- 1CNRS, INRAE, ENSCL, UMET, University Lille, Villeneuved’Ascq, France
- 2Departamento de Química Física, Universidad Complutense de Madrid, Madrid, Spain
- 3Instituto Pluridisciplinar, Universidad Complutense de Madrid, Madrid, Spain
- 4Instituto de Física del Sur (IFISUR UNS-CONICET), Bahía Blanca, Argentina
- 5Univ Rennes, CNRS, IPR (Institut de Physique de Rennes)-UMR 6251, Rennes, France
It is now well-known that the assembly of particles at fluid/fluid interfaces, and the resulting dynamical properties of such particle-laden interfaces can provide high stabilization of dispersed systems such as emulsions and foams. Here, we focus on the emerging case of “protein particles,” a novel family of bio particles. We provide an updated perspective about their definition, production, bulk and interface properties, highlighting the most recent results of the obtained bioparticle-laden interfaces, and how such protein particles can stabilize liquid dispersions. The ability of protein particles for undergoing a fast adsorption to fluid/fluid interfaces and for forming viscoelastic layers play a key role on the prevention of drainage, coalescence, or coarsening/ripening, which results in the formation of very stable particle-stabilized foams and emulsions. Therefore, protein particles are an excellent bio-based alternative to synthetic surfactants and other conventional stabilizers for ensuring the stabilization of a broad range of dispersed systems, opening new avenues for the design of new products with interest for cosmetic, food and biomedical industries.
1 Introduction
Interface-dominated systems have gained attention in recent years as result of their ubiquitous presence in nature and different technological fields, ranging from dietary products to cosmetic industries, and from pharmaceutical formulations to tissue engineering (Wege et al., 2008; Guo et al., 2016; Cristofolini et al., 2018). The adsorption of particles to fluid/fluid interfaces, both liquid/gas and liquid/liquid, is commonly exploited in the stabilization of dispersed systems, such as foams and emulsions, and for encapsulation in pharmaceutical formulations (Huang et al., 2015; Akbari and Wu, 2016; Murray and Phisarnchananan, 2016). They lead to the so-called Pickering or Ramsden foams and emulsions (Binks, 2002). This has attracted the interest of many researchers to untangle the mechanism underlying the formation of interfacial layers at fluid/fluid interfaces by the adsorption of both colloidal particles and microgels (Guzmán et al., 2022; Guzmán and Maestro, 2022).
Among the broad range of particles currently available, protein-based particles are more and more studied as they can be bio-based alternative for the stabilization of fluid/fluid interfaces, opening new avenues towards the design of most biocompatible and biodegradable consumer products (Wouters et al., 2019). For instance, the amphiphilicity of alcohol-soluble proteins (e.g., zein, kafirin and gliadin), and their low solubility in water, provide to these type of proteins promising properties to form colloidal particles with suitable surface activity for the stabilization of dispersed systems (Sun et al., 2019; Zhu et al., 2019; Ma et al., 2020; Wouters et al., 2020). Moreover, the ability of protein particles for stabilizing interfacial systems is strongly dependent on the specific environmental parameters (Thewissen et al., 2011; Bayles and Vermant, 2022). Protein particles show a strong responsiveness against changes on the pH, temperature and ionic strength. For instance, the modification of the above parameter can force the swelling-shrinking (size changes) processes of protein particles or modify the effective charge of the particles which can alter the adsorption kinetics of particles to the interface, the interfacial rheology or their ability for the stabilization of emulsions and foams (Wan et al., 2016a). This dependence on physical variables such as temperature and pH open the door to the design of responsive or smart dispersions by using protein particles as already described in the literature for other stabilizers (Lencina et al., 2018; Fameau and Fujii, 2020; Martinelli et al., 2021; Ritacco, 2022). Therefore, the identification of the most suitable protein particles for ensuring original fluid/fluid interfaces properties, and for obtaining tuneable biphasic dispersions, requires a careful examination of the specific properties of the selected particles (Liu et al., 2012; Liu and Tang, 2013). Still, several aspects related to the relationship between the properties of this novel family of bioparticles, and their ability to adsorb at liquid interfaces and to stabilize foams and emulsions are still unclear.
This review first provides the basic knowledge on solid particles at interfaces, for readers not familiar with this topic. Then, we introduce what are protein particles, how to produce them, and their different types and origins. In the next section, we focus on the behaviour of these particles at interfaces: their amphiphilicity, dynamics of adsorption and interfacial rheology. Finally, we summarize the current state of knowledge concerning the stabilization of foams and emulsions by these protein particles. We hope that the review will help the readers to better know this new type of particles, the state of the art on their use, and to contribute on the future development of potential applications. Their potential is huge: as listed at the end of the article, they have specific properties, differencing them from other stabilizers, and also as proteins are natural components, cheap and available in large quantity.
2 Driving forces guiding the assembly of particles at fluid/fluid interfaces
2.1 Free energy minimization: Surface tension decrease and contact angle
The particles adsorption at fluid/fluid interfaces are different compared to those of conventional low molecular weight surfactant (Tcholakova et al., 2008). The assembly of particles at fluid/fluid interfaces is mediated by a reduction of the fluid/fluid interfacial tension, γ12, which can be explained in term of the rupture of the continuity of the interface. Therefore, the trapping of particles at the interface reduces the contact area between the fluids, driving to a reduction of the mean free energy of the system. In fact, particle adsorption to the fluid/fluid interface induces a 2D lateral pressure Π at the interface that counteracts the tendency of the interfacial tension to reduce the area of the interface, guiding an effective reduction of the interfacial tension as result of the interplay between entropic contributions and the inter-particle interactions (Binks, 2002; Garbin, 2013; Maestro et al., 2015; Ballard et al., 2019; Forth et al., 2019; Guzmán, 2020; Guzmán et al., 2022). This leads to an interfacial tension for a particle-laden interface defined as
where the lateral pressure is dependent on the interfacial coverage (Γ). Eq. 1 assumes a microscopy description of the fluid interface where interface should be considered in the molecular scale, which is not the case when particles are considered. These are commonly larger than the interface width, and hence the microscopic view is lacked of physical meaning. This makes necessary to define the interfacial tension of a particle-laden interface as an effective magnitude which cannot be understood from a classical thermodynamic perspective (Guzmán et al., 2022). It should be noted that the understanding of the equilibration process of particle-laden interface also requires to consider the trapping energy of a particle to the fluid interface (ΔEp). In fact, the energy change associated with the transport of a particle from the bulk to its final equilibrium position at the interface allows characterizing the mechanical equilibrium of particle-laden fluid/fluid interface, defined by the Young’s Law, and hence defines the equilibrium position of particles with respect to the interfacial plane (Figure 1). Therefore, assuming mechanical equilibrium conditions are fulfilled (
with R being the particle radius. The
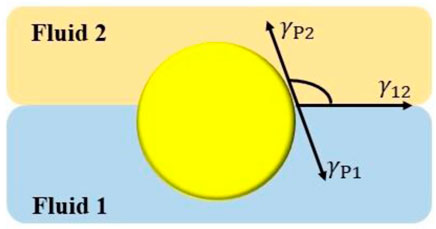
FIGURE 1. Geometrical description of the equilibrium position of a particle attached to a fluid/fluid interface. γ12 is the fluid/fluid interfacial tension; γP1 and γP2 are the solid/fluid interfacial tensions between the particle and fluid 1 and fluid 2, respectively, and θ is the particle contact angle with the interface.
It is worth mentioning that in most cases the capillary binding defined by ΔEp exceeds many times the thermal energy contributions (kBT, where kB is the Boltzmann`s constant and T the absolute temperature), which leads to a situation characterized by a quasi-irreversible trapping of the particles at the fluid interface. However, this irreversibility should be considered a matter of particle size. For instance, the adsorption of micron-sized particles is characterized for values of the trapping energy in the range 106 kBT −107 kBT, and hence their adsorption to fluid/fluid interfaces is quasi-irreversible, except when the fluid/fluid interfacial tension assumes a very low value, or the contact angle is close to the boundary values of 0° and 180°. On the other side, small particles, commonly with diameter below 10 nm, present lower values of the trapping energy at the fluid interface (several kBT) which can drive a thermal-activated escape of the particles from the interface, resulting in an adsorption-desorption equilibrium similar to that found in traditional surfactants, polymer and proteins (Guzmán et al., 2021; Guzmán et al., 2022). Therefore, it can be expected that in most cases the thermal fluctuations cannot force the detachment of particles from the interface, resulting in a quite different role of particles as stabilizing agents in relation to molecular systems which in turn defines the specific properties of particle-stabilized emulsions and foams. Further details on the driving forces governing the trapping of particles to fluid interfaces can be found in recent reviews and we invite the readers to have a look at them (Maestro et al., 2014; Zanini and Isa, 2016; Dasgupta et al., 2017; Guzmán et al., 2022).
2.2 Interparticle interactions at fluid/fluid interfaces
ΔEp is probably the most important contribution to the interfacial energetic landscape of particle-laden interfaces, and their effective contribution can be defined as a sum of the contribution associated with the trapping of each individual particle. However, as soon as the interfacial coverage increases, it is necessary to introduce additional contributions to the energetic balance associated with the interactions operating between particles which present different origins (Bresme and Oettel, 2007; Garbin et al., 2012; Maestro, 2019).
The analysis of the inter-particle interactions in particle-laden interfaces, in absence of externally triggered contribution, requires including two different types of contributions. The former includes the direct forces that are also present in bulk systems. However, the presence of a fluid/fluid interface changes their role. The second type of interactions emerges from the confinement within an interface, e.g., capillary and hydrodynamic ones. This type of forces is strongly dependent on the specific chemistry and morphology of the particles, and on the chemical nature of the fluid phases separated by the interface (Maestro et al., 2015; Maestro et al., 2018; Maestro and Guzmán, 2019; Guzmán et al., 2021; Guzmán et al., 2022).
As was stated above, the direct interactions can be also found when particle dispersions are considered. However, their characteristics and strength are changed by the presence of the interface. Despite, the importance of direct interactions when particle at fluid/fluid interfaces are studied, the many attractive and repulsive contributions that they include have made difficult to untangle their true contribution to the energetic landscape of particle-laden interface.
Van der Waals interactions are one of the most important contributions to the energetic landscape of particle-laden interface, accounting for the interactions between the different components of the particles, becoming weaker than for particle dispersions. It should be noted that the quantification of the van der Waals contribution is not trivial for particle-laden interfaces, and it is common to define an effective van der Waals contribution in terms on an effective Hamaker constant which considers the individual Hamaker constant of the particles across the two fluid phases, and the equilibrium position of the particles within the fluid/fluid interface. This leads to a situation characterized by an effective van der Waals contribution depending on the specific chemistries of the particles and the fluid phases, as well as on the relative wettability of the particles for the interface.
Electrostatic interactions also contribute to the energetic landscape of particle-laden interfaces when charged particles are considered. However, this contribution is quite different to what is found in particle dispersion, adding to the short-range screened Coulomb appearing in bulk system, a new long-range dipolar interaction, which depends on the chemical nature of the fluids separated by the interface. In fact, the presence of the interface introduces asymmetry to the electrostatic interactions between particles, occurring very differently across polar and non-polar phases. This can be rationalized considering the different origin of the dipolar interactions depending on the nature of the fluid (Bresme and Oettel, 2007). Thus, the interaction between the particle fractions immersed in a polar phase, such as water, occurs between the dipoles formed by the dissociated groups on the particle surface and the free counterions existing on the bulk phase, while the interaction across the non-polar phase is originated by an image charge effect.
Together with the electrostatic and van der Waals interactions, steric and hydrophobic interactions can also appear for particles trapped at fluid interfaces. However, their true contribution to the energetic landscape of particle-laden interfaces has not been properly quantified yet.
The interfacial confinement of particles drives the emergence of new types of inter-particle forces which are not present in bulk systems. In particular, capillary forces, resulting from the deformation of the interface due to the particle adsorption, play a key role on the energetic landscape of particle-laden interfaces. The capillary force is a vector which can be expressed in terms of the components in the direction of gravity and its normal, and equal to the product of the length of the three-phase contact line and the surface tension (Kralchevsky and Nagayama, 2000; Kralchevsky et al., 2001). If just one particle is floating at the interface, the deformation produced by the particle on the interface is symmetric about the direction of gravity, and the force components are balanced. But if there is another particle nearby the first one, the deformed interface around the particles lost the symmetry and the menisci of both particles can interact with each other to produce additional lateral forces, the so called lateral capillary forces. Those forces can be attractive or repulsive depending on the interface deformation around the neighbour particles, which in turn depends on particle size, density, shape, particle-particle distance, contact angle, surface tension, capillary length, etc. It should be noted that capillary interactions can involve large number of particles, which can lead to the formation of self-assembled particles films through the so-called “Cheerios” effect (Vella et al., 2006). The interfacial confinement also contributes to the appearance of the hydrodynamic interactions which are the result of the motion of particles through a viscous medium. This results in a flow modified by the presence of the rest of the particles. However, the role of this type of interaction is not always easy to quantify, appearing weaker than capillary interactions (Martínez-Pedrero, 2020; Guzmán et al., 2022).
3 Protein particles in bulk
3.1 Protein particles: Definition and sources
Most proteins can be considered as amphiphilic molecules characterized by their specific biological functions. Their amphiphilic character allows their adsorption to fluid/fluid interfaces, contributing to the interfacial tension reduction, which has led to their extensive exploitation since many years as agents enabling the stabilization of emulsions and foams in the Food industry (Evans et al., 2013; Lam et al., 2014). This ability for stabilizing emulsions and foams is then associated with their capacity of forming thick layers at the interfaces, providing efficient steric repulsions and eventually limiting the coalescence of bubbles (droplets) in foams (emulsions) (Murray and Ettelaie, 2004; Dickinson, 2009). Moreover, proteins are also known to form protein aggregates in solution, which can strengthen the stability of emulsions and foams (Murray and Ettelaie, 2004; Kargar et al., 2012). However, when compared to surfactants, the dynamics of adsorption of a protein is much slower (due to the large mass of the proteins); as a consequence, large concentrations of proteins are usually required to provide fast enough interfacial coverage and stability of foams and emulsions.
In recent years, it has been described in the literature how to convert proteins and especially food proteins into nanoparticles to produce and stabilize Pickering emulsions and foams (Wouters and Delcour, 2019). Note that the term protein particle is commonly referred to nanometric size (few hundred of nanometers), rigid, spherical, compact particles containing a high protein concentration, which can be based on animal, plant proteins or both (Yan et al., 2020) (Table 1). Protein aggregates are in a sense also particle-like stabilizers, but here we focus on rigid protein particles of nanometric size or protein aggregates of few hundred of nanometers. The difference between protein particles and protein aggregates is not so clear and not easy to define. Although the term “rigid” is commonly used, the protein particles may not be solid as commonly perceived. For example, proteins particles can rearrange at fluid/fluid interfaces. That is why we can find in the literature the term “soft solid” for these particles. In fact, there is still not a clear definition for solid protein particles or nanoparticles until now in the literature. In parallel, it is also possible to prepare soft and deformable particles containing a lower amount of protein. This type of particles are the so-called proteins microgels, also presenting a growing interest for the stabilization of dispersed systems (Dickinson, 2017). Protein microgels are micron-sized to sub-micron gel-like colloidal particles that essentially consist of a cross-linked network of proteins. The microgel particles are swollen by the solvent (water), and therefore contains a high quantity of water. In that respect, protein particle can be considered as “moderately soft”: an intermediate state between the real solid particle (such as silica particles) and the highly swellable protein microgels. Based on these criteria, in this review we only focus on protein nanoparticles, having high amount of protein and low amount of solvent in the core.
An important point regarding the sourcing for these particles, as that they can be obtained both from plant and animal proteins. As well, they can be produced from hydrophilic and hydrophobic proteins. For examples, soy protein, zein, prolamin, and milk protein have been described to easily produced protein particles (Wang et al., 2022). It is also important to notice that these particles are generally rough, not homogeneous and uniform. Finally, most of the particles described in the literature are also responsive to temperature, pH or ionic strength, because it leads to change of their surface charge and wettability. This feature is a major advantage if one wants to produce dispersions reacting to the environmental conditions. It is important to mention that protein particles offer a wide range of shape, even anisotropic ones: one can get spherical or globular particles, fibrils, tubes or fractal-like (Wang et al., 2022). For example, for zein, in addition to generating discrete particles trough liquid-liquid dispersion, zein also has been extensively studied to fabricate nanofibers via electrospinning (Patel, 2020). Although these anisotropic shapes are also very interesting as foam and emulsion stabilizers, they are not included here.
3.2 Protein particles formation
There are several methodologies for the fabrication of protein-based particles. Nanoparticles of proteins can be produced by sonication, desolvation, heating and pH adjustment, and anti-solvent precipitation (Figure 2). The most extended strategy is based on the liquid anti-solvent precipitation method. This requires a controlled decrease of the solvent quality of an aqueous protein solution, commonly by adding ethanol or acetic acid, for forcing a controlled aggregation of the protein, leading to a dispersion of homogeneously distributed particles (Joye and McClements, 2013) The use of the anti-solvent precipitation method is a reproducible, scalable and cheap strategy to obtain protein particles, finding a broad application field in food and pharmaceutical industries (Joye and McClements, 2013; Wouters and Delcour, 2019).
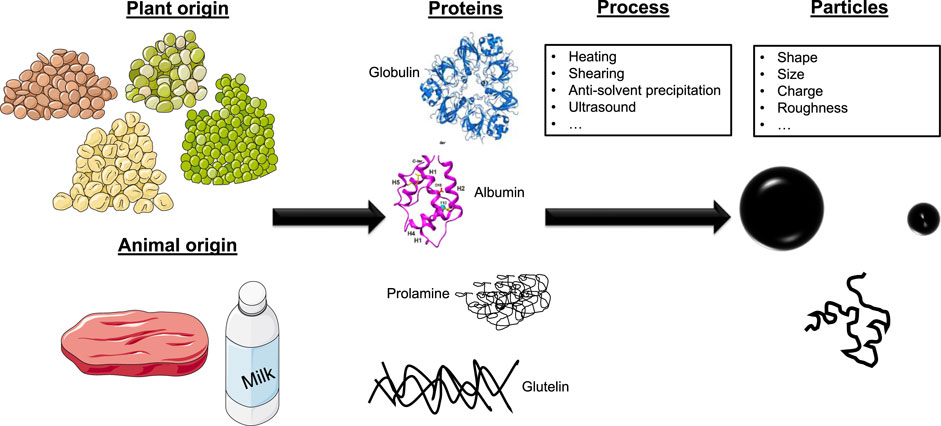
FIGURE 2. Schematic summarizing the origin of the proteins (animal or plant), the main proteins and the process used to produce the protein particles with different characteristics. The figure was partly generated using Servier Medical Art, provided by Servier, licensed under a creative commons attribution 3.0 unported license.
As already pointed out, particles can be obtained from both hydrophobic and hydrophilic proteins. One of the most used protein family to obtain protein particles is the prolamin. Prolamins are strongly hydrophobic proteins which can self-assemble to form spherical particles in presence of water. The prolamin family includes a wide range of chemically diverse proteins, including zein, kafirin, and gliadin. Nanoparticles of this proteins can be obtained by different methods, e.g., anti-solvent precipitation, solvent evaporation or pH driven precipitation.
The interest of prolamin particles is associated with their ability for stabilizing Pickering emulsions due to their insolubility in both water and oils (Hu et al., 2016; Dai et al., 2018). It should be noted that highly hydrophobic particles are not generally very effective for the stabilization of emulsions and foams due to their tendency to form insoluble clumps under specific conditions. However, their applicability can be optimized by the addition of hydrophilic molecules during particles formation, including hydrophilic proteins, polysaccharides or polyphenol, which provides a modulation of the hydrophilic-lipophilic balance of the protein particle surface to optimize their adsorption to fluid/fluid interfaces while it inhibits their self-aggregation (de Folter et al., 2012; Yan et al., 2020).
On the other side, hydrophilic proteins can be exploited under specific conditions to manufacture particles enabling the stabilization of dispersed systems. This is commonly possible by a controlled thermal denaturation of the specific proteins under appropriate solution conditions. Soy protein is probably the most widely used example of this latter type of proteins (Liu and Tang, 2013; Zhu et al., 2017; Zhu et al., 2018a). Soy proteins can form nanoparticles, fibrils and microparticles depending on the medium conditions and process parameters (Patel, 2020).
4 Protein particles at interfaces
Related to foam or emulsion formation is the ability of a “particle” to transport from bulk and adsorb at the fluid-fluid interface. Before dealing with dynamics and interfacial rheology, let’s first make a few remarks specific to protein particles. As described previously, the criterion required for adsorption is linked to the contact angle. An important difference between inorganic solid spherical particles, like silica ones, and protein particles is that the latter presents the ability to deform at interfaces and thus, the validity of Eq. 1, is questionable (Zanini and Isa, 2016; Sarkar and Dickinson, 2020). Furthermore, many protein-based particles are not completely spherical and rough in these cases obviously Eq. 1 is too simplistic (Binks and Horozov, 2006). Even more, it was found that even for smooth particles, the contact angle evolves in time logarithmically (Kaz et al., 2012), which means very long equilibration times (out of equilibrium systems). Besides these specific features, the adsorption energies are always generally several orders of magnitude larger than kBT, thus, as for all other particles, the adsorption of protein particles can be considered irreversible.
4.1 Adsorption dynamics of protein particles and interfacial rheology
Together with the ability to adsorb onto the interface—reducing surface tension and providing repulsive forces between bubbles or drops, the dynamics of this adsorption is also crucial in the formation of foams and emulsions (Langevin, 2000). For foaming and emulsification, a fast adsorption is required, so that the bubbles (drops) are already efficiently covered during the mixing process: they are thus “protected” when coming in contact and can repel each other’s.
As a first example, Peng et al. reported results on the adsorption of gliadin particles to the liquid/vapor interface. They evaluated the evolution of the surface pressure with time in the presence of gliadin particles (Peng et al., 2017). They show that a strong variation of the surface tension (pressure) within the first 10 min, due to the adsorption of the gliadin particles. Importantly, the mass transport rate of gliadin particles exceeds that what is found for most of the proteins, e.g., soy glycinin, β-lactoglobulin, and casein: by using proteins under the form of a particle, the adsorption rate can thus be enhanced. Note also that the increase in surface pressure is comparable to what is usually find for protein solutions (ΔΠ = 20 mN/m). After the initial adsorption, the decrease of the interfacial tension continues during at least 2 h until a constant value is reached. Further studies on the adsorption kinetics of gliadin particles pointed out that, as expected, the initial adsorption can be increased by raising the particle concentration from 0.05 mg/mL to 1 mg/mL (Peng et al., 2017). Figure 3 shows the time (t) evolution of the surface pressure for the adsorption of gliadin particles to the water/vapor interface from dispersions of different concentrations. According to other results by Peng et al. (Peng et al., 2018), the adsorption of protein particles at fluid/fluid interfaces slows down after a critical interfacial coverage is reached. This is associated with the presence of adsorption barriers. As a practical consequence, an increase of the charge density of the particles enhances this electrostatic barrier effect.
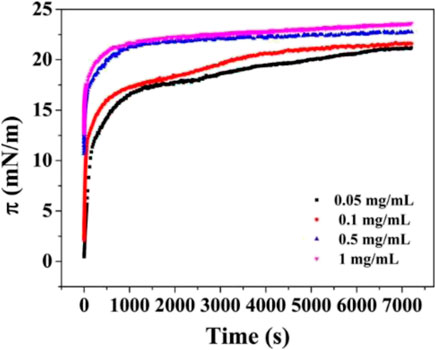
FIGURE 3. Adsorption kinetics, in terms of the time evolution of the surface pressure, for the adsorption of gliadin particles at the water/vapor function from dispersions of different concentrations. Reprinted from Peng et al. (2017), with permission from the American chemical society, copyright (2017).
Liu and Tang (Liu and Tang, 2014) explored the role of the particle concentration on the formation dynamics of the particle layer at an oil/water interface, describing the existence of up to three different steps: i) diffusion of particles from the bulk to the interface; ii) particle penetration and structural deformation at the interface, and iii) rearrangement of adsorbed particles at the interface and multilayer formation. In that respect, this process is comparable to what is usually found for polyelectrolytes adsorption; but it clearly shows that the integrity of the protein particles is—at least partially—removed once adsorbed at the interface.
Further studies on the adsorption of protein particles at fluid/fluid interfaces suggested the existence of an induction time during the first part of the adsorption following by a persistent increase of the adsorption with time (Peng et al., 2017). This induction time was dependent on the particle concentration. In fact, for the adsorption of soy glycinin particles, the induction was around 100 s for the lowest particle concentrations, and was shortened with the increase of concentration, reaching a quasi-null value for a particle concentration about 0.1% w/w. This dependence may be ascribed to a reduced flexibility and susceptibility of the particle to conformational changes, and hence the induction time could be mainly limited by the interfacial coverage. We can suppose that once the induction time is overcome, the interfacial coverage progress following a diffusion-controlled adsorption process and the diffusion constant increasing with the particle concentration. It is worth mentioning that the diffusion controls the adsorption over the whole adsorption process at low particle concentrations, while it is only relevant during the initial adsorption stages for the highest particle concentrations.
After the initial diffusion-controlled adsorption, the penetration of the particles into the interface together with their structural rearrangement proceed following a first order kinetics, which evidence the central role of the structural rearrangement of the adsorbed particles at the interface in the control of the formation process of protein particle interfacial layers (Liu and Tang, 2016).
Once adsorbed, “stabilizers” can provide—or not—mechanical responses to deformations, beyond the basic interfacial tension. These deformations can either correspond to a dilation/compression of the interface, or to shear of this interface. One can then introduce interfacial dilatational and shear viscoelasticity (Langevin, 2000; Murray and Ettelaie, 2004). For instance, simple surfactant does not provide any interfacial viscoelasticity under shear, while adsorbed proteins induce highly elastic interfacial layers both under compression and under shear (Tcholakova et al., 2008). The main interest of such interfacial viscoelasticity is that it is related to the foam and emulsion stability; foams and emulsions with interfaces having high interfacial viscoelastic moduli have slower aging process (see Section 5), and thus longer stability. This is also identified in terms of “surface mobility,” introducing non-dimensional numbers describing the coupling between the hydrodynamics in bulk and at interfaces (Saint-Jalmes, 2006; Manikantan and Squires, 2020). For interfaces covered by protein particles (Mendoza et al., 2014), only a few studies on dilatational interfacial rheology can be found in literature.
In most of the existing studies, it can be first pointed out that the interfacial layers show some non-zero dilational viscoelastic properties: this reflects a strong anchoring of the particles at the interfaces, which get packed and deformed rather than desorbing. The study by Peng et al. (2017) show that the viscoelastic modulus of gliadin particles adsorbed at fluid/fluid interfaces remains at low values until the interfacial coverage overcomes a threshold percolation value (corresponding to a surface pressure value of about 15 mN/m), and then it undergoes a fast increase as a result of the strong interfacial interactions emerging from the high particle density at the interface. Moreover, the viscoelastic character of interface covered by protein particle exceeds what is found for layers of common proteins. The dilatational viscoelastic modulus of gliadin particle layers at fluid/fluid interfaces under close-packing conditions reach values well above 50 mN/m, which are significantly higher than the values reported for conventional protein layers, e.g., β-casein (Fainerman et al., 2018).
In parallel, Wouters et al. (2020) studied the behaviour of gliadin-based nanoparticles at air/water interfaces and found that their viscoelastic dilatational modulus was strongly dependent on the pH. Layers obtained at pH 4 and 6 present low and high values of the viscoelastic dilational moduli, with the storage modulus being almost twice higher than the loss one. They found that the reduction of the pH was associated with an increase of the particle charge, which according to the work by Zhang et al. (2021b) introduces an electrostatic hindrance to the adsorption process, which leads to the slowing down of the initial adsorption rate at the water/gas interface (Peng et al., 2018). Finally, Zou et al. studied hydrophobic zein nanoparticles mixed with tannic acid spread at the air-water interface (Zou et al., 2018). They found an unexpected result, the interfacial elasticity decreased when surface pressure increased.
Concerning, interfacial shear rheometry, there are basically no reported measurements. However, based on previous works on proteins layers, one can predict that interfaces covered by these protein particles will have a high shear elastic modulus, as found for entangled proteins at interfaces. Thus, even if more work is needed to characterize the rheology of protein-based particles at fluid interfaces, the existing measurements converge to the existence of high interfacial viscoelastic moduli (in dilatation and probably in shear), as it is generally found for proteins solutions. In that respect, this is an advantage for foam and emulsion stability: such high viscoelastic interfaces imply low interfacial mobility, and must slow down aging effects, as discussed in Section 5 (Saint-Jalmes, 2006; Manikantan and Squires, 2020).
4.2 Structural aspects of protein particles at fluid/fluid interfaces
The occurrence of structural changes of proteins when they adsorb at the fluid-fluid interfaces is a non-resolved problem yet, despite the efforts made to elucidate the mechanisms and dynamics of these processes (Graham and Phillips, 1979; Postel et al., 2003; Yano et al., 2009). That is why it is even less documented and understood in the case of protein particles, despite its importance on the interfacial rheology.
In the case of oil/water interfaces, we can mention the studies by Bergfreund et al. (2021) and Liu and Tang (2016). The authors explored the organization of soy glycinin particles and found that these particles assemble forming a 2D-square array, which is quite unusual for the arrangement of particles at interfaces, for which hexagonal structures are often observed (Bonales et al., 2011). This is consistent with the existence of a strong lateral repulsion between the particles at the interface, trying to maximize as much as possible the inter-particle space (Liu and Tang, 2016). At air/water interfaces, two studies are worth to mention. Jordens et al. (2013) studied the structure of semiflexible β-lactoglobulin fibrils at the air-water interface by atomic force microscopy (AFM) and found that the fibrils form a surface structure in which nematic domains coexist with randomly oriented fibrils. The coexistence of these domains could explain the surface rheological behaviour of these fibrils that we mention in the previous section (Wan et al., 2016b) Also using the same technique AFM, Yang et al. (2020) studied whey protein isolates (WPI) beads at the air-water interface. They found that the beads are randomly distributed throughout the film, separated by areas, where smaller proteinaceous material is present. This is again related to the surface rheology behaviour, for WPI beads it was actually found that the films are more fluid-like than for native WPI.
As it happens with surface rheological properties of protein-based particles, the knowledge we have about the 2D structure of protein particles at fluid interfaces is scarce. Here also, much more work is needed if we want to understand the connections between this 2D organisation, the interfacial rheology and the ability of stabilizing dispersions. In the future, a better understanding could probably be obtained by using new developments of X-ray and neutron reflectivity techniques (Binks and Horozov, 2005; Skoda, 2019).
As a brief summary of this section on protein particles at interfaces, and to provide the main messages to the readers, we can mention that: 1) protein particles spontaneously and rapidly adsorb at liquid interfaces; 2) They are able to re-arrange once adsorbed at interfaces; 3) they provide high interfacial dilatational viscoelasticity, even higher than the one of the single protein solutions; 4) the interfacial mobility is low, and such interfaces can be considered are rigid for hydrodynamics issues; and 5) since these particles are responsive to medium changes, they exhibit responsive interfacial properties depending on the pH, ionic strength, salt and solvent.
5 Foam and emulsion stabilized by protein particles
Foams are complex systems formed by a dispersion of a gas in a liquid continuous phase. Similarly, emulsions are dispersions of a liquid into another liquid. These dispersions present a key role in a broad range of food and cosmetic products due to their ability for providing original properties, quite different from those of the non-dispersed initial phases (Sagis and Scholten, 2014; Narsimhan and Xiang, 2018). Such properties find their origin in self-organized hierarchical structures, ranging from molecular scale of liquid interfaces to the intermediate ones of thin fluid films and bubbles (or drops, for emulsions), and up to the macroscopic scale (Drenckhan and Hutzler, 2015). Dispersing one phase into another is not spontaneous, and requires delivering some work to the system, foaming and emulsifying techniques need to be used to that aim (Drenckhan and Saint-Jalmes, 2015). As well, stabilizing agents need to be added to the fluid phases to provide the creation of a dispersion, and to increase its stability in time. Without such an addition of stabilizing agents, a foam (or an emulsion) rapidly collapses, or can only be dynamically sustained thanks to a continuous injection of energy. For foams, the dynamics of destabilization are very complex (Ritacco, 2020), the three main processes involved in the temporal evolution are: drainage, coarsening and coalescence (Saint-Jalmes, 2006) but collective and cooperative dynamics also play an important role (Ritacco, 2020). For emulsions, one has to deal with sedimentation, creaming, ripening and coalescence, while specific effects, like flocculation, also occurs (Saint-Jalmes, 2006; Salonen et al., 2010; Langevin, 2015). Foams and emulsions stabilized by proteins as surface active agents are very common in food industry. The current trend in the food industry is to replace animal-based proteins by plant-based proteins. A recent review by Sagis and Yang (Sagis and Yang, 2022) describes the differences between foams and emulsions stabilized with animal-based proteins and plant-based proteins. Still, finding new stabilizers is a permanent quest, to tackle both environmental as well as consumer demands; therefore, various groups have recently started to study how protein particle could stabilize foams and emulsions.
5.1 Foams stabilized by protein particles
There are still not much results on foams, but all recent studies converge on a good efficiency of protein particles to stabilize foams, in strong correlations with fast adsorption and with a high interfacial viscoelasticity. The fast adsorption at the water/gas interface found during the initial adsorption stages enhances the foamability, while the foam stability is enhanced due to the formation of a viscoelastic interfacial network resulting from strong inter-particle interactions. More precisely, it is proposed that freshly formed foams are characterized by the formation of interfaces coated by non-homogenous particle layers, which undergo densification with time. This results in the progressive formation of a network structure characterized by the vanishing of discrete particles, providing efficient steric barriers against coalescence and hindering the gas diffusion between the bubbles (Peng et al., 2017). Therefore, the stability of foams depends on the interfacial coverage and hence is correlated to the dilatational viscoelasticity of single particle-laden interfaces (Peng et al., 2017). Such clear correlations between foam stability and high interfacial dilatational viscoelasticity were, for example, reported for foams based on gliadin nanoparticles (Peng et al., 2018). However, one must also point out that not all protein particles provide uniform and viscoelastic interfaces; this is not the case for gliadin particles as highlighted by Wouters et al. (2020), Wouters et al. (2019), Wouters and Delcour (2019).
It is also important to highlight that the medium condition can be used to tune the foaming properties. For example, pH has been exploited for controlling the ability of gliadin nanoparticles for the stabilization of foams. By tuning the adsorption rate with a pH reduction, Zhang et al. (2021b) have shown a reduction on the foamability and foam stability. On the other side, the temperature did not affect the ability of gliadin nanoparticles, resulting in the production of foams with similar characteristic, independently of the temperature (Peng et al., 2018). Moreover, Peng et al. (2020) studied the ability of stabilizing foams by gliadin nanoparticles obtained by the anti-solvent precipitation method (Viçosa et al., 2012). They used different liquids to produce the particles. They found that the solvent used for the preparation of the particles significantly influences the final stability of the obtained foams. The preparation of particles in the presence of ethanol leads to a significant increase of the foamability and foam stability. This can be ascribed to changes in the amphiphilicity, and to different ability of the particles to adsorb at a fluid interface. Moreover, this can also modify the final viscoelastic properties of the obtained particle-laden interface.
5.2 Emulsions stabilized by protein particles
Different studies compared emulsions stabilized by proteins and by the same proteins, but under the form of particles. For example, Burgos-Díaz et al. (2019) showed that lupin protein particles provided a better ability to stabilize emulsions than the isolated form of the protein. They also observed that particle-stabilized emulsions are formed of smaller droplets than those stabilized solely by the protein, mainly due to more efficient coverage of the droplets. As most of the aging effects of emulsions strongly depends on droplet size, having smaller sizes also provides higher stability, especially against gravitational destabilization.
Another important parameter for emulsion stabilization is the quantity of protein particles. Xiao et al. (2016) exploited kafirin particles for the stabilization of ultra-stable oil-in-water concentrated Pickering emulsions having volume fractions of oil phase in the range 59%–79%. The droplet size and rheological properties of these Pickering emulsions were strongly dependent on the particle concentration. The increase of the particle concentration leads to an increase of the emulsification ability, i.e., the emulsion volume increases, the average size of the oil droplets decreases together with an increase of the storage modulus of the emulsions, consistently with the results found for pea protein particles (Liang and Tang, 2014). In the same way, the droplets of Pickering emulsions stabilized with peanut protein particles at a concentration of 0.5% w/w undergo a fast growth in size during the first stages of their storage, whereas emulsions stabilized with higher particle concentration (2% w/w) do not present any significant modification during 40 days of aging. This is the result of the increase of nanoparticles adsorbed at the oil/continuous phase interface which prevents the vanishing of small droplets in larger ones and drives to the formation of a gel-like network of flocculated oil droplets, hindering the creaming (Ning et al., 2020). When the concentration of particles is not enough to cover the whole droplet/continuous phase interface, it is possible to observe also the formation of a gel-like network (Liu and Tang, 2016). Soy protein isolate nanoparticles have shown the same trend in oil-in-water Pickering emulsions (Liu and Tang, 2013; Xiao et al., 2016). At low volume fraction of the oil phase (around 0.2), the increase of particle concentration leads to a reduction of the droplet size, enhancing the stability of the obtained emulsions against coalescence and creaming (Figure 4A). On the other side, the increase of the volume fraction of oil phase drives a progressive increase of the emulsion stability against creaming, which is ascribed to the formation of gel-like networks entrapping the oil droplets (Figures 4B, C). The formation of this gel-like networks becomes much more favourable at the highest particle concentration, which leads to the formation of emulsions with small droplets and a high resistance against creaming (Figure 4D). At high particle concentration, the phenomena affecting the droplet integrity such as their collapse and coalescence are avoided (Ning et al., 2020). Even though the actual droplet-scale mechanisms leading to an increased stability remain questionable, it is well demonstrated that the concentration of the particles is a central parameter.
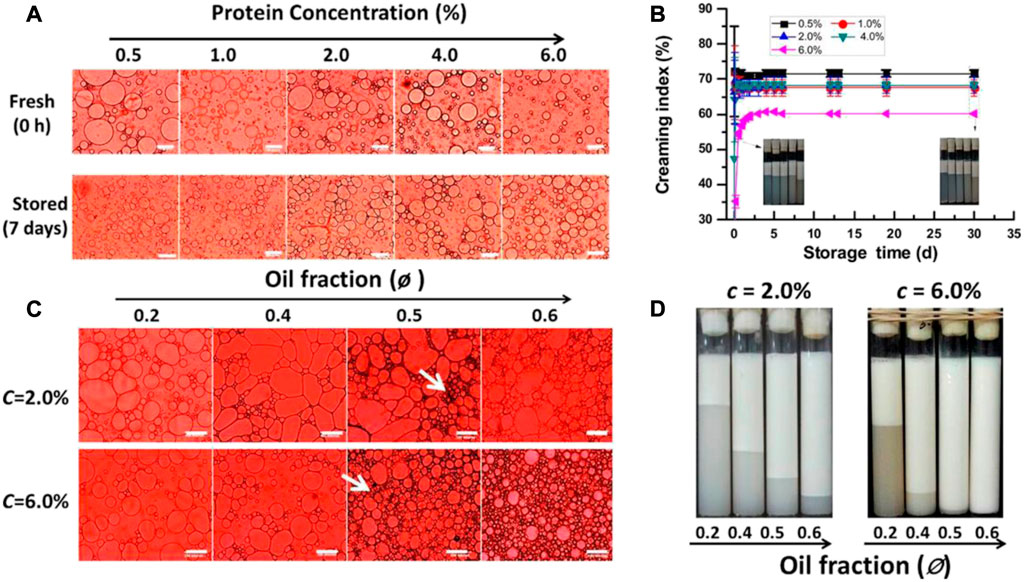
FIGURE 4. (A) Micrographs of emulsions droplets stabilized using soy protein nanoparticles obtained using dispersion of different concentration as were obtained and after aging for 1 week. The scale bars in the micrographs are 100 μm in length. (B) Evolution of the creaming index for emulsions prepared with a fixed particle concentration and different volume fractions of oil phase. (C) Micrographs of emulsions droplets stabilized using two different concentrations of soy protein nanoparticles and different oil volume fractions. The scale bars in the micrographs are 100 μm in length, and the arrows indicate flocculated oil droplets. (D) Aspects of emulsions stabilized using two different concentrations of soy protein nanoparticles and different oil volume fractions after 1 day of aging. Reprinted from Liu and Tang (2013), with permission from the American chemical society, copyright (2013).
The shape has also a strong effect on emulsion properties. The exploitation of the specific properties of fluid interfaces covered by anisotropic particles is an emerging area of colloid and interface science (Guzmán et al., 2022). Protein fibrils can be considered a good example of anisotropic particles that can be used for food application. Gao et al. (2017) prepared oil-in-water Pickering emulsions using β-lactoglobulin fibrils. These emulsions, with droplets with an average diameter in the range 10–20 μm, were obtained after homogenization using a shear-homogenizator at 20000 rpm: they had an excellent stability, remaining without coalescence at room temperature for 56 days, when they were prepared using a fibril concentration in the range of 5–20 mg/mL and at a pH far from the isoelectric point of the protein fibrils. However, the use of pH values close to the protein particle isoelectric point or a fibril concentration above 25 mg/mL results in the formation of coarse emulsions characterized by the presence of large droplets as a result of the fibril aggregation.
As already pointed out for foams, the aqueous medium conditions can tune the emulsion stability. The modification of the ionic strength in the aqueous phase allows changing the particle wettability as proved by Wu et al. (2015) using whey protein particles. They found that the stability of the emulsions can be ensured under a partial wetting. However, when the pH is close to the isoelectric point or the ionic strength is high, particles become more hydrophobic, and their ability for stabilizing Pickering emulsion is worsened. On the contrary, for pH above and below the isoelectric point, and low to moderate ionic strength, it is possible to obtain stable Pickering emulsions.
The role of the particle wettability on the ability for stabilizing emulsions was also evidenced from the studies of de Folter et al. (2012) who studied the ability of particles obtained from zein protein derived from corn for stabilizing oil-in-water emulsions. They found that zein proteins particles allows stabilizing oil-in-water emulsions at pH above and below their isoelectric point, whereas the ionic strength is maintained within moderate values (1–10 mM). Nevertheless, emulsions coalesce easily for low particle concentrations and pH, whereas at high ionic strength flocculated emulsion are obtained. Similar trends were observed by Hu et al. (2016) with gliadin particles stabilizing o/w emulsions. Gliadin particles produced micron-sized droplets for pH ≥ 4, but become unstable against coalescence when the pH drops to 3. At low pH, particles present a high charge density, which prevents the droplet stabilization, while as the charge density of the particles is reduced, the formation of the particle armour on the droplet surface together with the formation of a particle network bridging the droplets enhance the emulsion stabilization. This leads to the formation of emulsions made of flocculated droplets as the pH increases. For kafirin particles, the emulsion stability can be also enhanced by increasing the ionic strength of the aqueous phase. This favours the migration of the particles to the interface and their coagulation, forming gel-like emulsions. This is compatible with the results by Qin et al. (2018) who reported that quinoa protein particles allow the preparation of emulsions with better resistance to freeze-thaw cycles when salt is added. This was ascribed to the formation of gel-like 3D structures and multi-layered network on the droplet surface, which drives to the formation of small droplets. Moreover, as the salt concentration is increased, the stability of the emulsions is enhanced due to the inhibition of salt crystal formation (“salting-out” effect). Figure 5 shows a schematic representation of the mechanism controlling the salt effect on the stabilization of emulsions by quinoa protein particles. Zhu et al. (2018) confirmed the ability of salt for improving the stability of emulsions obtained using soy protein particles against droplet coalescence and creaming. Moreover, they reported, together with the “salting-out” effects, the formation of strong interfacial films on the droplet surface. It should be noted that the ability of salt for enhancing the stability of Pickering emulsions stabilized by proteins particles is independent of whether the salt was added in the aqueous phase before the emulsification process or once the emulsion is obtained. It is also worth mentioning that the addition of salts does not significantly affect the size of droplet. Nevertheless, they force the flocculation processes, resulting in emulsions with enhanced shear-thinning behaviour, and strong dependence of the viscosity with the shear rate. Therefore, it may be expected that the addition of (monovalent salts) may alter the microstructure and flow behaviour of the obtained Pickering emulsions, without affecting their stability (Benetti et al., 2019).
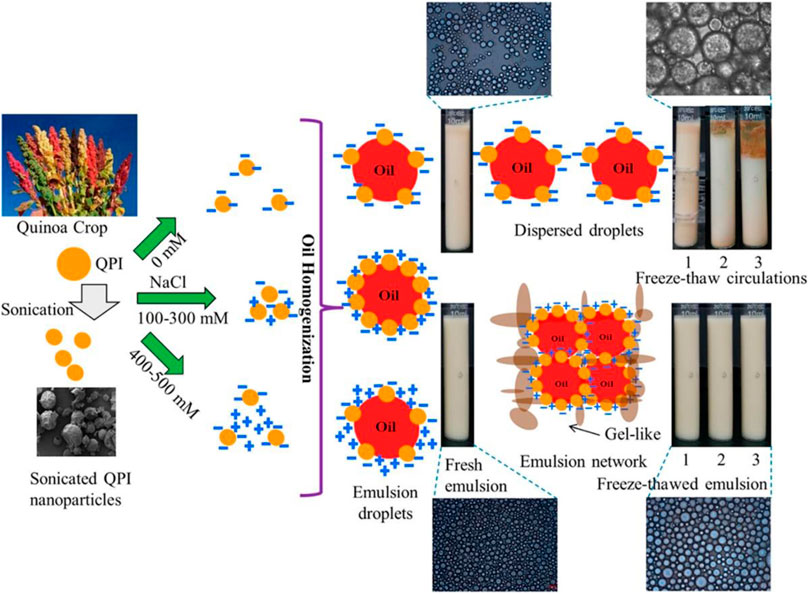
FIGURE 5. Schematic representation of the role of salt addition on the stabilization of pickering emulsions using quinoa protein particles. Reprinted from Qin et al. (2018), with permission from the American chemical society, copyright (2018).
Another way to tune the aqueous phase is to modulate the polarity. The ability of gliadin particles as emulsion stabilizer can be modulated by reducing the polarity of the aqueous phase leading to most stable Pickering emulsions than in pure aqueous solution as a result of the increase of the number of hydrophobic groups of the particles exposed to the interface. This favours the adsorption of the particles to the fluid/fluid interface, thus forming viscoelastic layers and hindering the destabilization phenomena (Zhang et al., 2021b).
The specific wettability of protein particles for the fluid/fluid interface can also influence the rheological properties of emulsions as pointed out by the studies by Zou et al. (2018). For instance, the decrease of the hydrophobicity leads to a significant increase of the storage modulus of the rheological response, which evidences the formation of a strong gel-like network. Moreover, the cross-over strain undergoes an increase with the decrease of the hydrophobicity, providing an indication that the gel network increases its resistance against yielding. On the other side, the particle concentration and oil volume fraction also play a very important role on the control of the rheological properties of emulsions stabilized by protein particles, resulting in power law dependences of the storage modulus as the protein concentration or oil content increase. These power-laws are characterized by an exponent that decrease with the weakening of the inter-particle hydrophobic interactions, which suggests an important role of the hydrophobic interactions within the particle networks in the continuous phase and the oil droplets on the control of the gel nature of the obtained emulsions.
One of the challenges is now to understand in detail the exact mechanisms connecting the changes in the hydrophobicity of the particles to the macroscopic foam/emulsion properties, via its interfacial and bulk organisation. The properties of the interfacial layer at the surface of the droplet are crucial, but it seems also that gelation in the continuous phase can be an important element. As well, comparing stability of emulsion requires that parameters like the droplet size and the oil fraction are controlled and kept constant, which is not always the case.
6 Conclusion and perspectives: Protein particles specificities, potential and issues
From the analysis of the most recent literature, it emerges that protein particles—in terms of interfacial properties and as dispersion stabilisers—have properties at least as good, or often better, than the ones of single proteins or of solid particles. First, protein particles exhibit intermediate softness: they are “real” particle and more rigid than the highly swellable microgels, but they can still be deformed and the particles can rearrange in terms of interfacial positions once adsorbed at interfaces (which is not the case for solid particles). In that sense, they share the advantages of the two extreme limits. As well, their initial shape can also be adjusted, from needle-like shapes to more spherical ones. Also, these protein particles have good ability to adsorb at fluid-fluid interfaces with a fast dynamic. This adsorption is followed by structural rearrangements, and - consequently—interfacial layers have high viscoelasticity (possibly even higher than the ones of protein solutions). Therefore, these proteins particles can efficiently stabilize foams and emulsions, with long stability in time, partially due to the high interfacial viscoelasticity (i.e., low interfacial mobility). They are easy to produce, cheap and that they can be made both from animal and vegetal proteins makes them excellent alternatives to more conventional stabilisers. Moreover, the responsiveness to pH, polarity and ionic strength allows an unprecedented ability to adjust the wettability, flexibility and integrity of the particles, thus controlling the final foam and emulsion properties.
Still, much more work is needed to understand all the aspects involved on the formation and long-term stability of dispersions formulated using protein particles. Among the remaining questions are the links between the 2D organisation of particles at the interfaces and how it impacts the interfacial dynamics; as well, one can wonder about the dependence of the foam and emulsion features on particle size, roughness, and shapes. Also, the role of traces of small proteinaceous compounds is not clear; such tiny molecules seem to play a role and may probably help to “soften” the interface, and to give them more flexibility (otherwise, they could be too brittle, like those covered by solid particles). This opens some routes on the optimisation of chemical formulations, by mixing small stabilizers and protein particles.
At this stage, we also miss some explanations, at the scale of the bubbles and drops, of what exactly happen within the foams and the emulsions (in the continuous bulk phase and at interface), as a function of the different experimental conditions and types of protein particles. Probably, dedicated experiments on interfaces, foams, and emulsions, using simple protein particle solutions but with controlled physical parameters (bubble sizes and volume fraction) will complement and help to identify the physical mechanisms, and to rationalize an increasing amount of data. A better understanding will also probably be obtained in the future by combining different in situ techniques to resolve the interfacial structures and rearrangement with time such as neutron and X-reflectivity. As well, new routes for the synthesis of more elaborated particles, with controlled wettability, amphiphilicity, shape, patchiness, will also be explored, offering us more and more efficient protein particles-based stabilizers and multiple opportunities for the fabrication of environmentally friendly products, with application in food, pharmacy and cosmetics. Nevertheless, most of the studies up to now are still at the laboratory scale, and up to the date their exploitation in commercially developed product remains limited.
Author contributions
All authors listed have made a substantial, direct, and intellectual contribution to the work and approved it for publication.
Funding
EG, acknowledge the financial support of MICINN under grant PID 2019-106557GB-C21, and EU on the framework of the European Innovative Training Network- Marie Sklodowska-Curie Action Nano Paint (Grant Agreement 955612). HR thanks the financial support of the Agencia Nacional de Promoción Científica y Tecnológica (ANPCyT), Argentina, under grant PICT-2019-3185, of the Universidad Nacional del Sur (UNS), Argentina, under grant PGI-UNS 24/F080 and from CONICET under grant PIP 11220200101754CO.
Conflict of interest
The authors declare that the research was conducted in the absence of any commercial or financial relationships that could be construed as a potential conflict of interest.
The handling editor FM declared a shared affiliation with the author(s) EG at the time of review.
Publisher’s note
All claims expressed in this article are solely those of the authors and do not necessarily represent those of their affiliated organizations, or those of the publisher, the editors and the reviewers. Any product that may be evaluated in this article, or claim that may be made by its manufacturer, is not guaranteed or endorsed by the publisher.
References
Akbari, A., and Wu, J. (2016). Cruciferin nanoparticles: Preparation, characterization and their potential application in delivery of bioactive compounds. Food Hydrocoll. 54, 107–118. doi:10.1016/j.foodhyd.2015.09.017
Ballard, N., Law, A. D., and Bon, S. A. F. (2019). Colloidal particles at fluid interfaces: Behaviour of isolated particles. Soft Matter 15, 1186–1199. doi:10.1039/C8SM02048E
Bayles, A., and Vermant, J. (2022). Divide, conquer, and stabilize: Engineering strong fluid–fluid interfaces. Langmuir 38, 6499–6505. doi:10.1021/acs.langmuir.2c00948
Benetti, J. V. M., do Prado Silva, J. T., and Nicoletti, V. R. (2019). SPI microgels applied to pickering stabilization of O/W emulsions by ultrasound and high-pressure homogenization: Rheology and spray drying. Food Res. Int. 122, 383–391. doi:10.1016/j.foodres.2019.04.020
Bergfreund, J., Diener, M., Geue, T., Nussbaum, N., Kummer, N., Bertsch, P., et al. (2021). Globular protein assembly and network formation at fluid interfaces: Effect of oil. Soft Matter 17, 1692–1700. doi:10.1039/D0SM01870H
Binks, B. P., and Horozov, T. S. (2005). Aqueous foams stabilized solely by silica nanoparticles. Angew. Chem. Int. Ed. 44, 3722–3725. doi:10.1002/anie.200462470
Binks, B. P., and Horozov, T. S. (2006). “Colloidal particles at liquid interfaces: An introduction,” in Colloidal particles at liquid interfaces. Editors B. P. Binks, and T. S. Horozov (Cambridge,UK: Cambridge University Press), 1–74. doi:10.1017/CBO9780511536670.002
Binks, B. P. (2002). Particles as surfactants—Similarities and differences. Curr. Opin. Colloid and Interface Sci. 7, 21–41. doi:10.1016/S1359-0294(02)00008-0
Bonales, L. J., Rubio, J. E. F., Ritacco, H., Vega, C., Rubio, R. G., and Ortega, F. (2011). Freezing transition and interaction potential in monolayers of microparticles at fluid interfaces. Langmuir 27, 3391–3400. doi:10.1021/la104917e
Bresme, F., and Oettel, M. (2007). Nanoparticles at fluid interfaces. J. Phys. Condens. Matter 19, 413101. doi:10.1088/0953-8984/19/41/413101
Burgos-Díaz, C., Wandersleben, T., Olivos, M., Lichtin, N., Bustamante, M., and Solans, C. (2019). Food-grade Pickering stabilizers obtained from a protein-rich lupin cultivar (AluProt-CGNA®): Chemical characterization and emulsifying properties. Food Hydrocoll. 87, 847–857. doi:10.1016/j.foodhyd.2018.09.018
Cristofolini, L., Orsi, D., and Isa, L. (2018). Characterization of the dynamics of interfaces and of interface-dominated systems via spectroscopy and microscopy techniques. Curr. Opin. Colloid Interface Sci. 37, 13–32. doi:10.1016/j.cocis.2018.06.001
Dai, L., Sun, C., Wei, Y., Zhan, X., Mao, L., and Gao, Y. (2018). Formation and characterization of zein-propylene glycol alginate-surfactant ternary complexes: Effect of surfactant type. Food Chem. 258, 321–330. doi:10.1016/j.foodchem.2018.03.077
Dasgupta, S., Auth, T., and Gompper, G. (2017). Nano- and microparticles at fluid and biological interfaces. J. Phys. Condens. Matter 29, 373003. doi:10.1088/1361-648x/aa7933
de Folter, J. W. J., van Ruijven, M. W. M., and Velikov, K. P. (2012). Oil-in-water Pickering emulsions stabilized by colloidal particles from the water-insoluble protein zein. Soft Matter 8, 6807–6815. doi:10.1039/C2SM07417F
Dickinson, E. (2017). Biopolymer-based particles as stabilizing agents for emulsions and foams. Food Hydrocoll. 68, 219–231. doi:10.1016/j.foodhyd.2016.06.024
Dickinson, E. (2009). Hydrocolloids as emulsifiers and emulsion stabilizers. Food Hydrocoll. 23, 1473–1482. doi:10.1016/j.foodhyd.2008.08.005
Drenckhan, W., and Hutzler, S. (2015). Structure and energy of liquid foams. Adv. Colloid Interface Sci. 224, 1–16. doi:10.1016/j.cis.2015.05.004
Drenckhan, W., and Saint-Jalmes, A. (2015). The science of foaming. Adv. Colloid Interface Sci. 222, 228–259. doi:10.1016/j.cis.2015.04.001
Evans, M., Ratcliffe, I., and Williams, P. A. (2013). Emulsion stabilisation using polysaccharide–protein complexes. Curr. Opin. Colloid and Interface Sci. 18, 272–282. doi:10.1016/j.cocis.2013.04.004
Fainerman, V., Kovalchuk, V., Aksenenko, E. V., Zinkovych, I. I., Makievski, A. V., Nikolenko, M. V., et al. (2018). Dilational viscoelasticity of proteins solutions in dynamic conditions. Langmuir 34, 6678–6686. doi:10.1021/acs.langmuir.8b00631
Fameau, A.-L., and Fujii, S. (2020). Stimuli-responsive liquid foams: From design to applications. Curr. Opin. Colloid and Interface Sci. 50, 101380. doi:10.1016/j.cocis.2020.08.005
Forth, J., Kim, P. Y., Xie, G., Liu, X., Helms, B. A., and Russell, T. P. (2019). Building reconfigurable devices using complex liquid–fluid interfaces. Adv. Mater. 31, 1806370. doi:10.1002/adma.201806370
Gao, Z., Zhao, J., Huang, Y., Yao, X., Zhang, K., Fang, Y., et al. (2017). Edible Pickering emulsion stabilized by protein fibrils. Part 1: Effects of pH and fibrils concentration. LWT - Food Sci. Technol. 76, 1–8. doi:10.1016/j.lwt.2016.10.038
Garbin, V. (2013). Colloidal particles: Surfactants with a difference. Phys. Today 66, 68–69. doi:10.1063/PT.3.2158
Garbin, V., Crocker, J. C., and Stebe, K. J. (2012). Nanoparticles at fluid interfaces: Exploiting capping ligands to control adsorption, stability and dynamics. J. Colloid Interface Sci. 387, 1–11. doi:10.1016/j.jcis.2012.07.047
Gou, S., Chen, Q., Liu, Y., Zeng, L., Song, H., Xu, Z., et al. (2018). Green fabrication of ovalbumin nanoparticles as natural polyphenol carriers for ulcerative colitis therapy. ACS Sustain. Chem. Eng. 6, 12658–12667. doi:10.1021/acssuschemeng.8b01613
Graham, D. E., and Phillips, M. C. (1979). Proteins at liquid interfaces: III. Molecular structures of adsorbed films. J. Colloid Interface Sci. 70, 427–439. doi:10.1016/0021-9797(79)90050-X
Guo, J., Zhou, Q., Liu, Y. C., Yang, X. Q., Wang, J. M., Yin, S. W., et al. (2016). Preparation of soy protein-based microgel particles using a hydrogel homogenizing strategy and their interfacial properties. Food Hydrocoll. 58, 324–334. doi:10.1016/j.foodhyd.2016.03.008
Guzmán, E., Abelenda-Núñez, I., Maestro, A., Ortega, F., Santamaria, A., and Rubio, R. G. (2021). Particle-laden fluid/fluid interfaces: Physico-chemical foundations. J. Phys. Condens. Matter 33, 333001. doi:10.1088/1361-648x/ac0938
Guzmán, E., and Maestro, A. (2022). Soft colloidal particles at fluid interfaces. Polym. (Basel) 14, 1133. doi:10.3390/polym14061133
Guzmán, E., Martínez-Pedrero, F., Calero, C., Maestro, A., Ortega, F., and Rubio, R. G. (2022). A broad perspective to particle-laden fluid interfaces systems: From chemically homogeneous particles to active colloids. Adv. Colloid Interface Sci. 302, 102620. doi:10.1016/j.cis.2022.102620
Hu, Y. Q., Yin, S. W., Zhu, J. H., Qi, J. R., Guo, J., Wu, L. Y., et al. (2016). Fabrication and characterization of novel Pickering emulsions and Pickering high internal emulsions stabilized by gliadin colloidal particles. Food Hydrocoll. 61, 300–310. doi:10.1016/j.foodhyd.2016.05.028
Huang, Z., Scicolone, J. v., Han, X., and Davé, R. N. (2015). Improved blend and tablet properties of fine pharmaceutical powders via dry particle coating. Int. J. Pharm. 478, 447–455. doi:10.1016/j.ijpharm.2014.11.068
Jordens, S., Isa, L., Usov, I., and Mezzenga, R. (2013). Non-equilibrium nature of two-dimensional isotropic and nematic coexistence in amyloid fibrils at liquid interfaces. Nat. Commun. 4, 1917. doi:10.1038/ncomms2911
Joye, I. J., and McClements, D. J. (2013). Production of nanoparticles by anti-solvent precipitation for use in food systems. Trends Food Sci. Technol. 34, 109–123. doi:10.1016/j.tifs.2013.10.002
Kargar, M., Fayazmanesh, K., Alavi, M., Spyropoulos, F., and Norton, I. T. (2012). Investigation into the potential ability of Pickering emulsions (food-grade particles) to enhance the oxidative stability of oil-in-water emulsions. J. Colloid Interface Sci. 366, 209–215. doi:10.1016/j.jcis.2011.09.073
Kaz, D., McGorty, R., Mani, M., Brenner, M. P., and Manoharan, V. (2012). Physical ageing of the contact line on colloidal particles at liquid interfaces. Nat. Mater. 11, 138–142. doi:10.1038/nmat3190
Kralchevsky, P. A., Denkov, N. D., and Danov, K. D. (2001). Particles with an undulated contact line at a fluid interface: Interaction between capillary quadrupoles and rheology of particulate monolayers. Langmuir 17, 7694–7705. doi:10.1021/la0109359
Kralchevsky, P. A., and Nagayama, K. (2000). Capillary interactions between particles bound to interfaces, liquid films and biomembranes. Adv. Colloid Interface Sci. 85, 145–192. doi:10.1016/S0001-8686(99)00016-0
Lam, S., Velikov, K. P., and Velev, O. D. (2014). Pickering stabilization of foams and emulsions with particles of biological origin. Curr. Opin. Colloid and Interface Sci. 19, 490–500. doi:10.1016/j.cocis.2014.07.003
Langevin, D. (2015). Bubble coalescence in pure liquids and in surfactant solutions. Curr. Opin. Colloid and Interface Sci. 20, 92–97. doi:10.1016/j.cocis.2015.03.005
Langevin, D. (2000). Influence of interfacial rheology on foam and emulsion properties. Adv. Colloid Interface Sci. 88, 209–222. doi:10.1016/S0001-8686(00)00045-2
Lencina, M. M. S., Fernández Miconi, E., Fernández Leyes, M. D., Domínguez, C., Cuenca, E., and Ritacco, H. A. (2018). Effect of surfactant concentration on the responsiveness of a thermoresponsive copolymer/surfactant mixture with potential application on “Smart” foams formulations. J. Colloid Interface Sci. 512, 455–465. doi:10.1016/j.jcis.2017.10.090
Liang, H.-N., and Tang, C. (2014). Pea protein exhibits a novel Pickering stabilization for oil-in-water emulsions at pH 3.0. LWT - Food Sci. Technol. 58, 463–469. doi:10.1016/j.lwt.2014.03.023
Liu, F., and Tang, C.-H. (2014). Emulsifying properties of soy protein nanoparticles: Influence of the protein concentration and/or emulsification process. J. Agric. Food Chem. 62, 2644–2654. doi:10.1021/jf405348k
Liu, F., and Tang, C.-H. (2016). Soy glycinin as food-grade Pickering stabilizers: Part. I. Structural characteristics, emulsifying properties and adsorption/arrangement at interface. Food Hydrocoll. 60, 606–619. doi:10.1016/j.foodhyd.2015.04.025
Liu, F., and Tang, C. H. (2013). Soy protein nanoparticle aggregates as pickering stabilizers for oil-in-water emulsions. J. Agric. Food Chem. 61, 8888–8898. doi:10.1021/jf401859y
Liu, H., Wang, C., Zou, S., Wei, Z., and Tong, Z. (2012). Simple, reversible emulsion system switched by pH on the basis of chitosan without any hydrophobic modification. Langmuir 28, 11017–11024. doi:10.1021/la3021113
Ma, L., Zou, L., McClements, D. J., and Liu, W. (2020). One-step preparation of high internal phase emulsions using natural edible Pickering stabilizers: Gliadin nanoparticles/gum Arabic. Food Hydrocoll. 100, 105381. doi:10.1016/j.foodhyd.2019.105381
Maestro, A., and Guzmán, E. (2019). Colloids at fluid interfaces. Processes 7, 942. doi:10.3390/pr7120942
Maestro, A., Guzmán, E., Ortega, F., and Rubio, R. G. (2014). Contact angle of micro- and nanoparticles at fluid interfaces. Curr. Opin. Colloid Interface Sci. 19, 355–367. doi:10.1016/j.cocis.2014.04.008
Maestro, A., Santini, E., and Guzmán, E. (2018). Physico-chemical foundations of particle-laden fluid interfaces. Eur. Phys. J. E 41, 97. doi:10.1140/epje/i2018-11708-6
Maestro, A., Santini, E., Zabiegaj, D., Llamas, S., Ravera, F., Liggieri, L., et al. (2015). Particle and particle-surfactant mixtures at fluid interfaces: Assembly, morphology, and rheological description. Adv. Condens. Matter Phys. 2015, 1–17. doi:10.1155/2015/917516
Maestro, A. (2019). Tailoring the interfacial assembly of colloidal particles by engineering the mechanical properties of the interface. Curr. Opin. Colloid and Interface Sci. 39, 232–250. doi:10.1016/j.cocis.2019.02.013
Manikantan, H., and Squires, T. (2020). Surfactant dynamics: Hidden variables controlling fluid flows. J. Fluid Mech. 892, P1. doi:10.1017/jfm.2020.170
Martinelli, H., Domínguez, C., Leyes, M. F., Moya, S., and Ritacco, H. (2021). A pH-responsive foam formulated with PAA/gemini 12-2-12 complexes. Colloids Interfaces 5, 37. doi:10.3390/colloids5030037
Martínez-Pedrero, F. (2020). Static and dynamic behavior of magnetic particles at fluid interfaces. Adv. Colloid Interface Sci. 284, 102233. doi:10.1016/j.cis.2020.102233
Mendoza, A. J., Guzmán, E., Martínez-Pedrero, F., Ritacco, H., Rubio, R. G., Ortega, F., et al. (2014). Particle laden fluid interfaces: Dynamics and interfacial rheology. Adv. Colloid Interface Sci. 206, 303–319. doi:10.1016/j.cis.2013.10.010
Murray, B. S., and Ettelaie, R. (2004). Foam stability: Proteins and nanoparticles. Curr. Opin. Colloid and Interface Sci. 9, 314–320. doi:10.1016/j.cocis.2004.09.004
Murray, B. S., and Phisarnchananan, N. (2016). Whey protein microgel particles as stabilizers of waxy corn starch + locust bean gum water-in-water emulsions. Food Hydrocoll. 56, 161–169. doi:10.1016/j.foodhyd.2015.11.032
Narsimhan, G., and Xiang, N. (2018). Role of proteins on formation, drainage, and stability of liquid food foams. Annu. Rev. Food Sci. Technol. 9, 45–63. doi:10.1146/annurev-food-030216-030009
Ning, F., Ge, Z., Qiu, L., Wang, X., Luo, L., Xiong, H., et al. (2020). Double-induced se-enriched peanut protein nanoparticles preparation, characterization and stabilized food-grade pickering emulsions. Food Hydrocoll. 99, 105308. doi:10.1016/j.foodhyd.2019.105308
Patel, A. (2020). Functional and engineered colloids from edible materials for emerging applications in designing the food of the future. Adv. Funct. Mater. 30, 1806809. doi:10.1002/adfm.201806809
Peng, D., Jin, W., Li, J., Xiong, W., Pei, Y., Wang, Y., et al. (2017). Adsorption and distribution of edible gliadin nanoparticles at the air/water interface. J. Agric. Food Chem. 65, 2454–2460. doi:10.1021/acs.jafc.6b05757
Peng, D., Jin, W., Tang, C., Lu, Y., Wang, W., Li, J., et al. (2018). Foaming and surface properties of gliadin nanoparticles: Influence of pH and heating temperature. Food Hydrocoll. 77, 107–116. doi:10.1016/j.foodhyd.2017.09.026
Peng, D., Jin, W., Zhou, P., Ren, C., and Li, B. (2020). Foaming and surface rheological behaviors of gliadin particles: Effect of solvent and concentration of gliadin stock solution. Food Hydrocoll. 106, 105868. doi:10.1016/j.foodhyd.2020.105868
Postel, C., Abillon, O., and Desbat, B. (2003). Structure and denaturation of adsorbed lysozyme at the air–water interface. J. Colloid Interface Sci. 266, 74–81. doi:10.1016/S0021-9797(03)00571-X
Qin, X.-S., Luo, Z.-G., Peng, X.-C., Lu, X.-X., and Zou, Y.-X. (2018). Fabrication and characterization of quinoa protein nanoparticle-stabilized food-grade pickering emulsions with ultrasound treatment: Effect of ionic strength on the freeze–thaw stability. J. Agric. Food Chem. 66, 8363–8370. doi:10.1021/acs.jafc.8b02407
Ren, Z., Chen, Z., ZhangLin, Y. X., and Li, B. (2019). Novel food-grade Pickering emulsions stabilized by tea water-insoluble protein nanoparticles from tea residues. Food Hydrocoll. 96, 322–330. doi:10.1016/j.foodhyd.2019.05.015
Ritacco, H. A. (2020). Complexity and self-organized criticality in liquid foams. A short review. Adv. Colloid Interface Sci. 285, 102282. doi:10.1016/j.cis.2020.102282
Ritacco, H. A. (2022). Polyelectrolyte/surfactant mixtures: A pathway to smart foams. ACS Omega 7, 36117–36136. doi:10.1021/acsomega.2c05739
Rutkevičius, M., Allred, S., Velev, O. D., and Velikov, K. (2018). Stabilization of oil continuous emulsions with colloidal particles from water-insoluble plant proteins. Food Hydrocoll. 82, 89–95. doi:10.1016/j.foodhyd.2018.04.004
Sagis, L. M. C., and Yang, J. (2022). Protein-stabilized interfaces in multiphase food: Comparing structure-function relations of plant-based and animal-based proteins. Curr. Opin. Food Sci. 43, 53–60. doi:10.1016/j.cofs.2021.11.003
Sagis, L., and Scholten, E. (2014). Complex interfaces in food: Structure and mechanical properties. Trends Food Sci. Technol. 37, 59–71. doi:10.1016/j.tifs.2014.02.009
Saint-Jalmes, A. (2006). Physical chemistry in foam drainage and coarsening. Soft Matter 2, 836–849. doi:10.1039/B606780H
Salonen, A., In, M., Emile, J., and Saint-Jalmes, A. (2010). Solutions of surfactant oligomers: A model system for tuning foam stability by the surfactant structure. Soft Matter 6, 2271–2281. doi:10.1039/B924410G
Sarkar, A., and Dickinson, E. (2020). Sustainable food-grade Pickering emulsions stabilized by plant-based particles. Curr. Opin. Colloid and Interface Sci. 49, 69–81. doi:10.1016/j.cocis.2020.04.004
Skoda, M. W. A. (2019). Recent developments in the application of X-ray and neutron reflectivity to soft-matter systems. Curr. Opin. Colloid and Interface Sci. 42, 41–54. doi:10.1016/j.cocis.2019.03.003
Sridharan, S., Meinders, M. B. J., Bitter, J. H., and Nikiforidis, C. (2020). On the emulsifying properties of self-assembled pea protein particles. Langmuir 36, 12221–12229. doi:10.1021/acs.langmuir.0c01955
Sun, G., Zhao, Q., Liu, S., Li, B., and Li, Y. (2019). Complex of raw chitin nanofibers and zein colloid particles as stabilizer for producing stable pickering emulsions. Food Hydrocoll. 97, 105178. doi:10.1016/j.foodhyd.2019.105178
Tcholakova, S., Denkov, N. D., and Lips, A. (2008). Comparison of solid particles, globular proteins and surfactants as emulsifiers. Phys. Chem. Chem. Phys. 10, 1608–1627. doi:10.1039/B715933C
Thewissen, B. G., Celus, I., Brijs, K., and Delcour, J. A. (2011). Foaming properties of wheat gliadin. J. Agric. Food Chem. 59, 1370–1375. doi:10.1021/jf103473d
Vella, D., Metcalfe, P. D., and Whitakker, R. J. (2006). Equilibrium conditions for the floating of multiple interfacial objects. J. Fluid Mech. 549, 215–224. doi:10.1017/S0022112005008013
Viçosa, A., Letourneau, J.-J., Espitalier, F., and Inês Ré, M. (2012). An innovative antisolvent precipitation process as a promising technique to prepare ultrafine rifampicin particles. J. Cryst. Growth 342, 80–87. doi:10.1016/j.jcrysgro.2011.09.012
Wan, Z., Yang, X., and Sagis, L. M. C. (2016a). Contribution of long fibrils and peptides to surface and foaming behavior of soy protein fibril system. Langmuir 32, 8092–8101. doi:10.1021/acs.langmuir.6b01511
Wan, Z., Yang, X., and Sagis, L. M. C. (2016b). Nonlinear surface dilatational rheology and foaming behavior of protein and protein fibrillar aggregates in the presence of natural surfactant. Langmuir 32, 3679–3690. doi:10.1021/acs.langmuir.6b00446
Wang, C., Wu, J., Wang, C., Mu, C., Ngai, T., and Lin, W. (2022). Advances in Pickering emulsions stabilized by protein particles: Toward particle fabrication, interaction and arrangement. Food Res. Int. 157, 111380. doi:10.1016/j.foodres.2022.111380
Wege, H. A., Kim, S., Paunov, V. N., Zhong, Q., and Velev, O. D. (2008). Long-term stabilization of foams and emulsions with in-situ formed microparticles from hydrophobic cellulose. Langmuir 24, 9245–9253. doi:10.1021/la801634j
Wouters, A. G. B., and Delcour, J. A. (2019). Cereal protein-based nanoparticles as agents stabilizing air–water and oil–water interfaces in food systems. Curr. Opin. Food Sci. 25, 19–27. doi:10.1016/j.cofs.2019.02.002
Wouters, A. G. B., Joye, I. J., and Delcour, J. A. (2020). Understanding the air-water interfacial behavior of suspensions of wheat gliadin nanoparticles. Food Hydrocoll. 102, 105638. doi:10.1016/j.foodhyd.2019.105638
Wouters, A. G. B., Schaefer, S., Joye, I. J., and Delcour, J. A. (2019). Relating the structural, air-water interfacial and foaming properties of wheat (Triticum aestivum L.) gliadin and maize (Zea mays L.) zein based nanoparticle suspensions. Colloids Surfaces A Physicochem. Eng. Aspects 567, 249–259. doi:10.1016/j.colsurfa.2019.01.071
Wu, J., Shi, M., Li, W., Zhao, L., Wang, Z., Yan, X., et al. (2015). Pickering emulsions stabilized by whey protein nanoparticles prepared by thermal cross-linking. Colloids Surfaces B Biointerfaces 127, 96–104. doi:10.1016/j.colsurfb.2015.01.029
Xiao, J., Wang, X., Perez Gonzalez, A. J., and Huang, Q. (2016). Kafirin nanoparticles-stabilized Pickering emulsions: Microstructure and rheological behavior. Food Hydrocoll. 54, 30–39. doi:10.1016/j.foodhyd.2015.09.008
Yan, X., Ma, C., Cui, F., McClements, D. J., Liu, X., and Liu, F. (2020). Protein-stabilized Pickering emulsions: Formation, stability, properties, and applications in foods. Trends Food Sci. Technol. 103, 293–303. doi:10.1016/j.tifs.2020.07.005
Yang, J., Thielen, I., Berton-Carabin, C. C., van der Linden, E., and Sagis, L. M. C. (2020). Nonlinear interfacial rheology and atomic force microscopy of air-water interfaces stabilized by whey protein beads and their constituents. Food Hydrocoll. 101, 105466. doi:10.1016/j.foodhyd.2019.105466
Yano, Y. F., Uruga, T., Tanida, H., Toyokawa, H., Terada, Y., Takagaki, M., et al. (2009). Driving force behind adsorption-induced protein unfolding: A time-resolved X-ray reflectivity study on lysozyme adsorbed at an air/water interface. Langmuir 25, 32–35. doi:10.1021/la803235x
Zanini, M., and Isa, L. (2016). Particle contact angles at fluid interfaces: Pushing the boundary beyond hard uniform spherical colloids. J. Phys. Condens. Matter 28, 313002. doi:10.1088/0953-8984/28/31/313002
Zhang, X., Zhang, Z., Liang, H., Li, J., Wen, L., Geng, F., et al. (2021b). Influence of solvent polarity of ethonal/water binary solvent on the structural, emulsifying, interfacial rheology properties of gliadin nanoparticles. J. Mol. Liq. 344, 117976. doi:10.1016/j.molliq.2021.117976
Zhang, X., Zuo, Z., Ma, W., Yu, P., Li, T., and Wang, L. (2021a). Assemble behavior of ultrasound-induced quinoa protein nanoparticles and their roles on rheological properties and stability of high internal phase emulsions. Food Hydrocoll. 117, 106748. doi:10.1016/j.foodhyd.2021.106748
Zhu, Q., Lu, H., Zhu, J., Zhang, M., and Yin, L. (2019). Development and characterization of pickering emulsion stabilized by zein/corn fiber gum (CFG) complex colloidal particles. Food Hydrocoll. 91, 204–213. doi:10.1016/j.foodhyd.2019.01.029
Zhu, X.-F., Chen, X., McClements, D. J., Zou, L., and Liu, W. (2018b). pH-ion- and temperature-dependent emulsion gels: Fabricated by addition of whey protein to gliadin-nanoparticle coated lipid droplets. Food Hydrocoll. 77, 870–878. doi:10.1016/j.foodhyd.2017.11.032
Zhu, X.-F., Zhang, N., Lin, W.-F., and Tang, C.-H. (2017). Freeze-thaw stability of pickering emulsions stabilized by soy and whey protein particles. Food Hydrocoll. 69, 173–184. doi:10.1016/j.foodhyd.2017.02.001
Zhu, X.-F., Zheng, J., Liu, F., Qiu, C.-Y., Lin, W.-F., and Tang, C.-H. (2018a). Freeze-thaw stability of Pickering emulsions stabilized by soy protein nanoparticles. Influence of ionic strength before or after emulsification. Food Hydrocoll. 74, 37–45. doi:10.1016/j.foodhyd.2017.07.017
Keywords: emulsions, fluid interfaces, foams, interfacial rheology, particles, proteins
Citation: Fameau A-L, Guzmán E, Ritacco HA and Saint-Jalmes A (2023) Interfacial properties of protein particles at fluid/fluid interfaces and relationship with the stability of foams and emulsions. Front. Soft. Matter 3:1016061. doi: 10.3389/frsfm.2023.1016061
Received: 10 August 2022; Accepted: 10 March 2023;
Published: 23 March 2023.
Edited by:
Francisco Monroy, Complutense University of Madrid, SpainReviewed by:
Todd Squires, University of California, Santa Barbara, United StatesHarishankar Manikantan, University of California, Davis, United States
Carlos M. Corvalan, Purdue University, United States
Copyright © 2023 Fameau, Guzmán, Ritacco and Saint-Jalmes. This is an open-access article distributed under the terms of the Creative Commons Attribution License (CC BY). The use, distribution or reproduction in other forums is permitted, provided the original author(s) and the copyright owner(s) are credited and that the original publication in this journal is cited, in accordance with accepted academic practice. No use, distribution or reproduction is permitted which does not comply with these terms.
*Correspondence: Anne-Laure Fameau, YW5uZS1sYXVyZS5mYW1lYXVAaW5yYWUuZnI=; Eduardo Guzmán, ZWR1YXJkb2dzQHF1aW0udWNtLmVz; Hernán Alejandro Ritacco, aGVybmFuLnJpdGFjY29AdW5zLmVkdS5hcg==; Arnaud Saint-Jalmes, YXJuYXVkLnNhaW50LWphbG1lc0B1bml2LXJlbm5lczEuZnI=