapsules via nanoscale interfacial complexation in emulsion (NICE). ACS Nano 9, 8269–8278. doi:10.1021/acsnano.5b02702
Koga, S., Williams, D. S., Perriman, A. W., and Mann, S. (2011). Peptide-nucleotide microdroplets as a step towards a membrane-free protocell model. Nat. Chem. 3, 720–724. doi:10.1038/nchem.1110
Lepeltier, E., Bourgaux, C., and Couvreur, P. (2013). Nanoprecipitation and the Ouzo effect: Application to drug delivery devices. Adv. Drug Deliv. Rev. 71, 86–97. doi:10.1016/j.addr.2013.12.009
Li, Y., and Shi, J. (2014). Hollow-structured mesoporous materials: Chemical synthesis, functionalization and applications. Adv. Mater. 26, 3176–3205. doi:10.1002/adma.201305319
Liu, J., Qiao, S. Z., Budi Hartono, S., and Lu, G. Q. (2010). Monodisperse yolk-shell nanoparticles with a hierarchical porous structure for delivery vehicles and nanoreactors. Angew. Chem. Int. Ed. Engl. 49, 4981–4985. doi:10.1002/anie.201001252
Ma, Q., Song, Y., Kim, J. W., Choi, H. S., and Shum, H. C. (2016). Affinity partitioning-induced self-assembly in aqueous two-phase systems: Templating for polyelectrolyte microcapsules. ACS Macro Lett. 5, 666–670. doi:10.1021/acsmacrolett.6b00228
Ma, Q., Yuan, H., Song, Y., Chao, Y., Mak, S. Y., and Shum, H. C. (2018). Partitioning-dependent conversion of polyelectrolyte assemblies in an aqueous two-phase system. Soft Matter 14, 1552–1558. doi:10.1039/c7sm02275a
Mason, A. F., Buddingh, B. C., Williams, D. S., and van Hest, J. C. M. (2017). Hierarchical self-assembly of a copolymer-stabilized coacervate protocell. J. Am. Chem. Soc. 139, 17309–17312. doi:10.1021/jacs.7b10846
Neuchl, C., and Mersmann, A. (1995). Fractionation of polydisperse dextran using ethanol. Chem. Eng. Sci. 50, 951–958. doi:10.1016/0009-2509(94)00492-a
Nguyen, B. T., Nicolai, T., and Benyahia, L. (2013). Stabilization of water-in-water emulsions by addition of protein particles. Langmuir 29, 10658–10664. doi:10.1021/la402131e
Peddireddy, K. R., Nicolai, T., Benyahia, L., and Capron, I. (2016). Stabilization of water-in-water emulsions by nanorods. ACS Macro Lett. 5, 283–286. doi:10.1021/acsmacrolett.5b00953
Perro, A., Coudon, N., Chapel, J.-P., Martin, N., Béven, L., and Douliez, J.-P. (2022). Building micro-capsules using water-in-water emulsion droplets as templates. J. Coll. Int. Sci. 613, 681–696. doi:10.1016/j.jcis.2022.01.047
Sciortino, F., Cuny, J., Grasset, F., Lagrost, C., Lemoine, P., Moriac, A., et al. (2018). The Ouzo effect to selectively assemble molybdenum clusters into nanomarbles or nanocapsules with increased HER activity. Chem. Comm. 54, 13387–13390. doi:10.1039/c8cc07402j
Sciortino, F., Thivolle, M., Kahn, M., Gaillard, C., Soizic, C., and Gauffre, F. (2017). Structure and elasticity of composite nanoparticle/polymer nanoshells (hybridosomes®). Soft Matter 13, 4393–4400. doi:10.1039/c7sm00705a
Song, Y., Chan, Y. K., Ma, Q., Liu, Z., and Shum, H. C. (2015). All-aqueous electrosprayed emulsion for templated fabrication of cytocompatible microcapsules. ACS Appl. Mater. Interfaces 7, 13925–13933. doi:10.1021/acsami.5b02708
Song, Y., Sauret, A., and Cheung Shum, H. (2013). All-aqueous multiphase microfluidics. Biomicrofluidics 7, 061301. doi:10.1063/1.4827916
Song, Y., Shimanovich, U., Michaels, T. C. T., Ma, Q., Li, J., Knowles, T. P. J., et al. (2016). Fabrication of fibrillosomes from droplets stabilized by protein nanofibrils at all-aqueous interfaces. Nat. Commun. 7, 12934. doi:10.1038/ncomms12934
Tea, L., Nicolai, T., and Renou, F. (2019). Stabilization of water-in-water emulsions by linear homo-polyelectrolytes. Langmuir 35, 9029–9036. doi:10.1021/acs.langmuir.9b01604
Thompson, K. L., Williams, M., and Armes, S. P. (2015). Colloidosomes: Synthesis, properties and applications. J. Coll. Int. Sci. 447, 217–228. doi:10.1016/j.jcis.2014.11.058
Wang, M., and Wang, Y. (2014). Development of surfactant coacervation in aqueous solution. Soft Matter 10, 7909–7919. doi:10.1039/c4sm01386g
Williams David, S., Patil Avinash, J., and Mann, S. (2014). Spontaneous structuration in coacervate-based protocells by polyoxometalate-mediated membrane assembly. Small 10, 1830–1840. doi:10.1002/smll.201303654
Windbergs, M., Zhao, Y., Heyman, J., and Weitz, D. A. (2013). Biodegradable core-shell carriers for simultaneous encapsulation of synergistic actives. J. Am. Chem. Soc. 135, 7933–7937. doi:10.1021/ja401422r
Wu, Y., Liao, I. C., Kennedy, S. J., Du, J., Wang, J., Leong, K. W., et al. (2010). Electrosprayed core-shell microspheres for protein delivery. Chem. Comm. 46, 4743–4745. doi:10.1039/c0cc00535e
Zhang, J., Hwang, J., Antonietti, M., and Schmidt, B. V. K. J. (2019). Water-in-Water pickering emulsion stabilized by polydopamine particles and cross-linking. Biomacromolecules 20, 204–211. doi:10.1021/acs.biomac.8b01301
Zhang, L., Cai, L.-H., Lienemann, P., Rossow, S, T., Polenz, I., Vallmajo-Martin, Q., et al. (2016). One-Step microfluidic fabrication of polyelectrolyte microcapsules in aqueous conditions for protein release. Angew. Chem. Int. Ed. Engl. 55, 13668–13672. doi:10.1002/ange.201606960
Keywords: water-in-water emulsion, solvent shifting, capsules, aqueous two phase system, encapsulation
Citation: Céré C, Béven L and Douliez J-P (2023) Stabilization of water-in-water emulsion upon solvent shifting. Front. Soft. Matter 3:1158285. doi: 10.3389/frsfm.2023.1158285
Received: 03 February 2023; Accepted: 08 March 2023;
Published: 17 March 2023.
Edited by:
Mohd Sajid Ali, King Saud University, Saudi ArabiaReviewed by:
Jordi Esquena, Spanish National Research Council (CSIC), SpainBen Erné, Utrecht University, Netherlands
Copyright © 2023 Céré, Béven and Douliez. This is an open-access article distributed under the terms of the Creative Commons Attribution License (CC BY). The use, distribution or reproduction in other forums is permitted, provided the original author(s) and the copyright owner(s) are credited and that the original publication in this journal is cited, in accordance with accepted academic practice. No use, distribution or reproduction is permitted which does not comply with these terms.
*Correspondence: Jean-Paul Douliez, amVhbi1wYXVsLmRvdWxpZXpAaW5yYWUuZnI=