- 1Université Lille, CNRS, INRAE, Centrale Lille, UMR 8207, UMET - Unité Matériaux et Transformations, Lille, France
- 2Biopolymères Interactions Assemblages, INRAE, Nantes, France
- 3Laboratoire Léon-Brillouin, Université Paris Saclay, CEA-CNRS UMR12, Gif-sur-Yvette, France
We describe in this study the aggregation behaviour of β-casein micelles from milk in bulk aqueous solution as function of both temperature and calcium content, and its influence on the foaming properties, in order to test if the different aggregation states of β-casein makes possible the design of proteins-based thermoresponsive foams. First, we characterized the morphology of the self-assembled β-casein molecules in solution by coupling turbidity measurements, Dynamic Light Scattering and Small Angle Neutron Scattering. They self-organize into individual micelles at low temperature (20°C) whatever the calcium content, and transit in a reversible way into aggregates of micelles at large temperature in presence of calcium, with a threshold transition that depend both on temperature and calcium content. The micelles aggregation is driven by the calcium through association with serine phosphate groups localized on the hydrophilic part of the β-casein. In the micelles regime, we demonstrated that the addition of calcium tunes the aggregation number of unimers per micelle in the same way than an increase of temperature through a change of hydrophobic interactions. The hydrophilic chains of the corona are however in a good solvent and interact through excluded volume interactions, even when the β-casein micelles aggregates themselves. The internal molecular structure of the micelles is thus not modified by calcium bridges, which explains the complete reversibility of the aggregation process over temperature cycling. Second, we studied the foam stability versus time as a function of the temperature and calcium content by measuring the kinetic evolution of both the foam volume and the liquid fraction. Foams produced by solutions containing only β-casein micelles were stable in terms of foam volume on a timescale of 1 h at 20°C but drained quickly. However, foams become unstable when the temperature was increased above 20°C. In presence of calcium, the aggregation of β-casein micelles inside the foam liquid channels enabled to increase foam stability at larger temperature by acting as a cork, which slows down the drainage. The increase of foam stability by such aggregates is however not sufficient on the long term to allow the design of thermoresponsive foams.
1 Introduction
Proteins are well-known surface-active components. They contribute to the formation of foams and to their stabilization for food products such as ice cream and whipped cream (Dickinson, 2020). Foams are thermodynamically unstable systems that tend to separate into their two original phases (gas and liquid) over time due to three concurrent main mechanisms that are closely linked to each other: liquid drainage, bubble coalescence and disproportionation of individual bubbles (Rio et al., 2014). Their formation necessitates the use of low molecular weight surfactants or proteins to reduce the air-water interfacial tension. Proteins are less active than low molecular weight surfactants but they form a viscoelastic interfacial film that enables efficiently the foam to resist destabilization (Saint-Jalmes et al., 2005). Foams are called stable when all of the destabilization mechanisms are drastically slowed down (Rio et al., 2014). The control of lifetime of liquid foams between stable to unstable state is possible by adjusting the rate of these destabilization mechanisms (Fameau et al., 2015a). When such a control is possible, responsive foams are obtained (Fameau and Fujii, 2020). These responsive foams can be obtained by three main routes: a stimulus which can tunes the physico-chemical conditions of the aqueous phase, (pH or ionic strength), a change of temperature or the application of an external field (light and magnetic field) (Fameau and Fujii, 2020). One strategy to tune the foam stability is to target large modifications of the drainage rate by playing on the reversible modification of the apparent viscosity of the bulk aqueous phase inside the foam liquid channels between the bubbles. Until now, thermoresponsive foams based on this approach were obtained respectively from: surfactant self-assemblies, crystalline particles, synthetically designed peptides, and polymers (Dexter et al., 2006; Fameau et al., 2011; Fameau et al., 2015b; Zhang et al., 2015; Weißenborn and Braunschweig, 2019). Proteins are well known to give foams with different stabilities as a function of the medium conditions: pH, ionic strength, and temperature (Murray, 2011). Indeed, the surface activity of the proteins depends on the exposure of the hydrophobic parts to the aqueous phase controlling the surface activity, which varies upon their level of folding, and depends on these three parameters (Murray, 2011). Moreover, proteins in bulk solution may show different states of aggregation as a function of such medium conditions, from well dispersed to large aggregates with various morphologies, which changes in turn the rheological properties such as the viscosity (Amin et al., 2014). In protein foams, the presence of aggregated structures in the foam liquid channels may then prevent foam destabilization by reducing drainage, acting either as a cork or by reducing disproportionation rate due to larger thickness of foam lamellae (Saint-Jalmes et al., 2005; Chen et al., 2017). However, up to date, there is no example in the literature describing in situ switchable foam stability for food proteins (Fameau and Fujii, 2020). Moreover, there is still no example in the literature of temperature tunable foams with an unstable foam at low temperature and a stable foam at high temperature to the best of our knowledge (Fameau and Fujii, 2020).
Herein, we probe if responsive foams can be achieved with milk proteins. We chose to work with β-casein which is one of the four different caseins existing in milk (Atamer et al., 2017). β-casein is a linear amphiphilic protein, consisting of a hydrophilic N-terminal domain and a hydrophobic C-terminal domain and considered as a natural diblock polymer (O’Connell et al., 2003). β-casein has a high surface activity and interesting bulk properties in terms of self-assembled structures and rheological properties depending on the medium conditions (O’Connell et al., 2003; McCarthy et al., 2014). At low concentrations and temperatures (0–4°C), β-casein exists as a unimer. However, β-casein self-assemble into a core-corona structure with a hydrophobic core and a hydrophilic surface, commonly called micelles, at concentrations larger than the critical micellar concentration (CMC) and for temperatures above 20°C (O’Connell et al., 2003; Dauphas et al., 2005). The hydrophilic N-terminal region is highly charged and forms a soft (mobile) layer covering the surface of the hard core consisting of the hydrophobic amino acids (Kajiwara et al., 1988). The formation of β-casein micelles is linked to hydrophobic interactions (Aschi et al., 2009). Adding calcium or increasing ionic strength can induce micelles aggregation due to change in micelles charge and thus of electrostatic repulsions (Leclerc and Calmettes, 1997a; O’Connell et al., 2003; Dauphas et al., 2005; Aschi et al., 2009). The equilibrium between β-casein micelles and aggregates structures is strongly influenced by β-casein concentration, temperature and calcium content (Dauphas et al., 2005). The self-association of β-casein and the stability of aggregates are mainly attributed to a delicate balance of hydrophobic and electrostatic interactions (Leclerc and Calmettes, 1997a; O’Connell et al., 2003; Aschi et al., 2009). These changes of aggregation states have already been used to tune oil-in-water emulsion properties (Dauphas et al., 2008). In the presence of calcium, aggregated β-casein in the aqueous phase led to the floculation of the emulsion, but by decreasing the temperatures leading to the recovery of β-casein micelles, emulsions deflocculated (Dauphas et al., 2008). This floculation was totally reversible by tuning temperature and calcium content (Dauphas et al., 2008). The floculation process comes from the binding of the calcium ions to the negatively charged phosphoserine residues of the protein, thus reducing their charge and in doing so reducing repulsions between molecules (Atkinson et al., 1995). This leads to molecular aggregation, bridging between neighbouring emulsion droplets, and extensive flocculation (Dickinson et al., 1992). At the air/water interface, the β-casein is also sensitive to change in temperatures and calcium content leading to a more rapid interfacial gelation when β-casein is under aggregated states in bulk (Niño et al., 1999; Dauphas et al., 2005; Vessely et al., 2005). Based on these promising results from literature, β-casein appears to be a good candidate to design a protein-based foam with increased stability with temperature and a thermoreversible behavior. Indeed, increasing temperature may reduce at the same time the drainage upon formation of aggregates in bulk and the other destabilization mechanisms by the formation of a gel interfacial layer at high temperature. Moreover, the transition between well-dispersed individual β-casein micelles and aggregates is reversible with temperature, which is a necessary prerequisite for obtaining thermoresponsive foams (Dauphas et al., 2005; Li et al., 2019).
The objective of this study is to determine the combined effects of temperature and calcium content on the stability of aqueous foams produced from β-casein. First, we systematically study the evolution of the morphology β-casein self-assembled structure in bulk solution as a function of two parameters: temperature and calcium content. By coupling turbidity, DLS and SANS measurements, we complete former studies on the reversible changes of micelles and aggregates structure in bulk (gyration radius, size of hydrophilic and hydrophobic core) to propose an exhaustive description of β-casein behavior (Leclerc and Calmettes, 1997a; Leclerc and Calmettes, 1997b; Aschi et al., 2007; Aschi et al., 2009). Second, we study the temporal stability of β-casein-based foams produced by bubbling gas. We establish the link between the foam stability and the different aggregated β-casein states determined in bulk.
2 Materials and methods
2.1 Materials and samples preparation
β-casein was extracted from bovine milk by rennet coagulation and solubilization at 4°C, by Lactalis industry (Laval, France). Its purity (>98%) was checked by RPHPLC (Dauphas et al., 2005). The β-casein used in this study was the same than previously described by Dauphas et al. and coming from the same supplier and batch (Dauphas et al., 2005). The β-casein was dissolved and stirred in 80 mM of NaCl, at pH = 6.8 at 5 g.L−1 during a minimum of 4 h at 4°C. This solution was studied in a range of temperatures between 20°C and 55°C. The calcium chloride (CaCl2; >99%) was purchased from Sigma Aldrich. CaCl2 was added directly into the β-casein solution. Two concentrations of CaCl2 was studied: 5 and 10 mM. After calcium addition, the solution was stirred during 30 min before measurements.
2.2 Characterization of beta-casein in solution
2.2.1 Turbidity measurements
The turbidity of β-casein solutions, with and without CaCl2, was expressed as the optical density at 600 nm using a Cary 100 Bio UV-visible Spectrophotometer (Varian Inc., Palo Alto, United States), which was equipped with a temperature control system. Samples were held at 20°C before their transfer to the spectrophotometer where they were heated in the chamber at different temperatures: 20, 25, 30, 35, 40, 45 and 50 °C. Before each measurement, the samples were held for an equilibration time of 20 min at each temperature. Measurements were taken in triplicate and each experiment was repeated three times.
2.2.2 Hydrodynamic radius measurements in bulk by dynamic light scattering
A Malvern Zetasizer APS (Malvern Instruments Ltd., Malvern Worcestershire, United Kingdom) was used to determine the distribution of the hydrodynamic radius of objects in solution (isolated protein or aggregates) by measuring scattered light intensity with an angle of 90°. The DLS setup was equipped with a temperature controlled cell holder. The β-casein solutions were held at 4°C and then were filtered with 0.45 µm PTFE porosity syringe filter before transfer to the thermo-equilibrated zetasizer at 4 °C. Temperature was increased stepwise at different temperatures: 20, 25, 30, 35, 40, 45 and 50 °C. Before each measurement, the samples were held 20 min as equilibration time at each temperature. Data collection and analyses were performed using the Nano software (version 7.01; Malvern Instruments; CONTIN algorithm), with a water refractive index value of 1.33. The temperature-dependence of the solvent viscosity was taken into account for the size calculations. All the samples were analyzed four times.
2.2.3 Self-assembly properties of beta-casein studied by Small Angle Neutron Scattering
SANS experiments were performed on the PACE diffractometer at the Laboratoire Léon Brillouin (Saclay, France). Three configurations of neutrons wavelength and sample-detector distances with a significant overlap were chosen to access a Q-range spanning from 5.10–3 to 3.10–1 Å−1 (respectively 5 Å at 1 m, 5 Å at 4.7 m and 13 Å at 4.7 m). The temperature was controlled within ±0.2°C by a thermalized circulator bath. The neutron wavelength was set to the desired value with a mechanical velocity selector (Δλ/λ ≈ 0.1). Standard procedures were applied by the PASINET software available at the beamline to correct the averaged spectra for empty quartz cell, background noise, and detector efficiency contributions in order to obtain scattering in cm−1. The incoherent scattering from solvent was then subtracted afterwards. In order to ensure a good contrast between the β-casein and the solvent, the solutions were prepared in deuterated water (D2O) as solvent. We took into account that pD = pH + 0.4 to prepare the samples. In the literature, it is considered that micelles of β-casein are spherical objects made up of a core of radius Rcore surrounded by a corona with external radius Rcorona with different but constant scattering length densities (Aschi et al., 2009). Thus, the core-corona model was used to fit the data by using the SASview software (Author Anonymous, 2022). Moreover, for very low Q-values, the Guinier approximation was used to determine the radius of gyration (Rg) of the scattering objects (Guinier et al., 1955).
2.3 Characterization of foaming properties
Foams were produced by bubbling gas into the protein solution using a Foamscan apparatus (I.T.Concept, France). Foams were generated in a glass cylindrical column with a 21 mm diameter and 400 mm height by bubbling nitrogen gas through a porous glass disc with a 10–16 µm pore size and a diameter of 3 mm. A fixed volume of 12 ml of the aqueous solution was used to produce the foam. The gas flow rate was fixed at 45 ml min−1. The gas flow stopped automatically when the final foam volume of 35 ml was reached. A camera recorded pictures of foams every 100 s. The foam formation and stability was monitored by image analysis. To study the effect of the temperature on the foam stability, protein solutions were introduced inside the glass column and left for 30 min to equilibrate to the required temperature before introducing the gas to produce the foam. The temperatures studied were: 20 °C, 30 °C, and 40 °C. The liquid volume in the foam was assessed by mean of conductivity measurements taken at different heights along the foam column.
2.4 Statistical analysis
The preparation of solutions and subsequent analyses for turbidity, DLS and foaming experiments were carried out in independent triplicate trials. The results were compared by one-way analysis of variance and Tukey’s test to analyze statistical differences (p < 0.05). The analysis was done using SAS V9.4 software (SAS Institute, Gary, NC, United States).
3 Results and discussion
3.1 Effect of temperature and CaCl2 content on the turbidity and hydrodynamic radius of β-casein solution
In this study, we chose to work with a concentration of β-casein at 5 g.L−1 at pH = 6.8 with 80 mM of NaCl in order to be markedly higher than the CMC (around 2 g.L−1 at 20°C, data not shown) in order to ensure that micelles exist in solutions for all probed conditions at 20°C and that sufficient amount of aggregates are formed at high temperatures to change the viscosity (Dauphas et al., 2005; Portnaya et al., 2006; Li et al., 2019). Temperature and calcium content are two parameters known to act in cooperative way to induce thermal aggregation and the formation of large aggregates in specific conditions (Dauphas et al., 2005). Moreover, at 5 g.L−1, it has been shown that the system behaves almost as a non-interacting system. Indeed, Leclerc et al. demonstrated by 2nd Virial measurements that such concentration corresponds to the threshold where the solutions of β-casein transit from attractions between unimers (single isolated β-casein molecule) at low concentration towards repulsive interactions between micelles at higher concentrations (Leclerc and Calmettes, 1997b). We have tested calcium concentration from 2 mM to 30 mM on β-casein aggregation (data not shown). At 2 mM calcium, aggregation occurred at temperature around 50 °C, which was a too high temperature to study foaming properties, since foam stability is known to decrease by increasing temperatures (Rio et al., 2014). At 20 and 30 mM, the β-casein aggregates were too large and precipitated quickly. From these preliminary results, we studied two parameters: temperature (from 20°C to 50°C) and three calcium content (0, 5 and 10 mM). First, we characterized the phase diagram as a function of these two parameters by coupling turbidity measurements and hydrodynamic radius measurements by DLS (Figure 1 and Figure 2).
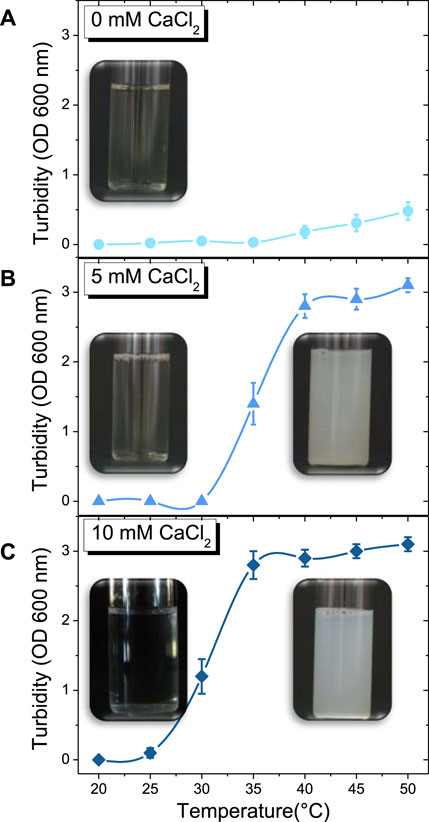
FIGURE 1. Temperature-dependent evolution in turbidity of β-casein solution along with the corresponding macroscopic pictures at: (A) 0 mM of CaCl2 (●), (B) 5 mM of CaCl2 (▲), (C) 10 mM of CaCl2 (♦).
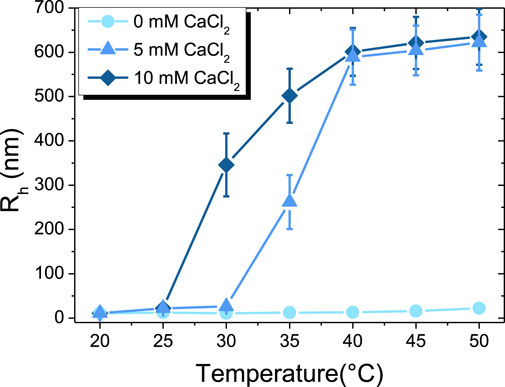
FIGURE 2. Temperature-dependent evolution of hydrodynamic radius (Rh) of β-casein at: 0 mM of CaCl2 (●), 5 mM of CaCl2 (▲), 10 mM of CaCl2 (♦).
The visual appearance of solutions of β-casein as a function of temperature for the three-calcium content are presented in Figure 1. Without calcium, the solution remains limpid by naked eyes. For 5 mM of CaCl2, the limpid solution turns into a turbid white solution around 35°C. For 10 mM of CaCl2, the transition between limpid solution and turbid white solution is around 30°C. In order to determine precisely the temperature of formation of objects in solution that have a sufficiently larger size to scatter light, we performed turbidity measurements (Figure 1). In absence of calcium, no significant difference in turbidity is detected before 35 °C (Figure 1A). When the temperature is increased above 35°C, the turbidity slightly increases, which is difficult to observe by naked eyes (Figure 1A). The addition of 5 mM of CaCl2 causes a noticeable increase of turbidity at 30 °C (Figure 1B), and at a reduced temperature of 25°C for 10 mM of CaCl2 (Figure 1C).
The hydrodynamical radius (Rh) of micelles or aggregates in the different regimes of temperature and calcium content has been determined by DLS, as shown in Figure 2. At 20 °C, Rh is around 11 ± 2 nm without calcium, which corresponds to the micelle state. When increasing temperature from 20 °C to 50 °C, the hydrodynamical radius continuously increases up to a mean value of 20 ± 5 nm. In the presence of 5 mM of CaCl2, Rh has the same value of 11 ± 2 nm at 20 °C as for the calcium-free case, then progressively increases with temperature to reach a mean value of 25 ± 8 nm up to 30 °C. From such threshold temperature, Rh drastically increases to typical radius of few hundreds of nm, revealing the onset of formation of large aggregates of β-casein micelles by calcium bridges (Dauphas et al., 2005). At 50 °C, the maximum value of around 620 ± 70 nm detected by the DLS apparatus is reached. At 10 mM of CaCl2, the same behavior is observed as for the 5 mM case, but the onset of aggregates formation is shifted down to a lower temperature of ∼25 °C.
3.2 Effect of temperature and CaCl2 content on the morphology of β-casein micelles and aggregates by SANS
The morphology of micelles and aggregated states in the different regimes of temperature and calcium content was assessed by SANS measurements. First, we checked that there was no effect of the nature of the solvent H2O versus D2O in this β-casein system (Bucciarelli et al., 2016). We measured the turbidity and the size by DLS for all β-casein solutions in D2O (see Supplementary Figures S1, S2), and showed that the transition between micellar states and aggregated states happens at around the same temperature for H2O and D2O, demonstrating that the impact of solvent deuteration was negligible for such system.
The SANS intensity profiles as a function of temperature and calcium content are presented in Figure 3. In absence of calcium, they are all similar at intermediate and large Q values (Figure 3A). At low Q, the scattered intensity increases progressively when increasing the temperature from 20 °C to 54 °C, showing an increase of size, in accordance with DLS results. These scattering profiles show all of the typical features of scattering intensity of β-casein micelles solutions, as previously observed (Leclerc and Calmettes, 1997a). This increase of size of the micelles with temperature increase is driven by a change of solvent quality and an increase of hydrophobic interactions. In presence of calcium, the scattering profiles at 20 °C for the three concentration in calcium probed (0, 5 and 10 mM) are close to each other and corresponds to those of isolated micelles in solution (Supplementary Figure S3). The presence of calcium induces a slight increase in micelles size. In the presence of 5 mM of CaCl2, we observed the same scattering profiles for 20 °C and 27 °C with a slight increase of intensity at low Q values showing a slight increase in micelles size (Figure 3B). At 32 °C, the intensity at low Q values increased drastically. Micelles are still present in solution, but a small amount of large aggregates starts to appear as well. Then at 40 °C, the scattering profile completely changes showing the presence of big aggregated β-casein micelles. In the presence of 10 mM of CaCl2, we observe that the scattering profile corresponds to the presence of micelles at 20 °C, but at 27 °C there is already an increase of intensity at low Q values showing that the micelles aggregation start to occur (Figure 3C). At 32 °C, the scattering profile is similar to the one observed at 40 °C for β-casein with 5 mM of CaCl2. Large aggregated β-casein micelles are present at 32 °C in solution for 10 mM of CaCl2. The temperatures at which we detect the presence of large aggregates by SANS corresponded to the temperatures at which we observed previously a change in turbidity and in size by DLS.
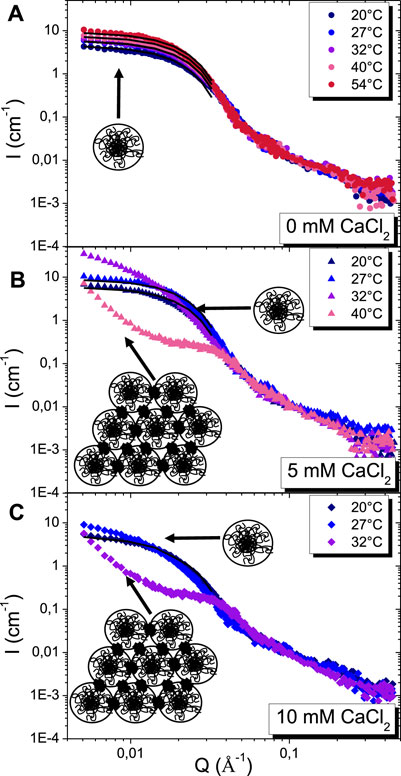
FIGURE 3. SANS intensity profiles as a function of temperature for β-casein solution at: (A) 0 mM of CaCl2 (●), (B) 5 mM of CaCl2 (▲), (C) 10 mM of CaCl2 (♦). The continuous black lines are fits with the core-corona spherical model. Schematics correspond to the presence of micelles or aggregates in solution. The calcium clusters are represented by a black spot.
In the case where only β-casein micelles are present in solution, some quantitative information can be obtained from fitting of SANS scattering curves. First, given that we chose to work at a concentration (5 g.L−1) for which the system is known to behave almost as a non-interacting system, the structure factor can be neglected and the scattered intensity can be modelled by a form factor only (Leclerc and Calmettes, 1997b). The scattered intensity shows three distinct regimes as function of Q: 1) at the lowest Q, a Guinier plateau; 2) at intermediate Q (down), the typical form factor of a spherical object with an oscillation; 3) and a Q−1.7 decay for Q > 0.075 Å-1, whatever the temperatures and calcium content, as evidenced in a Q1.7 I(Q) versus Q representation (Figure 4). This scattered intensity corresponds to those of a spherical self-assembled structure of hairy micelles with a hydrophobic core and a hydrophilic corona. The scattering intensity at large Q is dominated by those of the hydrophilic chains inside the corona, which enables to described their conformation since I(Q) decays like Q−1/ν, where ν is the Flory exponent. The hydrophilic chains of the corona are thus in a good solvent and interact through excluded volume interactions since ν = 0.588 (Figure 4). It is worth noting that such a behavior is observed in all of the conditions probed in the study, even when the β-casein micelles aggregates themselves, demonstrating that the hydrophilic chains composing the corona remain always in the same good solvent state.
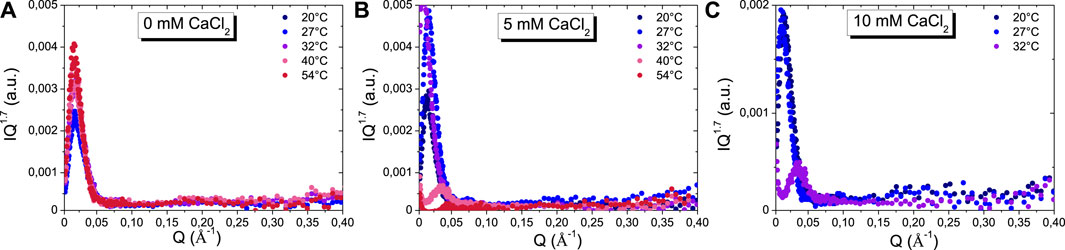
FIGURE 4. I(Q) Q1.7 versus Q plot of SANS scattering curves as a function of temperatures at: (A) 0 mM of CaCl2, (B) 5 mM of CaCl2, and (C) 10 mM of CaCl2.
Such a typical scattering curve was already obtained by Leclerc and Calmettes (Leclerc and Calmettes, 1997a) in absence of calcium and successfully fitted by a model form factor consisting of a core of constant density surrounded by a shell containing ideal random chains proposed by Pedersen and Gerstenberg (Pedersen and Gerstenberg, 1996). In order to fit the data, we have used a simpler approach, focusing only on the low Q and intermediate Q regions of the scattered intensity (Figure 3), in order to obtain quantitative parameters that can be directly compared with an interesting theroretical model developed by Aschi et al (Aschi et al., 2009) that demonstrates that the micelles formation is consistent with a model of self-association of diblock copolymers with an excluded volume parameter describing the hydrophobic sequences that depends on temperature. We determined first the evolution of the gyration radius by using Guinier approximation, as well as the radius of the hydrophobic core and of the hydrophilic corona by using a spherical core-shell model with an inner radius Rcore, and an outer shell thickness tcorona with two different scattering length densities for the core and the shell, as described previously in the literature (Supplementary Figure S4) (Aschi et al., 2009). Given the rather low value of the corona thickness of these β-casein micelles, such model assumes that the concentration profile decay within the corona from the outer core may be neglected in a first approximation (Aschi et al., 2009). In all cases, we used the same scattering length densities for Rcore and tcorona, respectively 4.46*10–6 Å−2 and 5.99*10–6 Å−2. Given that D2O has an SLD of 6.38*10–6 Å−2 and assuming that casein has an SLD of ∼2.43*10–6 Å−2, as most of proteins (Jacrot, 1976), the order of magnitude of water volume fraction content is of the order of 51% within the core and 90% within the corona.
Figure 5A shows the radius of gyration as function of temperature and calcium content (see the values in Supplementary Table S1). Without calcium, it increases from around 75 ± 1 Å at 20 °C to 86 ± 1 Å at 55°C, confirming the behavior observed with DLS. Such increase of radius of gyration is linked to an increase of the aggregation number of casein unimers with temperature (Aschi et al., 2009). The same evolution with temperature is recovered at 5 mM of calcium between 20 °C and 27 °C, before aggregation occurs. When focusing on the influence of calcium at the fixed temperature of 20 °C, the same trend as for the temperature increase in calcium-free solutions is observed with a progressive increase of the radius of gyration from 75 ± 1 Å without calcium to 86 ± 1 Å with 10 mM of calcium, revealing also an increase of the aggregation number. It demonstrates that both temperature and calcium content lead to an increase of aggregation number.
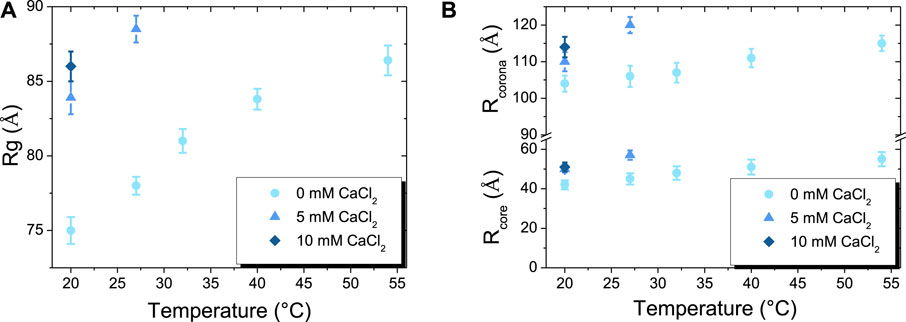
FIGURE 5. (A) Temperature-dependent changes of radius of gyration (Rg) of β-casein at: 0 mM of CaCl2 (●), 5 mM of CaCl2 (▲), 10 mM of CaCl2 (♦). (B) Temperature-dependent changes of the respective core (Rcore) and corona (Rcorona) radius of β-casein micelles at: 0 mM of CaCl2 (●), 5 mM of CaCl2 (▲), 10 mM of CaCl2 (♦).
The respective variations of the radius of Rcore and Rcorona (with Rcorona = Rcore + tcorona) with temperature and calcium content are shown Figure 5B and Supplementary Table S1. Without calcium, the Rcore increases with temperature from around 42 ± 3 Å at 20 °C to reach 55 ± 4 Å at 54°C. In the same time, Rcorona also increases with temperature but with a lower relative increase of Rcorona_54/Rcorona_20 than Rcore_54/Rcore_20, with an overall increase from 104 ± 2 Å at 20 °C to 115 ± 2 Å at 54 °C. The same behavior is observed when increasing calcium content to 5 and 10 mM of calcium at 20°C, with an increase of Rcore and Rcorona of the same order of magnitude as those obtained without calcium from 20°C to 54°C. Remarkably, the values obtained with 10 mM of calcium at 20°C (Rcore = 51 ± 2 Å and Rcorona = 114 ± 3 Å) almost perfectly match those obtained at 54°C without calcium. Such evolution of the micelles sizes with temperature has already been observed Aschi et al. (Aschi et al., 2009). Based on scaling laws arguments, they derived the aggregation number as function of temperature and established that Rcore ∼ 2.6*(Rcorona)5/8, that was consistent with their experimental data. We also tested such a scaling law and checked that it described our data accurately (see Supplementary Table S1). In the regime of physico-chemical conditions were micelles do not aggregate, we have thus demonstrated that the addition of calcium tunes the aggregation number of micelles in the same way as an increase of temperature, with the addition of 10 mM of calcium equivalent to an increase of ∼35°C.
In the regime of physico-chemical conditions where the formation of aggregates of micelles was evidenced by DLS, the scattering intensity is completely different at low and intermediate Q from those of isolated micelles, only the Q−1.7 decay at large Q being recovered (see Figures 4B,C with the scattering curves of 5 mM CaCl2 at 40 °C and 10 mM CaCl2 at 32 °C). There is a marked correlation peak at Q0 = 0.035Å−1, i.e. a distance in direct space of 2π/Q0 = 200 Å, a correlation hole below Q0, and a steep rise of the intensity at low Q when going towards low Q. The Guinier regime is thus no longer accessible within the SANS Q-range probed in our experiments, in accordance with the radius of gyration of aggregates determined at around 600 nm by DLS (Figures 3B,C). The correlation peak has already been observed numerous times and is now well described in the literature depicting casein micelles studied by SANS (De Kruif et al., 2012). It is the signature of the presence of colloidal calcium phosphate clusters. The micelles aggregation is induced through association with serine phosphate groups localized on the hydrophilic part of the β-casein. The typical distance of 200 Å between clusters associated with the correlation peak is slightly lower than twice the radius of the micelles (Rcorona lies between 105 and 120 Å in the conditions we probe, see Figure 4B). This demonstrates that the micelles are slightly interpenetrating one to each other within the aggregates.
To check the reversibility of the aggregation process, we measured the scattering intensity of a sample at 10 mM of CaCl2 over the following temperature cycle: first measurement at 20°C, a second one at 32 °C after heating above the threshold temperature of aggregates formation and then a third one after cooling the sample back down to 20 °C (Supplementary Figure S5). Both scattering curves at 20°C obtained before and after the heating step are similar, indicating that single micelles are fully recovered when aggregates break upon cooling. These two states (micelles and aggregates) are thus completely reversible by changing temperature. Therefore, the calcium bridges connecting the β-casein micelles drive the aggregation but they do not modify the internal molecular structure of the micelles, which leads to the complete reversibility of the aggregation process through temperature cycling.
3.3 Combined effect of temperature and CaCl2 content on the foaming properties
From all our measurements in bulk, it appears that solutions of β-casein micelles are suitable systems to test the possibility of producing thermoresponsive foams for which the stability can be increased by increasing temperature upon the formation of aggregates.
We first probed the foamability of solutions at different temperatures and calcium content, starting either from solution of isolated β-casein micelles or from aggregates. To this aim, we produced foams by bubbling gas in solutions in various conditions (Supplementary Figure S6). We compare the foam formation time, whose magnitude is of the order of seconds, which is necessary to reach the fixed foam targeted volume of 35 cm3 for all the samples (Supplementary Table S2). No statistical difference between all the samples are observed. The foam formation time is always around 52–55 s whatever the temperature and calcium content. There is thus no specific effect of the temperature or calcium content on the foam formation time in the experimental conditions we probed, i.e. the presence of either isolated micelles or aggregated structures do not influence the foam formation time.
Then, we followed the temporal evolution of the foam volume for two temperatures (20 °C and 40 °C) and for the three-calcium content (0 mM of CaCl2, 5 mM of CaCl2, and 10 mM of CaCl2) (Figure 6). Without calcium, a stable foam is obtained at 20 °C since its volume is around 32.5 cm3, i.e. 93% of its initial volume, 2500 s after the end of bubbling (Figure 6). By increasing the temperature, the foam stability decreases (Supplementary Figure S6). At 40 °C, the foam is much less stable than at 20°C since almost no foam remains at the end of the experiment. Given that only micelles are present for all the temperatures studied without calcium, it is likely that there is no noticeable change with respect to the drainage. Therefore, an increase of temperature lead to the collapse of the foam due to bubbles coarsening and bubbles dissolution, which are known to be enhanced upon a temperature increase (Poulichet and Garbin, 2015).
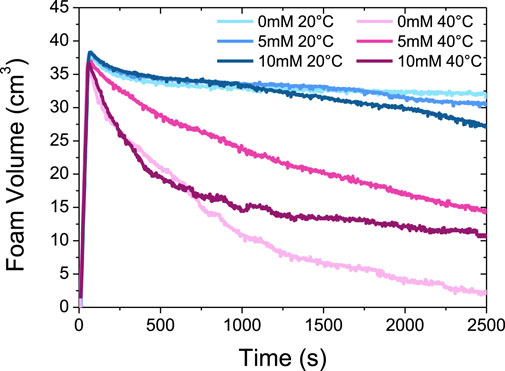
FIGURE 6. Evolution of the foam volume as a function of time at 20°C and 40°C with three different CaCl2 concentrations (0 mM, 5 mM and 10 mM).
For 5 mM and 10 mM of CaCl2, we also obtain stable foams at 20 °C, although slightly less than in absence of calcium, since the foam volume is respectively of around 30 cm3 (93% of initial volume) and 27.5 cm3 (78% of initial volume) after 2 500 s (Figure 6). We hypothesize that this slight foam stability decrease in the presence of calcium ions may be linked to a weak decrease of the adsorbed amount of protein in the presence of calcium ions. Indeed, at planar air/water interface, it has been shown by neutron reflectivity experiments that the presence of calcium ions led to thinner β-casein films and the adsorbed amount of protein decreased (Atkinson et al., 1995). This reduced adsorption of β-casein can be explained by the binding of calcium ions to the negatively charged phosphoserines residues in the hydrophilic domains, which in turn reduce the hydrophilicity (Atkinson et al., 1995). This is in accordance with our SANS results evidencing an increase of the aggregation number of the micelles in the presence of calcium, that arise from an increase of the hydrophobicity of the casein molecules.
At 40 °C, the behavior is markedly different from the calcium-free case since it remains an important volume of foam at the end of the experiment, respectively ∼15 cm3 (43% of initial volume) with 5 mM of CaCl2 and ∼10 cm3 (28.5% of initial volume) at 10 mM of CaCl2 (Figure 6). It is also worth noting that the decrease of the foam volume at 10 mM is almost similar to the calcium-free case in the first times up to around 600 s, but slows down strongly after this characteristic time at 10 mM while keeping continuously decreasing in the salt-free case. The existence of large aggregates of several hundreds of nm in solution, that are both present at 40°C with 5 or 10 mM of CaCl2, enhance thus markedly the foam stability, in comparison to the case where the foam is obtained at 40 °C from a solution of isolated β-casein micelles without calcium.
The average liquid fractions just after foam formation and after 2 500 s are presented in Figure 7 for 20°C and 40°C. Additional data at 30°C are shown Supplementary Tables S3, S4. At 20 °C, the foams are wet just after their formation since the average liquid fraction is around 17% for all the samples, whether they contain calcium or not (Figure 7A). This means that the bubble size lie in the range of 50–100 µm and that the bubbles remain small with almost a constant size during the foam production when only isolated micelles are present in solution (Figure 6A). When increasing temperature from 20 °C to 40 °C, only a slight decrease of the average liquid fraction is observed from around 17%–14% without CaCl2. A much larger decrease in average liquid fraction is observed with 5 and 10 mM of CaCl2. At 40 °C, the average fraction of liquid is around 8.6% with 10 mM of CaCl2. This demonstrates that the presence of aggregates in solution leads to dryer foams than those produced by solutions containing only micelles.
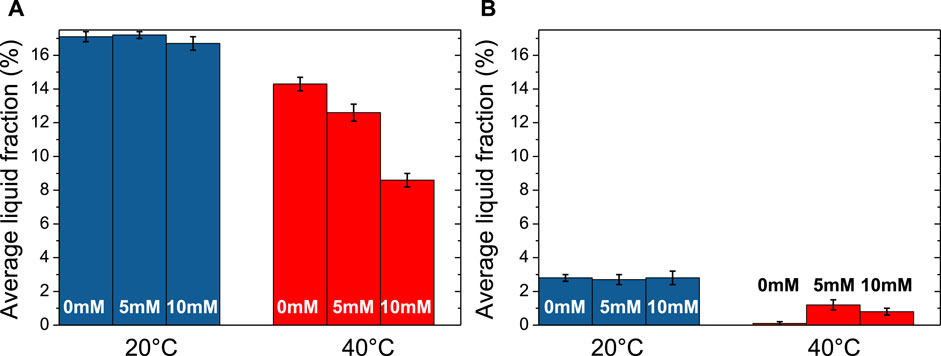
FIGURE 7. Evolution of the average liquid fraction at 20°C and 40°C with three different CaCl2 concentrations (0 mM, 5 mM and 10 mM): (A) just after foam formation and (B) after 2500 s.
We also determined the average liquid fraction at the end of experiments (after 2 500 s) (Figure 7B and Supplementary Table S4). At 20 °C, a relatively small amount of liquid remains in the foam since the average liquid fraction at the end is around 2.8% for all the samples. Although foams are stable, they become dry, showing that the β-casein micelles in liquid channels are not efficient to prevent drainage. At 40 °C, it was not possible to determine the average liquid fraction for the individual β-casein micelles solution without calcium since almost no foam remained. However, in presence of the aggregates obtained with 5 mM of CaCl2, the average liquid fraction is still around 1.2%, showing the ability of these aggregates to retain liquid inside the foam, and so to limit drainage. The same trend is observed for aggregates obtained with 10 mM of CaCl2.
All of these results enable to decouple the respective influence of temperature and calcium, and therefore on the influence of the presence of either isolated micelles or aggregates in solution, on the three different processes that leads to foam destabilization. At 20°C, the presence of calcium has almost no influence since all foams behave the same way, which is consistent with the fact that solutions contain only individual micelles whatever the calcium content. Foams are stable but dry quickly with time, passing from wet to dry foams. This demonstrates that micelles do not prevent drainage, but that the adsorbed proteins at the air/water interface form a sufficient elastic interfacial layer to block coarsening and coalescence.
On the reverse, at 40°C, the presence of calcium, and the subsequent formation of aggregates in solution, has a drastic influence on the foam behavior. When only micelles are present in absence of calcium, the foam almost fully destabilizes on a timescale of 2500s, evidencing that coarsening and/or coalescence now operate within the foam along with the drainage. This is line with the fact that bubble dissolution and coarsening is enhanced upon an increase in temperature, which is driven by the Laplace pressure across the curved gas-liquid interface (Poulichet and Garbin, 2015). Also, it is possible that the conformation change of the protein at the interface may be not fast enough to stabilize efficiently the bubbles or do not form a sufficient elastic interfacial layer to prevent bubbles dissolution (Poulichet and Garbin, 2015). In presence of aggregates when calcium is added in solution, the stability of foam is largely enhanced compared to the calcium-free case, although it is poorer than at 20°C, demonstrating that the destabilization processes are slowed down. For what concern coalescence and coarsening, concurrent mechanisms may occur that may act on the characteristic times of such processes. Indeed, on one hand, it has been described in the literature that the interfacial gelation occurs more rapidly at planar air/water interface when there are β-casein micelles aggregates in bulk than in the case of isolated β-casein micelles (Dauphas et al., 2005; Vessely et al., 2005). On the other hand, other studies have evidenced a decrease of the amount of adsorbed proteins and thinner films in the presence of calcium at planar air/water interface (Atkinson et al., 1995). Therefore, even if interfacial gelation happens, it is possible that the quantity of proteins adsorbed was too low to efficiently stabilize the interface. Finally, it is possible that the introduction of a large quantity of charged calcium cations screens also the electrostatic repulsions in the thin foam films and lead to their collapse (Murray, 2007; Rullier et al., 2008). It is however likely that the main mechanism that enables to keep a partial stability of the foam at 40°C in presence of calcium is the slowing down of the drainage, even if the size and the number of aggregates were too low within the foam liquid channels to arrest it completely. The peculiar two-step behavior of the temporal evolution of the foam produced at 10 mM of CaCl2 (an initial decrease of the foam volume similar to the salt-free case up to 400 s followed by a sudden important slow-down of foam destabilization) pleads for such a scenario. It is indeed likely that the initial viscosity of the aggregates solution remain too low to prevent drainage. However, as the foams progressively dries up, the large aggregates trapped within the Plateau borders concentrate themselves up to the point where they overlap or percolate, which in turn drastically enhance the viscosity which enables then to prevent efficiently the drainage. The presence of β-casein micelles aggregates in the foam liquid channels may then prevent foam destabilization by acting as a cork (Fameau and Salonen, 2014).
Overall, this demonstrates that the presence of aggregates induced by the addition of calcium at high temperatures enables to slow down the different process of foam destabilization, but do not allow a complete stabilization of the foam on the long-term, which is the prerequisite to produce temperature tunable foams (Fameau and Fujii, 2020). The system of pure β-casein micelles would then allow to obtain temperature tunable foam, with a stable foam at ambient temperature and a poor stable foam at ∼ 40°C, but the long-term stability of such foams is low in comparison to other foam systems described in literature with so-called ultrastable foams lasting over several months/years (Rio et al., 2014; Fameau and Fujii, 2020). The ability of the β-casein micelles system to form reversible large aggregates of micelles at a targeted temperature upon the proper addition of calcium amount does however not allow to obtain a temperature tunable foam with a reverse behavior, i.e. an unstable foam at low temperature and a stable foam at high temperature. Such kind of foam has not been yet designed in literature to the best of our knowledge and remains a challenge for the foam community (Fameau and Fujii, 2020).
4 Conclusion
Our results highlight that the addition of calcium in solutions of β-casein micelles allows to tune finely their thermal behaviour in two ways. First, we demonstrated by SANS that it is possible to obtain solution of isolated micelles that have exactly the same morphology, and therefore the same aggregation number of unimers, at two different temperatures by playing on such calcium content. This demonstrates that both parameters act on the hydrophobic interactions in the same way and that a “calcium-temperature superposition principle” (here, around 10 mM/35°C) may be applied if ones wants to design micelles with a given targeted number of aggregation. Second, the threshold of the transition from isolated micelles to aggregates that is driven by the formation of calcium bridges connecting the β-casein micelles, are also tunable by the interplay between calcium content and temperature. Remarkably, we showed by SANS that the hydrophilic chains of the corona were in a good solvent for all the conditions we probed and interact through excluded volume interactions even when the β-casein micelles aggregates themselves. The formation of such bridges do not modify the internal molecular structure of the micelles, which leads to the complete reversibility of the aggregation process through temperature cycling.
In terms of foam properties, the aggregation of β-casein micelles inside the foam liquid channels induced by the combined effects of calcium and temperature enabled to obtain stable dry foams on a timescale of 1 hour at a large temperature for which calcium-free foams have completely destabilized over such a characteristic time. This enhanced stability comes from the slowing down of the drainage due to the presence of the β-casein micelles aggregates that act as a cork in the foam liquid channels when the foam is enough dry to entrap such large aggregates within the Plateau borders. Although the transition between β-casein micelles and aggregates was reversible in bulk, such foams containing aggregates were not sufficiently stable on the very long term in the range of temperature and calcium content under scrutiny to obtain thermoresponsive foams for which the stable state happens above the triggering temperature threshold of stabilization/destabilization. Our study however demonstrates that the slowing down of drainage by aggregates β-casein micelles just after foam formation is a promising strategy to design such kinds of thermoresponsive foams, provided that drainage would be completely arrested by either larger aggregates or larger concentrations of aggregates. Since it is known that the self-association of β-casein micelles is also dependent on various physico-chemical parameters such as buffer types, solvent mixtures (addition of ethanol), or the presence of other proteins (Mikheeva et al., 2003), it would be interesting in the future to study if such parameters would allow for the conception of foams with enhanced properties.
Data availability statement
The original contributions presented in the study are included in the article/Supplementary Material, further inquiries can be directed to the corresponding authors.
Author contributions
AF designed, directed, performed the research and wrote the manuscript. BH performed experiments. AR designed the research. FC analysed the data and wrote the manuscript. All the authors were involved in the discussion throughout the manuscript preparation and writing.
Funding
This work benefited from the use of the SasView application, originally developed under NSF award DMR-0520547. SasView contains code developed with funding from the European Union’s Horizon 2020 research and innovation programme under the SINE2020 project, grant agreement No 654000.
Acknowledgments
The authors gratefully acknowledge the Laboratoire Léon Brillouin (LLB) for the allocation of neutron beam time on the diffractometer PACE. The authors also gratefully acknowledge INRAE and G. Delaplace for providing analytical tools, sharing expertise about casein structure. The acquisition of DLS was possible through ANR Proteinolab Project and ANR Proteinopeps at Laboratoire UMET, which is a project on innovative based casein ingredient.
Conflict of interest
The authors declare that the research was conducted in the absence of any commercial or financial relationships that could be construed as a potential conflict of interest.
Publisher’s note
All claims expressed in this article are solely those of the authors and do not necessarily represent those of their affiliated organizations, or those of the publisher, the editors and the reviewers. Any product that may be evaluated in this article, or claim that may be made by its manufacturer, is not guaranteed or endorsed by the publisher.
Supplementary material
The Supplementary Material for this article can be found online at: https://www.frontiersin.org/articles/10.3389/frsfm.2022.1008965/full#supplementary-material
References
Amin, S., V Barnett, G., Pathak, J. A., Roberts, C. J., and Sarangapani, P. S. (2014). Protein aggregation, particle formation, characterization & rheology. Curr. Opin. Colloid Interface Sci. 19, 438–449. doi:10.1016/j.cocis.2014.10.002
Aschi, A., Calmettes, P., Daoud, M., Douillard, R., and Gharbi, A. (2009). Micelle formation in beta-casein solutions. Polym. Guildf. 50, 6024–6031. doi:10.1016/j.polymer.2009.10.051
Aschi, A., Gharbi, A., Daoud, M., Douillard, R., and Calmettes, P. (2007). Study of structure and scaling behavior of chemically unfolded β-casein by means of small-angle neutron scattering. Polym. Int. 56, 606–612. doi:10.1002/pi.2176
Atamer, Z., Post, A. E., Schubert, T., Holder, A., Boom, R. M., and Hinrichs, J. (2017). Bovine β-casein: Isolation, properties and functionality. A review. Int. Dairy J. 66, 115–125. doi:10.1016/j.idairyj.2016.11.010
Atkinson, P. J., Dickinson, E., Horne, D. S., and Richardson, R. M. (1995). Neutron reflectivity of adsorbed β-casein and β-lactoglobulin at the air/water interface. J. Chem. Soc. Faraday Trans. 91, 2847–2854. doi:10.1039/ft9959102847
Author Anonymous (2022). Sasview for small angle scattering analysis. No Title, (n.d.)Availble at: https://www.sasview.org/.
Bucciarelli, S., Mahmoudi, N., Casal-Dujat, L., Jéhannin, M., Jud, C., and Stradner, A. (2016). Extended law of corresponding states applied to solvent isotope effect on a globular protein. J. Phys. Chem. Lett. 7, 1610–1615. doi:10.1021/acs.jpclett.6b00593
Chen, M., Sala, G., Meinders, M. B. J., van Valenberg, H. J. F., van der Linden, E., and Sagis, L. M. C. (2017). Interfacial properties, thin film stability and foam stability of casein micelle dispersions. Colloids Surfaces B Biointerfaces 149, 56–63. doi:10.1016/j.colsurfb.2016.10.010
Dauphas, S., Amestoy, M., Llamas, G., Anton, M., and Riaublanc, A. (2008). Modification of the interactions between β-casein stabilised oil droplets with calcium addition and temperature changing. Food Hydrocoll. 22, 231–238. doi:10.1016/j.foodhyd.2006.11.006
Dauphas, S., Mouhous-Riou, N., Metro, B., Mackie, A. R., Wilde, P. J., Anton, M., et al. (2005). The supramolecular organisation of β-casein: Effect on interfacial properties. Food Hydrocoll. 19, 387–393. doi:10.1016/j.foodhyd.2004.10.005
De Kruif, C. G., Huppertz, T., Urban, V. S., and V Petukhov, A. (2012). Casein micelles and their internal structure. Adv. Colloid Interface Sci. 171, 36–52. doi:10.1016/j.cis.2012.01.002
Dexter, A. F., Malcolm, A. S., and Middelberg, A. P. J. (2006). Reversible active switching of the mechanical properties of a peptide film at a fluid–fluid interface. Nat. Mat. 5, 502–506. doi:10.1038/nmat1653
Dickinson, E. (2020). Advances in food emulsions and foams: Reflections on research in the neo-pickering era. Curr. Opin. Food Sci. 33, 52–60. doi:10.1016/j.cofs.2019.12.009
Dickinson, E., Hunt, J. A., and Horne, D. S. (1992). Calcium induced flocculation of emulsions containing adsorbed β-casein or phosvitin. Food Hydrocoll. 6, 359–370. doi:10.1016/s0268-005x(09)80003-9
Fameau, A.-L., Carl, A., Saint-Jalmes, A., and Von Klitzing, R. (2015). Responsive aqueous foams. ChemPhysChem 16, 66–75. doi:10.1002/cphc.201402580
Fameau, A.-L., and Fujii, S. (2020). Stimuli-responsive liquid foams: From design to applications. Curr. Opin. Colloid & Interface Sci. 50, 101380. doi:10.1016/j.cocis.2020.08.005
Fameau, A.-L., Lam, S., Arnould, A., Gaillard, C., Velev, O. D., and Saint-Jalmes, A. (2015). Smart nonaqueous foams from lipid-based oleogel. Langmuir 31, 13501–13510. doi:10.1021/acs.langmuir.5b03660
Fameau, A.-L., Saint-Jalmes, A., Cousin, F., Houinsou Houssou, B., Novales, B., Navailles, L., et al. (2011). Smart foams: Switching reversibly between ultrastable and unstable foams. Angew. Chem. Int. Ed. 50, 8264–8269. doi:10.1002/anie.201102115
Fameau, A.-L., and Salonen, A. (2014). Effect of particles and aggregated structures on the foam stability and aging. C. R. Phys. 15, 748–760. doi:10.1016/j.crhy.2014.09.009
Guinier, A., Fournet, G., and Yudowitch, K. L. (1955). Small-angle scattering of X-rays. New York: John Wiley & Sons, Inc.
Jacrot, B. (1976). The study of biological structures by neutron scattering from solution. Rep. Prog. Phys. 39, 911–953. doi:10.1088/0034-4885/39/10/001
Kajiwara, K., Niki, R., Urakawa, H., Hiragi, Y., Donkai, N., and Nagura, M. (1988). Micellar structure of β-casein observed by small-angle X-ray scattering. Biochimica Biophysica Acta - Protein Struct. Mol. Enzym. 955, 128–134. doi:10.1016/0167-4838(88)90186-0
Leclerc, E., and Calmettes, P. (1997). Interactions in micellar solutions ofβ-casein. Phys. Rev. Lett. 78, 150–153. doi:10.1103/physrevlett.78.150
Leclerc, E., and Calmettes, P. (1997). Structure of β-casein micelles. Phys. B Condens. Matter 241, 1141–1143. doi:10.1016/s0921-4526(97)00850-8
Li, M., Auty, M. A. E., Crowley, S. V., Kelly, A. L., O’Mahony, J. A., and Brodkorb, A. (2019). Self-association of bovine β-casein as influenced by calcium chloride, buffer type and temperature. Food Hydrocoll. 88, 190–198. doi:10.1016/j.foodhyd.2018.09.035
McCarthy, N. A., Kelly, A. L., O’Mahony, J. A., and Fenelon, M. A. (2014). Sensitivity of emulsions stabilised by bovine β-casein and lactoferrin to heat and CaCl2. Food Hydrocoll. 35, 420–428. doi:10.1016/j.foodhyd.2013.06.021
Mikheeva, L. M., V Grinberg, N., Grinberg, V. Y., Khokhlov, A. R., and de Kruif, C. G. (2003). Thermodynamics of micellization of bovine β-casein studied by high-sensitivity differential scanning calorimetry. Langmuir 19, 2913–2921. doi:10.1021/la026702e
Murray, B. S. (2011). Rheological properties of protein films. Curr. Opin. Colloid Interface Sci. 16, 27–35. doi:10.1016/j.cocis.2010.06.005
Murray, B. S. (2007). Stabilization of bubbles and foams. Curr. Opin. Colloid Interface Sci. 12, 232–241. doi:10.1016/j.cocis.2007.07.009
Niño, M. R. R., Sánchez, C. C., and Patino, J. M. R. (1999). Interfacial characteristics of β-casein spread films at the air–water interface. Colloids Surfaces B Biointerfaces 12, 161–173. doi:10.1016/s0927-7765(98)00072-1
O’Connell, J. E., Grinberg, V. Y., and De Kruif, C. G. (2003). Association behavior of β-casein. J. Colloid Interface Sci. 258, 33–39. doi:10.1016/s0021-9797(02)00066-8
Pedersen, J. S., and Gerstenberg, M. C. (1996). Scattering form factor of block copolymer micelles. Macromolecules 29, 1363–1365. doi:10.1021/ma9512115
Portnaya, I., Cogan, U., Livney, Y. D., Ramon, O., Shimoni, K., Rosenberg, M., et al. (2006). Micellization of bovine β-casein studied by isothermal titration microcalorimetry and cryogenic transmission electron microscopy. J. Agric. Food Chem. 54, 5555–5561. doi:10.1021/jf060119c
Poulichet, V., and Garbin, V. (2015). Cooling particle-coated bubbles: Destabilization beyond dissolution arrest. Langmuir 31, 12035–12042. doi:10.1021/acs.langmuir.5b03480
Rio, E., Drenckhan, W., Salonen, A., and Langevin, D. (2014). Unusually stable liquid foams. Adv. Colloid Interface Sci. 205, 74–86. doi:10.1016/j.cis.2013.10.023
Rullier, B., Novales, B., and V Axelos, M. A. (2008). Effect of protein aggregates on foaming properties of β-lactoglobulin. Colloids Surfaces A Physicochem. Eng. Aspects 330, 96–102. doi:10.1016/j.colsurfa.2008.07.040
Saint-Jalmes, A., Peugeot, M.-L., Ferraz, H., and Langevin, D. (2005). Differences between protein and surfactant foams: Microscopic properties, stability and coarsening. Colloids Surfaces A Physicochem. Eng. Aspects 263, 219–225. doi:10.1016/j.colsurfa.2005.02.002
Vessely, C. R., Carpenter, J. F., and Schwartz, D. K. (2005). Calcium-induced changes to the molecular conformation and aggregate structure of β-casein at the air− water interface. Biomacromolecules 6, 3334–3344. doi:10.1021/bm050353w
Weißenborn, E., and Braunschweig, B. (2019). Hydroxypropyl cellulose as a green polymer for thermo-responsive aqueous foams. Soft Matter 15, 2876–2883. doi:10.1039/c9sm00093c
Keywords: foam, protein, beta-casein, micelle, aggregation
Citation: Fameau A-L, Houinsou Houssou B, Riaublanc A and Cousin F (2022) Interplay of temperature and calcium content in beta-casein solutions: From controlled self-aggregation of micelles in bulk to the design of stable foams. Front. Soft. Matter 2:1008965. doi: 10.3389/frsfm.2022.1008965
Received: 01 August 2022; Accepted: 22 November 2022;
Published: 07 December 2022.
Edited by:
Francisco Monroy, Complutense University of Madrid, SpainReviewed by:
Vasil M. Garamus, Helmholtz Centre for Materials and Coastal Research (HZG), GermanyMarcel Krzan, Jerzy Haber Institute of Catalysis and Surface Chemistry (PAN), Poland
Copyright © 2022 Fameau, Houinsou Houssou, Riaublanc and Cousin. This is an open-access article distributed under the terms of the Creative Commons Attribution License (CC BY). The use, distribution or reproduction in other forums is permitted, provided the original author(s) and the copyright owner(s) are credited and that the original publication in this journal is cited, in accordance with accepted academic practice. No use, distribution or reproduction is permitted which does not comply with these terms.
*Correspondence: Anne-Laure Fameau, QW5uZS1sYXVyZS5mYW1lYXVAaW5yYWUuZnI=; Fabrice Cousin, RmFicmljZS5jb3VzaW5AY2VhLmZy