- 1Department of Biology, University of Vermont, Burlington, VT, United States
- 2Redpath Museum and Department of Biology, McGill University, Montreal, QC, Canada
- 3Fundacion Panacetacea, Panama City, Panama
- 4Smithsonian Tropical Research Institute, Panama City, Panama
- 5Institute of Biology, Rio de Janeiro State University, Maracanã, Rio de Janeiro, Brazil
- 6Biology and Conservation of Amazonian Aquatic Mammals (BioMA), Federal Rural University of the Amazon, Belém-Pará, PA, Brazil
- 7Department of Biology, Dalhousie University, Halifax, NS, Canada
Underwater noise from human activities is recognized as a world-wide problem, with important repercussions on the acoustic communication of aquatic mammals. During the COVID-19 pandemic, the government of Panama went into a nationwide lockdown to limit the spread of the virus. This lockdown resulted in the closing of tourism infrastructure and limited mobility in both land and coastal areas. We used this “natural experiment” as an opportunity to study the impact of tour-boat activities on dolphin communication by using passive acoustic monitoring data collected before and during the lockdown at Dolphin Bay, Bocas del Toro, Panama. During the lockdown, tour-boat activity was absent, but boats transporting people and supplies were allowed to circulate. The shift in type of boat activity within the lockdown resulted in lower ambient noise levels and more frequent detections of dolphin sounds. We also detected a more diverse whistle repertoire during the lockdown than in the pre-lockdown period, even when accounting for variation in sample coverage. A Random Forest Analysis classified whistles between the two periods with high accuracy (92.4% accuracy, κ = 0.85) based primarily on whistle modulation and duration. During the lockdown, whistles were longer in duration and less modulated than pre-lockdown. Our study shows that a shift in boat traffic activity can generate significant changes in dolphin habitat, and in their communicative signals, an important consideration given ongoing unregulated ecotourism in the region.
1 Introduction
Underwater noise from human activities is recognized as a world-wide problem (Radford et al., 2014; Williams et al., 2015; André, 2018). Over the past 60 years, ocean ambient noise levels have increased at both low and high frequencies, largely due to an increase in commercial shipping and coastal boat traffic (Hildebrand, 2009; Frisk, 2012). The detrimental impact of increasing noise levels from these human activities have been shown experimentally and in observational studies on the physiology, development, and behavior of a variety of marine taxa (Hawkins & Popper 2017; Erbe et al., 2019).
The impact of anthropogenic noise has been studied extensively in cetaceans. Both baleen and toothed whales respond acoustically to boat noise exposure by adjusting signal emission rate (Guerra et al., 2014). Bottlenose dolphins (Luis et al., 2014), humpback dolphins (Hu et al., 2022), and right whales (Parks et al., 2007a) are reported to decrease mean call rates in the presence of vessels. Changes in signal amplitude and frequency are also reported in various cetacean species. For example, bottlenose dolphins have been shown to increase signal amplitude in the range of 0.1–0.3 dB per 1 dB increase in ambient noise (Kruskal and Wallis, 1952). Adjustments in signal frequency have been reported in several populations of bottlenose dolphins. Under conditions of elevated low and high frequency ambient noise, bottlenose dolphins produce high frequency signals (Churchill et al., 2016) and low frequency signals, respectively (Morisaka et al., 2005; Gospić & Picciulin 2016; Perez-Ortega et al., 2021). Those adjustments can be important to minimize auditory masking (Cunningham & Mountain, 2014), compensate for a reduced communication range (Clark et al., 2009; Erbe et al., 2019), and communicate stress and alertness to conspecifics (Esch et al., 2009; Perez-Ortega et al., 2021).
Dolphins have a diverse repertoire of communicative signals. Among the most studied are narrowband and frequency-modulated tonal sounds called whistles, that can be classified into signature and non-signature whistles based on their function and pattern of emission (Caldwell et al., 1990; Janik and Sayigh, 2013). Signature whistles are stereotypic sounds that encode information about individual identity and, thus, are used as contact calls (Caldwell et al., 1990), whereas non-signature whistles are non-stereotypic sounds produced in a wide range of social contexts (Sayigh et al., 1990; Macfarlane et al., 2017; 2017 Rachinas-Lopes et al., 2017). However, most studies of the impact of anthropogenic noise on dolphin whistles do not take into account whistle function, and limit descriptions to overall changes in frequency, contour complexity, and temporal characteristics (Morisaka et al., 2005a; May-Collado & Wartzok 2008; Perez-Ortega et al., 2021). These changes include lengthening or shortening of whistle duration, increasing or decreasing whistle frequency, and simplification of whistles contours when ship noise increases (Fouda et al., 2018; Morisaka et al., 2005a).
In Latin America and the Caribbean, marine ecotourism has become a major catalyst of the local economy (Tambutti & Gómez, 2020). For example, between 1996 and 2006, boat-based whale watching activities in Latin America grew at a rate three times higher than the rate of world tourism and five times higher than the rate of all Latin American tourism over the same period (Hoyt and Iniguez, 2008). In several countries, the rapid increase in the number of tour-boats and operators occurred without concurrent training for compliance with national whale watching guidelines, inevitably resulting in disturbances in cetacean behavior (New et al., 2015). Although many countries have adopted the International Whaling Commission’s whale watching guidelines (IWC, 1996), enforcement remains limited. As a result, cetaceans in these contexts might be particularly susceptible to altered soundscapes.
A case study of the impact of unregulated whale watching is the bottlenose dolphin (Tursiops truncatus) population of Dolphin Bay, in the archipelago of Bocas del Toro, Panama. These dolphins belong to the coastal ecotype and live in a small and genetically isolated population (Barragán-Barrera et al., 2017). Although dolphins are found throughout the archipelago, Dolphin Bay is their main habitat for foraging and social activities, as a result, the bay has become the hotspot for dolphin watching activities (May-Collado et al., 2012; Kassamali-Fox et al., 2020). When multiple boats are present, interactions with dolphins can be intense due to the lack of compliance with national regulations for approaching tactics and distance (Sitar et al., 2016). During these encounters, dolphins produce whistles with significantly higher modulation than in the absence of boats (Perez-Ortega et al., 2021), potentially indicating changes in their behavioral and ‘emotional’ states (Esch et al., 2009).
In March 2020, during the COVID-19 pandemic, the government of Panama declared a state of emergency and went into a nationwide lockdown to limit the spread of the virus. From March to July 2020, all tourism infrastructure closed, and from August to December 2020, tourism remained minimal due to a combination of curfews and international and national travel restrictions. According to the CEIC Global Economy Database, tourist arrival to Panama after the lockdown remained below 25,000 tourists until February 2021 (CEIC, 2022). Although tour-boat activities were thus restricted, boat presence in Dolphin Bay was permitted for transport of people and supplies during the lockdown. Here we study the impact of unregulated tour-boat activity at Dolphin Bay by comparing the soundscape and dolphin acoustic activity before versus during the COVID-19 lockdowns. We hypothesized that the shift from tour boats to transport boats during most of 2020 resulted in a quieter and subsequently a less stressful habitat for dolphins. Based on our previous work (Perez-Ortega et al., 2021) we predict that a quieter environment will result in an increase in dolphin acoustic detections, a richer and more diverse whistle repertoire, and a decrease in whistle modulation during lockdown.
2 Materials and methods
2.1 Study site
This study took place in Dolphin Bay, located in the archipelago of Bocas del Toro in the western region of Panama. This site consists of coral reef, mangrove, and seagrass habitats (Guzman et al., 2005; Figure 1). The archipelago is home to a small resident population of bottlenose dolphins with high levels of both male and female philopatry (Barragán-Barrera et al., 2017). Resident bottlenose dolphins are primarily found in Dolphin Bay, a semi-enclosed bay that is particularly important for mothers with calves (May-Collado et al., 2014; Do Nascimento et al., 2020). Dolphin presence, activity, and calf presence does not vary seasonally (Sitar et al., 2017). Because of this dolphin population’s high site fidelity, the bay has become a hotspot for dolphin-watching activities occurring throughout the year, with boats arriving every day primarily between 9 a.m. to 12 p.m. (noon), but also in the afternoons depending on the season (Perez-Ortega et al., 2021). The boats used for this purpose are small (typically 10 m in length) and are fitted with one or two engines that can range between 50 and 110 hp (Hu et al., 2022).
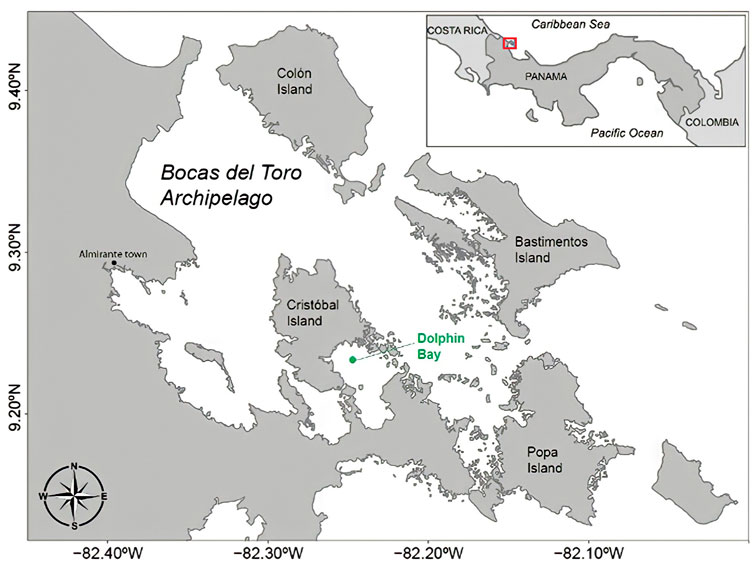
FIGURE 1. Archipelago of Bocas del Toro, Panamá. The green dot shows the location within Dolphin Bay where the autonomous recorders were deployed during pre-lockdown and lockdown periods (latitude and longitude in decimal degrees).
Once a group of dolphins is spotted by the tour operators, the group is often approached by multiple tour boats at distances of 50 m or less and the group is then followed on average for 36 min (SE = 3.6, range: 3–87 min) (Kassamali-Fox et al., 2019). Since boats continue to arrive to the bay, dolphins are cumulatively exposed to these activities for hours. Tour-boat captains often make rapid changes in speed and direction during dolphin encounters, sometimes resulting in separation of group members and mothers from calves (Kassamali-Fox et al., 2020). Although Panama has established national whale-watching guidelines (Resolution N° Dm-0530-2017, 2017), they are not enforced. In addition, efforts by national NGOs to provide boat captains/operators training have proven fruitless due to the high turnover of captains in the area (May-Collado et al., 2018).
2.2 Recordings
Recordings of the soundscape were made using autonomous underwater recorders at a sampling rate of 48 kHz. Recordings from 2017, 2018 and 2019 are herein referred to as “pre-lockdown” and from July to September 2020 as “lockdown.” Details of the recording effort and data used for each analysis is shown in Table 1. In 2017, 2018, 2019, and 2020 autonomous recorders were bottom mounted by attaching the recorder on a pole 1.5 m above the seafloor and anchoring it with a concrete block (approx. 30 kg) at 12 m depth. The site of deployment was located at 9.230°N/-82.246°W (green dot in Figure 1) and it was surrounded by various types of substrates, including a muddy area, seagrass, and a few patches of coral reef (Perez-Ortega et al., 2021). This location is also the area where most of the dolphin-boat interactions occur within the bay (May-Collado pers. obs. 2022). Recordings from 2017 to 2018 were made using the model RUDAR-mk (sampling rate up to 96 kHz -169 dB re:1V/uPa) from Cetacean Research Technology (www.cetaceanresearch.com) and from 2019 to 2020 with a SoundTrap 300 STD (frequency range 20 Hz-150 kHz ±3 dB; self-noise of less than sea-state in the bandwidth 100 Hz-20 kHz, and sensitivity of −203 dB re V/µPa) from Ocean Instruments (http://www.oceaninstruments.co.nz/).
2.3 Ambient noise levels
To calculate broadband ambient noise levels at Dolphin Bay, we used the recordings obtained with the calibrated SoundTrap 300 STD recorder in 2019 (pre-lockdown) and 2020 (lockdown) (Table 1). Broadband ambient noise levels were calculated as the average root-mean-square (RMSdB) by taking the first minute of every hour between 9 a.m. to 12 p.m., when we expected the highest presence of dolphin-watching boats. RMS calculations covered a range of frequencies from 100 to 40 kHz and were done using the acoustic analysis software dBWav from Marshall-Day Acoustics (https://www.marshallday.com). RMS calculations were weighted using NOAA criteria to represent the hearing range of dolphins (NMFS, 2018).
2.4 Dolphin and boat presence
To determine if dolphin and boat detection acoustic rate changed between pre-lockdown (2017-2019) and lockdown (Sprogis et al., 2020) a 10-min sample was manually taken per hour and uploaded to the online platform RFCx ARBIMON (arbimon.rfcx.org). ARBIMON only accepts 1-min long files. Therefore, we uploaded the 10 continuous minutes in separate 1-min files. Using the spectrogram in the Visualizer tool, a spectrogram was created using a Hann window -w parameter, 256 frequency bins -y parameter, 172-time bins per second -x parameter, and 105 dB dynamic range -z parameter. Each 10-min sample was manually annotated in a separate excel file with 1 for the presence of dolphins when at least one of four types of sounds was present: echolocation clicks, buzzes, calls, and whistles; and for boats when broadband noise was present in any point within the 10-min period. A 0 was assigned when dolphins or boats were not detected. It is important to note that there are no other cetacean species inhabiting Dolphin Bay, therefore, we are confident these sounds are coming from bottlenose dolphins only. The presence-absence data matrix was then used to determine the proportion of recordings containing boat and dolphin detections for each hour for pre-lockdown and lockdown.
2.5 Whistle acoustic structure
Tables 1, 2, show the recordings used to search for dolphin whistles and extract contours for frequency and time measurements. To locate whistles, recording files were opened in RAVEN PRO 1.5 build 37 (Center for Conservation Bioacoustics, 2014) and a spectrogram was generated with a fast Fourier transform (FFT) size of 1,024 points, an overlap of 50%, and a 1,024-sample Hann window. Once dolphin whistles were found, we proceeded to manually select whistles for extraction of acoustic characteristics. The selection process was based on the following rules 1) a clear and visible contour from start to end, with a signal-to-noise ratio (SNR) above 6 dB (estimated using the RAVEN SNR protocol); and 2) whistles with different contour modulations were selected using Barzúa-Durán and Au (2002) categorization (constant, upsweep, down sweep, sine, concave, and convex) to maximize representation of the whistle repertoire. Overlapping whistles were selected only if distinguishable from one another. For each whistle, the following acoustic values were extracted: minimum frequency (Hz) (the lowest frequency portion of the selection), maximum frequency (Hz) (the highest frequency portion of the selection), frequency range frequency (Hz) (the difference between maximum and minimum frequency), duration (s) (the difference between begin and end time of the selection), and peak frequency (Hz) (frequency in the contour with greatest energy). To better understand frequency modulation patterns, we also extracted the number of inflection points along the peak frequency contour (PFC Num Inf Pts) (Stặ;nescu et al., 2018). The selection process yielded a total of 1.446 whistles, with 52% from pre-lockdown and 48% from lockdown recordings. It is important to highlight that for this section, whistles were not classified according to their function as described in Section 2.6.

TABLE 2. Recording and analysis effort during pre-lockdown and lockdown periods in Dolphin Bay, Bocas del Toro, Panama (S, signature whistles, NS, non-signature whistles).
2.6 Whistle contours and repertoire
To extract the contours from the fundamental frequency, we used a MATLAB routine called BELUGA (https://synergy.st-andrews.ac.uk/soundanalysis/) using the following settings: a FFT of 4,096 points, frame length of 512, 87.5% overlap between frames, and a Hamming window. For this part of the analysis, we used a higher SNR threshold (above 8 dB), reducing sample size from the 1,446 whistles identified to 524 high quality contours (Table 1). Whistles from 2019 did not have sufficient contour quality for extraction. Selected whistles were classified into signature and non-signature in two ways: 1) using the Signature Identification (SIGID) method by (Janik and Sayigh. 2013). Whistles with more than four repetitions within a 10 s period of highly similar whistle contours were considered signature whistles; and 2) using ARTwarp grouping as described below. Two independent observers reviewed the original recordings from which the 524 whistles were extracted to determine if they were signature or non-signature whistles.
Files were analyzed using ARTwarp (Adaptive Resonance Theory neural network and dynamic time-warping), a whistle categorization software implemented in MATLAB (Clark et al., 2006). ARTwarp uses an unsupervised adaptive resonance theory neural network combined with dynamic time-warping to group the contours into distinct categories. Mammals and birds are more sensitive to changes in the frequency domain than in duration (Dooling, 1982). This means that changes in duration do not affect how these animals perceived acoustic signals. The dynamic time-warping algorithm in ARTwarp allows for contours to be shortened or lengthened to maximize overlap in the frequency domain during each pairwise comparison (Deecke & Janik, 2006). Whistle contours are compared to a set of reference whistle contours using a critical value or ‘vigilance’. If the contour similarity meets the vigilance value, the observed signal is assigned to a reference category, and if it is below the vigilance value, a new reference category is created (Deecke and Janick, 2006). We used a vigilance value of 96%, which has previously been demonstrated to reliably identify signature whistles in bottlenose dolphins (Deecke and Janik, 2006). The analysis was performed for the entire data set and by type of whistle (signature and non-signature) including both periods to examine repertoire overlap. After categorization analysis, if non-signature whistles and signature whistles were assigned to the same category, the original recordings were inspected to verify whistle type. Sometimes, a high-quality signature whistle was produced, with subsequent repetitions that varied due to poor signal-to-noise ratio, resulting in misclassification of signature whistles as non-signatures. Once observers agreed to and were confident of the visual and automated categorization, we proceeded with the final analysis.
3 Statistical analysis
3.1 Characterization of the soundscape
A matched pair Wilcoxon Signed Rank test was used with a 2 tailed sample test (S) to compare RMS and the mean proportion of dolphin and boat detections per hour between pre-lockdown and lockdown periods.
3.2 Characterization of dolphin whistle acoustic structure
Dolphin whistle frequency and duration variables were not normally distributed even after Box-Cox transformation (Shapiro–Wilk Test p < 0.05) (Shapiro and Wilk, 1965). In SPSS version 28, we used a Mann–Whitney U test (Mann & Whitney, 1947) to test for differences in whistle frequency, duration, and modulation (PFC Num Inf Pts) between recordings made during pre-lockdown and lockdown. We used a p < 0.05 as the threshold statistical significance for these analyses.
A Random Forest Analysis (RFA) was used to identify the acoustic variables that contributed most to the classification of whistles into pre-lockdown and lockdown periods. This analysis was fit using the randomForest package in R v. 4.1.3 (Liaw & Wiener, 2002). The approach requires bootstrapped samples of a training data set to create multiple decision trees with the final classification based on a majority vote of the ensemble (Breiman, 1996). A random subsample was taken of the larger dataset (the pre-lockdown period) to match the sample size of the lockdown period to prevent bias in the model. Our dataset was split using the out-of-bag procedure in randomForest, where two-thirds of data is designated as training and one-third as test segments. The model was fine-tuned by determining the optimal number of predictor variables considered at each split, as well as the number of trees using repeated k-fold cross-validation, with the optimal value determined as the model with the largest area under the receiver operating characteristic (ROC) curve. An analysis of Pearson’s correlation coefficients revealed high linear correlation between delta frequency and high frequency (ρ = 0.94). Because previous studies have shown the inclusion of highly correlated variables impacts variable importance weightings in the model (Gregorutti et al., 2017; Barkley et al., 2019), we removed variables from the RFA if the Pearson’s correlation coefficient exceeded ±0.8 as recommended by Barkley et al. (2019). We used the Cohen’s Kappa statistic (Landis & Koch 1977) to evaluate the model performance (Cohen, 1960) which measures the strength of agreement as follows; 0.01–0.20 slight, 0.21–0.40 fair, 0.41–0.60 moderate, 0.61–0.80 substantial, and 0.81–100 nearly perfect. Finally, to determine overall variable importance, we used the mean decrease in accuracy, which measures the extent to which including each variable improves the accuracy of the forest in classification, as well as the mean decrease in Gini, which reflects the average decrease in node impurity (based on Gini Impurity) for each variable.
3.3 Comparison of dolphin whistle repertoires
To determine changes in whistle repertoire diversity between pre-lockdown and lockdown periods, we used the R package iNEXT (Hsieh et al., 2016). To compare whistle repertoire between periods, we based our analysis on sample completeness instead of sample size and linking with diversity. Sample completeness was analyzed by integrating rarefaction and extrapolation (R/E) curves (Chao et al., 2014) of Hill numbers (Hill, 1973), which represent the effective number of species or ‘species equivalents,’ in this case, ARTwarp-generated categories of whistles (Hsieh et al., 2016). A common solution to avoid effects of sample size on diversity estimates is to down-sample the larger sample to reach equal sample size. This down-sampling might not give a fair comparison in communities with differing number of species (in our case, whistle categories) since the difference observed depends on sample size (Chao & Jost, 2012). For this reason, we opted to use sample coverage (equal completeness) to compare the diversity measures of each period. Coverage-based diversity estimates were made using the framework outlined by Chao et al. (2020). In smaller samples, diversity can be inferred from standardized coverage, which can then serve as a fair comparison for diversity estimates. This inference is made up to a maximum, known as Cmax, which reflects the minimum coverage obtained from an extrapolation of double the reference sample (Chao et al., 2020). In this study, the maximum sample coverage for the full repertoire was determined to be at 82.5%. This yielded a repertoire size of 277 extrapolated pre-lockdown samples and 247 interpolated lockdown samples for analysis. These sample sizes were then used to estimate repertoire richness and diversity using Hill numbers (Hsieh et al., 2016), and the process was repeated for the signature and non-signature whistle repertoires. Hill number q = 0 estimates species richness and is particularly sensitive to rare species, Hill number q = 1 is the exponential of Shannon entropy, which equally weights rare and common species, and q = 2 is the inverse of the Simpson concentration index, which describes the expected number of dominant species in an assemblage (Hill, 1973). For purposes of this study, we view distinct whistle categories as the “species” in our populations.
Because under-sampling data can result in unique categories across identical populations by chance alone, we used a permutation test with 1,000 permutations to estimate the expected number of unique whistle categories (full and signature whistle repertoires) from random sampling of a completely shared repertoire. To measure compositional similarity for both richness and diversity, we calculated four community similarity indices using the SpadeR package in R v. 4.1.3 (Chao et al., 2016). Indices were chosen based on representation of each Hill number. The Sorenson and Jaccard indices are derived from Hill number q = 0, and thus are more sensitive to rare categories as they do not account for category abundances (Jost, 2007). The Horn index is derived from Hill number q = 1 and weights both rare and common categories equally. Morisita-Horn index is derived from Hill number q = 2 and is sensitive to common categories (Jost, 2007). Because ambient noise likely impacted the sample size of whistles representing the repertoire of this dolphin population, we incorporated indices that account for both rare and common whistle categories. All statistical analyses were carried out in R v.4.1.2 (Rey-Baquero et al., 2021) and RStudio v.2021.09.0 (RStudio Team, 2021). Finally, a contingency and correspondence analysis were performed in JMP Pro 15.0 (SAS, 2019) to determine if broad contour categories (concave, convex, constant, sine, upsweep, and downsweep) were associated with period.
4 Results
4.1 Dolphin bay’s soundscape
The soundscape of Dolphin Bay differed between pre-lockdown and lockdown periods. Ambient sound levels (RMS) were significantly lower during lockdown (S = 7.24, df = 26, p < 0.0001, Figure 2) than in pre-lockdown. The proportion of dolphin sounds detected was higher during lockdowns than in pre-lockdown (S = −15.252, df = 23, p < 0.0001, Figure 2) but the proportion of recordings with boat sound detections did not differ between the periods (p > 0.05).
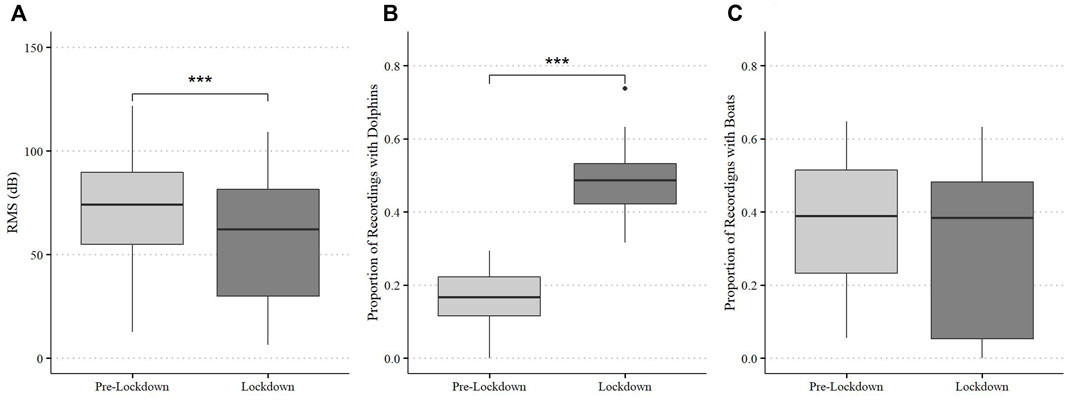
FIGURE 2. Broadband ambient noise levels (RMSdB) (A) and proportion of recordings containing dolphin (B) and boat (C) detections at Dolphin Bay during pre-lockdown and lockdown. Box plots display medians, first and third quartiles, as well as standard error.
4.2 Dolphin whistle acoustic structure
Dolphins in Dolphin Bay during lockdown produced, on average, whistles with higher minimum and maximum frequencies relative to pre-lockdown, (LF: W = 174,280, df = 1, p-value < 0.0001; HF: W = 224,351, df = 1, p-value < 0.0001; PF: W = 233,420, df = 1, p-value < 0.0001, Figure 3) and with lower modulation (PFC Num Inf Pts: W = 347,840, df = 1, p-value < 0.0001 Figure 3) (Supplementary Table S2). Mean frequency range (kHz) and duration (s) did not differ between periods (p > 0.05). Descriptive statistics of dolphin whistle contour frequency and duration by period are provided in Supplementary Table S1.
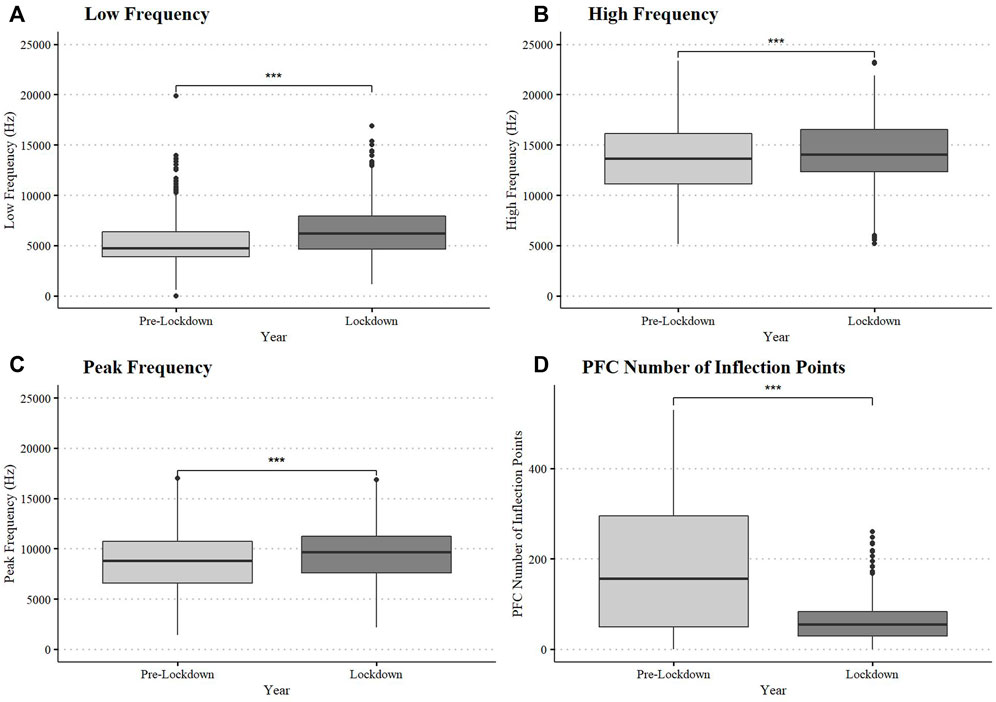
FIGURE 3. Dolphin whistle frequency and modulation variation between pre-lockdown and lockdown. Box plots display medians, first and third quartiles, as well as standard error (A = Low Frequency, B = High Frequency, C = Peak Frequency, and D = Modulation measured as PFC Number of Influection Points).
The RFA classified whistles between periods with an accuracy rate of 92.4% with a kappa statistic of 0.85 (p < 0.0001) indicating near-perfect agreement within the model (Supplementary Table S2). The two whistle variables that contributed the most to the classification model were modulation (PFC number of inflections points) and duration (Table 3). The partial dependence plots suggest that during the lockdown, dolphins produced whistles that were longer and with less inflection points along the frequency contour than in pre-lockdown (Supplementary Figure S1). Because the RFA results on whistle duration contrast those described above, we ran two additional RFAs including only duration and modulation, respectively. These yielded a much lower classification accuracy (55.2%) and kappa statistic (0.1048, p = 0.02) for whistle duration than for whistle modulation (77.4%, kappa = 0.55, p < 0.0001). This suggests that the relationship between duration and other whistle characteristics may play an important role in distinguishing whistles from each period (as in the multi-predictor RFA), despite being relatively similar across periods when considered alone.
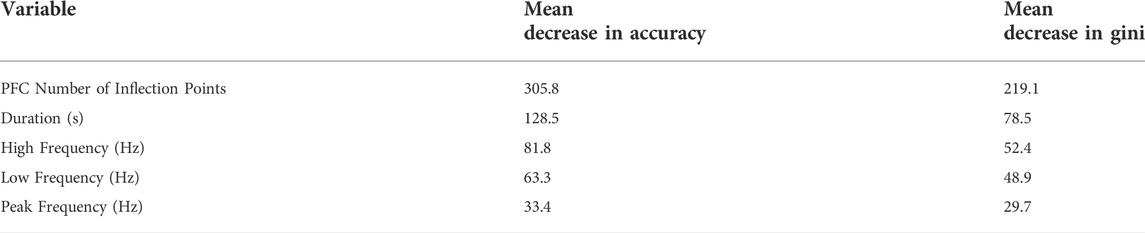
TABLE 3. Variable importance measures from Random Forest Analysis, in descending order. Mean decrease in accuracy measures and mean decrease in Gini effectively describe variable importance.
4.3 Whistle repertoire diversity
Table 4 shows the results of the richness and diversity analysis for the full, signature, and the non-signature whistle repertoires at 82.5%, 90.4%, and 21.5% sample coverage (Cmax), respectively. The number of whistles that were re-classified as “signature whistles” following re-examination were 46 for pre-lockdown and 79 for lockdown. As a result, the sample size for the non-signature whistle repertoire was too small for comparison (n = 44). We then focus on of the full and signature whistle repertoires (Table 2). The full and the signature whistle repertoires were richer and more diverse during lockdown (relative to pre-lockdown), as indicated by the lack of overlap in the 95% confidence intervals (Figure 4). The permutation test showed that the proportion of whistle types for each repertoire unique to either period was higher than expected by chance (full repertoire meanpermuted = 63.2%, signature whistle repertoire meanpermuted = 66.9%). This result aligns with estimates for four compositional similarity indices shown in Table 5.
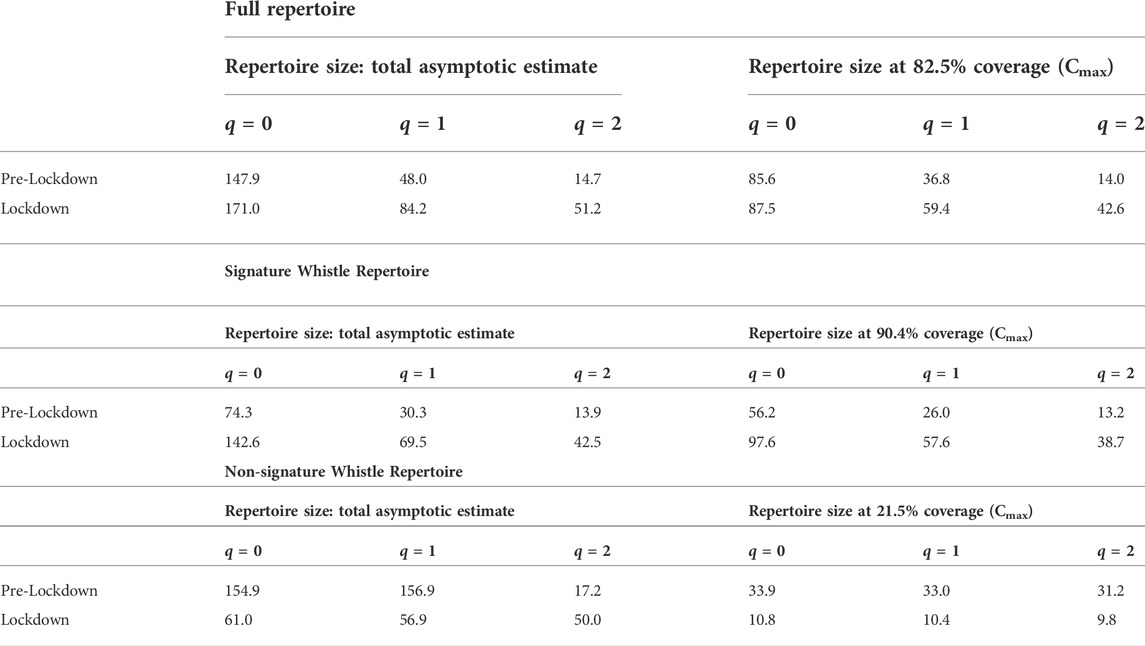
TABLE 4. Asymptotic diversity estimates and estimates at 82.5%, 90.4%, and 21.5% sample coverage for the full repertoire, only signature whistles, and only non-signature whistles, respectively. Values represent effective number of whistles. (q = 0 estimates species richness, q = 1 is the exponential of Shannon entropy, q = 2 is the inverse of the Simpson concentration index).
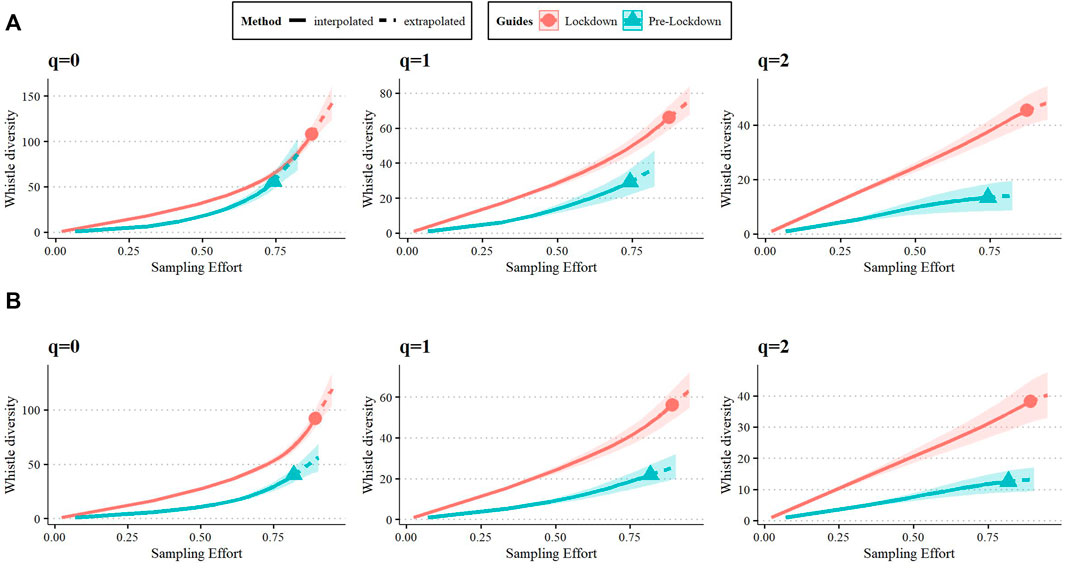
FIGURE 4. Coverage-based rarefaction/extrapolation curve of whistle diversity for each Hill number determined for the full (A) and signature whistle (B). The curve represents both interpolated (solid) and extrapolated (dashed) estimates with a confidence interval of 95%.

TABLE 5. Estimated similarity indices for comparing classic richness-based similarity and whistle relative abundances.
Finally, we found that whistle contour type was significantly associated with the period (χ2= 76.62, df = 5, p < 0.005). Whistles with sine and constant contours were primarily produced in pre-lockdown while a diversity of broad whistle contour types were produced during lockdown (Supplementary Figure S2).
5 Discussion
Comparisons between the pre-lockdown and COVID-19 lockdown periods at Dolphin Bay revealed significant changes in the soundscape, dolphin acoustic detectability, and in dolphin whistle repertoires. These changes highlight the impact that unregulated tour boat activity can have on dolphin habitat and communication.
5.1 Differences in soundscapes and dolphin acoustic presence
As predicted, the shift in boat traffic within Dolphin Bay from primarily tour boats to transport boats resulted in a quieter and likely more favorable acoustic habitat for dolphins (given reduced signal masking). In the pre-lockdown period, tour boats congregate inside the bay, where they follow dolphins at close distances (<50 m) and for long periods (36–87 min) (Sitar et al., 2016; Kassamali-Fox et al., 2020). Maneuvering around the animals involves frequent changes in boat speed (May-Collado and Wartzok 2008; Cranford and Krysl, 2015), an additional contributor to noise which has been shown to impact other dolphin species (Trevorrow et al., 2008; Houghton et al., 2015). Boats are the main mode of transport within the archipelago, and during the pandemic, boat mobilization was allowed to transport people and supplies between islands. This explains the similarity in proportion of boat detections between periods and show how differently transport and tour boat activities noise levels contribute to the soundscape.
During the lockdown, we observed a twofold increase in dolphin acoustic detections in the bay which is likely the result of a combination of factors. These include: 1) a less ‘stressful’ habitat attracting more dolphins. Alternatively, dolphins might just prefer areas with less signal masking. Areas with masking might be stressful but also might just be less preferred, without inducing a significant stress response; 2) an increase in communication range due to lower ambient noise levels resulting in increased detectability from our hydrophones, and 3) an increase in acoustic communication, with dolphins emitting more whistles. Support for an increased communication range was found in Hauraki Gulf in New Zealand during COVID-19 lockdowns. The study found that ambient noise levels dropped nearly threefold, resulting in an increased in dolphin and fish communication ranges to up to 65% (Pine et al., 2021).
5.2 Differences in whistle frequency modulation and duration
We also observed differences in the whistles that dolphins used during the lockdown, compared to the pre-lockdown period. Random Forest Analysis identified the number of inflection points and duration as the variables that contributed the most to the classification of whistles by period. During the lockdown, the number of inflection points along the frequency contour (PFC Num Inf Pts) dropped fourfold. It is important to highlight that this variable is sensitive to background ambient noise levels, and spikes in the contour can occur when overlapping with noise. Throughout our selection of whistles, we tried to minimize this effect by selecting only whistles with high SNR, but it is possible that at least some of the variation in this variable could be a product of noise. However, we did find the most modulated whistles, sines, were more commonly detected during pre-lockdown than in lockdown (Supplementary Figure S2). This result is also supported by the whistle repertoire analysis (see Section 5.3). Changes in whistle modulation, could indicate a change in dolphin behavioral activities and levels of alertness during the lockdown (Caldwell et al., 1990; Janik et al., 1994; Sayigh et al., 1995; Esch et al., 2009; Corrias et al., 2021). Kassamali-Fox et al. (2019) found that dolphins in Dolphin Bay are less likely to stay socializing and foraging in the presence of tour boats, and tend to increase avoidance behaviors (i.e., traveling) as an effect of tour boat presence. Without interference from tour boats, dolphins could have an opportunity to invest more time in social and foraging activities, which could lead to changes in whistle contour. In addition, it is important to highlight that while overall noise levels were reduced, transport boats were still present. A simplification of whistle contours would allow these dolphins to minimize masking from passing transport boats, as simpler whistles propagate better in noisy environments (Lesage et al., 1999; Fouda et al., 2018). This is supported by our previous work in the archipelago, where we have documented dolphin producing simpler whistle contours in areas where transport boats are more common than tour boats (Perez-Ortega et al., 2021).
Whistle duration was the second-most important factor when classifying whistles by period. Classification studies of dolphin species have found whistle duration as an important contributor to the classification model (Hernandez et al., 2010). We found that shorter whistles were more likely to be classified to pre-lockdown than to the lockdown period. In two previous studies, dolphins in Dolphin Bay were reported to whistle on average 30 ms longer in the presence of tour boat than when found in areas dominated by transport boats (0.96 ± 0.71 vs 0.63 ± 0.55 in Perez-Ortega et al., 2021) and when only the research boat was present (1.12 ± 0.58 vs. 0.88 ± 0.58 in May-Collado and Wartzok, 2008). Previous studies have associated whistle duration with context and group size (Janik et al., 1994; Wang et al., 1995; Morisaka et al., 2005b). For example, in Croatia and Sardinia bottlenose dolphins increased whistle duration with increasing group size regardless of boat traffic (La Manna et al., 2020). Unfortunately, we do not have data on the number of dolphins present at Dolphin Bay during the lockdown. Therefore, we are unable to determine if the increase in whistle duration during lockdown is the result of larger group sizes (but see Section 5.3).
5.3 Differences in whistle repertoire
Overall, we found that differences in whistle repertoires between periods were driven by dolphin signature whistle diversity during the lockdown. Signature whistles broadcast an individual identity (Caldwell et al., 1990) and are used to mediate dolphin group formation and activity patterns (Wang et al., 1995; Azevedo and Van Sluys, 2005; Hawkins, 2010). A more diverse signature whistle repertoire during the lockdown could suggest an increase in dolphin presence. Because Dolphin Bay is a critical habitat for dolphins (May-Collado et al., 2017; Kassamali-Fox et al., 2020), it is possible that in the absence of tour boats, more groups were present resulting in a richer repertoire (Melos-Santos et al., 2019). In addition, the permutation test and similarity indices revealed that different signature whistles were detected during the lockdown, suggesting the possible presence of “new” dolphins within the bay. The archipelago of Bocas del Toro’s dolphin population is estimated to be around 100-150 that vary in their level of residency. Of these 48 dolphins are regularly seen inside Dolphin Bay (May-Collado et al., 2018). The presence of distinct signature whistles during the lockdown could be due to dolphins from other parts of the archipelago entering the bay, new calves, or a combination of both. These results further highlight the importance of distinguishing between non-signature and signature whistles when comparing dolphin whistle repertoires. Signature whistles often contain segments that are repeated, and the number of repeats can vary between bouts due to context (Janik et al., 1994), and ARTwarp might classify them into separate whistle categories, significantly impacting the estimates of repertoire size and diversity. Although we selected whistles with high SNR and used estimation techniques that control for differences in sample size, it is still possible that we underestimated the repertoire size during the pre-lockdown.
6 Conclusion
The shift in boat activity within Dolphin Bay during the COVID-19 lockdown allowed us to study what happens when tour boats are eliminated from dolphin habitats. Our results reveal a clear change in ambient noise levels, as well as in proportion of dolphin detections, and their whistle acoustic structure and diversity during the lockdown. As lockdown restrictions are lifted, tour boat presence is expected to grow in Dolphin Bay. In addition, the only airport in the archipelago is being expanded to accommodate more flights, and this development will inevitably result in a higher demand for tour boat activities. The lack of resources to enforce whale-watching regulation and the prospect of an even higher presence of tour boats at Dolphin Bay paint a difficult scenario for this small bottlenose dolphin population. This study shows the substantial impacts of unregulated boat-based whale watching activities on dolphin acoustic habitats and behavior, an important consideration for mitigation efforts and government investment in the enforcement of whale-watching regulations.
Data availability statement
The raw data supporting the conclusion of this article will be made available by the authors upon request, without undue reservation.
Ethics statement
The animal study was reviewed and approved by AICUC #2019-0201-2022 protocol was approved by Smithsonian Tropical Research Institute, Panama.
Author contributions
Experimental design was conceived by EG and LJM-C. Funding for recorders and deployments were obtained by AH, LJM-C, and BP-O. BP-O and LJM-C coordinated recorder deployment. EG, MA, and LJM-C processed recordings and performed statistical analyses. MR-C provide script assistance for random forest analysis. SFW, GMS, and MR-C provided the analytical basis and helped run the analysis of whistle repertoire diversity. EG and LJM-C wrote the first drafts of the manuscripts and all authors revised and contributed to the draft manuscript and approved its submission.
Funding
Fund for this project came from Rufford Small Grants, the ROC Grant from Waitt Foundation, SENACYT of Panama, Smithsonian Tropical Research Institute (STRI), Panacetacea.org, Conservation International Costa Rica, Society for Marine Mammalogy Travel Grant, Hendry Lab, Redpath Museum Class 66 Award, Biology Department travel grant, Neotropical Environment Option (NEO) and Biodiversity, Ecosystem Services and Sustainability (BESS) program of McGill University, and May-Collado Lab.
Acknowledgments
Special thanks to Rachel Collin, Urania Gonzalez, Plinio Gondola, and Gilberto Murray for their logistic support at the Smithsonian Bocas del Toro Field Research Station; and Demetrio Georget, Davis González, Cynthia Peña, Arcadio Castillo, Chelina Batista, and Maddy Tregenza for help in field work and data collection. We would also like to thank Gloria Keough, Logan Hillger, and Emma Shapera for data collection assistance. Dr. James Murdoch and Dr. J. Ellen Marsden provided editorial comments and feedback that significantly improved this manuscript. Data was collected under the scientific permit No. SE/A-75-17, SE/A-90-18, and SE/A-13-2020 from the Environment Ministry of Panama.
Conflict of interest
The authors declare that the research was conducted in the absence of any commercial or financial relationships that could be construed as a potential conflict of interest.
Publisher’s note
All claims expressed in this article are solely those of the authors and do not necessarily represent those of their affiliated organizations, or those of the publisher, the editors and the reviewers. Any product that may be evaluated in this article, or claim that may be made by its manufacturer, is not guaranteed or endorsed by the publisher.
Supplementary material
The Supplementary Material for this article can be found online at: https://www.frontiersin.org/articles/10.3389/frsen.2022.934608/full#supplementary-material
References
André, M. (2018). Ocean noise: Making sense of sounds. Soc. Sci. Inf. 57 (3), 483. doi:10.1177/0539018418793052
Azevedo, A. F., and Van Sluys, M. (2005). Whistles of tucuxi dolphins (Sotalia fluviatilis) in Brazil: Comparisons among populations. J. Acoust. Soc. Am. 117 (3), 1456–1464. doi:10.1121/1.1859232
Barkley, Y., Oleson, E. M., Oswald, J. N., and Franklin, E. C. (2019). Whistle classification of sympatric false killer whale populations in Hawaiian waters yields low accuracy rates. Front. Mar. Sci. 645, 1–5. doi:10.3389/fmars.2019.00645
Barragán-Barrera, D. C., May-Collado, L. J., Tezanos-Pinto, G., Islas-Villanueva, V., Correa Cárdenas, C. A., Caballero, S., et al. (2017). High genetic structure and low mitochondrial diversity in bottlenose dolphins of the archipelago of Bocas del Toro, Panama: A population at risk? PloS One 12 (12), e0189370. doi:10.1371/journal.pone.0189370
Caldwell, M. C., Caldwell, D. K., and Tyack, P. L. (1990). “Review of the signature whistle hypothesis for the Atlantic bottlenose dolphin,” in The Bottlenose Dolphin. Editor Leatherwood, S., and Reeves, R. R. (San Diego: Academic Press), 199–234.
CEIC (2022). Available at: https://www.ceicdata.com/en/indicator/panama/visitor-arrivals.
Center for Conservation Bioacoustics (2014). Raven Pro: Interactive sound analysis software. Ithaca, NY, USA: The Cornell Lab of Ornithology. [Computer software]Available at http://ravensoundsoftware.com/.
Chao, A., Gotelli, N. J., Hsieh, T. C., Sander, E. L., Ma, K. H., Colwell, R. K., et al. (2014). Rarefaction and extrapolation with Hill numbers: A framework for sampling and estimation in species diversity studies. Ecol. Monogr. 84 (1), 45–67. doi:10.1890/13-0133.1
Chao, A., and Jost, L. (2012). Coverage‐based rarefaction and extrapolation: Standardizing samples by completeness rather than size. Ecology 93 (12), 2533–2547. doi:10.1890/11-1952.1
Chao, A., Kubota, Y., Zelený, D., Chiu, C. H., Li, C. F., Kusumoto, B., et al. (2020). Quantifying sample completeness and comparing diversities among assemblages. Ecol. Res. 35 (2), 292–314. doi:10.1111/1440-1703.12102
Chao, A., Ma, K. H., Hsieh, T. C., and Chiu, C. H. (2016). SpadeR (Species-richness prediction and diversity estimation in R): an R package. in CRAN. Program and User’s Guide also published atAvailable at; http://chao.stat.nthu.edu.tw/wordpress/software_download/.
Churchill, M., Martinez-Caceres, M., de Muizon, C., Mnieckowski, J., and Geisler, J. H. (2016). The origin of high-frequency hearing in whales. Curr. Biol. 26 (16), 2144–2149. doi:10.1016/j.cub.2016.06.004
Clark, C. W., Ellison, W. T., Southall, B. L., Hatch, L., Van Parijs, S. M., Frankel, A., et al. (2009). Acoustic masking in marine ecosystems: Intuitions, analysis, and implication. Mar. Ecol. Prog. Ser. 395, 201–222. doi:10.3354/meps08402
Clark, L. S., Cowan, D. F., and Pfeiffer, D. C. (2006). Morphological changes in the Atlantic bottlenose dolphin (Tursiops truncatus) adrenal gland associated with chronic stress. J. Comp. Pathology 135 (4), 208–216. doi:10.1016/j.jcpa.2006.07.005
Cohen, J. (1960). A coefficient of agreement for nominal scales. Educ. Psychol. Meas. 20, 37–46. doi:10.1177/001316446002000104
Corrias, V., de Vincenzi, G., Ceraulo, M., Sciacca, V., Sala, A., de Lucia, G. A., et al. (2021). Bottlenose dolphin (Tursiops truncatus) whistle modulation during a trawl bycatch event in the adriatic sea. Animals 11 (12), 3593. doi:10.3390/ani11123593
Cranford, T. W., and Krysl, P. (2015). Fin whale sound reception mechanisms: Skull vibration enables low-frequency hearing. PloS One 10 (1), e0116222. doi:10.1371/journal.pone.0116222
Cunningham, K. A., and Mountain, D. C. (2014). Simulated masking of right whale sounds by shipping noise: Incorporating a model of the auditory periphery. J. Acoust. Soc. Am. 135, 1632–1640. doi:10.1121/1.4864470
Deecke, V. B., and Janik, V. M. (2006). Automated categorization of bioacoustic signals: Avoiding perceptual pitfalls. J. Acoust. Soc. Am. 117 (4), 2470. doi:10.1121/1.4787454
Do Nascimento, L. A., Campos-Cerqueira, M., and Beard, K. H. (2020). Acoustic metrics predict habitat type and vegetation structure in the Amazon. Ecol. Indic. 117, 106679. doi:10.1016/j.ecolind.2020.106679
Dooling, R. J. (1982). Auditory perception in birds. Acoust. Commun. birds 1, 95–130. doi:10.1016/b978-0-08-092416-8.50013-9
Erbe, C., Marley, S. A., Schoeman, R. P., Smith, J. N., Trigg, L. E., Embling, C. B., et al. (2019). The effects of ship noise on marine mammals—A review. Front. Mar. Sci. 6, 606. doi:10.3389/fmars.2019.00606
Esch, H. C., Sayigh, L. S., Blum, J. E., and Wells, R. S. (2009). Whistles as potential indicators of stress in bottlenose dolphins (Tursiops truncatus). J. Mammal. 90 (3), 638–650. doi:10.1644/08-mamm-a-069r.1
Fouda, L., Wingfield, J. E., Fandel, A. D., Garrod, A., Hodge, K. B., Rice, A. N., et al. (2018). Dolphins simplify their vocal calls in response to increased ambient noise. Biol. Lett. 14 (10), 20180484. doi:10.1098/rsbl.2018.0484
Freeberg, T. M., Dunbar, R. I., and Ord, T. J. (2012). Social complexity as a proximate and ultimate factor in communicative complexity. Phil. Trans. R. Soc. B 367 (1597), 1785–1801. doi:10.1098/rstb.2011.0213
Frisk, G. V. (2012). Noiseonomics: The relationship between ambient noise levels in the sea and global economic trends. Sci. Rep. 2 (1), 437. doi:10.1038/srep00437
Gospić, N. R., and Picciulin, M. (2016). Changes in whistle structure of resident bottlenose dolphins in relation to underwater noise and boat traffic. Mar. Pollut. Bull. 105 (1), 193–198. doi:10.1016/j.marpolbul.2016.02.030
Gregorutti, B., Michel, B., and Saint-Pierre, P. (2017). Correlation and variable importance in random forests. Stat. Comput. 27, 659–678. doi:10.1007/s11222-016-9646-1
Guerra, M., Dawson, S. M., Brough, T. E., and Rayment, W. J. (2014). Effects of boats on the surface and acoustic behaviour of an endangered population of bottlenose dolphins. Endanger. Species Res. 24 (3), 221–236. doi:10.3354/esr00598
Guzman, H. M., Barnes, P. A., Lovelock, C. E., and Feller, I. C. (2005). A site description of the CARICOMP mangrove, seagrass and coral reef sites in Bocas del Toro, Panama. Caribb. J. Sci. 43 (3), 430–440.
Hawkins, A. D., and Popper, A. N. (2017). A sound approach to assessing the impact of underwater noise on marine fishes and invertebrates. ICES J. Mar. Sci. 74 (3), 635–651. doi:10.1093/icesjms/fsw205
Hawkins, E. R. (2010). Geographic variations in the whistles of bottlenose dolphins (Tursiops aduncus) along the east and west coasts of Australia. J. Acoust. Soc. Am. 128 (2), 924–935. doi:10.1121/1.3459837
Hernandez, E. N., Solangi, M., and Kuczaj, S. A. (2010). Time and frequency parameters of bottlenose dolphin whistles as predictors of surface behavior in the Mississippi Sound. J. Acoust. Soc. Am. 127 (5), 3232–3238. doi:10.1121/1.3365254
Hildebrand, J. (2009). Anthropogenic and natural sources of ambient noise in the ocean. Mar. Ecol. Prog. Ser. 395, 5–20. doi:10.3354/meps08353
Hill, M. O. (1973). Diversity and evenness: A unifying notation and its consequences. Ecology 54 (2), 427–432. doi:10.2307/1934352
Houghton, J., Holt, M. M., Giles, D., A., Hanson, M. B., Emmons, C. K., Hogan, J., T., et al. (2015). The relationships between vessel traffic and noise levels received by killer whales (Orcinus orca). PloS One 10 (12), e0140119. doi:10.1371/journal.pone.0140119
Hoyt, E., and Iñíguez, M. (2008). The state of whale watching in Latin America. Chippenham, Uk: Wdcs.
Hsieh, T. C., Ma, K. H., and Chao, A. (2016). iNEXT: an R package for rarefaction and extrapolation of species diversity (Hill numbers). Methods Ecol. Evol. 7 (12), 1451–1456. doi:10.1111/2041-210x.12613
Hu, W. C., Siddagangaiah, S., Chen, C. F., and Pieretti, N. (2022). Impact of vessel transit on vocalizations of the Taiwanese humpback dolphin. Diversity 14 (426), 426. doi:10.3390/d14060426
Janik, V. M., and Sayigh, L. S. (2013). Communication in bottlenose dolphins: 50 years of signature whistle research. J. Comp. Physiol. A 199 (6), 479–489. doi:10.1007/s00359-013-0817-7
Janik, V. M., Todt, D., and Dehnhardt, G. (1994). Signature whistle variations in a bottlenosed dolphin, Tursiops truncatus. Behav. Ecol. Sociobiol. 35 (4), 243–248. doi:10.1007/bf00170704
Jost, L. (2007). Partitioning diversity into independent alpha and beta components. Ecology 88 (10), 2427–2439. doi:10.1890/06-1736.1
Kassamali-Fox, A., Christiansen, F., May-Collado, L. J., Ramos, E. A., and Kaplin, B. A. (2020). Tour boats affect the activity patterns of bottlenose dolphins (Tursiops truncatus) in Bocas del Toro, Panama. PeerJ 8, e8804. doi:10.7717/peerj.8804
Kruskal, W. H., and Wallis, W. A. (1952). Use of ranks in one-criterion variance analysis. J. Am. Stat. Assoc. 47, 583–621. doi:10.1080/01621459.1952.10483441
La Manna, G., Rako‐Gospić, N., Sarà, G., Gatti, F., Bonizzoni, S., Ceccherelli, G., et al. (2020). Whistle variation in Mediterranean common bottlenose dolphin: The role of geographical, anthropogenic, social, and behavioral factors. Ecol. Evol. 10 (4), 1971–1987. doi:10.1002/ece3.6029
Landis, J. R., and Koch, G. G. (1977). The measurement of observer agreement for categorical data. Washingtong D.C: International Biometric Society, 159–174.
Lesage, V., Barrette, C., Kingsley, M. C., and Sjare, B. (1999). The effect of vessel noise on the vocal behavior of belugas in the St. Lawrence River estuary, Canada. Mar. Mamm. Sci. 15 (1), 65–84. doi:10.1111/j.1748-7692.1999.tb00782.x
Liaw, A., and Wiener, M. (2002). Classification and regression by randomForest. R. news 2 (3), 18–22.
Luís, A. R., Couchinho, M. N., and dos Santos, M. E. (2014). Changes in the acoustic behavior of resident bottlenose dolphins near operating vessels. Mar. Mamm. Sci. 30 (4), 1417–1426. doi:10.1111/mms.12125
Macfarlane, N., Janik, V., Jensen, F. H., McHugh, K., Sayigh, L., and Wells, R. (2017). Signature whistles facilitate reunions and/or advertise identity in bottlenose dolphins. The Journal of the Acoustical Society of America 141, 3543. doi:10.1121/1.4987492
May-Collado, L. J., Amador-Caballero, M., Casas, J. J., Gamboa-Poveda, M. P., Garita-Alpízar, F., Gerrodette, T., and Rodríguez-Fonseca, J. (2018). “Ecology and conservation of cetaceans of Costa Rica and Panama,” in Advances in marine vertebrate research in Latin America (Cham: Springer), 293–319.
May-Collado, L. J., Barragán-Barrera, D. C., Quiñones-Lebrón, S. G., and Aquino Reynoso, W. (2012). Dolphin watching boats impact on habitat use and communication of bottlenose dolphins in Bocas del Toro. Panama Dur. 2004, 2006–2010. Report Presented to the Scientific Committee of the International Whaling Commission SC/64/WW2.
May-Collado, L. J., Quiñones-Lebrón, S. G., Barragán-Barrera, D. C., Palacios, J. D., and Gamboa Poveda, M. (2014). The dolphin watching industry of Bocas del Toro continues impacting the resident bottlenose dolphin population. Int. Whal. Com.
May-Collado, L. J., and Wartzok, D. (2008). A comparison of bottlenose dolphin whistles in the atlantic ocean: Factors promoting whistle variation. J. Mammal. 89 (5), 1229–1240. doi:10.1644/07-mamm-a-310.1
Melo-Santos, G., Rodrigues, A. L. F., Tardin, R. H., de Sá Maciel, I., Marmontel, M., Da Silva, M. L., et al. (2019). The newly described Araguaian river dolphins, Inia araguaiaensis (Cetartiodactyla, Iniidae), produce a diverse repertoire of acoustic signals. PeerJ 7, e6670. doi:10.7717/peerj.6670
Morisaka, T., Shinohara, M., Nakahara, F., and Akamatsu, T. (2005a). Effects of ambient noise on the whistles of Indo-Pacific bottlenose dolphin populations. J. Mammal. 86 (3), 541–546. doi:10.1644/1545-1542(2005)86[541:eoanot]2.0.co;2
Morisaka, T., Shinohara, M., Nakahara, F., and Akamatsu, T. (2005b). Geographic variations in the whistles among three Indo-Pacific bottlenose dolphin Tursiops aduncus populations in Japan. Fish. Sci. 71 (3), 568–576. doi:10.1111/j.1444-2906.2005.01001.x
New, L. F., Hall, A. J., Harcourt, R., Kaufman, G., Parsons, E. C. M., Pearson, H. C., et al. (2015). The modelling and assessment of whale-watching impacts. Ocean Coast. Manag. 115, 10–16. doi:10.1016/j.ocecoaman.2015.04.006
Parks, S. E., Clark, C. W., and Tyack, P. L. (2007a). Short- and long-term changes in right whale calling behavior: The potential effects of noise on acoustic communication. J. Acoust. Soc. Am. 122, 3725–3731. doi:10.1121/1.2799904
Perez-Ortega, B., Daw, R., Paradee, B., Gimbrere, E., and May-Collado, L. J. (2021). Dolphin watching boats affect whistle frequency modulation in bottlenose dolphins. Front. Mar. Sci. 8, 102. doi:10.3389/fmars.2021.618420
Pieretti, N., Farina, A., and Morri, D. (2011). A new methodology to infer the singing activity of an avian community: The Acoustic Complexity Index (ACI). Ecol. Indic. 11 (3), 868–873. doi:10.1016/j.ecolind.2010.11.005
Pine, M. K., Wilson, L., Jeffs, A. G., McWhinnie, L., Juanes, F., Scuderi, A., et al. (2021). A Gulf in lockdown: How an enforced ban on recreational vessels increased dolphin and fish communication ranges. Glob. Chang. Biol. 27 (19), 4839–4848. doi:10.1111/gcb.15798
Rachinas-Lopes, P., Luis, A. R., Borges, A. S., Neto, M., and dos Santos, M. E. (2017). Whistle stability and variation in captive bottlenose dolphins (Tursiops truncatus) recorded in isolation and social contexts. Aquat. Mamm. 43 (1)–13. doi:10.1578/am.43.1.2017.1
Radford, A. N., Kerridge, E., and Simpson, S. D. (2014). Acoustic communication in a noisy world: Can fish compete with anthropogenic noise. Behav. Ecol. 25 (5), 1022–1030. doi:10.1093/beheco/aru029
Rey-Baquero, M. P., Huertas-Amaya, L. V., Seger, K. D., Botero-Acosta, N., Luna Acosta, A., Perazio, C. E., et al. (2021). Understanding effects of whale-watching vessel noise on humpback whale song in the north pacific coast of Colombia with propagation models of masking and acoustic data observations. Front. Mar. Sci. 182. doi:10.3389/fmars.2021.623724
Sayigh, L. S., Tyack, P. L., Wells, R. S., Scott, M. D., and Irvine, A. B. (1995). Sex difference in signature whistle production of free-ranging bottlenose dolphins, Tursiops truncates. Behav. Ecol. Sociobiol. 36 (3), 171–177. doi:10.1007/bf00177793
Sayigh, L. S., Tyack, P. L., Wells, R. S., and Scott, M. D. (1990). Signature whistles of free-ranging bottlenose dolphins Tursiops truncatus: Stability and mother-offspring comparisons. Behav. Ecol. Sociobiol. 26 (4), 247–260. doi:10.1007/bf00178318
Sitar, A., May-Collado, L. J., Wright, A. J., Peters-Burton, E., Rockwood, L., Parsons, E. C. M., et al. (2016). Boat operators in Bocas del Toro, Panama display low levels of compliance with national whale-watching regulations. Mar. Policy 68, 221–228. doi:10.1016/j.marpol.2016.03.011
Sitar, A., May-Collado, L. J., Wright, A., Peters-Burton, E., Rockwood, L., Parsons, E. C. M., et al. (2017). Tourists' perspectives on dolphin watching in Bocas del Toro, Panama. Tour. Mar. Environ. 12 (2), 79–94. doi:10.3727/154427316x14820977775343
Sprogis, K. R., Videsen, S., and Madsen, P. T. (2020). Vessel noise levels drive behavioural responses of humpback whales with implications for whale-watching. Elife 9, e56760. doi:10.7554/elife.56760
Tambutti, M., and Gómez, J. J. (2020). The outlook for oceans, seas and marine resources in Latin America and the Caribbean: Conservation, sustainable development and climate change mitigation. America: ECLAC.
Trejos-Lasso, L., and May-Collado, L. J. (2015). Bottlenose dolphins Tursiops truncatus strandings in Bocas del Toro caused by boat strikes and fishing entanglement. Int. Whal. Comm., 6. SC/66a/WW7.
Trevorrow, M. V., Vasiliev, B., and Vagle, S. (2008). Directionality and maneuvering effects on a surface ship underwater acoustic signature. J. Acoust. Soc. Am. 124 (767)–778. doi:10.1121/1.2939128
Wang, D. W., Wursig, B., and Evans, W. E. (1995). Whistles of bottlenose dolphins: Comparisons among populations. Aquat. Mamm. 21, 65–77.
Williams, R., Wright, A. J., Ashe, E., Blight, L. K., Bruintjes, R., Canessa, R., et al. (2015). Impacts of anthropogenic noise on marine life: Publication patterns, new discoveries, and future directions in research and management. Ocean Coast. Manag. 115, 17–24. doi:10.1016/j.ocecoaman.2015.05.021
Keywords: repertoire, boat traffic, whale-watching, anthropogenic noise, signal masking, whistles
Citation: Gagne E, Perez-Ortega B, Hendry AP, Melo-Santos G, Walmsley SF, Rege-Colt M, Austin M and May-Collado LJ (2022) Dolphin communication during widespread systematic noise reduction-a natural experiment amid COVID-19 lockdowns. Front. Remote Sens. 3:934608. doi: 10.3389/frsen.2022.934608
Received: 02 May 2022; Accepted: 04 July 2022;
Published: 17 August 2022.
Edited by:
Marc Lammers, Hawaiian Islands Humpback Whale National Marine Sanctuary, United StatesReviewed by:
Luane Stamatto Ferreira, Federal University of Rio Grande do Norte, BrazilZhitao Wang, Institute of Hydrobiology (CAS), China
Copyright © 2022 Gagne, Perez-Ortega, Hendry, Melo-Santos, Walmsley, Rege-Colt, Austin and May-Collado. This is an open-access article distributed under the terms of the Creative Commons Attribution License (CC BY). The use, distribution or reproduction in other forums is permitted, provided the original author(s) and the copyright owner(s) are credited and that the original publication in this journal is cited, in accordance with accepted academic practice. No use, distribution or reproduction is permitted which does not comply with these terms.
*Correspondence: Laura J. May-Collado, laura.may-collado@uvm.edu
†These authors have contributed equally to this work and share first authorship