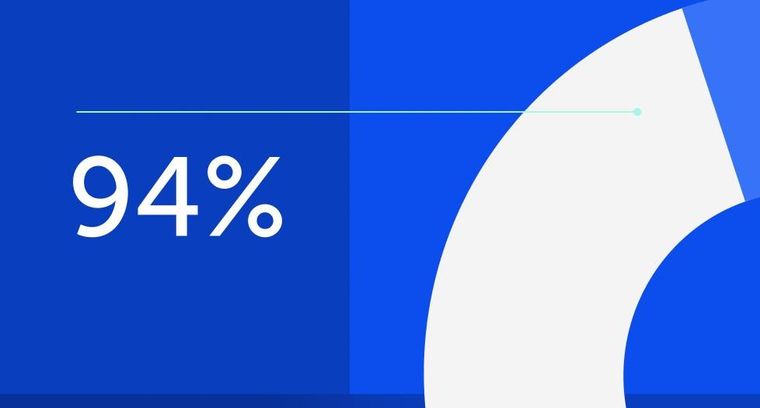
94% of researchers rate our articles as excellent or good
Learn more about the work of our research integrity team to safeguard the quality of each article we publish.
Find out more
ORIGINAL RESEARCH article
Front. Soft Matter, 10 March 2023
Sec. Colloids and Emulsions
Volume 3 - 2023 | https://doi.org/10.3389/frsfm.2023.1093168
This article is part of the Research TopicLiquid foams and emulsions stabilized by bio-based particlesView all 6 articles
Pea protein isolate (Pisum sativum L., PPI) has been much studied in the last decade because of its potential as a bio-based alternative for surfactants to produce innovative and environmentally friendly emulsion products. PPI is ideal due to its favorable nutritional properties, low allergenicity and low environmental impact. Despite its growing popularity, understanding the stabilisation mechanism of emulsions stabilized with PPI remains a key question that requires further investigation. Here, we use fluorescence lifetime microscopy with molecular rotors as local probes for interfacial viscosity of PPI stabilized emulsions. The fluorescence lifetime correlates to the local viscosity at the oil-water interface allowing us to probe the proteins at the interfacial region. We find that the measured interfacial viscosity is strongly pH-dependent, an observation that can be directly related to PPI aggregation and PPI reconformation. By means of molecular rotor measurements we can link the local viscosity of the PPI particles at the interface to the Pickering-like stabilisation mechanism. Finally, this can be compared to the local viscosity of PPI solutions at different pH conditions, showing the importance of the PPI treatment prior to emulsification.
Emulsions are mixtures of two or more liquid immiscible phases, one dispersed in the other as droplets. Due to high interfacial tensions and droplet sizes greater than 0.1 µm, these systems are typically thermodynamically unstable Shahidzadeh et al. (1999); Aswathanarayan and Vittal (2019). Despite the existence of thermodynamically stable emulsions (e.g., microemulsions) or systems that display spontaneous emulsification, standard emulsion preparation includes other surface-active ingredients to guarantee kinetic stability, such as classic surfactants, proteins and other bio-based particles Shahidzadeh et al. (1997); McClements (2004); Yan et al. (2020). The use of proteins to stabilize emulsions has been extensively studied in recent decades, especially in the case of dairy proteins such as casein and whey Zhang et al. (2021); Hinderink et al. (2020); Burger and Zhang (2019); Lee et al. (2009); Tcholakova et al. (2006). Lately, the food industry has developed a great interest in the development of products with plant-based proteins. Indeed, the global context has shown a need for a shift in dietary habits from synthetic or animal-based products to other alternatives Poore and Nemecek (2018). Thus, complex systems such as emulsions stabilized by soy or lentil proteins have become alternatives for surfactants for environmentally responsible product development Tang (2017); Liu and Tang (2013); Can Karaca et al. (2011).
Pea is one of the plant-based proteins that has attracted a lot of attention recently. Due to its nutritional and emulsifying properties as well as low allergenicity, research on PPI-stabilized emulsions has grown Kornet et al. (2022c); Li et al. (2022); Sridharan et al. (2020b); Lu et al. (2020); Lam et al. (2018). Pea protein isolates (PPI) are mainly composed by two globular proteins: Globulin and Albumin in a minor percentage. The former can be also separated into Legumin and Vicilin. In addition, there is also a minority presence of carbohydrates, lipids and moisture Burger and Zhang (2019). The relative amounts of these components can vary and how these different components interact with water-oil interfaces depending to the environment is responsible for the great complexity of the behavior of these proteins.
PPI as an emulsion stabilizer has been studied from different points of view. For instance, many parameters such as the globulin/albumin ratio, protein solution pH Kornet et al. (2022c),Kornet et al. (2022a); Liang and Tang (2013); Liu et al. (2009) or the agitation process Yang et al. (2022); Kornet et al., 2022b, Kornet et al., 2021 have been identified as key aspects in the emulsifying capacity, the stability of emulsions against coalescence, their rheological behavior Kornet et al. (2020) and interfacial activity Drusch et al. (2021); Chang et al. (2015); Gharsallaoui et al. (2009). Given its amphiphilic nature and interfacial activity, PPI has been considered to stabilize interfaces through a mechanism equivalent to classical surfactants (hereafter referred to as the “molecular mechanism”). This includes having a decrease in interfacial tension but also other mechanisms and forces of uttermost importance such as van der Waals interactions, electrostatic repulsions and depletion forces Burger and Zhang (2019); Tcholakova et al. (2008). However, it has been reported in several cases that these macromolecules can also behave as particles to stabilize interfaces through a Pickering mechanism Wang et al. (2022); Li et al. (2022); Sridharan et al. (2020a); Liang and Tang (2014). Pickering emulsions are emulsions whose interface stabilisation is mainly based on the existence of a steric barrier of particles around the interfaces, without a significant decrease in interfacial tension Velandia et al., 2021a; Velandia et al., 2021b); Dinkgreve et al. (2016). Such emulsions have attracted attention for food applications in recent years as they are believed to be highly resistant to coalescence due to an almost irreversible adsorption of the particles Schmitt et al. (2014); Sarkar and Dickinson (2020); Yan et al. (2020); Berton-Carabin and Schroën (2015). Nevertheless, whether particles decrease the interfacial tension is a matter of debate due to the many articles reporting both behaviors Manga et al. (2016); Kutuzov et al. (2007); Forth et al. (2019). Similarly, the high resistance to coalescence has been questioned, since it has been shown in several cases that Pickering emulsions can coalesce relatively easily Dinkgreve et al. (2016); French et al., 2016, French et al., 2015). For PPI-stabilized emulsions, front surface fluorescence and gravimetric techniques have been used to deduce the existence of this stabilization mechanism Hinderink et al. (2021); Sridharan et al. (2020b). Also, imaging methods including confocal laser scanning microscopy (CLSM) and cryo scanning electron microscopy (CryoSEM) have been employed to observe the location of PPI at the interfaces as Pickering particles Kornet et al. (2022a); Sridharan et al. (2020a). While all these methods have yielded valuable information, more work is needed to understand in detail how PPI films are built and stabilize interfaces.
Perhaps one of the most important features of protein-stabilized emulsions is the strong dependence on the behavior of their interfaces Berton-Carabin et al. (2018). In this aspect, interfacial rheology plays a key role in protein film characterization. Whether by means of dilational tests or interfacial shear, interfacial rheology techniques allow to obtain information on molecular interactions and network formation in the protein films Niu et al. (2023); Tseng et al. (2022). Also, it has been shown that interfacial rheology can be related to the bulk rheology of emulsions. This is notably the case under high dispersed phase volume fraction conditions in which the energy required to deform emulsions is strongly related to the energy to deform an oil droplet Fuhrmann et al. (2022); Kim and Mason (2017); Kim et al. (2016); Mason and Scheffold (2014). In other cases, these methods have also been related to emulsion stability. For example, it has been shown in protein films of emulsions stabilized with oxidized proteins that a decrease in the interfacial elastic modulus is characteristic of samples that are more prone to coalescence Berton-Carabin et al. (2018). On a smaller and local scale, microrheology techniques have also been used to study interfacial properties of food gels and emulsions Moschakis (2013); Lu and Corvalan (2016); Moschakis et al. (2006); Tisserand et al. (2012); Wu and Dai (2007); Yang et al. (2017). The latter are very attractive as they allow to obtain more information on the interface stabilization process at a nanometer scale. However, these still present challenges to be solved as the spatial resolution is limited to thermal fluctuations and the tracer particles are limited by their size and the nature of their surface Moschakis (2013); Wu and Dai (2007); Yang et al. (2017). In the context of the complexity and industrial interest in PPI as an emulsifier, the mechanical properties of PPI films have been characterized Gharsallaoui et al. (2009). Higher values of the interfacial elastic modulus were identified under pH conditions in which PPI is charged. Interestingly, this correlates with other researches in which PPI-stabilized emulsions show better emulsifying capacity in the same conditions Liang and Tang (2013); Gharsallaoui et al. (2012).
We set out here to obtain more information about this interfacial stabilisation mechanism using molecular rotors. Upon photo-excitation, these molecules can return to their ground energetic state through intramolecular twisting and fluorescence, with both pathways being dependent on their local environment, in particular on their local viscosity Mirzahossein et al. (2022); Caporaletti et al. (2022); Bittermann et al. (2021); Hosny et al. (2013); Uzhinov et al. (2011); Jee et al. (2010); Haidekker and Theodorakis (2010, 2007); Strehmel et al. (1997). The scale of sensitivity of the fluorescence probes to local structural changes and microviscosity is about a few nm. Therefore, this property has been used to measure the local viscosity of complex systems such as cells Kuimova (2012); Kuimova et al. (2008), lipid membranes Páez-Pérez et al. (2021), micro bubbles Hosny et al. (2013) and water-in-oil interfaces Kang et al. (2020). Additionally, they have been successfully used to assess the structural variation and aggregation process of proteins such as lysozyme and insulin in solution Kubánková et al. (2017); Thompson et al. (2015); Hawe et al. (2010); Kuimova et al. (2008). Due to the scale which they are sensitive to, the use of molecular rotors at oil-water interfaces can potentially be viewed as a microrheology technique allowing to measure structural changes in the constituent parts of protein films.
In this research we probe the interfacial local viscosity of silicone oil-in-water emulsion droplets stabilized with PPI by means of the molecular rotor trans-4-[4-(dimethylamino)styryl]-1-methyl-pyridinium iodide (4-DASPI). We are particularly interested in getting a better understanding on how PPI, which is said to exhibit both molecular and Pickering stabilisation mechanisms, stabilizes water-oil interfaces. To this end, measurements of the fluorescence lifetime of the molecular rotor within the protein films are performed. To induce PPI structural modifications, pH variations prior to emulsification are done. We then compare these results to the fluorescence lifetime of 4-DASPI in PPI solutions and relate them to the protein structure. To our knowledge, this is the first time that the local viscosity of oil-water interfaces stabilized with proteins are probed with molecular rotors and our results pave the way to the understanding of the microscopic, molecular mechanisms responsible for protein-based stabilisation of emulsions.
Yellow pea seed (Pisum sativum L.) were provided by Alimex Europe BV (Sint Kruis, Netherlands). Milli-Q filtrated and deionized water (18 MΩ cm resistivity) was used to prepare all the protein solutions. Silicone Oil (density 0.96 g/mL at 25°C, kinematic viscosity 50 cSt at 25°C), hydrochloric acid (HCl) 0.1 M and 4-DASPI (98% purity) were purchased from Sigma-Aldrich. All reagents were used as received unless specified.
Pea protein isolate was obtained from a standard isolation process and based on previous studies Kornet et al. (2020, 2021, 2022a). Briefly, yellow pea flour was dispersed and stirred for 2 h in deionized water (1:10 ratio). Prior to stirring, the pH was adjusted to 8 with NaOH 1 M. The solution was then centrifuged at 20°C and 10000 g for 30 min to remove the starch fraction. The supernatant was then fractionated through a freeze-drying process by means of an Alpha 2–4 LD plus freeze dryer (Christ, Osterode am Harz, Germany). The resulting isolate was stocked at −18°C.
Protein solutions were prepared by dispersing 1 wt% of the protein isolate in water (pH ≈ 7.3). To break up as many aggregates as possible resulting from the extraction process, the mixture was magnetically stirred at 500 rpm and 20°C for 3 h. The solution was stocked under refrigerated conditions overnight to ensure complete protein hydration. Subsequently, the solution was diluted to a final protein concentration of 0.5 wt% and the molecular rotor 4-DASPI was added to obtain a 10–5 M concentration. The pH was adjusted to acidic (pH = 3.0), isoelectric (pH = 4.6) or close to neutral conditions (pH = 6.3) with 0.1 M HCl and the resulting solution was magnetically stirred for 1 h.
Silicone oil-in-water emulsions were stabilized using the PPI solution of 0.5 wt% as the continuous phase. The dispersed phase volume fraction remained constant at ϕO = 0.5, all samples had a total emulsion volume of 40 mL and the emulsification temperature was held constant at 20°C. Silicone oil was progressively added to the PPI solution while using a Silverson L5 M-A emulsifier at 2000 rpm for approximately 2 min. Then, the samples were homogenized by stirring at 6000 rpm for 18 min. An ice bath was used to control the temperature. Emulsions were characterized after preparation and stored in a fridge at 4°C. The pH was verified before characterization with a pH-meter.
An inverted confocal microscope (Leica TCS SP8) with a hybrid detector was used for the fluorescence lifetime imaging (FLIM) measurements of the emulsions. A 470 nm wavelength pulsed laser with 40 MHz repetition rate was used for all measurements. 4-DASPI presents an absorption maximum at 488 nm and an emission maximum at 600 nm Kim and Lee (1999). Therefore, an emission range between 500 nm and 700 nm was selected. A ×20 dry objective and a ×100 oil immersion objective were used for emulsion visualization (Figure 1), to follow the droplet size variation and to study the fluorescence lifetime at the interfaces (Figure 2A). It is worth noting that only the fluorescent signal immediately surrounding the drops, as shown in yellow in Figure 2A, is selected as the interface. This is done using the Leica Application Suite X software. For each drop of emulsion, a minimum of 10000 counts were taken to obtain a representative signal. To obtain an average lifetime value, a fluorescence decay curve is analysed as shown in Figure 2B. All fluorescence lifetime measurements (interfacial and proteins in solution) were fitted to a bi-exponential decay as hemicyanine dyes like 4-DASPI usually require for better fit Jee et al. (2010); Kim and Lee (1999):
where I(t) is the number of detected photons at time t, while Ai and τi are the amplitude and the lifetime of the ith decay component respectively. The average lifetime values reported here are amplitude-weighted average lifetimes
The error bars indicate the standard error obtained from 10 measurements (10 drops of emulsion) for each pH tested.
FIGURE 1. (Top) Fluorescence intensity images of 4-DASPI in PPI stabilized emulsions at pH 3.0 (A), 4.6 (B) and 6.3 (C). (Bottom) Line profiles of a selection of ≈10 oil droplets per sample. The data is based on the fluorescence intensity I measured in each pixel normalized with the minimum and the maximum value (Imin and Imax) along the normalized cross section of each drop L/Lmax (red line illustrated in B-top).
FIGURE 2. Fluorescence lifetime analysis of 4-DASPI at the interface of PPI-stabilized emulsions at different values of pH. (A) Snapshot of an interface (interface selection in yellow, pH = 4.6) investigated using fluorescence lifetime microscopy (magnification = ×100). Scale bar is 25 μm. (B) Fluorescence decay curves of droplet interfaces at different pH’s. (C) 4-DASPI exhibits the highest value in ⟨τ⟩ (circles) at the interface stabilized at pH 4.6. Using the calibration curve predicts a sharp increase in viscosity (squares) with respect to the emulsions stabilized at pH 3.0 and 6.3.
Fluorescence intensity images along with the normalized line profiles
The fluorescence lifetime analysis at the interface of the PPI-stabilized emulsions is shown in Figure 2. Based on the known mechanism of interface stabilisation with proteins and the proven interfacial activity from interfacial tension studies at different pH’s, PPI forms an interfacial film around the droplets Drusch et al. (2021); Gharsallaoui et al. (2009). We anticipate that both film structure and emulsification properties of proteins vary with pH as shown in other studies Chang et al. (2015); Liang and Tang (2013); Gharsallaoui et al. (2009). For instance, pH influences protein aggregation and therefore the local (nm) viscosity which is probed by molecular rotors (see, for example, Thompson et al. (2015)): a longer fluorescence lifetime will reflect a more viscous environment. The fluorescence decay curves measured directly at the oil-water interfaces (Figures 2A,B) allow us to confirm this effect. For the case of pH 3.0 an equivalent measurement was performed as for the other conditions, selecting the fluorescent area in the interfacial region. A maximum amplitude-weighted average lifetimes of ⟨τ⟩ = 1.78 ± 0.11 ns is observed at the IEP followed by an intermediate (⟨τ⟩ = 1.23 ± 0.04 ns) and minimum value (⟨τ⟩ = 0.58 ± 0.01 ns) at pH 6.3 and pH 3.0 respectively (Figure 2C). It is important to mention that through molecular rotors we are probing the film structure once a state of equilibrium between proteins in the bulk and proteins at the interface is reached. Also, it has been shown that molecular rotor binding can influence the average lifetime in cellular systems Thompson et al. (2015). In our case we know that 4-DASPI does not generate covalent bonds with PPI. Possibly other interactions (electrostatic, hydrophobic, van der Waals) may be influencing our measurement but quantifying their effect separately in a complex system such as proteins at interfaces is beyond the scope of our study. The empirical Förster-Hoffmann equation τf = k ⋅ ηx with τf the fluorescence lifetime and η the viscosity can be used to relate the local viscosity with the fluorescence lifetime of 4-DASPI at the PPI stabilized interfaces (See Supplementary Figure S4 for the calibration curve of 4-DASPI) Förster and Hoffmann (1971). From extrapolation of the obtained calibration curve, an inferred viscosity is calculated for each interfacial condition as shown in Figure 2C right Y-axis. Such values reflect states of higher (pH 4.6) or lower (pH 6.3 and pH 3.0) film density in the environment of 4-DASPI. Surprisingly, the values for the inferred viscosities are between two to three orders of magnitude above the viscosity of a PPI solution measured using rheometry (
A higher film density would intuitively be related to better stability properties of the emulsions or to an increase of the interfacial viscoelastic modulus. However, multiple reports indicate that emulsions stabilized with PPI (and any other protein) have the least optimal stability conditions against coalescence at the IEP Chang et al. (2015); Liang and Tang (2013); Gharsallaoui et al. (2009). Such behavior was also observed by us for the samples at pH 4.6 (data now shown). As mentioned before, confocal images of samples at the IEP (denser film condition) show that the protein films are not homogeneous (See Supplementary Figure S1). As there are areas of high PPI density, e.g., where proteins are shared between interfaces, there are also less denser regions similar to those observed at pH 6.3. The former are produced by PPI aggregation which, in turn, is mainly due to intraprotein electrostatic interactions and Van der Waals forces Zhang et al. (2022a). Indeed, at the IEP almost no electrostatic interactions occur which favours protein aggregation Lam et al. (2018). However, at pH 6.3 and pH 3.0 repulsive electrostatic interactions arise which also result in a decrease of the particle size compared to pH 4.6. The PPI aggregation was confirmed on the protein solutions and characterized with dynamic light scattering (DLS) and infrared spectroscopy (IR) (See Supplementary Figures S2, S3 for details about DLS and IR results). By comparing how the PPI hydrodynamic radius in solution varies with pH and the behavior of the local viscosity in the interface we identify that both follow the same trend. The denser environment probed with 4-DASPI at the IEP suggests that the observed behavior results from the nanometric scale (protein aggregates) and not the microscopic scale (protein film). This also agrees with the spatial resolution of the molecular rotor since its estimated size is 1 nm. Thus, it is to be expected that 4-DASPI shows greater sensitivity to structural changes occurring at a scale close to their spatial resolution (PPI particles and not protein films). Protein aggregation and the consequent increase in the density of the medium explain the different local viscosities measured at the interface. However, to go further, it is important to determine if what is observed in Figure 2C comes only from PPI aggregation or whether other mechanisms play a role. For this, we measured the amplitude-weighted average lifetimes for PPI solutions under different pH conditions in a separate experiment, as shown in Figure 3A. This provides a structural indicator on how pH impacts the building blocks in the bulk, before film formation. Indeed, being in solution, only the confinement associated with inter-protein and intra-protein interactions in the bulk is probed. Two aspects stand out about these results. First, the major effect of pH on the amplitude-weighted average lifetime is also seen for PPI solutions. The same trend is observed as for 4-DASPI behaviour at oil-water interfaces. ⟨τ⟩ has a maximum under isoelectric conditions and two lower values at pH 6.3 and pH 3.0. This can be again correlated to protein aggregation (See Supplementary Figure S3). Higher hydrodynamic radius in solution suggest that the protein aggregates are more densely packed at pH 4.6. As a consequence this induces greater local confinement to the fluorescence probe and causes a larger fluorescence lifetime with τsolution = 2 ns at the IEP. Since the fluorescence lifetime measures the local confinement of the protein aggregates and local crowding, it follows the same trend as the hydrodynamic radius of PPI measured by DLS. Secondly, τinterface shows comparable values to τsolution, which indicates that PPI preserves its structure at the interface. This highlights that the final film structure greatly depends on the protein structure and conformation before emulsification Zhang et al. (2022a); Kornet et al. (2022b), Kornet et al., 2021). Such a characteristic supports both the particle-like activity of proteins as emulsifiers and the presence of a Pickering stabilizing mechanism with PPI. These results can also be contrasted to the dependence on pH of the dilational elastic modulus of PPI films and the stability of emulsions with PPI as measured in other studies under comparable circumstances Amine et al. (2014); Liang and Tang (2013); Gharsallaoui et al. (2009) While we observe a maximum in the IEP and minima in electrostatically charged conditions, the opposite trend has been reported in literature for emulsion stability and interfacial elastic modulus Ladjal-Ettoumi et al. (2016); Gharsallaoui et al. (2009). This confirms, on the one hand, that we are not directly measuring the resistance of the film to deformation with 4-DASPI, but rather the molecular structure of the constituent parts. On the other hand, our findings are consistent with the theory that a more homogeneous film is produced under electrostatically charged conditions (i.e., pH 3.0), primarily as a result of a significantly smaller PPI size Gharsallaoui et al. (2009). It was previously proposed that the high elasticity of the interfacial films under non-isoelectric conditions could be due to the fact that the subunits of PPI (molecular mechanism) had enough time to adsorb and reorganise at the interface Gharsallaoui et al. (2009). By directly measuring the interfacial film region with molecular rotors and confocal microscopy observations, the results support this theory. This remains an interesting piece of information as it is also seen that the Pickering mechanism is more marked as a function of particle aggregation and favoring it implies less optimal stability properties for the emulsions Amine et al. (2014). This suggests, from a nanometre scale, that the most important mechanism for film elasticity and emulsion stability is also the molecular one as mentioned by other studies Sridharan et al. (2020a).
FIGURE 3. Fluorescence lifetime analysis of 4-DASPI in PPI solutions (empty squares) with varying pH (at constant protein concentration 0.5 wt%) (A) and varying protein concentration (B). The amplitude-weighted average lifetime reaches a maximum at the isoelectric point, whilst remaining largely independent of protein concentration. The lifetimes measured at the oil-water interfaces (filled squares in A) are systematically lower than the ones observed in solutions at the same pH.
While the values for τinterface and τsolution are comparable in magnitude, it should be mentioned that a small difference between amplitude-weighted average lifetime in solution and at the interface is observed. The τinterface is in all cases slightly smaller than τsolution. To identify whether this is caused by PPI interparticle interactions or is due to the oil-water interface, exploring a solution-based environment analogous to the protein film at the interface is a useful method. This can be accomplished by increasing the protein concentration in solution to look for a rise in fluorescence lifetime brought on by a change in confinement. This was carried out in the isoelectric case where higher PPI aggregation was found as shown in Figure 3B. Interestingly, no amplitude-weighted average lifetime variation was observed while increasing protein concentration in the studied range. This suggests that increasing the concentration of protein does not change the structure of aggregates, and only increases the number of aggregates. PPI structural modifications in the oil-water interfacial region is thus the last feature that could explain the observed lifetime of 4-DASPI. Indeed, it is well known that the way in which proteins interact with oil also impacts the interfacial reconformations Zhang et al. (2022b). Therefore, in presence of an interface, the hydrophobic regions tend to relocate to be in contact with the oil Beverung et al. (1999). This might explain the small but systematic decrease in the lifetime of 4-DASPI at the oil-water interface compared to the solutions. Additionally, this is in agreement with the molecular size of 4-DASPI given that such reconformation is occurring on the scale of protein particles. Molecular rotors therefore appear as a robust tool to identify multiple features of protein interfacial films, notably how these are built in a nanometric scale. In the future, we foresee that these tools are also used to probe multiscale properties so a more direct relationship between local viscosity of the protein films and properties such as film elasticity or resistance to coalescence can be obtained.
To conclude, the molecular rotor 4-DASPI was used to probe local interfacial viscosities of silicone-oil-in-water emulsions stabilized with PPI under pH modifications. A Pickering stabilisation mechanism was identified with PPI proteins, especially under isoelectric conditions. Bridging of PPI aggregates was observed between oil droplet interfaces. We successfully identified how protein film structures are modified as a function of pH. PPI aggregation and PPI reconformation in the interface characterise the stabilisation mechanism of these oil-water interfaces and can be correctly quantified with 4-DASPI. Amplitude-weighted average fluorescence lifetime in solutions showed an equivalent trend, but systematically higher, as the measurements in the interface with varying pH. Protein aggregates at the interface thus present lower local viscosity compared to simple solutions. We also observe that increasing the protein solution concentration did not impact the measured local viscosities, showing that 4-DASPI is mainly measuring a structural modification at the nanometer scale of PPI. From this study we identify that molecular rotors can be a robust tool to characterize oil-water interfaces with globular proteins that exhibit a Pickering stabilisation mechanism such as PPI.
The raw data supporting the conclusion of this article will be made available by the authors, without undue reservation.
All authors contributed in the design of this research. SV, MB, and EM did the experiments with support from GG and FC. T-CR, PM, VS, MM, and DB guided the research, edited the manuscript and contributed in the analysis. The manuscript was written by SV with support from MB and EM. All authors contributed to the article and approved the submitted version.
This work was supported by Netherlands Organization for Scientific Research (NWO) and Top Institute Food and Nutrition (TiFN).
The authors declare that the research was conducted in the absence of any commercial or financial relationships that could be construed as a potential conflict of interest.
All claims expressed in this article are solely those of the authors and do not necessarily represent those of their affiliated organizations, or those of the publisher, the editors and the reviewers. Any product that may be evaluated in this article, or claim that may be made by its manufacturer, is not guaranteed or endorsed by the publisher.
The Supplementary Material for this article can be found online at: https://www.frontiersin.org/articles/10.3389/frsfm.2023.1093168/full#supplementary-material
Amine, C., Dreher, J., Helgason, T., and Tadros, T. (2014). Investigation of emulsifying properties and emulsion stability of plant and milk proteins using interfacial tension and interfacial elasticity. Food Hydrocoll. 39, 180–186. doi:10.1016/j.foodhyd.2014.01.001
Aswathanarayan, J. B., and Vittal, R. R. (2019). Nanoemulsions and their potential applications in food industry. Front. Sustain. Food Syst. 3, 1. doi:10.3389/fsufs.2019.00095
Berton-Carabin, C. C., Sagis, L., and Schroën, K. (2018). Formation, structure, and functionality of interfacial layers in food emulsions. Annu. Rev. Food Sci. Technol. 9, 551–587. doi:10.1146/annurev-food-030117-012405
Berton-Carabin, C. C., and Schroën, K. (2015). Pickering emulsions for food applications: Background, trends, and challenges. Annu. Rev. Food Sci. Technol. 6, 263–297. doi:10.1146/annurev-food-081114-110822
Beverung, C. J., Radke, C. J., and Blanch, H. W. (1999). Protein adsorption at the oil/water interface: Characterization of adsorption kinetics by dynamic interfacial tension measurements. Biophys. Chem. 81, 59–80. doi:10.1016/S0301-4622(99)00082-4
Bittermann, M. R., Grzelka, M., Woutersen, S., Brouwer, A. M., and Bonn, D. (2021). Disentangling nano- and macroscopic viscosities of aqueous polymer solutions using a fluorescent molecular rotor. J. Phys. Chem. Lett. 12, 3182–3186. doi:10.1021/acs.jpclett.1c00512
Burger, T. G., and Zhang, Y. (2019). Recent progress in the utilization of pea protein as an emulsifier for food applications. Trends Food Sci. Technol. 86, 25–33. doi:10.1016/j.tifs.2019.02.007
Can Karaca, A., Nickerson, M. T., and Low, N. H. (2011). Lentil and chickpea protein-stabilized emulsions: Optimization of emulsion formulation. J. Agric. Food Chem. 59, 13203–13211. doi:10.1021/jf203028n
Caporaletti, F., Bittermann, M. R., Bonn, D., and Woutersen, S. (2022). Fluorescent molecular rotor probes nanosecond viscosity changes. J. Chem. Phys. 156, 201101. doi:10.1063/5.0092248
Chang, C., Tu, S., Ghosh, S., and Nickerson, M. T. (2015). Effect of pH on the inter-relationships between the physicochemical, interfacial and emulsifying properties for pea, soy, lentil and canola protein isolates. Food Res. Int. 77, 360–367. doi:10.1016/j.foodres.2015.08.012
Dinkgreve, M., Velikov, K. P., and Bonn, D. (2016). Stability of LAPONITE®-stabilized high internal phase Pickering emulsions under shear. Phys. Chem. Chem. Phys. 18, 22973–22977. doi:10.1039/C6CP03572H
Drusch, S., Klost, M., and Kieserling, H. (2021). Current knowledge on the interfacial behaviour limits our understanding of plant protein functionality in emulsions. Curr. Opin. Colloid and Interface Sci. 56, 101503. doi:10.1016/j.cocis.2021.101503
Förster, T., and Hoffmann, G. (1971). Die viskositätsabhängigkeit der Fluoreszenzquantenausbeuten einiger farbstoffsysteme. Z. für Phys. Chem. 75, 63–76. doi:10.1524/zpch.1971.75.1_2.063
Forth, J., Kim, P. Y., Xie, G., Liu, X., Helms, B. A., and Russell, T. P. (2019). Building reconfigurable devices using complex liquid–fluid interfaces. Adv. Mater. 31, 1806370. doi:10.1002/adma.201806370
French, D. J., Brown, A. T., Schofield, A. B., Fowler, J., Taylor, P., and Clegg, P. S. (2016). The secret life of Pickering emulsions: Particle exchange revealed using two colours of particle. Sci. Rep. 6, 31401. doi:10.1038/srep31401
French, D. J., Taylor, P., Fowler, J., and Clegg, P. S. (2015). Making and breaking bridges in a Pickering emulsion. J. Colloid Interface Sci. 441, 30–38. doi:10.1016/j.jcis.2014.11.032
Fuhrmann, P. L., Breunig, S., Sala, G., Sagis, L., Stieger, M., and Scholten, E. (2022). Rheological behaviour of attractive emulsions differing in droplet-droplet interaction strength. J. Colloid Interface Sci. 607, 389–400. doi:10.1016/j.jcis.2021.08.124
Gharsallaoui, A., Cases, E., Chambin, O., and Saurel, R. (2009). Interfacial and emulsifying characteristics of acid-treated pea protein. Food Biophys. 4, 273–280. doi:10.1007/s11483-009-9125-8
Gharsallaoui, A., Saurel, R., Chambin, O., and Voilley, A. (2012). Pea (pisum sativum, L.) protein isolate stabilized emulsions: A novel system for microencapsulation of lipophilic ingredients by spray drying. Food Bioprocess Technol. 5, 2211–2221. doi:10.1007/s11947-010-0497-z
Haidekker, M. A., and Theodorakis, E. A. (2010). Environment-sensitive behavior of fluorescent molecular rotors. J. Biol. Eng. 4, 11. doi:10.1186/1754-1611-4-11
Haidekker, M. A., and Theodorakis, E. A. (2007). Molecular rotors—Fluorescent biosensors for viscosity and flow. Org. Biomol. Chem. 5, 1669–1678. doi:10.1039/B618415D
Hawe, A., Filipe, V., and Jiskoot, W. (2010). Fluorescent molecular rotors as dyes to characterize polysorbate-containing IgG formulations. Pharm. Res. 27, 314–326. doi:10.1007/s11095-009-0020-2
Hinderink, E. B. A., Berton-Carabin, C. C., Schroën, K., Riaublanc, A., Houinsou-Houssou, B., Boire, A., et al. (2021). Conformational changes of whey and pea proteins upon emulsification approached by front-surface fluorescence. J. Agric. Food Chem. 69, 6601–6612. doi:10.1021/acs.jafc.1c01005
Hinderink, E. B. A., Sagis, L., Schroën, K., and Berton-Carabin, C. C. (2020). Behavior of plant-dairy protein blends at air-water and oil-water interfaces. Colloids Surfaces B Biointerfaces 192, 111015. doi:10.1016/j.colsurfb.2020.111015
Hosny, N. A., Mohamedi, G., Rademeyer, P., Owen, J., Wu, Y., Tang, M.-X., et al. (2013). Mapping microbubble viscosity using fluorescence lifetime imaging of molecular rotors. Proc. Natl. Acad. Sci. U. S. A. 110, 9225–9230. doi:10.1073/pnas.1301479110
Jee, A.-Y., Bae, E., and Lee, M. (2010). Internal motion of an electronically excited molecule in viscoelastic media. J. Chem. Phys. 133, 014507. doi:10.1063/1.3454724
Kang, J., Lhee, S., Lee, J. K., Zare, R. N., and Nam, H. G. (2020). Restricted intramolecular rotation of fluorescent molecular rotors at the periphery of aqueous microdroplets in oil. Sci. Rep. 10, 16859. doi:10.1038/s41598-020-73980-7
Kim, H. S., and Mason, T. G. (2017). Advances and challenges in the rheology of concentrated emulsions and nanoemulsions. Adv. Colloid Interface Sci. 247, 397–412. doi:10.1016/j.cis.2017.07.002
Kim, H. S., Scheffold, F., and Mason, T. G. (2016). Entropic, electrostatic, and interfacial regimes in concentrated disordered ionic emulsions. Rheol. Acta 55, 683–697. doi:10.1007/s00397-016-0946-3
Kim, J., and Lee, M. (1999). Excited-state photophysics and dynamics of a hemicyanine dye in AOT reverse micelles. J. Phys. Chem. A 103, 3378–3382. doi:10.1021/jp984167e
Kornet, C., Venema, P., Nijsse, J., van der Linden, E., van der Goot, A., and Meinders, M. (2020). Yellow pea aqueous fractionation increases the specific volume fraction and viscosity of its dispersions. Food Hydrocoll. 99, 105332. doi:10.1016/j.foodhyd.2019.105332
Kornet, R., Roozalipour, S. L., Venema, P., van der Goot, A. J., Meinders, M. B. J., and van der Linden, E. (2022a). Coacervation in pea protein solutions: The effect of pH, salt, and fractionation processing steps. Food Hydrocoll. 125, 107379. doi:10.1016/j.foodhyd.2021.107379
Kornet, R., Sridharan, S., Venema, P., Sagis, L. M. C., Nikiforidis, C. V., van der Goot, A. J., et al. (2022b). Fractionation methods affect the gelling properties of pea proteins in emulsion-filled gels. Food Hydrocoll. 125, 107427. doi:10.1016/j.foodhyd.2021.107427
Kornet, R., Veenemans, J., Venema, P., van der Goot, A. J., Meinders, M., Sagis, L., et al. (2021). Less is more: Limited fractionation yields stronger gels for pea proteins. Food Hydrocoll. 112, 106285. doi:10.1016/j.foodhyd.2020.106285
Kornet, R., Yang, J., Venema, P., van der Linden, E., and Sagis, L. M. C. (2022c). Optimizing pea protein fractionation to yield protein fractions with a high foaming and emulsifying capacity. Food Hydrocoll. 126, 107456. doi:10.1016/j.foodhyd.2021.107456
Kubánková, M., López-Duarte, I., Bull, J. A., Vadukul, D. M., Serpell, L. C., de Saint Victor, M., et al. (2017). Probing supramolecular protein assembly using covalently attached fluorescent molecular rotors. Biomaterials 139, 195–201. doi:10.1016/j.biomaterials.2017.06.009
Kuimova, M. K. (2012). Mapping viscosity in cells using molecular rotors. Phys. Chem. Chem. Phys. 14, 12671–12686. doi:10.1039/C2CP41674C
Kuimova, M. K., Yahioglu, G., Levitt, J. A., and Suhling, K. (2008). Molecular rotor measures viscosity of live cells via fluorescence lifetime imaging. J. Am. Chem. Soc. 130, 6672–6673. doi:10.1021/ja800570d
Kutuzov, S., He, J., Tangirala, R., Emrick, T., Russell, T. P., and Böker, A. (2007). On the kinetics of nanoparticle self-assembly at liquid/liquid interfaces. Phys. Chem. Chem. Phys. 9, 6351–6358. doi:10.1039/B710060B
Ladjal-Ettoumi, Y., Boudries, H., Chibane, M., and Romero, A. (2016). Pea, chickpea and lentil protein isolates: Physicochemical characterization and emulsifying properties. Food Biophys. 11, 43–51. doi:10.1007/s11483-015-9411-6
Lam, A. C. Y., Can Karaca, A., Tyler, R. T., and Nickerson, M. T. (2018). Pea protein isolates: Structure, extraction, and functionality. Food Rev. Int. 34, 126–147. doi:10.1080/87559129.2016.1242135
Lee, S.-H., Lefèvre, T., Subirade, M., and Paquin, P. (2009). Effects of ultra-high pressure homogenization on the properties and structure of interfacial protein layer in whey protein-stabilized emulsion. Food Chem. 113, 191–195. doi:10.1016/j.foodchem.2008.07.067
Li, S., Jiao, B., Meng, S., Fu, W., Faisal, S., Li, X., et al. (2022). Edible mayonnaise-like Pickering emulsion stabilized by pea protein isolate microgels: Effect of food ingredients in commercial mayonnaise recipe. Food Chem. 376, 131866. doi:10.1016/j.foodchem.2021.131866
Liang, H.-N., and Tang, C.-h. (2014). Pea protein exhibits a novel Pickering stabilization for oil-in-water emulsions at pH 3.0. LWT - Food Sci. Technol. 58, 463–469. doi:10.1016/j.lwt.2014.03.023
Liang, H.-N., and Tang, C.-H. (2013). pH-dependent emulsifying properties of pea [Pisum sativum (L.)] proteins. Food Hydrocoll. 33, 309–319. doi:10.1016/j.foodhyd.2013.04.005
Liu, F., and Tang, C.-H. (2013). Soy protein nanoparticle aggregates as pickering stabilizers for oil-in-water emulsions. J. Agric. Food Chem. 61, 8888–8898. doi:10.1021/jf401859y
Liu, S., Low, N. H., and Nickerson, M. T. (2009). Effect of pH, salt, and biopolymer ratio on the formation of pea protein isolate-gum Arabic complexes. J. Agric. Food Chem. 57, 1521–1526. doi:10.1021/jf802643n
Lu, J., and Corvalan, C. M. (2016). Soft food microrheology. Curr. Opin. Food Sci. 9, 112–116. doi:10.1016/j.cofs.2016.10.004
Lu, Z. X., He, J. F., Zhang, Y. C., and Bing, D. J. (2020). Composition, physicochemical properties of pea protein and its application in functional foods. Crit. Rev. Food Sci. Nutr. 60, 2593–2605. doi:10.1080/10408398.2019.1651248
Manga, M. S., Hunter, T. N., Cayre, O. J., York, D. W., Reichert, M. D., Anna, S. L., et al. (2016). Measurements of submicron particle adsorption and particle film elasticity at oil–water interfaces. Langmuir 32, 4125–4133. doi:10.1021/acs.langmuir.5b04586
Mason, T. G., and Scheffold, F. (2014). Crossover between entropic and interfacial elasticity and osmotic pressure in uniform disordered emulsions. Soft Matter 10, 7109–7116. doi:10.1039/C4SM01125B
McClements, D. J. (2004). Protein-stabilized emulsions. Curr. Opin. Colloid and Interface Sci. 9, 305–313. doi:10.1016/j.cocis.2004.09.003
Mirzahossein, E., Grzelka, M., Pan, Z., Demirkurt, B., Habibi, M., Brouwer, A. M., et al. (2022). Molecular rotors to probe the local viscosity of a polymer glass. J. Chem. Phys. 156, 174901. doi:10.1063/5.0087572
Moschakis, T. (2013). Microrheology and particle tracking in food gels and emulsions. Curr. Opin. Colloid and Interface Sci. 18, 311–323. doi:10.1016/j.cocis.2013.04.011
Moschakis, T., Murray, B. S., and Dickinson, E. (2006). Particle tracking using confocal microscopy to probe the microrheology in a phase-separating emulsion containing nonadsorbing polysaccharide. Langmuir 22, 4710–4719. doi:10.1021/la0533258
Niu, H., Wang, W., Dou, Z., Chen, X., Chen, X., Chen, H., et al. (2023). Multiscale combined techniques for evaluating emulsion stability: A critical review. Adv. Colloid Interface Sci. 311, 102813. doi:10.1016/j.cis.2022.102813
Páez-Pérez, M., López-Duarte, I., Vyšniauskas, A., Brooks, N. J., and Kuimova, M. K. (2021). Imaging non-classical mechanical responses of lipid membranes using molecular rotors. Chem. Sci. 12, 2604–2613. doi:10.1039/D0SC05874B
Poore, J., and Nemecek, T. (2018). Reducing food’s environmental impacts through producers and consumers. Science 360, 987–992. doi:10.1126/science.aaq0216
Sarkar, A., and Dickinson, E. (2020). Sustainable food-grade Pickering emulsions stabilized by plant-based particles. Curr. Opin. Colloid and Interface Sci. 49, 69–81. doi:10.1016/j.cocis.2020.04.004
Schmitt, V., Destribats, M., and Backov, R. (2014). Colloidal particles as liquid dispersion stabilizer: Pickering emulsions and materials thereof. Comptes Rendus Phys. 15, 761–774. doi:10.1016/j.crhy.2014.09.010
Shahidzadeh, N., Bonn, D., Aguerre-Chariol, O., and Meunier, J. (1999). Spontaneous emulsification: Relation to microemulsion phase behaviour. Colloids Surfaces A Physicochem. Eng. Aspects 147, 375–380. doi:10.1016/S0927-7757(98)00711-0
Shahidzadeh, N., Bonn, D., and Meunier, J. (1997). A new mechanism of spontaneous emulsification: Relation to surfactant properties. Europhys. Lett. 40, 459–464. doi:10.1209/epl/i1997-00488-0
Sridharan, S., Meinders, M. B. J., Bitter, J. H., and Nikiforidis, C. V. (2020a). On the emulsifying properties of self-assembled pea protein particles. Langmuir 36, 12221–12229. doi:10.1021/acs.langmuir.0c01955
Sridharan, S., Meinders, M. B. J., Bitter, J. H., and Nikiforidis, C. V. (2020b). Pea flour as stabilizer of oil-in-water emulsions: Protein purification unnecessary. Food Hydrocoll. 101, 105533. doi:10.1016/j.foodhyd.2019.105533
Strehmel, B., Seifert, H., and Rettig, W. (1997). Photophysical properties of fluorescence probes. 2. A model of multiple fluorescence for stilbazolium dyes studied by global analysis and quantum chemical calculations. J. Phys. Chem. B 101, 2232–2243. doi:10.1021/jp962835v
Tang, C.-H. (2017). Emulsifying properties of soy proteins: A critical review with emphasis on the role of conformational flexibility. Crit. Rev. Food Sci. Nutr. 57, 2636–2679. doi:10.1080/10408398.2015.1067594
Tcholakova, S., Denkov, D., and Lips, A. (2008). Comparison of solid particles, globular proteins and surfactants as emulsifiers. Phys. Chem. Chem. Phys. 10, 1608–1627. doi:10.1039/B715933C
Tcholakova, S., Denkov, N. D., Ivanov, I. B., and Campbell, B. (2006). Coalescence stability of emulsions containing globular milk proteins. Adv. Colloid Interface Sci. 123 (126), 259–293. doi:10.1016/j.cis.2006.05.021
Thompson, A. J., Herling, T. W., Kubánková, M., Vyšniauskas, A., Knowles, T. P. J., and Kuimova, M. K. (2015). Molecular rotors provide insights into microscopic structural changes during protein aggregation. J. Phys. Chem. B 119, 10170–10179. doi:10.1021/acs.jpcb.5b05099
Tisserand, C., Fleury, M., Brunel, L., Bru, P., and Meunier, G. (2012). “Passive microrheology for measurement of the concentrated dispersions stability,” in UK Colloids 2011. Editors V. Starov, and P. Griffiths (Berlin, Heidelberg: Springer), 101–105. doi:10.1007/978-3-642-28974-3_17
Tseng, W.-C., Tsay, R.-Y., Le, T. T.-Y., Hussain, S., Noskov, B. A., Akentiev, A., et al. (2022). Evaluation of the dilational modulus of protein films by pendant bubble tensiometry. J. Mol. Liq. 349, 118113. doi:10.1016/j.molliq.2021.118113
Uzhinov, B. M., Ivanov, V. L., and Melnikov, M. Y. (2011). Molecular rotors as luminescence sensors of local viscosity and viscous flow in solutions and organized systems. Russ. Chem. Rev. 80, 1179–1190. doi:10.1070/RC2011v080n12ABEH004246
Velandia, S. F., Marchal, P., Lemaitre, C., Sadtler, V., and Roques-Carmes, T. (2021a). Evaluation of the repartition of the particles in Pickering emulsions in relation with their rheological properties. J. Colloid Interface Sci. 589, 286–297. doi:10.1016/j.jcis.2021.01.005
Velandia, S. F., Ramos, D., Lebrun, M., Marchal, P., Lemaitre, C., Sadtler, V., et al. (2021b). Exploring the link between interfacial and bulk viscoelasticity in reverse Pickering emulsions. Colloids Surfaces A Physicochem. Eng. Aspects 624, 126785. doi:10.1016/j.colsurfa.2021.126785
Wang, C., Wu, J., Wang, C., Mu, C., Ngai, T., and Lin, W. (2022). Advances in Pickering emulsions stabilized by protein particles: Toward particle fabrication, interaction and arrangement. Food Res. Int. 157, 111380. doi:10.1016/j.foodres.2022.111380
Wu, J., and Dai, L. L. (2007). Apparent microrheology of oil-water interfaces by single-particle tracking. Langmuir 23, 4324–4331. doi:10.1021/la0625190
Yan, X., Ma, C., Cui, F., McClements, D. J., Liu, X., and Liu, F. (2020). Protein-stabilized Pickering emulsions: Formation, stability, properties, and applications in foods. Trends Food Sci. Technol. 103, 293–303. doi:10.1016/j.tifs.2020.07.005
Yang, J., Mocking-Bode, H. C. M., van den Hoek, I. A. F., Theunissen, M., Voudouris, P., Meinders, M. B. J., et al. (2022). The impact of heating and freeze or spray drying on the interface and foam stabilising properties of pea protein extracts: Explained by aggregation and protein composition. Food Hydrocoll. 133, 107913. doi:10.1016/j.foodhyd.2022.107913
Yang, N., Lv, R., Jia, J., Nishinari, K., and Fang, Y. (2017). Application of microrheology in food science. Annu. Rev. Food Sci. Technol. 8, 493–521. doi:10.1146/annurev-food-030216-025859
Zhang, M., Fan, L., Liu, Y., Huang, S., and Li, J. (2022a). Effects of proteins on emulsion stability: The role of proteins at the oil-water interface. Food Chem. 397, 133726. doi:10.1016/j.foodchem.2022.133726
Zhang, W., Xu, X., Zhao, X., and Zhou, G. (2022b). Insight into the oil polarity impact on interfacial properties of myofibrillar protein. Food Hydrocoll. 128, 107563. doi:10.1016/j.foodhyd.2022.107563
Keywords: pea protein isolate, molecular rotor, interfaces, emulsions, local viscosity
Citation: Velandia SF, Bittermann MR, Mirzahossein E, Giubertoni G, Caporaletti F, Sadtler V, Marchal P, Roques-Carmes T, Meinders MBJ and Bonn D (2023) Probing interfaces of pea protein-stabilized emulsions with a fluorescent molecular rotor. Front. Soft. Matter 3:1093168. doi: 10.3389/frsfm.2023.1093168
Received: 08 November 2022; Accepted: 28 February 2023;
Published: 10 March 2023.
Edited by:
Anne-laure Fameau, Institut National de recherche pour l’agriculture, l’alimentation et l’environnement (INRAE), FranceReviewed by:
Fabrice Cousin, UMR12 Laboratoire Léon Brillouin (LLB), FranceCopyright © 2023 Velandia, Bittermann, Mirzahossein, Giubertoni, Caporaletti, Sadtler, Marchal, Roques-Carmes, Meinders and Bonn. This is an open-access article distributed under the terms of the Creative Commons Attribution License (CC BY). The use, distribution or reproduction in other forums is permitted, provided the original author(s) and the copyright owner(s) are credited and that the original publication in this journal is cited, in accordance with accepted academic practice. No use, distribution or reproduction is permitted which does not comply with these terms.
*Correspondence: Santiago F. Velandia , cy5mLnZlbGFuZGlhcm9kcmlndWV6QHV2YS5ubCYjeDAyMDBhOw==
Disclaimer: All claims expressed in this article are solely those of the authors and do not necessarily represent those of their affiliated organizations, or those of the publisher, the editors and the reviewers. Any product that may be evaluated in this article or claim that may be made by its manufacturer is not guaranteed or endorsed by the publisher.
Research integrity at Frontiers
Learn more about the work of our research integrity team to safeguard the quality of each article we publish.