- 1Laboratory of Marine Geosciences, Postgraduate Program in Environmental Oceanography, Department Oceanography, Universidade Federal do Espírito Santo, Vitória, Brazil
- 2Instituto de Biologia and SAGE-COPPE, Universidade Federal do Rio de Janeiro, Rio de Janeiro, Brazil
Geomorphology provides the core attributes for outlining marine seascapes, once the structural complexity of the seafloor mediates several oceanographic processes and ecosystem services, and is positively associated with biodiversity. Shelf-incised valleys and other prominent meso-scale structures such as reefs and sinkholes have a great potential for the discrimination of benthic habitat groups. Here, we investigate shelf-incised valleys as a mesophotic habitat, by focusing on their geomorphological control in defining distinct habitats in comparison with the flat surrounding area. The study was based on the integration of high-resolution bathymetry data (multibeam echosounder), video imaging, and physical-chemical parameters of the water column. Habitat mapping was conducted using object-based image analysis segmentation and clustering. Principal Component Analysis was used to assess the variables associated with habitat distribution at each morphological region of the valleys. Bathymetric data revealed the presence of 5 shelf-incised valleys and 5 seabed classes were defined as carbonate crusts, Rhodoliths (3 distinct classes) and unconsolidated sediments. A comprehensive habitat map with 17 classes was produced, and 13 are associated with valley´s relief. Extensive rhodolith beds were mapped in the valley flanks/bottom and in the flat areas. Shelf-incised valleys are prominent morphological features that add complexity to the seascape, contrasting with the flat relief that dominates the seascape. The seabed footage obtained in the valleys revealed that their heterogeneous, complex and irregular topography harbors a great diversity of epibionts, such as scleractinian corals, coralline algae, sponges and bryozoans. Most of the variability in the dataset is correlated with salinity, temperature and carbonate sediments, which seem to be the most influential variables over the biological assemblage, together with water depth and seabed slope. Shelf-incised valleys, similarly to submarine canyons, can define a complex mesophotic habitat and sustain distinct biodiversity, and even form mesophotic reefs. These features are the legacy of Quaternary sea-level changes and should be further investigated as important mesophotic habitats.
1 Introduction
Habitat mapping is an essential tool for the analysis and monitoring of coastal and marine systems, and comprise the basis for marine spatial planning (Pandian et al., 2009; Brown et al., 2011; Micallef et al., 2012). Nonetheless, a better knowledge of habitat structure and its association with biological assemblages is a major requirement for improving the planning and implementation of management measures, as biological communities might suffer direct and/or indirect consequences of anthropic activities, including global climate changes (Harris and Baker, 2020). Habitat is where a plant or an animal lives (Begon et al., 1996; Veech, 2021), and its study is based on the distribution of biological assemblages and their underlying physical and chemical environmental gradients (Kostylev et al., 2001; Brown and Blondel, 2009). Seabed sediments and geomorphological patterns are among the most influential drivers of benthic assemblages (Jerosch et al., 2015; Kaskela et al., 2017), and seabed mapping generates essential information for geo and biodiversity interdisciplinary analyses.
The geomorphological and faciological patterns along continental shelves are the result of distinct temporal and spatial scales processes. During ice ages, relative sea level dropped and expose continental shelves, while shelves were inundated due to the rise of relative sea level during interglacial stages (Green et al., 2014). Those long-term processes associated with short-term processes influence the continental shelf morphology, leaving relict features formed during sea-level lowstand or deglacial stillstands. Features resulting from sea-level oscillations such as incised valleys, drowned reefs, hardgrounds, relict sediments, submerged sinkholes, paleodunes, paleolagoons and paleocoastlines are present in the continental shelves and, along with other geomorphological features, drive marine habitat distribution (Harris et al., 2005; Wright et al., 2012; Bourguignon et al., 2018; Sherman et al., 2019). Drowned features in the mid and outer part of the continental shelf form mesophotic habitats (Loya et al., 2019). Mesophotic habitats are characterized by an environment that is associated with light-dependent organisms and filter feeders in a photic-aphotic transition zone between 30 to approximately 150 m water depth in tropical and subtropical continental shelves (Lesser et al., 2009; Hinderstein et al., 2010; Kahng et al., 2017). Also, other factors such as the bed slope, the micro-topography, and oceanographic forces play an important role in the mesophotic reefs’ habitat distribution (Bridge et al., 2011). For some time, mesophotic reefs have received considerable attention once they are often under less fishing pressure and can be used as climatic refugia (Bongaerts et al., 2010; Hinderstein et al., 2010; Bridge et al., 2011; Baker et al., 2016). Detailed mapping of mesophotic areas revealed extensive drowned/mesophotic reefs in the Amazonas outer shelf/shelf break (Moura et al., 2016; Lavagnino et al., 2020), in South Atlantic’s largest reef complex (Moura et al., 2013), in Australia shelf/slope and the Great Barrier reef (Harris et al., 2004; Bridge et al., 2012), Hawaiian Islands shelf (Grigg et al., 2002), Gulf of Mexico and Caribbean (Locker et al., 2010) and elsewhere.
Here, we investigate a mesophotic habitat defined by outer shelf-incised valleys. Similarly to submarine canyons that are known for their high habitat heterogeneity (Kottke et al., 2003; Schlacher et al., 2007), we consider that shelf-incised valleys could also present distinct mesophotic communities and reefs. Thus, the objective of this paper is to characterize the shelf-incised valleys as a mesophotic benthoscape or seascape, by focusing on their geomorphological control in forming distinct habitats in comparison with the flat surrounding area. To accomplish that, a high-resolution acoustic survey (multibeam echosounder) was combined with underwater footage for a broad view on the benthos distribution, and CTD profiles in order to characterize the physical oceanography conditions in the mesophotic habitat.
2 Study area
The study area is located in the central part of the Espirito Santo Continental Shelf (ESCS), southeast Brazil (Figure 1). The ESCS is a mixed sedimentation shelf with a one significant terrigenous riverine sediment input (Doce river) nearshore and a carbonate domain along the mid-outer shelf, that is largely comprised by rhodolith beds (Bastos et al., 2015; Vieira et al., 2019) and carbonate concretions near the shelf edge (Holz et al., 2020). Bastos et al. (2015) described three main geomorphological sectors in the ESCS: the Doce River shelf (dominated by riverine terrigenous sediment input); the Paleovalley shelf (characterized by the presence of shelf-incised valleys, low terrigenous sediment input and the dominance of carbonate sedimentation); and the Abrolhos shelf (shelf enlargement with carbonate sedimentation).
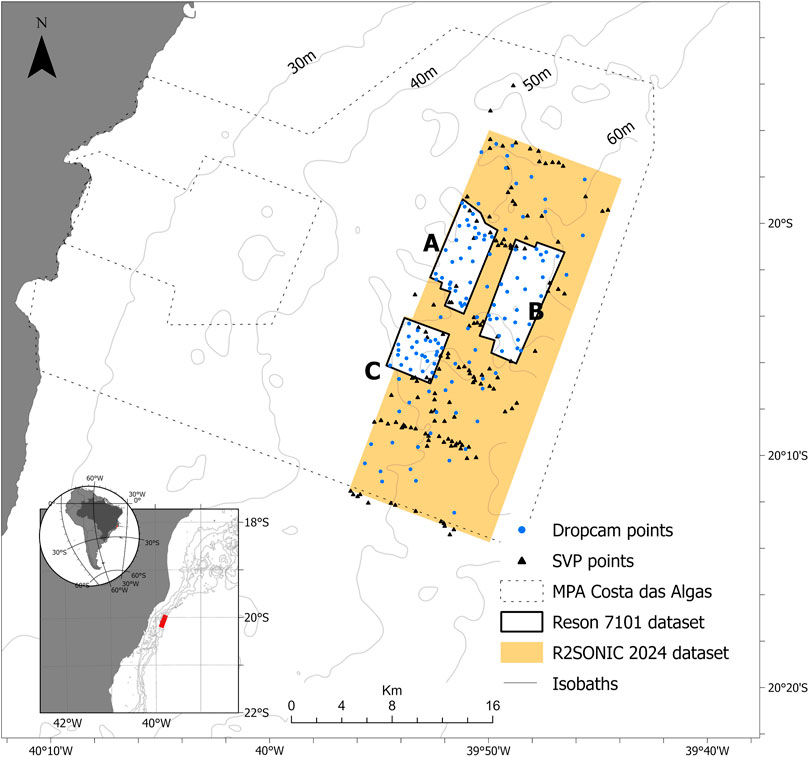
FIGURE 1. Map showing the study area within the MPA Costa das Algas, including the multibeam survey area (Reson 7,101 and R2Sonic 2024), the Dropcam sampling points and the SVP/CTD stations. (A–C) are areas surveyed by Reson 7,101. Isobaths were obtained from Bastos et al. (2015). Datum: WGS84.
The surveyed area (Figure 1) is located in the Costa das Algas Marine Protected Area (MPA), which is located in the Paleovalley shelf. This MPA was established in 2010 aiming to protect a high macroalgal biodiversity, including endemic species (IBAMA, 2006). The two hard bottom types—carbonate concretions (bioincrustation) and rodoliths—play an important ecological role by providing an adequate substrate for benthic macroalgae and invertebrates’ settlement, increasing community complexity (Holz et al., 2020). In the MPA, Vieira et al. (2019), Bourguignon et al. (2018) and Holz et al. (2020) showed that seabed sediments are characterized by a complex mosaic of fine sediments, Rhodoliths, hard-grounds (biogenic crusts), bioclastic gravel and maerl. Rhodolith nodule coverage varies along the outer shelf and can reach up to 85% of bed coverage (Rocha et al., 2020).
Previous shelf morphology and sedimentation studies in the study region focused on the influence of sedimentary regimes and shelf morphology in fishing (Bourguignon et al., 2018); the relationship between seabed morphology and sea-level changes (Bastos et al., 2015); shelf morphology influence on seabed habitats (Oliveira et al., 2020); sedimentations patterns (Vieira et al., 2019); and backscatter response to rhodolith coverage (Rocha et al., 2020).
The climate in the study area is tropical, hot, and humid. Seasonality is marked by a rainy season during summer, with prevailing NE to E winds. The winter is marked by a dry season with frequent storms with waves from S to SE (Niemer, 1977; Vera et al., 2002). The tidal regime is semi-diurnal and classified as micro tides. Mesoscale ocean circulation is dominated by the south-flowing Brazil Current, with warmer (22°C) and saline (>36) tropical water (Palóczi et al., 2016). A summer upwelling occurs in the central ESCS and contributes to nutrient enrichment (Mazzini and Barth, 2013; Palóczi et al., 2016).
3 Materials and methods
Figure 2 shows the methodological flow used herein, considering the different steps: Data collection, processing, analysis and habitat map.
3.1 Seabed acoustic mapping
The MPA Costa das Algas acoustic survey covered 294 km2 from 40 to 275 m water depths (Figure 1). The acoustic dataset was collected using two Multibeam Echosounders (MBES): 2018 survey used a Reson 7,101 operating in 240 kHz (Areas A, B and C, Figure 1); and the 2019 survey used a R2Sonic 2024 operating in 170 kHz (Area R, Figure 1). Different workflows were used for each MBES since the transducers and the acquisition mode are distinct. In both systems, a motion correction equipment was used to compensate for pitch, roll, yaw and heave. Also, water column velocity profiles were carried out using a mini Valeport SVP in regular intervals (149 stations) (Figure 1). Data were processed using software Caris Hips and Sips (9.1 and 11.1) and Qimera, corrections were applied as SVP and tides. The description of each system and the associated equipment used in each survey acquisition are summarized in Table 1.
3.2 Ground truth—Video imaging
Seabed was video imaged aiming to record the epibionts associated with the mapped area. Samples were obtained using a drop camera system operated in 129 stations between 43 and 77 m depths (Figure 1). The drop camera system used high-resolution cameras (GoPro Hero 3, 4, and 7) and torches coupled in a pyramidal metal structure with a 60 × 60 cm square base. The system was setup with an orthogonal camera looking downward and a second camera attached to the side of the structure, looking laterally. Images from the orthogonal camera were used to classify bottom types, while the lateral camera was used to obtain a panoramic view of the seabed. Images were obtained by recording three videos with 2-minutes at each station (drop camera was lifted three times during each cast). The analysis of the best still frames extracted from the video footage was conducted using the software Coral Point Count (CPCe, National Institute of Health, EUA), which allows for the identification of the organisms and sea bottom type from 50 points randomly distributed in each frame (Kohler and Gill, 2006). Images were classified in 5 seabed type classes, being 0—Reef, 1—Rodoliths, 2—Unconsolidated sediment, 3—Maërl, and 4—Rodoliths with Unconsolidated sediment. Rhodolith and unconsolidated sediments were quantified following the methodology presented by Rocha et al. (2020) and Matsuda and Iryu (2011). Rhodolith percentage was considered high when the nodules covered at least 40% of a given image (Rocha et al., 2020).
3.3 Segmentation and habitat map
A supervised segmentation approach, using an Object Based Image Analysis (OBIA), was applied to classify the seabed based on the bathymetric dataset. This allows the identification of homogeneous characteristics within an area of interest using image segmentation (Lacharité et al., 2018). OBIA takes multi-layer raster imagery and segments data into geographic zones with similar statistics properties (Innangi et al., 2019). In this process, it is expected that the desired objects are automatically extracted from the image (Lucieer et al., 2017), where the segmentation joins small objects (one-pixel size) with larger objects (Janowski et al., 2020). In this paper, RSOBIA (Remote Sensing Object Based Image Analysis, Le Bas, 2016) was applied in a 2-meter bathymetric map resolution. The number of classes and minimum object size (parameters needed to run RSOBIA) were defined as 6 and 2,000, respectively. After defining the seabed geomorphic classes, the Habitat classes were defined using a cluster analysis, that grouped the 6 geomorphic classes (bathymetry/RSOBIA) with 6 image-derived seabed classes (Figure 2). This type of non-supervised analysis classifies elements in groups so that elements from the same group are similar and the number of groups is unknown (Kaufman and Rousseeuw, 1990). The group number was analyzed through the k-means method (MacQueen, 1967) and ArcGIS was used for visualization.
3.4 Water parameters
Temperature (°C) and salinity (PSU) data were collected during sound velocity measurements in the water column during the multibeam data acquisition. Profiles were obtained in 149 stations along the study area using a mini SVP Valeport (Figure 1). All valley related profiles were collected during the summer of 2019 and they are presented here as an average, while T/S profiles along the adjacent flat areas were collected during 2019 summer and winter months. These data are presented here as an avarage to summer and an another to winter data. Water physical-chemical parameters were used to investigate the influence of the valley morphology in the water column structure and consequently in the biological community found. The profiles were analyzed and presented as valley bottom and flat adjacent areas.
3.5 Statistical analysis
3.5.1 Similarity
Statistical similarity analysis is a relational measurement between individual pairs or populations (Regazzi, 2001) and was used to compare the presence of groups of organisms with the seabed classes derived from multibeam bathymetry segmentation. The similarity among objects varies from 0 (highest difference) to 1 (highest similarity). Similarity analyses were carried out with the software PAST 2.17.
3.5.2 Principal Components Analysis
Principal Component Analysis (PCA) is a mathematical algorithm that reduces the dimensionality of the dataset, simplifying its description (Ringnér, 2008; Abdi and Williams, 2010). New variables (principal components) are obtained as a linear combination of the original variables (Abdi and Williams, 2010). The first principal component is the direction along which the samples show more variation and the second principal component is the non-correlated direction with the first component (Ringnér, 2008). Seafloor footage was used to determine the most influential variables over distinct organisms. Seven variables were used for each station: depth m), slope (°), rugosity, temperature (°C), salinity (PSU), carbonate content (%) and mud content (%). All parameters were retrieved from the primary dataset, except for rugosity. Rugosity was calculated using Benthic Terrain Modeler (BTM) (Oliveira et al., 2020). Carbonate and Mud contents were obtained from Vieira et al. (2019). The raw values of each variable in each station were normalized to avoid discrepancies. The analysis considered the distinct zones/classes defined for the study area. The dataset was analyzed in two ways: an integrated mode and by geomorphic classes (valley bottom, valley margin, and valley adjacent area).
4 Results
4.1 Seabed morphology
Seabed mapping revealed five valleys within the study area (Figure 3). The two northernmost valleys and the two NW-SE oriented central channels converged and formed one deeper and wider channel close to their mouth, while the southernmost and smaller one is SW-NE orientated. All valleys extended eastward to the continental shelf break. Overall, valley depths varied from 60 to 90 m depths, and valley widths from 70 to 500 m. The northern valleys (V1 and V2) are straight, while the central ones are meandering (V3 and V4). The southern valley (V5) is narrower and presents meanders and a more rectilinear channel. The area adjacent to the valleys presents a diverse morphology with positive and negative relief, including little channels many times connected to the valleys.
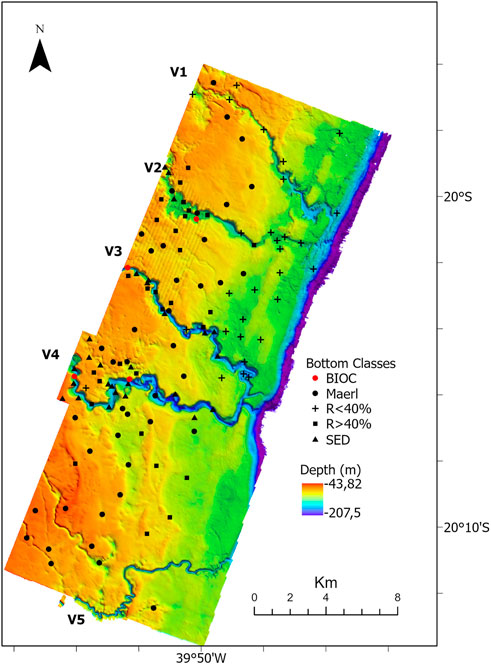
FIGURE 3. Shelf morphology highlighting the five shelf-incised valleys. Locations of classified seabed images defined from the dropcams are also shown. Bioincrustations (BIOC- rigid bottom with benthic cover); Unconsolidated sediment (SED-unconsolidated fine and coarse sediments); Rhodoliths (R>40% - >40% of the frame covered by rhodoliths). V1, V2, V3, V4, V5—shelf-incised valleys.
4.2 Seabed bottom classes from video footages
Five seabed type classes were defined from underwater imagery: Bioincrustations (BIOC- rigid bottom with benthic cover) (Figure 4A); Unconsolidated sediment (SED-unconsolidated fine and coarse sediments) (Figure 4B); Rhodoliths (R>40% - >40% of the frame covered by rhodoliths) (Figure 4C); Mäerl (carbonatic fragments) (Figure 4D); Rhodoliths with sediments (R<40%- <40% of the frame cover with rhodoliths) (Figure 4E).
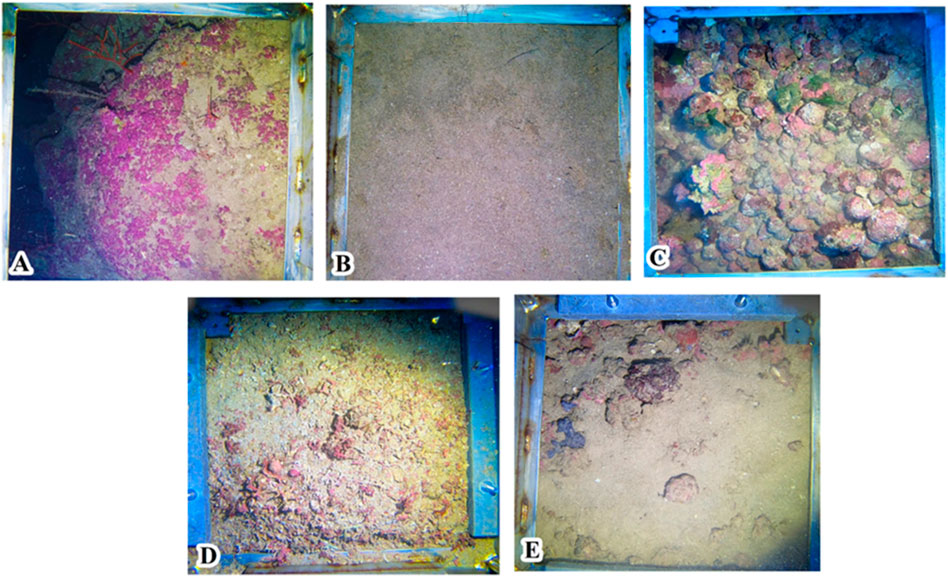
FIGURE 4. Seabed images representing the five seabed classes derived from the video footage: (A) Bioencrustation (BIOC); (B) Unconsolidated sediment (SED); (C) Rhodoliths (R>40%); (D) Mäerl; and (E) Rhodoliths with sediments (R<40%).
Bioencrustation class refers to biogenic crusts forming a hard ground, and in this case, mostly formed by calcareous algae. BIOC is largely associated with the valley flanks. The unconsolidated sediment class is characterized by fine sediments (fine sands with mud) with no gravel or bioclastic fragments associated. SED class were observed mainly in the valley bottom, but also locally associated with flat areas (Figure 3A). Mäerl class dominated the flat area adjacent to the valleys and is characterized by a bioclastic gravel with living algae in a fine sediment matrix. The Mäerl class does not show sparse rhodoliths in the image frame, however, it is possible that spatially, this class intermingle with the low rodolith coverage class (R<40%).
The rhodolith classes were defined based on the percentage of nodules in the image frame. In general, R>40% represents a rhodolith bed that can be treated as an irregular and quasi-rigid bottom, giving a three-dimensional aspect to the seafloor. R<40% represents areas where nodule coverage is less than 40%, indicating the presence of more sparse rhodoliths in a fine sediment bed. The rhodolith beds (R>40%) occurs mainly associated with the valley classes (flank) and the flat margin adjacent to the valleys, but mainly in areas deeper than 50 m. R<40% are present in flat areas.
4.3 Benthic coverage
Benthic organisms were identified in major taxonomic and functional groups including rhodoliths (carbonatic nodules covered largely by Crustose Coralline Algae—CCA), macroalgae, Geniculate Coralline Algae (GCA), sponges, corals, bryozoans, sea squirts, biofilm (consortia with microalgae and filamentous cyanobacteria) and others (echinoderms and non-identified organisms). The epifauna was more abundant in the rigid valley flanks or in the carbonate crusts adjacent to the valleys. At the valleys’ bottom dominated by fine sediment dominates, epifaunal organisms were not visible. Rhodoliths were identified close to the valleys and, together with GCA, macroalgae, bryozoans, sea squirts, encrusting sponges, and corals (Antipathes and Cirrhipathes) (Figure 5), comprised the most common groups recorded from the imagery.
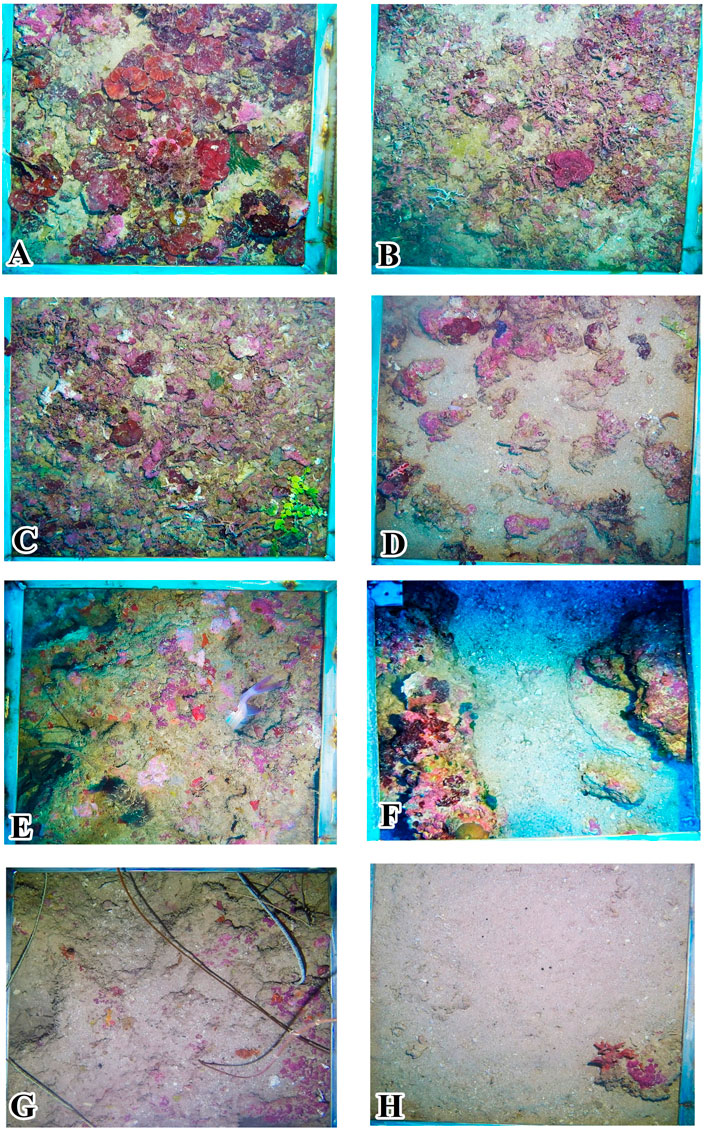
FIGURE 5. Examples of organisms found in the MPA Costa das Algas: (A) Valley flanks covered by sponges, biofilm, and black corals; (B) Bryozoans and sea squirts; (C) Rhodoliths covered by Peyssonnelia sp. (red algae); (D) Sponges and biofilm on valley flank; (E) Rhodoliths and Codium sp. (green algae); (F) GCA, green algae and sea urchin; (G) Bryozoans and sea star; (H) Biofilm, CCAs on hardgrounds and black corals.
The general distribution of the benthic assemblages and its relationship with water depth is shown in Figure 6. In the shallower water depth range (45–55 m), the bottom was dominated by CGA, followed by rhodoliths, bryozoans, macroalgae, and sea squirts. From 55 to 65 m deep, rhodoliths dominate, followed by GCA, bryozoans, macroalgae, and biofilm. The deeper depth range (65–77 m) was marked by the dominance of rhodoliths, biofilm and corals. Macroalgae, GCA, bryozoans and sponges were less frequent in this stratum, while sea squirts were not observed.
A similarity analysis among the biotic assemblages of valley margin, valley bottom and adjacent flat areas is shown in Figure 7. The higher similarity between the biotic assemblages of valley margin and bottom (0.83) can be explained by the proximity and similar habitat structure. The similarity between the bottom and valley adjacent areas is intermediary (0.465), as well the margin portion near to these areas (0.415).
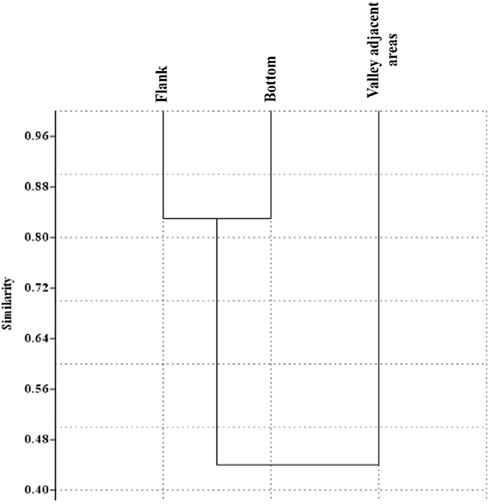
FIGURE 7. Similarity (Bray Curtis) in biotic composition among the valley bottom, valley flank, and adjacent areas.
4.4 Segmentation and habitat map
The OBIA segmentation resulted in six classes based on bathymetry derivatives (slope) and depth range (Table 2; Figure 8). Table 2 presents the classes descriptions based on morphological features and the area (km2) covered by each class.
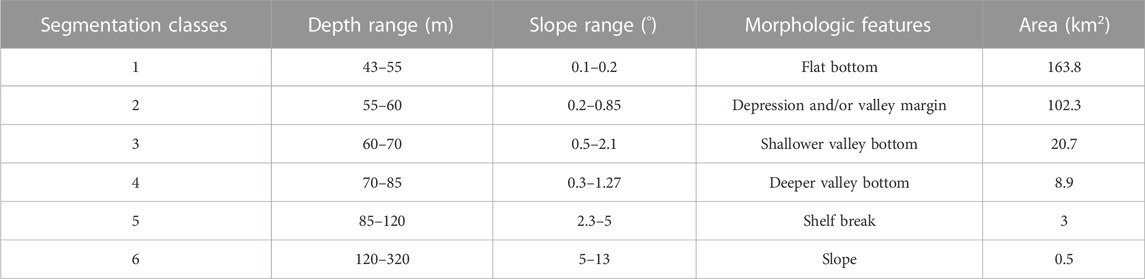
TABLE 2. Segmentation classes (geomorphic classes) and their geomorphometric and geomorphological features.
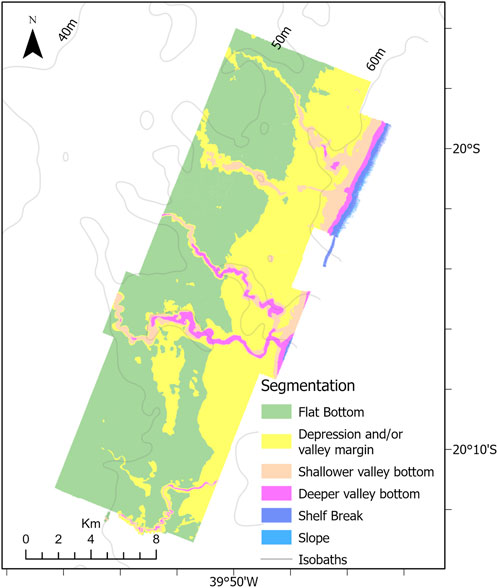
FIGURE 8. Map showing the results from OBIA segmentation. Six distinct classes were defined based on the geomorphometric analysis.
Shelf Break and Slope are the less representative classes, as they are at the depth limit of the study area. Conversely, classes Flat bottom (class 1) and Depression and/or Valley Margin class (class 2) cover most of the study area, 163.8 km2 and 102.3 km2, respectively and dominate the bottom morphology adjacent to the shelf valleys. These two classes differ in terms of depth range and slope, with the Depression and/or Valley Margin class occurring in a deeper depth range and with a slightly higher slope. The shelf valleys are characterized by the two Valley Bottom classes, Shallower valley bottom and Deeper valley bottom. These two latter classes comprise 29.6 km2, almost 10% of the study area and represent the largest relief changes and highest slopes. Also, two classes—Shelf Break and Slope—were not included in the analysis due their minimal area coverage and not present any sample of image over the bottom.
Seventeen habitat classes were defined from the clustering of seabed imagery and geomorphometric data (geomorphic classes, Figure 9; Table 3). The final habitat map is shown in Figure 10. Valley margins concentrate most habitat classes: three classes related to Deeper valley bottom; five associated with Shallower valley bottom; and five associated with Incised valley margin. Bioincrustation classes are limited to channel margin and shallow channel regions. Unconsolidated sediment bottoms (SED) are associated with higher slopes (Shallower and Deeper valley bottom, valley margins, and depressions). The other habitat classes are distributed among other types of morphology. Slope classes do not present any habitat classes since video footage was not obtained in these areas.
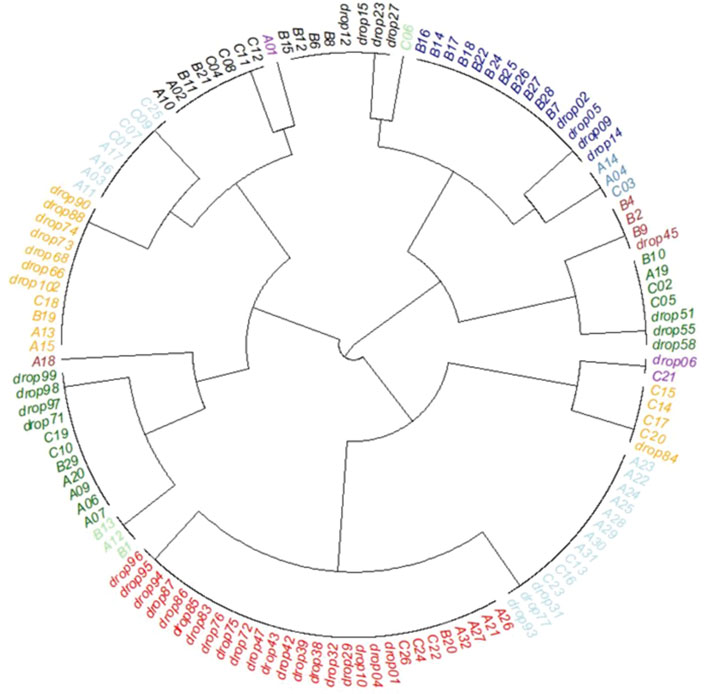
FIGURE 9. Cluster analysis (clockwise direction starting on C06): Bioencrustation on paleovalley margin (cyan); Rhodoliths on valley margin and depression (dark blue); Bioencrustation on shallower valley bottom (petroleum blue); Rhodoliths on deeper valley bottom (brown); Unconsolidated sediment on deeper valley bottom (dark green); Rhodoliths on flat bottom (purple); Unconsolidated sediment on flat bottom (orange); Rhodoliths with sediments on flat bottom (cyan); Mäerl on flat bottom (red), Rhodoliths with sediments on shallower valley bottom (light green); Rhodoliths with sediments on depression and valley margin (dark green); Rhodoliths with sediments on deeper valley bottom (brown); Mäerl on valley margin and depression (orange); Unconsolidated sediment on depression and valley margin (cyan); Unconsolidated sediment on shallower valley bottom (black); Mäerl on shallower valley bottom (purple); Rhodoliths on shallower valley bottom (black).
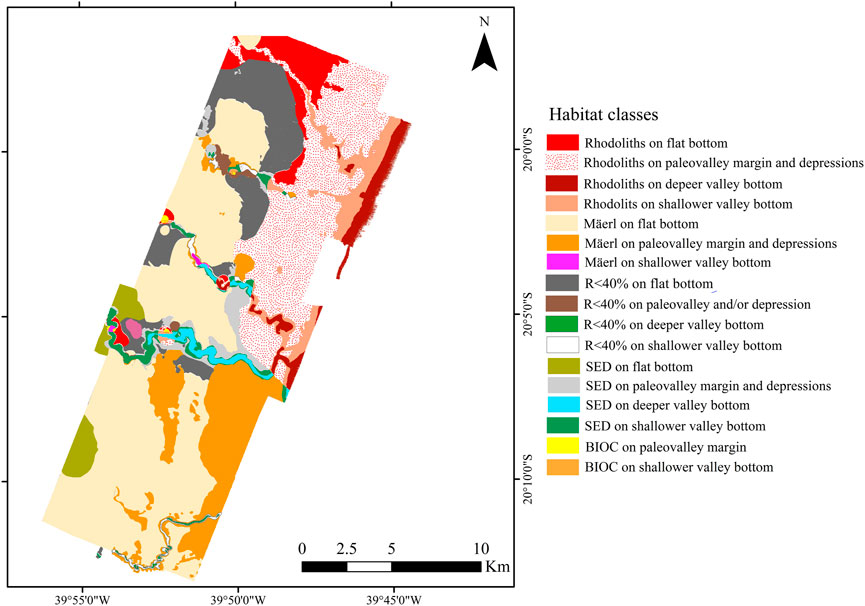
FIGURE 10. Marine habitat map based on the results of the cluster analysis. Habitat classes were defined by clustering data obtained from seabed imagery and geomorphometric analysis. Bioincrustations (BIOC-rigid bottom with benthic cover); Unconsolidated sediment (SED-unconsolidated fine and coarse R> sediments); Rhodoliths (40%->40% of the frame covered by rhodoliths).
4.5 Water column parameters
The valley water profiles present a temperature variation of ∼7°C between surface and bottom (up to 76 m) (Figure 11A). The greatest variation in thermocline was ∼6°C in a 28-meter interval starting at 20 m water depth. The high/lowest salinity variations were found on the same thermocline interval.
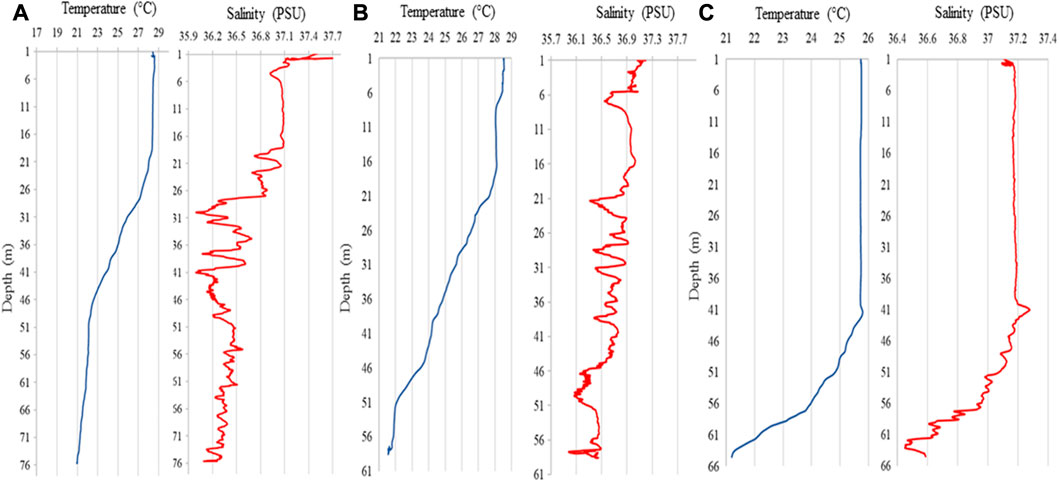
FIGURE 11. Temperature (°C) and Salinity (PSU) profiles: (A) Valley bottom during summer; (B) Adjacent area during summer; (C) Adjacent area during fall/winter.
Comparing T/S results for the flat areas adjacent to the valley (only summer 2019 measurements), the temperature ranged ∼7°C between surface and bottom (up to 59 m depth). The thermocline spanned 28 m, starting at 18 m depth, and a 4°C variation between the lowest values close to the bottom and the surface (Figure 11B). Fall/winter results showed a smaller temperature and a different thermocline when compared to summer behavior. The difference between highest and lowest temperatures was 5°C and the thermocline was deeper, starting at 42 m up to 64 m deep, with ∼5°C range. The greatest salinity variation occurs within the same depth range (Figure 11C).
4.6 Principal Component Analysis
PCA was run for the entire dataset and individually for each morphological class of the valleys. The first PCA accounted for 59.1% of the total variance in the dataset (Figure 12A). In general, the first component was associated to most of the variability in the dataset, and is correlated with salinity, temperature and carbonate sediments, which seem to be the most influential variables over the biological assemblages. The second component revealed a consistent but smaller influence of depth and slope variations.
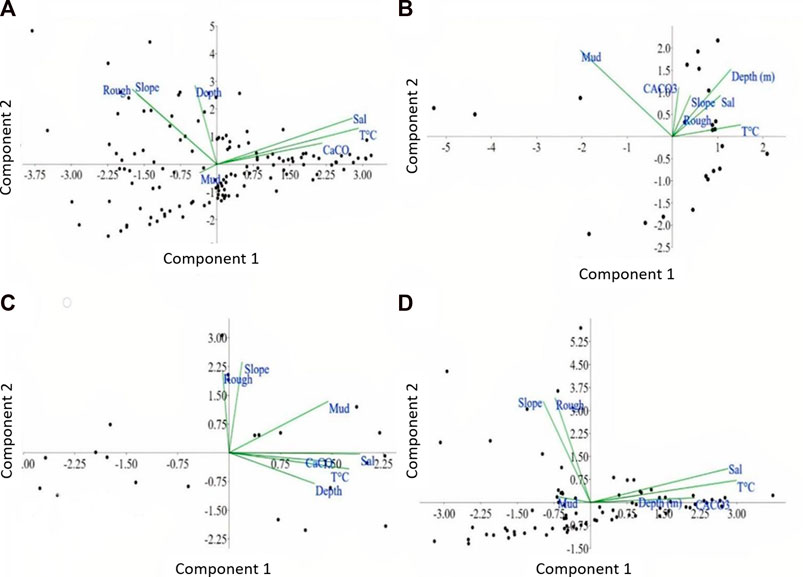
FIGURE 12. Principal Components Analysis results: (A) Integrated analysis (valley bottom, flanks and valley adjacencies areas; (B) Valley bottom; (C) Valley flanks; (D) Valley Adjacent areas.
Applying the principal components individually for each morphological class of the valleys provided different results. This means that the variables can explain each morphological feature in a different approach. In the valley bottom (segmentation classes Deeper valley bottom and shallower valley bottom) the two first principal components explain 63.4% of the total variance (Figure 12B). Analyzing the two components and the variables within them, temperature and depth were dominant in the component 1, while mud content and depth were dominant in component 2.
The valley flanks/margins (segmentation classes Depression and/or valley margin) PCA shows that the two first principal components explain 65.4% of the total variance (Figure 12C). Salinity, temperature, mud content and carbonate content are dominant on component 1, while slope and roughness (0.590) were dominants on component 2. This was expected since the images showed that flanks and margins with high slopes are fixed by bioencrustation.
In the valley adjacent areas (segmentation classes Flat bottom and shelf break), the two first principal variance components explain 53.5% of the total variance (Figure 12D). Component 1 shows that temperature and salinity were dominant, while component 2 indicates that roughness and slope were the most dominant. In this flatter region, with smooth slope and depth variation, it was expected that sediment type coverage was a preponderant factor for the presence of epifauna groups. The regions where the contents of mud and carbonate are the most variable components are the ones closer to the channels.
5 Discussion
Substrate relief and types play a major role in the distribution of benthic communities, together with other biotic and abiotic parameters of the water column such as depth, light penetration, and productivity (Bridge et al., 2011; Rattray et al., 2013; Kaskela et al., 2017; Turner et al., 2017). The physical properties of the seabed are major surrogates to forecast or model benthic habitat and species’ distribution. For instance, grain size and shelf morphology (slope, rugosity) are among the variables with the largest explanatory power for benthic biological assemblages (Kostylev et al., 2001; Beaman and Harris, 2008), and interact with depth, temperature, light penetration and other drivers (Greene et al., 2007; Lesser et al., 2009; Kahng et al., 2007; Locker et al., 2010; Bridge et al., 2011; Kahng et al., 2012; Kaskela et al., 2017). Grazing, competition and recruitment are among the biological drivers of community structure (James, 2000; Cochrane and Lafferty, 2002).
Shelf habitat distribution tends to be closely related to seascape/benthoscape and seabed sediment types (Brown et al., 2011). As a consequence, much of benthic “physical” habitats are strongly controlled by shelf morphology, which is the result of a combination of processes that operate at different time scales, including relative and eustatic sea-level fluctuations, modern sedimentary regimes, hydrodynamic conditions, sediment transport, antecedent geology, and biological activity (Sternberg and Nowell, 1999; Pratson et al., 2007; Schattner et al., 2010; Brothers et al., 2013; Bastos et al., 2015). Glacial and interglacial stages lead to global sea-level fluctuations that exposed and drowned the continental shelf. These processes were responsible to shape the seafloor and, in the case of mesophotic habitats and mesophotic reefs, most of shelf morphology reflects features formed during sea-level fall and lowstand, which drowned during the deglaciation process. The development of mesophotic reefs, for instance, is related to submarine morphology resembling features such as drowned reefs (Harris and Davies, 1989; Bridge et al., 2011; Abbey et al., 2013), paleoshorelines (Brooke et al., 2014; Pretorius et al., 2016), hard grounds/terraces (Khanna et al., 2017), cemented dunes and barriers (Brooke et al., 2014; Passos et al., 2019), and incised valleys (Bastos et al., 2022).
The MPA Costa das Algas shelf-incised valleys are prominent morphological features that add complexity to the seascape, contrasting with the flat relief that dominates the seascape. The seabed footage obtained in the valleys revealed that their heterogeneous, complex and irregular topography harbors a great diversity of epibionts, such as scleractinian corals, coralline algae, sponges and bryozoans, which are typical of mesophotic reef systems (Hinderstein et al., 2010).
Geomorphological processes are controlling parameters to the distribution and composition of mesophotic ecosystems (Bridge et al., 2011; Sherman et al., 2019) since positive relief can direct the sediment transport and accumulation (Sherman et al., 2019). Herein, the geomorphological heterogeneity of the MPA Costa das Algas is associated with a broad range of biotic and abiotic variables, including benthic community distribution, substrate composition, terrain derivatives (slope, rugosity), temperature and salinity.
The valley margins portion was the segment that showed the greatest relationship among hardgrounds, terrain complexity (slope), and epibiont diversity. The higher carbonate content in these areas, largely accounted by encrusters, enabling higher hard bottom stability, providing n important settlement habitat for reef-associated organisms. Organisms on rigid bottoms are influenced by slope and sediment deposition (Colin et al., 1986). Steep slopes generate terrain complexity and influence the availability of settlement surfaces, food and protection against predators (Ierodiaconou et al., 2007). Besides that, the terrain slope angle also plays an important role in determining water column vertical zones (Bridge et al., 2011). Rugosity, especially when associated with bottom type and steeper slopes, also increases seabed complexity and macrofaunal diversity (Bridge et al., 2011). The hard and rugose structure of channel walls may also contribute to increase the biodiversity of this habitat by creating a positive feedback system. The reef community along the margins and walls restrict the channel growth, which, in turn, may create different water flow patterns (Harris et al., 2005). Such distinctive current flux influences food supply and organic matter in these regions (Okamura and Partridge, 1999; Cochrane and Lafferty, 2002), and might aid the transport and settlement of several sessile species (Gili and Coma, 1998). Moreover, the valleys may play an important role during summer upwelling (Palóczi et al., 2016) by channeling colder and nutrient enriched water masses.
On the valley flanks portion, CCA, corals and sponges are the most conspicuous groups, with the extensive live coverage of CCAs being frequent above the flanks. These organisms are well adapted to low light conditions (Kühl et al., 2001) and also play an important role as binders of several other framework builders such as corals (Riding, 2002). The corals in the valley flanks are black corals belonging to genera Antipathes and Cirrhipathes (Loiola et al., 2007), which benefit from their hard substrate for fixation (Rivero-Calle et al., 2008; Wagner et al., 2012). These organisms are filter feeders that require a strong and consistent current to settle (Tazioli et al., 2007) such as in valley flanks.
On the valley bottom portion, the abundance and diversity of macroorganisms was overall low. Despite the slightly lower temperatures and constrained light penetration near the bottom, the low diversity in these areas seem to be more related with the finer grain size, which is also a depth-related consequence (Watling and Skinder, 2007). Although sediments tend to constrain the abundance and diversity of macroorganisms in the bottom surface, soft bottom realms may harbor rich meiofaunal and other smaller sized assemblages that were not targeted by our study (Beaman and Harris, 2008). Bryozoans, which are colonial and sessile filter feeders (Lidgard, 2008), were conspicuous in the valley bottom portion. Bryozoans can settle in shelf environments that are shaded or cryptic, and with lower sedimentation rates (Winston et al., 2007).
The valley adjacent area comprises a flat region dominated by rhodoliths, CGA, macroalgae, and bryozoans. Less frequent groups included black corals, sponges, and sea squirts. Temperature, carbonate and mud content were correlated with the distribution of the benthic organisms. The flat and less complex topography of the valley adjacent area seems to be associated with a less diverse morphological setting, especially when compared with the valley flanks and bottom. These flat areas are also more susceptible to sediment transport and deposition (Sherman et al., 2010; 2019), which might damage filter feeders and macroalgae development, but allowing the development of other biological communities (Locker et al., 2010). In these areas, rhodoliths are the dominant group, often forming rhodolith beds. The Brazilian margin (3°–22°S) encompasses the world’s largest rhodolith beds (Foster, 2001; Amado-Filho et al., 2012), formed largely by calcareous and geniculate coralline algae (Foster, 2001) and associated preferably to coarse sandy sediment with bioclastic origin (Sañé et al., 2016) (Amado-Filho et al., 2012; Bastos et al., 2015; Vieira et al., 2019; Holz et al., 2020). The tri-dimensional rhodolith nodular structure, specially on seabed with a nodule coverage above 40%, forms a fabric that add more dimension to the habitat so rhodoliths are known as “ecosystem engineers” (Crain and Bertness, 2006). This tri-dimensional structure increases benthic diversity and provides an important habitat for reef fishes (Steller and Foster, 1995; Moura et al., 2021). Also, the nodular rhodoliths’ form attenuates turbulent disturbances (Hinojosa-Arango and Riosmena-Rodríguez, 2004), leading to a higher stability of the seabead and ultimately favoring sessile organism settlement. As observed in the video footage and presented by Holz et al. (2020) in the study area, the rhodolith beds are denser towards the continental shelf break, transitioning from sparse nodules intercalated by carbonate/coarse/bryozoan sands to very dense aggregations that can also merge and form carbonate crusts. Macroalgae is widely observed in associated with rhodoliths in the MPA. The majority of macroalgae requires a rigid substrate to settle (Steller and Foster, 1995). Due to their capacity to adapt in a variety of light and nutrient conditions, macroalgae are found in the entire range of mesophotic communities (Baker et al., 2016). When associated with rhodoliths, they are highly dependent on light and their abundance usually decreases with depth (Amado-Filho et al., 2010). Regarding the rigid substrate provided by rhodoliths, sea squirt was also observed. They are encrusted organisms that constitute an important part of the benthic fauna and consolidated substrate and could live solitary or in a colony (Brusca et al., 2007).
The combined analysis of benthic terrain model with the general benthic community description showed a closed association between benthic groups and mapped features. The results altogether allowed us to indicate that shelf-incised valleys are a major geomorphological feature that lead to a complex mosaic of habitats and potentially create a considerable variation in depth and slope-controlled habitats. Further detailed studies on the benthic community distribution and their ecological dynamics in the valleys and adjacent areas can provide a better understanding of the ecological significance of these features and increase our knowledge about this understudy mesophotic habitat. Moreover, mesophotic reefs are not necessarily potential refuge from natural and anthropogenic impacts, with evidences that even deep reefs are impacted worldwide (Rocha et al., 2018). Thus, for a better conservation planning and management, a seascape ecology investigation approach is strongly encouraged for future works in the MPA Costa das Algas mesophotic valleys.
6 Conclusion
A geomorphometric analysis combined with seabed imagery defined a complex mosaic of mesophotic habitats that are, in part, a legacy of Quaternary sea-level fluctuations. The presence of shelf-incised valleys imprints a geomorphological feature that is responsible for a distinct and heterogeneous habitat. The incised valleys enable a variety of benthic community settlements due to their complex morphology (margin, flank and bottom), producing distinct habitats within and among the valleys.
Valley flanks and bottom represent distinct habitats when compared to adjacent flatter areas. The morphological complexity and the valleys’ relief enable the occurrence of a diverse mesophotic community. Steeper areas covered by rigid substrates, such as the valley flanks, are high potential areas that can be related to higher diversity of epifauna in comparison with the unconsolidated substrate in gentler slopes such as on the bottom of the valleys and marginal flat areas. Although temperature and low light incidence may limit the fauna in the valleys, the terrain slope is one of the determinant factors influencing diverse and the occurrence of distinct group of organisms.
Shelf-incised valleys, similarly to submarine canyons, can define a complex mesophotic habitat and sustain distinct biodiversity. Shelf valleys form mesophotic reefs dominated by rhodoliths and calcareous algae crusts. The valley adjacent flatter areas were also recognized to be important habitats, mostly because of extensive rhodolith beds.
The mesophotic habitats described herein are worth of attention regarding the MPA management plans. A seascape ecology study is an important step forward to investigate the benthic dynamics and the ecological functions on this mesophotic habitats and understand their potential response to climate vulnerability.
Data availability statement
The raw data supporting the conclusion of this article will be made available by the authors, without undue reservation.
Author contributions
NO: field work, conceptualization, formal analysis, Investigation, writing—original draft; AB: conceptualization, investigation, review, editing, supervisor; AL: field work, investigation, review, and editing; GR: field work, investigation, review; RM investigation, review, and editing.
Funding
Data collection was carried out as part of CNPq PELD and FAPES PELD, Recuperação da Bacia do Rio Doce (FAPES Grant 77683390) and Environmental Monitoring Program (PMBA) (Fundação Renova-FEST-RRDM).
Acknowledgments
We are very much thankful to CNPq, FAPES, CAPES and Fundação Renova for financial support. First Author received a scholarship from Capes and FAPES. ACB and RLM are CNPq Research Fellows (PQ1). We are grateful to all LaboGeo colleagues that collaborated during field work, data processing and further discussions. This research is a contribution to Rede Abrolhos. We are thankfull to ICMBio-MMA.
Conflict of interest
The authors declare that the research was conducted in the absence of any commercial or financial relationships that could be construed as a potential conflict of interest.
Publisher’s note
All claims expressed in this article are solely those of the authors and do not necessarily represent those of their affiliated organizations, or those of the publisher, the editors and the reviewers. Any product that may be evaluated in this article, or claim that may be made by its manufacturer, is not guaranteed or endorsed by the publisher.
References
Abbey, E., Webster, J. M., Braga, J. C., Jacobsen, G. E., Thorogood, G., Thomas, A. L., et al. (2013). Deglacial mesophotic reef demise on the great barrier reef. Palaeogeogr. Palaeoclimatol. Palaeoecol. 392, 473–494. doi:10.1016/j.palaeo.2013.09.032
Abdi, H., and Williams, L. J. (2010). Principal component analysis. Wiley Interdiscip. Rev. Comput. Stat. 2, 433–459. doi:10.1002/wics.101
Amado-Filho, G., Maneveldt, G. W., Pereira-Filho, G. H., Manso, R. C. C., Bahia, R. G., Barros-Barreto, M. B., et al. (2010). Seaweed diversity associated with a Brazilian tropical rhodolith bed. Ciencias Mar. 36, 371–391. doi:10.7773/cm.v36i4.1782
Amado-Filho, G. M., Moura, R. L., Bastos, A. C., Salgado, L. T., Sumida, P. Y., Guth, A. Z., et al. (2012). Rhodolith beds are major CaCO 3 BIO-factories in the tropical south West Atlantic. PLoS One 7, 351711–e35210. doi:10.1371/journal.pone.0035171
Baker, E. K., Puglise, K. A., and Harris, P. T. (2016). Mesophotic coral ecosystems - a lifeboat for coral reefs? UN environment. Arendal, Norway: GRID-Arendal.
Bastos, A. C., D’Agostini, D. P., Silva, A. E., Menandro, P. M., Vieira, F. V., Boni, G. C., et al. (2022). Sedimentological and morphological evidences of meltwater pulse 1B in the southwestern atlantic margin. Mar. Geol. 450, 106850. doi:10.1016/j.margeo.2022.106850
Bastos, A. C., Quaresma, V. S., Marangoni, M. B., D’Agostini, D. P., Bourguignon, S. N., Cetto, P. H., et al. (2015). Shelf morphology as an indicator of sedimentary regimes: A synthesis from a mixed siliciclastic-carbonate shelf on the eastern Brazilian margin. J. South Am. Earth Sci. 63, 125–136. doi:10.1016/j.jsames.2015.07.003
Beamen, R. J., and Harris, P. T. (2008). Geophysical variables as predictors of megabenthos assemblages from the northern great barrier reef, Australia. Canada: Seafloor Habitat Charact. Geol. Assoc. Canada 241–258.
Begon, M., Harper, J. L., and Townsend, C. R. (1996). Ecology: Individuals, popula-tions and communities. Cambridge, UK: Blackwell Scientific Publications, 945.
Bongaerts, P., Ridgway, T., Sampayo, E. M., and Hoegh-Guldberg, O. (2010). Assessing the ‘deep reef refugia’ hypothesis: Focus on caribbean reefs. Coral Reefs 29, 309–327. doi:10.1007/s00338-009-0581-x
Bourguignon, S. N., Bastos, A. C., Quaresma, V. S., Vieira, F. V., Pinheiro, H., Amado-Filho, G. M., et al. (2018). Seabed morphology and sedimentary regimes defining fishing grounds along the eastern Brazilian shelf. Geosci 8, 91. doi:10.3390/geosciences8030091
Bridge, T., Beaman, R., Done, T., and Webster, J. (2012). Predicting the location and spatial extent of submerged coral reef habitat in the great barrier reef world heritage area, Australia. PLoS One 7, e48203. doi:10.1371/journal.pone.0048203
Bridge, T. C. L., Done, T. J., Beaman, R. J., Friedman, A., Williams, S. B., Pizarro, O., et al. (2011). Topography, substratum and benthic macrofaunal relationships on a tropical mesophotic shelf margin, central Great Barrier Reef, Australia. Coral Reefs 30, 143–153. doi:10.1007/s00338-010-0677-3
Brooke, B. P., Olley, J. M., Pietsch, T., Playford, P. E., Haines, P. W., Murray-Wallace, C. V., et al. (2014). Chronology of quaternary coastal aeolianite deposition and the drowned shorelines of southwestern Western Australia – A reappraisal. Quat. Sci. Rev. 93, 106–124. doi:10.1016/j.quascirev.2014.04.007
Brothers, D. S., ten Brink, U. S., Andrews, B. D., and Chaytor, J. D. (2013). Geomorphic characterization of the U.S. Atlantic continental margin. Mar. Geol. 338, 46–63. doi:10.1016/j.margeo.2012.12.008
Brown, C. J., and Blondel, P. (2009). Developments in the application of multibeam sonar backscatter for seafloor habitat mapping. Appl. Acoust. 70, 1242–1247. doi:10.1016/j.apacoust.2008.08.004
Brown, C. J., Smith, S. J., Lawton, P., and Anderson, J. T. (2011). Benthic habitat mapping: A review of progress towards improved understanding of the spatial ecology of the seafloor using acoustic techniques. Estuar. Coast. Shelf Sci. 92, 502–520. doi:10.1016/j.ecss.2011.02.007
Brusca, R. C., Brusca, G. J., and Silveira, F. L. (2007). Invertebrados. 2. Rio de Janeiro: Guanabara Koogan.
Cochrane, G. R., and Lafferty, K. D. (2002). Use of acoustic classification of sidescan sonar data for mapping benthic habitat in the Northern Channel Islands, California. Cont. Shelf Res. 22, 683–690. doi:10.1016/S0278-4343(01)00089-9
Colin, P. L., Devaney, D. M., Hillis-Colinvaux, L., Suchanek, T. H., and Harrison, J. T. (1986). Geology and biological zonation of the reef slopes, 50-360 m depth at enewetok atoll, Marshall Islands. Bull. Mar. Sci. 38, 111–128.
Crain, C. M., and Bertness, M. D. (2006). Ecosystem engineering across environmental stress gradients: Implications for conservation and management. Bioscience 56, 211–216. doi:10.1641/0006-3568(2006)056[0211:EEAEGI]2.0.CO;2
Foster, M. S. (2001). Rhodoliths: Between rocks and soft places. J. Phycol. 37, 659–667. doi:10.1046/j.1529-8817.2001.00195.x
Gili, J. F., and Coma, R. (1998). Benthic suspension feeders: Their paramount role in littoral marine food webs. Trends Ecol. Evol. 13, 316–321. doi:10.1016/S0169-5347(98)01365-2
Green, A. N., Cooper, J. A. G., and Salzmann, L. (2014). Geomorphic and stratigraphic signals of postglacial meltwater pulses on continental shelves. Geology 42 (2), 151–154. doi:10.1130/G35052.1
Greene, H. G., Bizzarro, J. J., O’Connell, V. M., and Brylinsky, C. K. 2007. Construction of digital potential marine benthic habitat maps using a coded classification scheme and its application, Mapping the seafloor for habitat characterization 141 B J. Todd, and H. G. Greene, Geological Association Canada.
Grigg, R., Grossman, E., Earle, S., Gittings, S., Lott, D., and McDonough, J. (2002). Drowned reefs and antecedent karst topography, Au'au Channel, SE Hawaiian Islands. Coral Reefs 21 (1), 73–82. doi:10.1007/s00338-001-0203-8
Harris, P. T., and Baker, E. (2020). Seafloor Geomorphology as benthic habitat. Elsevier Sci, 1030. 2, doi:10.1016/C2017-0-02139-0
Harris, P. T., and Davies, P. J. (1989). Submerged reefs and terraces on the shelf edge of the Great Barrier Reef, Australia - morphology, occurrence and implications for reef evolution. Coral reefs. 8 (2), 87–98. doi:10.1007/BF00301807
Harris, P. T., Heap, A. D., Wassenberg, T., and Passlow, V. (2004). Submerged coral reefs in the Gulf of Carpentaria, Australia. Mar. Geol. 207 (1-4), 185–191. doi:10.1016/j.margeo.2004.03.002
Harris, P. T., Heap, A., Passlow, V., Hughes, M., Daniell, J., Hemer, M., et al. (2005). Tidally incised valleys on tropical carbonate shelves: An example from the northern Great Barrier Reef, Australia. Mar. Geol. 220, 181–204. doi:10.1016/j.margeo.2005.06.019
Hinderstein, L. M., Marr, J. C. A., Martinez, F. A., Dowgiallo, M. J., Puglise, K. A., Pyle, R. L., et al. (2010). Theme section on “mesophotic coral ecosystems: Characterization, ecology, and management. Coral Reefs 29, 247–251. doi:10.1007/s00338-010-0614-5
Hinojosa-Arango, G., and Riosèmena-Rodríguez, R. (2004). Influence of rhodolith-forming species and growth-form on associated fauna of rhodolith beds in the central-west Gulf of California, Mexico. México. Mar. Ecol. 25, 109–127. doi:10.1111/j.1439-0485.2004.00019.x
Holz, V. L., Bahia, R. G., Karez, C. S., Vieira, F. V., Moraes, F. C., Vale, N. F., et al. (2020). Structure of rhodolith beds and surrounding habitats at the doce river shelf (Brazil). Diversity 12, 75–19. doi:10.3390/d12020075
Ierodiaconou, D., Laurenson, L., Burq, S., and Reston, M. (2007). Marine benthic habitat mapping using Multibeam data, georeferenced video and image classification techniques in Victoria, Australia. J. Spat. Sci. 52, 93–104. doi:10.1080/14498596.2007.9635105
Innangi, S., Tonielli, R., Romagnoli, C., Budillon, F., Di Martino, G., Innangi, M., et al. (2019). Seabed mapping in the pelagie Islands marine protected area (sicily channel, southern mediterranean) using remote sensing object based image analysis (RSOBIA). Mar. Geophys Res. 40, 333–355. doi:10.1007/s11001-018-9371-6
James, D. W. (2000). Diet, movement, and covering behavior of the sea urchin Toxopneustes roseus in rodolith beds in the Gulf of California. México. Mar. Bio 137, 913–923. doi:10.1007/s002270000423
Janowski, L., Madricardo, F., Fogarin, S., Kruss, A., Molinaroli, E., Kubowicz-Grajewska, A., et al. (2020). Spatial and temporal changes of tidal inlet using object-based image analysis of multibeam echosounder measurements: A case from the lagoon of venice, Italy. Remote Sens. 12, 2117. doi:10.3390/rs12132117
Jerosch, K., Kuhn, G., Krajnik, I., Scharf, F. K., and Dorschel, B. (2015). A geomorphological seabed classification for the Weddell Sea, Antarctica. Mar. Geophys. Res. 37, 127–141. doi:10.1007/s11001-015-9256-x
Kahng, S., Copus, J. M., and Wagner, D. (2017). Mesophotic coral ecosystems. Mar. Anim. For. 10, 978–983. doi:10.1007/978-3-319-17001-5
Kahng, S. E., and Kelley, C. (2007). Vertical zonation of habitat forming benthic species on a deep photosynthetic reef (50–140 m). Au’au Channel. Hawaii. Coral Reefs 26, 679–687. doi:10.1007/s00338-007-0253-7
Kahng, S., Wagner, D., Lantz, C., Vetter, O., Gove, J., and Merrifield, M. (2012). Temperature-related depth limits of warm-water corals. Available at: www.icrs2012.com/proceedings/manuscripts/ICRS2012_9C_1.pdf.
Kaskela, A. M., Rousi, H., Ronkainen, M., Orlova, M., Babin, A., Gogoberidze, G., et al. (2017). Linkages between benthic assemblages and physical environmental factors: The role of geodiversity in Eastern Gulf of Finland ecosystems. Cont. Shelf Res. 142, 1–13. doi:10.1016/j.csr.2017.05.013
Kaufman, L., and Rousseeuw, P. J. (1990). Finding groups in data: An introduction to cluster analysis. New York: John Wiley.
Khanna, P., Droxler, A. W., Nittrouer, J. A., Tunnell Jr, J. W., and Shirley, T. C. (2017). Coralgal reef morphology records punctuated sea-level rise during the last deglaciation. Nat. Commun. 8 (1046), 1046–1048. doi:10.1038/s41467-017-00966-x
Kohler, K. E., and Gill, S. M. (2006). Coral point Count with excel extensions (CPCe): A visual basic program for the determination of coral and substrate coverage using random point count methodology. Comput. Geosci. 32 (9), 1259–1269. doi:10.1016/j.cageo.2005.11.009
Kostylev, V. E., Todd, B. J., Fader, G. B. J., Courtney, R. C., Cameron, G. D. M., and Pickrill, R. A. (2001). Benthic habitat mapping on the Scotian Shelf based on multibeam bathymetry, surficial geology and sea floor photographs. Mar. Ecol. Prog. Ser. 219, 121–137. doi:10.3354/meps219121
Kottke, B., Schwenk, T., Breitzke, M., Wiedicke, M., Kudrass, H. R., and Spiess, V. (2003). Acoustic facies and depositional processes in the upper submarine canyon swatch of No Ground (Bay of Bengal). Deep-Sea Res. Part II 50, 979–1001. doi:10.1016/S0967-0645(02)00616-1
Kühl, M., Glud, R. N., Borum, J., Roberts, R., and Rysgaard, S. (2001). Photosynthetic performance of surface-associated algae below sea ice as measured with a pulse-amplitude-modulated (PAM) fluorometer and O2 microsensors. Mar. Ecol. -Prog. Ser. 223, 1–14. doi:10.3354/meps223001
Lacharité, M., Brown, C. J., and Gazzola, V. (2018). Multisource multibeam backscatter data: Developing a strategy for the production of benthic habitat maps using semi-automated seafloor classification methods. Mar. Geophys. Res. 39, 307–322. doi:10.1007/s11001-017-9331-6
Lavagnino, A. C., Bastos, A. C., Amado Filho, G. M., de Moraes, F. C., Araujo, L. S., and de Moura, R. L. (2020). Geomorphometric seabed classification and potential megahabitat distribution in the amazon continental margin. Front. Mar. Sci. 7, 1–19. doi:10.3389/fmars.2020.00190
Le Bas, T. P., 2016. RSOBIA-A new OBIA toolbar and toolbox in ArcMap 10. x for segmentation and classification. In Geobia 2016: Solutions and synergies; N. Kerle, M. Gerke, and S. Lefevre, Eds.; University of Twente Faculty of Geo-Information and Earth Observation (ITC): Enschede, Netherlands.
Lesser, M. P., Slattery, M., and Leichter, J. J. (2009). Ecology of mesophotic coral reefs. J. Exp. Mar. Bio. Ecol. 375, 1–8. doi:10.1016/j.jembe.2009.05.009
Lidgard, S. (2008). Predation on marine bryozoan colonies: Taxa, traits and trophic groups. Mar. Ecol. Prog. Ser. 359, 117–131. doi:10.3354/meps07322
Locker, S. D., Armstrong, R. A., Battista, T. A., Rooney, J. J., Sherman, C., and Zawada, D. G. (2010). Geomorphology of mesophotic coral ecosystems: Current perspectives on morphology, distribution, and mapping strategies. Coral Reefs 29, 329–345. doi:10.1007/s00338-010-0613-6
Loiola, L. (2007). Black corals (Cnidaria: Antipatharia) from Brazil: An overview conserv. Adapt. Manag. Seamount Deep. Coral Ecosyst. 19662285, 12.
Loya, Y., Puglise, K. A., and Bridge, T. C. L. (2019). Mesophotic coral ecosystems. Springer Nat. Switz. 23, 1003. doi:10.1007/978-3-319-92735-0
Lucieer, V., Roche, M., Degrendele, K., Malik, M., Dolan, M., and Lamarche, G. (2017). User expectations for multibeam echo sounders backscatter strength data-looking back into the future. Mar. Geophys. Res. 1-24, 23–40. doi:10.1007/s11001-017-9316-5
MacQueen, J. (1967). Some methods for classification and analysis of multivariate observations. Proc. fifth Berkeley symposium Math. statistics Probab. 1, 281–297.
Matsuda, S., and Iryu, Y., I. (2011). Rhodoliths from deep fore-reef to shelf areas around Okinawa-jima, Ryukyu Islands, Japan. Mar. Geol. 282, 215–230. doi:10.1016/j.margeo.2011.02.013
Mazzini, P. L. F., and Barth, J. A. (2013). A comparison of mechanisms generating vertical transport in the Brazilian coastal upwelling regions. J. Geophys. Res. 118 (11), 5977–5993. doi:10.1002/2013JC008924
Micallef, A., Le Bas, T. P., Huvenne, V. A. I., Blondel, P., Deidun, A., and Veit, H. (2012). A multi-method approach for benthic habitat mapping of shallow coastal areas with high-resolution multibeam data. Cont. Shelf Res. 39-40, 14–26. doi:10.1016/j.csr.2012.03.008
Moura, R. L., Abieri, M. L., Castro, G. M., Carlos-Junior, L. A., Chiroque-Solano, P. M., Fernandes, N. C., et al. (2021). Tropical rhodolith beds are a major and belittled reef fish habitat. Sci. Rep. 11, 794. doi:10.1038/s41598-020-80574-w
Moura, R. L., Amado-Filho, G. M., Moraes, F. C., Brasileiro, P. S., Salomon, P. S., Mahiques, M. M., et al. (2016). An extensive reef system at the Amazon River mouth. Sci. Adv. 2 (4), e1501252. doi:10.1126/sciadv.1501252
Moura, R. L., Secchin, N. A., Amado-Filho, G. M., Francini-Filho, R. B., Freitas, M. O., Minte-Vera, C. V., et al. (2013). Spatial patterns of benthic megahabitats and conservation planning in the Abrolhos Bank. Cont. Shelf Res. 70, 109–117. doi:10.1016/j.csr.2013.04.036
Okamura, B., and Partridge, J. C. (1999). Suspension feeding adaptations to extreme flow environments in a marine bryozoan. Biol. Bull. 196, 205–215. doi:10.2307/1542566
Oliveira, N. de, Bastos, A. C., da Silva Quaresma, V., and Vieira, F. V. (2020). The use of Benthic Terrain Modeler (BTM) in the characterization of continental shelf habitats. Geo-Marine Lett. 1, 1087–1097. doi:10.1007/s00367-020-00642-y
Palóczy, A., Brink, K. H., da Silveira, I. C. A., Arruda, W. Z., and Martins, R. P. (2016). Pathways and mechanisms of offshore water intrusions on the Espírito Santo Basin shelf (18°S–22°S, Brazil). J. Geophys Res. Oceans 121, 5134–5163. doi:10.1002/2015JC011468
Pandian, P. K., Ruscoe, J. P., Shields, M., Side, J. C., Harris, R. E., Kerr, S. A., et al. (2009). Seabed habitat mapping techniques: An overview of the performance of various systems. Mediterr. Mar. Sci. 10 (2), 29–43. doi:10.12681/mms.107
Passos, T. U., Webster, J. M., Braga, J. C., Voelker, D., Renema, W., Beaman, R. J., et al. (2019). Paleoshorelines and lowstand sedimentation on subtropical shelves: A case study from the fraser shelf, Australia. Aust. J. Earth Sci. 66 (4), 547–565. doi:10.1080/08120099.2018.1558417
Pratson, L. F., Nittrouer, A. C. A., Wiberg, P. L., Steckler, M. S., Swenson, J. B., Cacchione, D. A., et al. 2007. Seascape evolution on clastic continental shelves and slopes, In Continental margin sedimentation: From sediment transport to sequence stratigraphy/edited by C. A. Nittrouer Blackwell, Oxford: Special publication number 37 of the International Association of Sedimentologists 339.
Pretorius, L., Green, A., and Cooper, A. (2016). Submerged shoreline preservation and ravinement during rapid postglacial sea-level rise and subsequent “slowstand”. Bulletin 128 (7-8), 1059–1069. doi:10.1130/B31381.1
Rattray, A., Ierodiaconou, D., Monk, J., Versace, V. L., and Laurenson, L. J. B. (2013). Detecting patterns of change in benthic habitats by acoustic remote sensing. Mar. Ecol. Prog. Ser. 477, 1–13. doi:10.3354/meps10264
Regazzi, A. J. (2001). Análise multivariada. Viçosa: Universidade Federal de Viçosa, Centro de Ciências Exatas e Tecnológicas. Dep. Inform. 2001, 166p.
Riding, R. (2002). Structure and composition of organic reefs and carbon-ate mud mounds: Concepts and categories. Earth Sci. Rev. 58, 163–231. doi:10.1016/S0012-8252(01)00089-7
Ringnér, M. (2008). What is principal component analysis? Nat. Biotechnol. 26, 303–304. doi:10.1038/nbt0308-303
Rivero-Calle, S., Armstrong, R. a., and Soto-Santiago, F. (2008). Biological and physical characteristics of a mesophotic coral reef: Black Jack reef, Vieques, Puerto Rico. Proc. 11th Int. Coral Reef. Symp. Int. Coral Reef. Symp., 567–571.
Rocha, G. A., Bastos, A. C., Amado-Filho, G. M., Boni, G. C., Moura, R. L., and Oliveira, N. (2020). Heterogeneity of rhodolith beds expressed in backscatter data. Mar. Geol. 423, 106136. doi:10.1016/j.margeo.2020.106136
Rocha, L. A., Pinheiro, H. T., Shepherd, B., Papastamatiou, Y. P., Luiz, O. J., Pyle, R. L., et al. (2018). Mesophotic coral ecosystems are threatened and ecologically distinct from shallow water reefs. Science 361 (6399), 281–284. doi:10.1126/science.aaq1614
Sañé, E., Chiocci, F. L., Basso, D., and Martorelli, E. (2016). Environmental factors controlling the distribution of rhodoliths: An integrated study based on seafloor sampling, ROV and side scan sonar data, offshore the W-Pontine Archipelago. Cont. Shelf Res. 129, 10–22. doi:10.1016/j.csr.2016.09.003
Schattner, U., Lazar, M., Tibor, G., Ben-Avraham, Z., and Makovsky, Y. (2010). Filling up the shelf — a sedimentary response to the last post-glacial sea rise. Mar. Geol. 278, 165–176. doi:10.1016/j.margeo.2010.10.006
Schlacher, T. A., Schlacher-Hoenlinger, M. A., Williams, A., Althaus, F., Hooper, J. N. A., and Kloser, R. (2007). Richness and distribution of sponge megabenthos in continental margin canyons off southeastern Australia. Mar. Ecol. Prog. Ser. 340, 73–88. doi:10.3354/meps340073
Sherman, C. E., Locker, S. D., Webster, J. M., and Weinstein, D. K. (2019). “Geology and Geomorphology 849–878,” in Mesophotic coral ecosystems. Coral reefs of the world. Editors Y. Loya, K. Puglise, and T. Bridge (Cham: Springer), Vol. 12. doi:10.1007/978-3-319-92735-0_44
Sherman, C., Nemeth, M., Ruíz, H., Bejarano, I., Appeldoorn, R., Pagán, F., et al. (2010). Geomorphology and benthic cover of mesophotic coral ecosystems of the upper insular slope of southwest Puerto Rico. Coral Reefs 29, 347–360. doi:10.1007/s00338-010-0607-4
Steller, D. L., and Foster, M. S. (1995). Environmental factors influencing distribution and morphology of rhodoliths in Bahía Concepción, B.C.S., México. J. Exp. Mar. Bio. Ecol. 194, 201–212. doi:10.1016/0022-0981(95)00086-0
Sternberg, R. W., and Nowell, A. R. M. (1999). Continental shelf sedimentology: Scales of investigation define future research opportunities. J. Sea Res. 41 (1), 55–71. doi:10.1016/S1385-1101(98)00037-9
Tazioli, S., Bo, M., Boyer, M., Rotinsulu, H., and Bavestrello, G. (2007). Ecological observations of some common antipatharian corals in the marine park of Bunaken (North Sulawesi, Indonesia). Zool. Stud. 46 (2), 227–241.
Turner, J. A., Babcock, R. C., Hovey, R., and Kendrick, G. A. (2017). Deep thinking: A systematic review of mesophotic coral ecosystems. ICES J. Mar. Sci. 74, 2309–2320. doi:10.1093/icesjms/fsx085
Veech, J. A. (2021). Habitat ecology and analysis, 215pp. USA: Oxford University Press. 978-0-19-882941-6.
Vera, C. S., Vigliarolo, P. K., and Berbery, E. H. (2002). Cold season synoptic-scale waves over subtropical South America. Mon. Weather Rev. 130, 684–699. doi:10.1175/1520-0493(2002)130<0684
Vieira, F. V., Bastos, A. C., Quaresma, V. S., Leite, M. D., Jr, A. C., Oliveira, K. S. S., et al. (2019). Along-shelf changes in mixed carbonate-siliciclastic sedimentation patterns. Cont. Shelf Res. 187, 103964. doi:10.1016/j.csr.2019.103964
Wagner, D., Luck, D. G., and Toonen, R. J. (2012). The biology and ecology of black corals (Cnidaria: Anthozoa: Hexacorallia: Antipatharia). Adv. Mar. Biol. Elsevier Ltd, 1. doi:10.1016/B978-0-12-394282-1.00002-8
Watling, L., and Skinder, C. (2007). Video analysis of megabenthos assemblages in the central Gulf of Maine information from submersible dives. Geol. Assoc. Can. - Special Pap. 368, 359.
Winston, J. E. (2007). Diversity and distribution of bryozoans in the pelican cays, Belize, central America. Atoll Res. Bull. 546, 1. doi:10.5479/si.00775630.546.1
Keywords: marine habitat mapping, shelf geomorphology, shelf-incised valleys, mesophotic habitats, eastern brazilian shelf
Citation: Oliveira Nd, Lavagnino AC, Rocha GA, Moura RLd and Bastos AC (2023) Geomorphological significance of shelf-incised valleys as mesophotic habitats. Front. Remote Sens. 4:1111825. doi: 10.3389/frsen.2023.1111825
Received: 30 November 2022; Accepted: 29 March 2023;
Published: 13 April 2023.
Edited by:
Craig John Brown, Dalhousie University, CanadaReviewed by:
Tim Le Bas, University of Southampton, United KingdomNarelle Maia De Almeida, Federal University of Ceara, Brazil
Copyright © 2023 Oliveira, Lavagnino, Rocha, Moura and Bastos. This is an open-access article distributed under the terms of the Creative Commons Attribution License (CC BY). The use, distribution or reproduction in other forums is permitted, provided the original author(s) and the copyright owner(s) are credited and that the original publication in this journal is cited, in accordance with accepted academic practice. No use, distribution or reproduction is permitted which does not comply with these terms.
*Correspondence: Alex Cardoso Bastos, YWxleC5iYXN0b3NAdWZlcy5icg==