- 1Fakultät für Chemie, Physikalische und Biophysikalische Chemie, Universität Bielefeld, Bielefeld, Germany
- 2Fakultät Chemie, Institut für Physikalische Chemie, Universität Stuttgart, Stuttgart, Germany
- 3Laboratoire Charles Coulomb (L2C), Université de Montpellier, CNRS, Montpellier, France
Recent studies of self-assembly in binary systems of bio-surfactants, either of microbial origin or saponins extracted from plants, are reviewed. Saponins in water reported in the first section include aescin, glycyrrhizin, and quillaja saponins, while rhamnolipids are discussed in the second section on microbial surfactants. Studies of surface activities are a natural starting point of the characterization of surfactants, but here we focus mainly on physico-chemical and structural properties of self-assembled bulk structures in solution, often characterized by scattering techniques. When quantitative modelling is performed, self-assembly parameters like aggregation numbers, head group areas, and resulting shapes can be followed as a function of physical-chemical parameters like concentration, composition, temperature, or pH. Morphologies include micelles and their structural evolution with addition of other bio- or synthetic surfactants, co-surfactants, proteins or phospholipids.
1 Introduction
Biosurfactants are structurally divers amphiphiles stemming from natural resources. The mode of production by plants or microorganisms is reflected by the classification into plant saponins and microbial biosurfactants, produced by bacteria or yeast. Within saponins, molecules are usually named after the plant used to produce them, although a chemical variety of biosurfactants may correspond to the same name. Microbial biosurfactants are mainly glycolipids including trehalolipids, sophorolipids, and in particular rhamnolipids, which serve us here as paradigm: produced by the bacterium Pseudomonas aeruginosa, they possess outstanding surface-activity. In many cases biosurfactants offer significant benefits compared to synthetic petrochemistry-based surfactants, especially in the context of sustainability: bio-surfactants are made using mild production conditions (bio-reactors or extraction from plants), they often have lower toxicity and enhanced biocompatibility, and saponins possess pharmacological activity, e.g., antiviral [Bailly & Vergoten (2020)] or antimicrobial, justifying the growing interest in their large-scale production [Kitamoto et al. (2002)]. Biosurfactant properties are linked to their molecular hydrophilic/hydrophobic structure—which is often less clearly separated as compared to synthetic molecules –, resulting in self-assembly in solution with sometimes surprising properties. One may think of the spontaneous formation of so-called ISCOMs (immune-stimulating complexes containing Quillaja saponins used as adjuvants to vaccines) [Morein et al. (1984); Sjolander et al. (1998); Pedersen et al. (2012)], or of gelation induced by network formation of elongated micellar structures [Saha et al. (2015)]. It is the purpose of this article to review important recent progress on aggregate formation in (mixed) biosurfactant systems. Mixtures of biosurfactants with lipids have been investigated due to their use in vesicle-based drug delivery (saponins) and also to test their haemolytic activity which is necessary for their application in consumer care products. Moreover, in such products synthetic surfactants are also present and their interaction with biosurfactants might be relevant.
Saponins occur in plants and are part of the defense against fungi and bacteria. Historically, the name was due to foaming and emulsifying properties of, e.g. Quillaja bark saponin used for washing. Nowadays, saponins are still mainly extracted from different plants (e.g. horse chestnuts, licorice, soapbark tree quillaja saponaria, digitonin from common foxglove, oleandrin, gypsogenin from saponaria officinalis, etc.). Saponins are of growing interest due to their pharmacological activity. They exhibit typical surfactant properties, like a critical micellar concentration (CMC) [Geisler et al. (2019a); Dargel et al. (2019)], or interfacial activity [Wojciechowski (2013); Góral & Wojciechowski (2020)]. All saponins have in common that the hydrophobic part is formed by a triterpenic or steroidic structure with attached sugar moieties, enabling this group of molecules to stabilize emulsions and to solubilize bulky hydrophobic molecules.
The second group of bio-surfactants is produced by microorganisms. The most important low-molecular weight representatives of this group are rhamnolipids, sophorolipids and threhalolipids, but also surfactin [Lewinska et al. (2022)], and mannosylerythritol lipids [Kim et al. (2002)], which are of interest in the context of bioemulsifiers, or lipopetides and phospholipids. Their hydrophobic tail is usually composed by one or several, possibly branched fatty acid chains, while their hydrophilic head group can contain a phosphate group (phospholipids), a peptide loop (lipopeptides), or a sugar moiety (glycolipids), e.g. based on the monosaccharide (“ose”) rhamnose, or disaccharides like sophorose, trehalose, or maltose. The latter may also be dirhamnose, as opposed to monorhamnose [Chen et al. (2010b)]. A summary of these compounds can be found in [Rodrigues (2015); Jahan et al. (2020)]. Other types of biosurfactants, such as the well-studied model sugar surfactants (alkyl polyglucosides, abbreviated CiGj), which can be obtained by esterification of bio-sourced sugar head groups and fatty acids, or microbial polymeric biosurfactants (high-molecular weight) exist, but are outside the scope of this mini-review.
The shape of—pure or mixed—biosurfactant aggregates formed in water is controlled by the underlying chemistry: the spontaneous curvature of the hydrophilic-hydrophobic amphiphilic film reflects the interactions between each moiety. The bending energy to be paid for deviations from the spontaneous curvature defines the available shapes, as illustrated by some glycolipids which possess large hydrophilic sugar head groups, favoring high curvatures, and thus globular micelles. As the curvature is reduced, rod- and disk-like structures may be found. At higher concentrations, where competition for water molecules may induce a reduction of the head group, such aggregates may form liquid crystalline phases. In this short review we are mainly addressing the formation of micellar aggregates of saponins and microbial surfactants in water, respectively. Saponins are exemplarily discussed in section 2 for aescin, glycyrrhizin, and quillaja saponins, while we have focused on rhamno- and sophorolipids in the third section. Scattering techniques are ubiquitous to the field, and the interested reader is referred to ref. [Zemb & Lindner (1988)].
2 Binary systems of saponins in water: Aescin, glycyrrhizin, and quillaja saponins
Saponins are plant-derived biosurfactants. In applications, they serve as emulsifier in food foams, or in pharmaceutical or cosmetic formulations. Prominent representatives of this group like aescin (sometimes also named escin), glycyrrhizin, and quillaja saponins (for chemical structures see Figures 1A–D), as well as their mixtures with synthetic surfactants are discussed below.
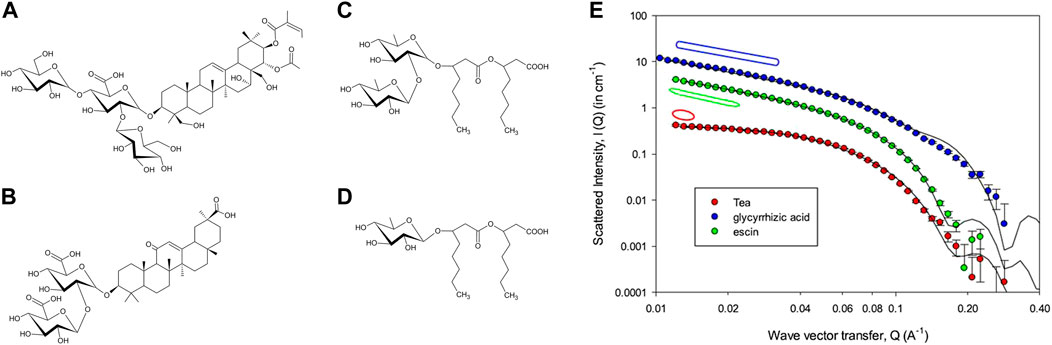
FIGURE 1. Chemical formulas of (A) aescin, (B) glycyrrhizin, (C) a di-rhamnolipid, and (D) a mono-rhamnolipide. (E) SANS intensities for tea, aescin (shifted vertically ×8), and glycyrrhizic acid saponin (shifted ×16), at 5 mM with 0.1 M NaCl. The solid lines are model fits, the corresponding geometries are sketched in the same color, and parameters are reported in the original article. Figure reprinted from ref [Tucker et al. (2021b)] with permission from Elsevier.
Aescin
Aescin is one of the most studied saponins. It stems from the horse chestnut tree (aesculus hippocastanum) and is available commercially in a rather pure form. Its predominant component has the typical triterpenoid hydrophobic moiety with several polar groups attached one-sided, and a large hydrophilic head group of three saccharide groups carrying a single carboxyl group. The latter confers a possibly slightly ionic character to the molecule, at high pH.
In pure water, this saponin behaves like an amphiphile. The determination of the CMC at fixed physiological pH 7.4, between 10°C and 50°C, has been performed recently, using the autofluorescence of aescin [Dargel et al. (2019)]. Moreover, modeling of SAXS intensities shows that with increasing temperature, and only slightly affected by concentration, aescin micelles evolve from cylindrical (typical length 10 nm, radius 2 nm) into shorter, ellipsoidal shapes.
Aescin has a strong biological activity and interacts with 1,2-dimyristoyl-sn-glycero-3-phosphocholine (DMPC) phospholipid membranes decomposing them to bicelles (or lipid nano-disks, with bilayer structure), by stabilizing edges [Geisler et al. (2019b)]. Similarly, when conventional surfactants are added to lipid bilayers, the formation of bicelles was found, in which the surfactant molecules form the highly curved edge [Dürr et al. (2013)]. Small-angle scattering and TEM with a combined analysis by indirect Fourier transformation [Fritz & Glatter (2006)] and simultaneous modelling of SAXS and SANS showed that the nanodisk diameter decreases from ca. 30 nm to 12 nm with increasing aescin content. Moreover, depending on the amount of aescin, the disks reassemble upon temperature increase from the gel to the fluid phase of the phospholipid. Later, the same group showed that aggregate morphology can evolve from nano-disks into larger ribbons and finally stacks of sheets [Geisler et al. (2021)].
Very recently, Tucker et al. have studied the influence of oxyethylene chain length of model non-ionic surfactants (n-dodecyl polyglycol ether, C12E3 to C12E8) on the interactions with aescin by SANS [Tucker et al. (2022b)]. The limited compatibility between the oxyethylene and sugar headgroup controls the self-assembly, which encompasses elongated aescin micelles and intermediate planar (vesicular or locally lamellar) morphologies. It is striking that the transition to the latter does not depend much on the curvature of the added non-ionic surfactant. In this context, we note that the spontaneous formation of very small micro (or “nano”)-vesicles has been evidenced with synthetic surfactants by scattering before [Oberdisse et al. (1996); Oberdisse & Porte (1997); Gradzielski et al. (1997); Gradzielski (2003); Grillo et al. (2008); Bressel et al. (2010)]. Although such strong curvatures were believed to be impossible for bilayers, they have now shown to exist also in biosurfactant systems.
The same group in the United Kingdom has also investigated the properties of mixtures of glycyrrhizic acid, aescin, and tea saponin [Tucker et al. (2021b)]. This is an interesting series due to the increase in saccharide groups from two to four. As shown in Figure 1E, tea saponins form globular structures due to the largest headgroup, and this is also the preferred morphology in any mixture including tea saponins. Blends without tea saponin have been found to keep the elongated structure as seen by the low-q slope, with a subtle interplay between glycyrrhizin and aescin curvatures.
It is noteworthy that in all these studies small-angle scattering with detailed quantitative modelling (namely core-shell) is needed to characterize each population of aggregates, and their coexistence. In the last study, e.g., the absence of the structure factor gives access to the form factor, permitting a trustworthy determination of average aggregation numbers of about 80 for tea saponins. This is similar to that observed in most globular micelles formed by conventional surfactants, whereas elongated structures reach 300. Increasing the head group size leads to smaller spherical micelles, thus following the well-known packing criteria of Israelachivili and Ninham [Israelachvili et al. (1976)]. However, in detail, the packing requirements are more sophisticated due to the much more complex structure of saponins and biosurfactants [Tucker et al. (2021b)].
Glycyrrhizin
Glycyrrhizin (or glycyrrhizic acid, known for its sweet taste) is extracted from licorice roots (Glycyrrhiza glabra). This saponin is based on a triterpenoid tail, and the hydrophilic part is a disaccharide carrying carboxyl groups. As opposed to simpler molecules like dodecyl maltoside, which forms globular micelles in agreement with its conical packing, the large hydrophobic group favors a lower spontaneous curvature. It is now established that glycyrrhizin forms elongated structures as seen from the q−1-power law behavior of the SAXS data (and also directly in TEM or AFM) [Saha et al. (2015); Matsuoka et al. (2016)], which presumably connect to form gels in water after a heating-cooling cycle. Matsuoka et al. have followed the CMC as a function of pH by fluorescence and light scattering. Tucker et al. have studied the aggregate morphology by SANS [Tucker et al. (2021a)]. In pure D2O, detailed modelling provides the lateral dimensions (typically 3-4 nm core, plus a 2 nm thick hydrated shell) of elongated (length ca. 27 nm) aggregates, with aggregation numbers of 150. Different salts then induce moderate micellar growth, presumably due to reduction in head group interaction. Note that there has been a debate about the existence of a CMC, and on the size of glycyrrhizin micelles; different values have been reported under different experimental conditions, in particular pH. Due to the presence of carboxyl groups on some saponins, it is important to take pH modifications into account, or use buffers. The carboxyl groups (especially the one at the C20 position) are protonated up to a pH value of 6. Below this value glycyrrhizin is amphiphilic with CMC [Matsuoka et al. (2016)] and self-assembly [Dargel et al. (2021)].
Quillaja saponins
This group of saponins can be extracted from Quillaja Saponaria, the soap bark tree. The hydrophobic part is again a steroid or triterpenoid. The sugar head group illustrates the great chemical variability of saponins. Its saccharides may be glucose, galactose, Orczykxylose, rhamnose, arabinose, and glucuronic acid, substituted at up to three positions in the glycone (mono-, bis, and tri-desmosides, respectively). More than sixty different saponins have been identified in Quillaja Saponaria by mass spectroscopy [Nord & Kenne (1999)]. The surface activity of Quillaja saponins is suspected to be involved in their cytotoxic and haemolytic properties, and it has been investigated by Wojciechowski et al. by surface tension and neutron reflectometry [Wojciechowski et al. (2014a)]. In a second article, dynamic light scattering showed that aggregation properties depend on both the origin of the molecules and on time [Kezwon & Wojciechowski (2014)]. Initially small globular micelles (5–7 nm) evolve into objects of hundreds of nanometers. The same surface techniques have been applied to characterize the penetration of phospholipids [Wojciechowski et al. (2014b)]. Very recently, a comparative study of different saponins has also been conducted, including their effect on model lipid monolayers [Wojciechowski et al. (2021)].
The incorporation of different molecules into Quillaja micelles has attracted quite some interest, including mixtures with conventional surfactants [Tucker et al. (2022a)]. Tippel et al. have studied the uptake of lutein (a carotenoid), resulting in micellar growth [Tippel et al. (2016)]. In a structural investigation including SANS, Peixotoa et al. have investigated mixtures of mate saponin, and in particular benchmarked them against Quillaja saponins in terms of uptake of two model drugs, carbamazepine and flurbiprofen [Peixoto et al. (2011)]. Kezwon and Wojciechowski have studied interactions of Quillaja saponin with food-related proteins (hen egg lysozyme, bovine β-lactoglobulin and β-casein) by surface rheology (Kezwon & Wojciechowski (2014)). The authors explain the differences observed for saponins of different origins by different acidities, modifying electrostatic interactions with proteins. The biological activity of saponins may be due to their interaction with cholesterol embedded in phospholipid membranes. Adding cholesterol to Quillaja saponin has been shown using SAXS and SANS to trigger a transition from globular to spherohelical micelles, followed by a reentrant phase transition [Liu et al. (2013)].
3 Binary systems of microbial surfactants in water: rhamnolipids
Microbial biosurfactants possess intrinsically the same advantages as saponins. Their most important macroscopic physico-chemical manifestation of amphiphilicity is the strong surface activity leading to foaming. e.g., very low concentrations of surfactin (a cyclic lipopeptide produced, among others, by Bacillus subtilis) or rhamnolipids lower the surface tension of the water/air interface by more than a factor of two [Rodrigues (2015)]. Rhamnolipids favor the formation of bacterial biofilms, they possess a CMC, and form micelles above it. All (bio-) surfactants have the capacity to penetrate and possibly disrupt membranes or phospholipid systems. Although this may be vital for any pharmacological activity, we focus on the bulk behavior through the formation of biosurfactant aggregates in water.
In a continuous effort to understand the phase behavior of blends of anionic surfactants as used in detergency applications with biosurfactants, the Oxford group has published a series of articles starting about a decade ago, in collaboration with I. Grillo (ILL), an expert of determination of aggregate morphology. Chen et al. have studied the adsorption and bulk properties of mono- and dirhamnolipids and their blends [Chen et al. (2010b)], of their mixtures with the anionic surfactant sodium dodecyl 6-benzene sulfonate (LAS) [Chen et al. (2010a)], and of different types of sophorolipids (lactonic or acidic, varying hydrophobicity) [Penfold et al. (2011)]. Using neutron reflectometry and small-angle scattering with ca. 90% deuterated biosurfactant provided a detailed view of aggregate morphology. While the phase diagrams of these systems are usually complex, the underlying tendencies can be rationalized by simple rules: sugar head groups are bulky and mostly non-ionic, lowering electrostatic charge density, spontaneous curvature, and introducing steric repulsion, thus preventing the complex formation. In a follow-up, the tolerance of surfactant mixtures including rhamnolipids and LAS to precipitation induced by calcium has been investigated [Chen et al. (2013)]. Whereas synthetic anionic surfactants employed in detergent formulations are highly sensitive to hard water, with possibly disastrous effects on their function, the addition of rhamnolipids, which are mostly non-ionic, somewhat increase the tolerance towards multivalent ions. Depending on the surfactant system, ions of high valency promote micellar growth and sometimes morphological transition to bilayer phases—demonstrating the influence of electrostatics on spontaneous curvature. Incorporating the non-ionic surfactants, and in particular biosurfactants, increases minimal ion concentration for precipitation. In this study of morphologies before precipitation, the influence of the calcium ions is characterized by the change in aggregation number and surface micellar charge. Pure rhamnolipids are found to show practically superimposed scattering curves with and without Ca2+ (2 mM), indicating that CaCl2 has little impact on the self-assembly. In contrast, the anionic surfactant and its mixtures with rhamnolipids show a complex response to Ca2+ addition with a tendency to form planar phases.
More recently, the same group has again performed small-angle scattering on blends of rhamnolipids with the non-ionic model surfactant C12E8 (n-dodecyl octaethylene glycol ether), LAS, and yet another ionic one, sodium dioxyethylene monododecyl sulfate [Liley et al. (2017)]. The influence of the rhamnolipids is to favor the formation of bilayer (vesicular) phases at high rhamnolipid and LAS content. Coexistence of the two morphologies is observed as superimposed contributions in SAXS, with increased low-q scattering including first- and second-order Bragg peaks characteristic of lamellar phases, presumably multilayer vesicles or onions. In other phase regions, small interacting micelles with internal composition equal to the nominal one are formed.
In very recent work, Palos Pacheco et al. [Pacheco et al. (2021)] have tuned the self-assembly of nature-inspired (mono-) rhamnolipids via the shape of the hydrophobic moiety. These compounds rely on a remarkable effort in organic synthesis, circumventing thus the usual lack of pure compounds. The effect of asymmetry between the two lipid tails has been highlighted by surface tension measurements, dynamic light scattering, and fluorescence quenching. The combination of a long (C14) tail with a second shorter one (C6 to C14) gives access to a range of molecular packings in-between conical or cylindrical shapes, and thus more globular or more planar aggregates. Surface tension shows a strong dependence on chain asymmetry. DLS results are difficult to interpret due to superposition of different populations; a q-resolved scattering technique (SAXS/SANS) might have given further insight into local structures. The location of the pyrene fluorescence probe, finally, provides molecular understanding of the observed changes: the more asymmetric the tails, the less polar the core and the higher the free volume, allowing accommodation of the probe molecule. The same group has also used numerical approaches to understand the phase behavior of rhamnolipids better [Eismin et al. (2017)].
The impact of mono- and dirhamnolipids on giant unilamellar phospholipid vesicles has been studied by the group of Winter [Herzog et al. (2020); Herzog et al. (2021)]. The formation of daughter vesicles upon addition of either RhaRhaC10C10, RhaC10C10 (corresponding to the di- and monorhamnolipids as illustrated for RhaRhaC8C8 and RhaC8C8 in Figures 1C,D), and the sugar-free precursor C10C10 has been imaged as shown in Figure 2 and is interpreted in terms of the different spontaneous curvatures.
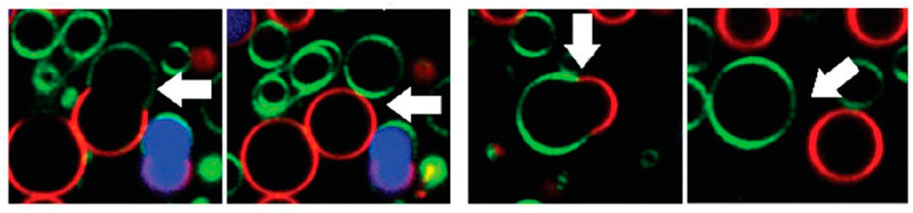
FIGURE 2. Confocal fluorescence microscopy of giant unilamellar vesicles showing budding and fission of domains after addition of RhaC10C10. reproduced from reference [Herzog et al. (2021)] with permission from the Royal Society of Chemistry.
The group of J.S. Pedersen has studied the interaction of biosurfactants with proteins in water, via the formation of mixed self-assembled aggregates. The authors underline that changes in enzymatic activity caused by biosurfactants may occur, depending on the supramolecular structure of the latter. Binding to proteins may be favored or hindered by electrostatics, and may be cooperative or individual, thereby affecting the interactions. A light scattering and SAXS-study of rhamnolipid aggregates has been performed, followed by a comparison of synthetic sodium dodecyl sulfate (SDS) to rhamnolipid aggregates incorporating α-lactalbumin and myoglobin [Mortensen et al. (2017)]. Pure rhamnolipids form small prolate micelles containing some eleven molecules. The failure of modelling the scattering intensity by simple addition of individual contributions, and a detailed analysis of the scattering data by indirect Fourier transform shows that the mixed SDS/rhamnolipid aggregates harbor only a single protein, with little change of the overall shape and size of the micelle for lactalbumin, whereas myoglobin induces pairwise micellar association. This contrasts with SDS, which in pure solution has a larger core. Upon addition of α-lactalbumin, the micellar shape changes from oblate into prolate, with up to three proteins.
4 Summary and outlook
We have reviewed recent progress in the determination of biosurfactant self-assembly in water. The precise measurement of physico-chemical properties of biosurfactants in water is still in its infancy, in spite of the growing importance of biosurfactants for a sustainable economy. This is presumably due to the large chemical variety—making the understanding of mixtures even more relevant. Clearly, the most quantitative techniques are small-angle and dynamic light scattering, because they enable precise measurements of biosurfactant aggregate morphologies.
The surfactant properties of biosurfactants and conventional surfactants are comparable. For example, the cmc of rhamnolipids (RhaRhaC10C10, RhaC10C10) is close to the one of the standard surfactant LAS, of about 10−1 mmol/l−1. Similarly, the minimum surface tension value is comparable (30 mNm−1) [Chen et al. (2010a)], increasing as expected with the number of rhamnose units due to the increasing bulkiness of the biosurfactants [Chen et al. (2010a)]. A major difference of especially saponins with conventional surfactant is the stronger pH-dependence of the surfactant properties, sometimes up to absence of micellization.
We anticipate biosurfactants to be of growing importance in the progressive replacement and improvement of synthetic surfactant mixtures by sustainable and biodegradable molecules in many applications, from pharmacology to detergency. Besides being used as “simple” surfactant, saponins also offer a large potential in pharmacy, and especially the antiviral activity of glycyrrhizin remains to be fully exploited e.g. in treatment of SARS-Cov2.
Author contributions
JO, TS, and TH have jointly planned and written the article.
Acknowledgments
We acknowledge support for the publication costs by the Open Access Publication Fund of Bielefeld University and the Deutsche Forschungsgemeinschaft (DFG).
Conflict of interest
The authors declare that the research was conducted in the absence of any commercial or financial relationships that could be construed as a potential conflict of interest.
Publisher’s note
All claims expressed in this article are solely those of the authors and do not necessarily represent those of their affiliated organizations, or those of the publisher, the editors and the reviewers. Any product that may be evaluated in this article, or claim that may be made by its manufacturer, is not guaranteed or endorsed by the publisher.
References
Bailly, C., and Vergoten, G. (2020). Glycyrrhizin: An alternative drug for the treatment of COVID-19 infection and the associated respiratory syndrome? Pharmacol. Ther. 214, 107618. doi:10.1016/j.pharmthera.2020.107618
Bressel, K., Muthig, M., Prévost, S., Grillo, I., and Gradzielski, M. (2010). Mesodynamics: Watching vesicle formation in situ by small-angle neutron scattering. Colloid Polym. Sci. 288, 827–840. doi:10.1007/s00396-010-2212-z
Chen, M., Dong, C., Penfold, J., Thomas, R. K., Smyth, T. J. P., Perfumo, A., et al. (2013). Influence of calcium ions on rhamnolipid and rhamnolipid/anionic surfactant adsorption and self-assembly. Langmuir 29 (12), 3912–3923. doi:10.1021/la400432v
Chen, M. L., Penfold, J., Thomas, R. K., Smyth, T. J. P., Perfumo, A., Marchant, R., et al. (2010a). Mixing behavior of the biosurfactant, rhamnolipid, with a conventional anionic surfactant, sodium dodecyl benzene sulfonate. Langmuir 26 (23), 17958–17968. doi:10.1021/la1031834
Chen, M. L., Penfold, J., Thomas, R. K., Smyth, T. J. P., Perfumo, A., Marchant, R., et al. (2010b). Solution self-assembly and adsorption at the air-water interface of the monorhamnose and dirhamnose rhamnolipids and their mixtures. Langmuir 26 (23), 18281–18292. doi:10.1021/la1031812
Dargel, C., Geisler, R., Hannappel, Y., Kemker, I., Sewald, N., and Hellweg, T. (2019). Self-assembly of the bio-surfactant aescin in solution: A small-angle x-ray scattering and fluorescence study. Colloids Interfaces 3 (2), 47. doi:10.3390/colloids3020047
Dargel, C., Gräbitz-Bräuer, F., Geisler, R., Fandrich, P., Hannappel, Y., Porcar, L., et al. (2021). Stable DOPG/glycyrrhizin vesicles with a wide range of mixing ratios: Structure and stability as seen by scattering experiments and cryo-TEM. Molecules 26 (16), 4959. doi:10.3390/molecules26164959
Dürr, U. H., Soong, R., and Ramamoorthy, A. (2013). When detergent meets bilayer: Birth and coming of age of lipid bicelles. Prog. Nucl. Magnetic Reson. Spectrosc. 69, 1–22. doi:10.1016/j.pnmrs.2013.01.001
Eismin, R. J., Munusamy, E., Kegel, L. L., Hogan, D. E., Maier, R. M., Schwartz, S. D., et al. (2017). Evolution of aggregate structure in solutions of anionic monorhamnolipids: Experimental and computational results. Langmuir 33 (30), 7412–7424. doi:10.1021/acs.langmuir.7b00078
Fritz, G., and Glatter, O. (2006). Structure and interaction in dense colloidal systems: Evaluation of scattering data by the generalized indirect fourier transformation method. J. Phys. Condens. Matter 18 (36), S2403–S2419. doi:10.1088/0953-8984/18/36/s14
Geisler, R., Pedersen, M. C., Hannappel, Y., Schweins, R., Prévost, S., Dattani, R., et al. (2019b). Aescin-induced conversion of gel-phase lipid membranes into bicelle-like lipid nanoparticles. Langmuir 35 (49), 16244–16255. doi:10.1021/acs.langmuir.9b02077
Geisler, R., Dargel, C., and Hellweg, T. (2019a). The biosurfactant β-aescin: A review on the physico-chemical properties and its interaction with lipid model membranes and Langmuir monolayers. Molecules 25 (1), 117. doi:10.3390/molecules25010117
Geisler, R., Pedersen, M. C., Preisig, N., Hannappel, Y., Prévost, S., Dattani, R., et al. (2021). Aescin a natural soap for the formation of lipid nanodiscs with tunable size. Soft Matter 17 (7), 1888–1900. doi:10.1039/d0sm02043e
Góral, I., and Wojciechowski, K. (2020). Surface activity and foaming properties of saponin-rich plants extracts. Adv. Colloid Interface Sci. 279, 102145. doi:10.1016/j.cis.2020.102145
Gradzielski, M., Langevin, D., Sottmann, T., and Strey, R. (1997). Droplet microemulsions at the emulsification boundary: The influence of the surfactant structure on the elastic constants of the amphiphillic film. J. Chem. Phys. 106 (19), 8232–8238. doi:10.1063/1.473888
Gradzielski, M. (2003). Vesicles and vesicle gels - structure and dynamics of formation. J. Phys. Condens. Matter 15 (19), R655–R697. doi:10.1088/0953-8984/15/19/202
Grillo, I., Penfold, J., Tucker, I., and Cousin, F. (2008). Spontaneous formation of nanovesicles in mixtures of nonionic and dialkyl chain cationic surfactants studied by surface tension and SANS. Langmuir 25 (7), 3932–3943. doi:10.1021/la802435h
Herzog, M., Tiso, T., Blank, L. M., and Winter, R. (2020). Interaction of rhamnolipids with model biomembranes of varying complexity. Biochimica Biophysica Acta (BBA) - Biomembr. 1862 (11), 183431. doi:10.1016/j.bbamem.2020.183431
Herzog, M., Li, L., Blesken, C. C., Welsing, G., Tiso, T., Blank, L. M., et al. (2021). Impact of the number of rhamnose moieties of rhamnolipids on the structure, lateral organization and morphology of model biomembranes. Soft Matter 17 (11), 3191–3206. doi:10.1039/d0sm01934h
Israelachvili, J., Mitchell, D. J., and Ninham, B. W. (1976). Theory of self-assembly of hydrocarbon amphiphiles into micelles and bilayers. J. Chem. Soc. Faraday Trans 72, 1525–1568. doi:10.1039/f29767201525
Jahan, R., Bodratti, A. M., Tsianou, M., and Alexandridis, P. (2020). Biosurfactants, natural alternatives to synthetic surfactants: Physicochemical properties and applications. Adv. Colloid Interface Sci. 275, 102061. doi:10.1016/j.cis.2019.102061
Kezwon, A., and Wojciechowski, K. (2014). Interaction of quillaja bark saponins with food-relevant proteins. Adv. Colloid Interface Sci. 209, 185–195. doi:10.1016/j.cis.2014.04.005
Kim, H.-S., Jeon, J.-W., Kim, S.-B., Oh, H.-M., Kwon, T.-J., and Yoon, B.-D. (2002). Surface and physico-chemical properties of a glycolipid biosurfactant, mannosylerythritol lipid, from candida Antarctica. Biotechnol. Lett. 24 (19), 1637–1641. doi:10.1023/a:1020309816545
Kitamoto, D., Isoda, H., and Nakahara, T. (2002). Functions and potential applications of glycolipid biosurfactants from energy-saving materials to gene delivery carriers. J. Biosci. Bioeng. 94 (3), 187–201. doi:10.1016/s1389-1723(02)80149-9
Lewinska, A., Domza-Kedzia, M., Wójtowicz, K., and Bazylinska, U. (2022). Surfactin-stabilized poly(D, L-lactide) nanoparticles for potential skin application. Colloids Surfaces A Physicochem. Eng. Aspects 648, 129216. doi:10.1016/j.colsurfa.2022.129216
Liley, J., Penfold, J., Thomas, R., Tucker, I., Petkov, J., Stevenson, P., et al. (2017). Self-assembly in dilute mixtures of non-ionic and anionic surfactants and rhamnolipd biosurfactants. J. Colloid Interface Sci. 487, 493–503. doi:10.1016/j.jcis.2016.10.071
Liu, J., Harms, M., Garamus, V. M., and Mã¼ller-Goymann, C. C. (2013). Reentrant structural phase transition in amphiphilic self-assembly. Soft Matter 9 (28), 6371. doi:10.1039/c3sm51239h
Matsuoka, K., Miyajima, R., Ishida, Y., Karasawa, S., and Yoshimura, T. (2016). Aggregate formation of glycyrrhizic acid. Colloids Surfaces A Physicochem. Eng. Aspects 500, 112–117. doi:10.1016/j.colsurfa.2016.04.032
Morein, B., Sundquist, B., Höglund, S., Dalsgaard, K., and Osterhaus, A. (1984). ISCOM, a novel structure for antigenic presentation of membrane proteins from enveloped viruses. Nature 308 (5958), 457–460. doi:10.1038/308457a0
Mortensen, H. G., Madsen, J. K., Andersen, K. K., Vosegaard, T., Deen, G. R., Otzen, D. E., et al. (2017). Myoglobin and α-lactalbumin form smaller complexes with the biosurfactant rhamnolipid than with SDS. Biophysical J. 113 (12), 2621–2633. doi:10.1016/j.bpj.2017.10.024
Nord, L. I., and Kenne, L. (1999). Separation and structural analysis of saponins in a bark extract from quillaja saponaria molina. Carbohydr. Res. 320 (1-2), 70–81. doi:10.1016/s0008-6215(99)00134-2
Oberdisse, J., Couve, C., Appell, J., Berret, J. F., Ligoure, C., and Porte, G. (1996). Vesicles and onions from charged surfactant Bilayers: A neutron scattering study. Langmuir 12, 1212–1218. doi:10.1021/la950313l
Oberdisse, J., and Porte, G. (1997). Size of microvesicles from charged surfactant bilayers: Neutron scattering data compared to an electrostatic model. Phys. Rev. E 56 (2), 1965–1975. doi:10.1103/physreve.56.1965
Pacheco, R. P., Kegel, L. L., and Pemberton, J. E. (2021). Interfacial and solution aggregation behavior of a series of bioinspired rhamnolipid congeners rha-C14-cx (x = 6, 8, 10, 12, 14). J. Phys. Chem. B 125 (49), 13585–13596. doi:10.1021/acs.jpcb.1c09435
Pedersen, J. S., Oliveira, C. L., Hã¼bschmann, H. B., Arleth, L., Manniche, S., Kirkby, N., et al. (2012). Structure of immune stimulating complex matrices and immune stimulating complexes in suspension determined by small-angle x-ray scattering. Biophysical J. 102 (10), 2372–2380. doi:10.1016/j.bpj.2012.03.071
Peixoto, M. P. G., Treter, J., de Resende, P. E., da Silveira, N. P., Ortega, G. G., Lawrence, M. J., et al. (2011). Wormlike micellar aggregates of saponins from ilex paraguariensis a. St, hil. (mate): A characterisation by cryo-tem, rheology, light scattering and small-angle neutron scattering. J. Pharm. Sci. 100 (2), 536–546. doi:10.1002/jps.22283
Penfold, J., Chen, M., Thomas, R. K., Dong, C., Smyth, T. J. P., Perfumo, A., et al. (2011). Solution self-assembly of the sophorolipid biosurfactant and its mixture with anionic surfactant sodium dodecyl benzene sulfonate. Langmuir 27 (14), 8867–8877. doi:10.1021/la201661y
Rodrigues, L. R. (2015). Microbial surfactants: Fundamentals and applicability in the formulation of nano-sized drug delivery vectors. J. Colloid Interface Sci. 449, 304–316. doi:10.1016/j.jcis.2015.01.022
Saha, A., Adamcik, J., Bolisetty, S., Handschin, S., and Mezzenga, R. (2015). Fibrillar networks of glycyrrhizic acid for hybrid nanomaterials with catalytic features. Angew. Chem. Int. Ed. Engl. 54 (18), 5498–5502. doi:10.1002/ange.201411875
Sjolander, A., Cox, J. C., and Barr, I. G. (1998). Iscoms: An adjuvant with multiple functions. J. Leukoc. Biol. 64, 713–723. doi:10.1002/jlb.64.6.713
Tippel, J., Lehmann, M., von Klitzing, R., and Drusch, S. (2016). Interfacial properties of quillaja saponins and its use for micellisation of lutein esters. Food Chem. 212, 35–42. doi:10.1016/j.foodchem.2016.05.130
Tucker, I., Burley, A., Petkova, R., Hosking, S., Penfold, J., Thomas, R., et al. (2021a). Adsorption and self-assembly properties of the plant based biosurfactant, glycyrrhizic acid. J. Colloid Interface Sci. 598, 444–454. doi:10.1016/j.jcis.2021.03.101
Tucker, I., Burley, A., Petkova, R., Hosking, S., Webster, J., Li, P., et al. (2021b). Self-assembly in saponin mixtures: Escin/tea, tea/glycyrrhizic acid, and escin/glycyrrhizic acid mixtures. Colloids Surfaces A Physicochem. Eng. Aspects 629, 127420. doi:10.1016/j.colsurfa.2021.127420
Tucker, I., Burley, A., Petkova, R., Hosking, S., Webster, J. P., Li, P., et al. (2022a). Self-assembly of quillaja saponin mixtures with different conventional synthetic surfactants. Colloids Surfaces A Physicochem. Eng. Aspects 633, 127854. doi:10.1016/j.colsurfa.2021.127854
Tucker, I., Burley, A., Petkova, R., Hosking, S., Webster, J. R. P., Li, P. X., et al. (2022b). Self-assembly in escin-nonionic surfactant mixtures: From micelles to vesicles. J. Colloid Interface Sci. 626, 305–313. doi:10.1016/j.jcis.2022.06.122
T. Zemb, and P. Lindner (Editors) (1988). “Neutron, X-rays and light,” Scattering methods applied to Soft condensed matter (North Holland, Netherland: North-Holland Delta Series).
Wojciechowski, K., Jurek, I., Góral, I., Campana, M., Geue, T., and Gutberlet, T. (2021). Surface-active extracts from plants rich in saponins - effect on lipid mono- and bilayers. Surfaces Interfaces 27, 101486. doi:10.1016/j.surfin.2021.101486
Wojciechowski, K., Orczyk, M., Marcinkowski, K., Kobiela, T., Trapp, M., Gutberlet, T., et al. (2014a). Effect of hydration of sugar groups on adsorption of quillaja bark saponin at air/water and Si/water interfaces. Colloids Surfaces B Biointerfaces 117, 60–67. doi:10.1016/j.colsurfb.2014.02.010
Wojciechowski, K., Orczyk, M., Gutberlet, T., Trapp, M., Marcinkowski, K., Kobiela, T., et al. (2014b). Unusual penetration of phospholipid mono- and bilayers by quillaja bark saponin biosurfactant. Biochimica Biophysica Acta - Biomembr. 1838 (7), 1931–1940. doi:10.1016/j.bbamem.2014.04.008
Keywords: rhamnolipid, saponin, glycyrrhizin, aescin, self-assembly, micelles, scattering techniques
Citation: Hellweg T, Sottmann T and Oberdisse J (2023) Recent advances in biosurfactant-based association colloids—Self-assembly in water. Front. Soft. Matter 2:1081877. doi: 10.3389/frsfm.2022.1081877
Received: 27 October 2022; Accepted: 07 December 2022;
Published: 04 January 2023.
Edited by:
Anne-laure Fameau, Institut National de recherche pour l’agriculture, l’alimentation et l’environnement (INRAE), FranceReviewed by:
Alesya Mikhailovskaya, UMR7182 Institut de Chimie et des Matériaux Paris-Est (ICMPE), FranceLeonardo Chiappisi, Institut Laue-Langevin, France
Copyright © 2023 Hellweg, Sottmann and Oberdisse. This is an open-access article distributed under the terms of the Creative Commons Attribution License (CC BY). The use, distribution or reproduction in other forums is permitted, provided the original author(s) and the copyright owner(s) are credited and that the original publication in this journal is cited, in accordance with accepted academic practice. No use, distribution or reproduction is permitted which does not comply with these terms.
*Correspondence: Thomas Hellweg, dGhvbWFzLmhlbGx3ZWdAdW5pLWJpZWxlZmVsZC5kZQ==; Julian Oberdisse, anVsaWFuLm9iZXJkaXNzZUB1bW9udHBlbGxpZXIuZnI=