- Department of Civil Engineering, American University of Sharjah, Sharjah, United Arab Emirates
Introduction: There has been a significant increase in global energy usage due to urbanization and population growth. The built environment is responsible for over one third of global energy consumption, carbon dioxide emissions, and over a quarter of greenhouse gas emissions. The United Arab Emirates building, and construction sector consumes 70% of the entire country’s electricity demand and uses large amounts of raw materials, accelerating resource scarcity. The tiny house offers several environmental and sustainability benefits that have contributed to its growing popularity.
Methods: In this study, the environmental impacts of a tiny house, built on the American University of Sharjah campus will be evaluated using life cycle impact assessment. A cradle-to-grave evaluation was conducted for the life cycle assessment of the tiny house, with a functional unit of 1 m2, using the ReCiPe 2016 V1.03 midpoint method.
Results: The environmental impact associated with the production and operational phases was most severe, aligning with similar studies on residential buildings. In the material production phase, concrete and steel had the most significant environmental impacts, particularly in the climate change category. These results highlight the importance of focusing on sustainable innovations in material production and recycling to mitigate environmental impacts. The operational phase contributed to approximately 77% of the total carbon dioxide emissions over the 50-year lifespan of the tiny house, primarily due to energy consumption for heating and cooling.
Discussion: While the findings align with previous studies on residential buildings, it is important to consider the context of a tiny house; its small size results in a significantly lower overall environmental impact compared to larger homes.
1 Introduction
There has been a significant increase in global energy usage due to urbanization. The built environment is responsible for over 40% of global energy consumption, 36% of carbon dioxide (CO2) emissions, and 28% of greenhouses gas (GHG) emissions (Rauf et al., 2022). In 2017, residential buildings contributed to more than 70% of the world’s total energy demand, and it is projected to increase by 50% by the year 2050 (Crawford and Stephan, 2020; Rauf et al., 2022). In addition, the rapid growth in population and residential areas has led to a rise in energy demand, despite the implementation of energy efficiency practices (Crawford and Stephan, 2020). According to Lin et al. (2018) and Rauf et al. (2022) the United Arab Emirates (UAE) building, and construction sector consumes 70% of the entire country’s electricity demand, predominantly for cooling purposes. There is a strong relationship between energy consumption and GHG emissions, indicating high-energy consumptions result in adverse environmental impacts (Jin and Kim, 2018; Coolen et al., 2022). The building sector in the UAE also consumes large amounts of materials, which has contributed to resource scarcity and caused the construction industry to produce 75% of the country’s waste (Al-Hajj and Hamani, 2011). Resource scarcity is a critical issue, particularly in the construction sector, as resources are currently being used at a rate 50% faster than they can be replenished (Cruz Rios et al., 2019). The demand for materials like iron ore and steel are continuously increasing, so resource scarcity is anticipated to cause high material and energy prices (Cruz Rios et al., 2019). By 2030, half of the global population could experience water scarcity, a vital resource for the mining and manufacturing of steel, concrete, plastics, and ceramics (Cruz Rios et al., 2019). For this reason, it is important for the UAE to reduce the energy consumption of its built environment.
In the UAE, the construction industry is a leading sector that drives the country’s economy (Rahman et al., 2022). Due to the rapid urbanization of the UAE, many major cities such as Abu Dhabi, Dubai, and Sharjah have experienced a large increase in resource consumption (Sadar Din and Ishak, 2024). This has not only impacted the country’s climate, urban heat island, and water and energy resources, but has also increased social costs related to desalination, carbon, and power (Rauf et al., 2023). In the year 2020, residential buildings had the second highest energy consumption in the UAE, responsible for consuming 32.8% of the country’s total energy usage (Rauf et al., 2022). The UAE aims to reduce energy emissions and related emissions by the year 2030, to help achieve their sustainable development goals (SDGs) in line with the United Nations agenda (Rauf et al., 2023). In order to minimize energy footprint and other environmental impacts of residential buildings, operational energy and embodied energy must be reduced (Mourão et al., 2019). Residential projects are expected to account for 21% of UAE’s construction projects in the year 2024 (Morgan, 2024). According to Morgan (2024), the city of Dubai is projected to deliver 35,000 residential units in the year 2024. Numerous studies have shown that average operational energy of a single villa in the UAE can reach as high as 273.36 kW/m2/yr (Giusti and Almoosawi, 2017; Al-Alili and Al Qubaisi, 2018; Abu-Hijleh and Jaheen, 2019; Bande et al., 2020). Other studies have demonstrated the correlation between the area of a house and its operational energy use. A study by Clune et al. (2012) found that the dimension of a house has a significant impact on the capacity of residential building codes to reduce emissions. Additionally, another research paper found that building characteristics, such as household size and income, account for a 42% variation in energy use for heating (Guerra Santin et al., 2009). Furthermore, another study concluded that housing size is a major factor in determining life-cycle energy use (Fuller and Crawford, 2011). Therefore, given the strong influence of housing size on energy usage, small homes present an opportunity to reduce global energy consumption and GHG emissions.
Research on developing energy-efficient residential spaces in the UAE has become increasingly prevalent, driven by current efforts to implement sustainable practices in the country’s built environment (Abdulmaksoud and Beheiry, 2023). A recent study by Rauf et al. (2024) conducted a life cycle embodied water assessment of a 532 m2 two-story case study concrete villa in the UAE. The study found that the initial and recurrent embodied water constitutes 70% of the total water consumption of the building’s life, and the operational water is 30% (Rauf et al., 2024). This study illustrated the importance of finding alternative methods, as well as building components and materials to reduce embodied water consumption (Rauf et al., 2024). Another study by Salameh et al. (2022) proposed the use of solar cooling technology in air conditioning (AC) systems to reduce energy and costs of excess use of AC in residential buildings. The life cycle analysis results of the study revealed that the solar-powered absorption cooling system uses only 8.5% of the energy consumed by traditional electricity powered vapor compression systems (Salameh et al., 2022). While the UAE community do tend to live in larger homes, many young expatriates are opting for smaller living spaces due to rising economic pressures and increasing environmental awareness. Although no current studies exist on tiny houses in the UAE, it is worth exploring their potential to mitigate negative environmental impacts.
A tiny house is defined as a house that has a floorspace less than 37 m2 and a full-time home that is usually mobile (Shearer and Burton, 2019; Crawford and Stephan, 2020). The two main drivers of the tiny house movement are economic and environmental sustainability. Studies have shown that the biggest incentives to living in a small space are housing affordability, cost reduction, and mortgage debt reduction (Kilman, 2010; Shearer and Burton, 2019). Reducing debt allows for more disposable income and more flexibility in employment choices. Additionally, tiny houses are more cost-efficient than traditional homes because they require significantly fewer construction materials. Consequently, the demand for wood, steel, and concrete is much lower, reducing the environmental impact associated with resource extraction and production processes. Compared to traditional houses, tiny houses require less energy for heating, cooling, and lighting, encouraging lower consumption (Kilman, 2010; Crawford and Stephan, 2020). Therefore, the tiny house movement offers a potential solution to resource depletion, climate change, and environmental damage caused by unsustainable housing.
There are limited studies on the environmental impact of tiny houses. One study analyzed a tiny house’s global warming potential and found that the tiny house had a 70% reduction in GHG emissions compared to traditional houses, on a per capita basis (Crawford and Stephan, 2020). In addition, the indoor quality and thermal comfort of tiny houses were studied by Stratton and Corneal (2023), and it was recommended to incorporate tiny houses into residential building codes. In particular, Stratton and Corneal (2023) recommended adding sizing requirements for mechanical ventilation that consider combustion-based appliances into building codes, as well as CO2—based occupancy sensors and moisture sensors. Therefore, the comparatively smaller sizes of tiny houses promote resource efficiency and a reduced ecological footprint. Overall, tiny houses offer a potential approach to sustainable living, promoting a transition towards more responsible and eco-friendly housing options at a time when the world faces urgent environmental concerns.
There are a variety of sustainable assessment tools that are available to analyze the environmental, economic, and social factors related to a product or process. While some approaches reside at level of concept, such as design for environment or cleaner production, other approaches rely on quantitative models (Heijungs et al., 2010). These quantitative models include but are not limited to life cycle assessment (LCA), material flow accounting, life cycle costing (LCC), and cost–benefit analysis (CBA; Heijungs et al., 2010; Hoogmartens et al., 2014). LCA is a quantitative assessment approach that aims to analyze technical systems, such as products and processes, in terms of their environmental impact (Mukhopadhyay, 2020). The LCA tool assembles and estimates resource inputs, outputs, process designs, and potential environmental impacts of a product system throughout its lifecycle (Curran, 2013). The LCA methodology follows four main steps, and the first step involves defining the goal and scope of the study. The first step also includes selecting the study’s functional unit (FU) and system boundary. The FU is a quantified description of the performance of the product system and the system boundary refers to the initial and ending phases of the analysis (Curran, 2013). There are three main system boundaries implemented in LCA: cradle-to-gate, cradle-to-grave, and cradle-to-cradle. Cradle-to gate refers to the assessment of a product or process from its initial inception (resource extraction) till the factory gate, while cradle-to-grave assesses the footprint of the entire life cycle of a product, until its disposal (grave; Cao, 2017). On the other hand, cradle-to-cradle assess a product/process from resource extraction until the product can be repurposed or recycled, reducing environmental impacts (Preethi Kavitha et al., 2020). The second step is the life cycle inventory (LCI) analysis, which involves inserting the relevant material inputs, energy inputs, and environmental releases (Curran, 2013). The third step is life cycle impact assessment (LCIA) evaluating the potential environmental impact assessments associated with the inputs and releases (Curran, 2013). Finally, the fourth step is interpreting the results of the LCA (Curran, 2013; Hoogmartens et al., 2014).
To identify potential energy saving interventions in tiny houses, a LCA must be conducted to evaluate the use of energy and resources throughout its lifespan. LCAs have proven effective in addressing issues related to climate change and resource depletion (Alhazmi et al., 2021). An LCA allows researchers to examine the energy usage and resource consumption of buildings and houses from the extraction phase until the demolition and waste management phase (Yang et al., 2018). Insights from an LCA can help in optimizing energy and resource use at all stages of a building structures life, as it addresses the impact of raw material choice and sourcing on a building’s energy and environmental footprint (Cuéllar-Franca and Azapagic, 2012). The construction phase produces significant waste and pollution, and the operational phase accounts for the highest energy consumption, ranging between 40 and 90% of total life cycle energy use, due to its long lifespan (Cuéllar-Franca and Azapagic, 2012). Finally, demolition and disposal activities utilize energy and generate waste that can be either recycled, reused, or landfilled, affecting the overall LCA.
The LCA methodology is a globally recognized approach that evaluates the comparative environmental performance of buildings, emphasizing the need for sustainable practices in the construction industry and a systemic approach for conducting LCA studies (Alhazmi et al., 2021). For instance, Nwodo and Anumba (2019) reviewed LCA studies on buildings, highlighting the importance of integrating LCA into building design to reduce environmental impacts, and the need for a scientific approach for LCA studies to reduce individual bias. Furthermore, Roberts et al. (2020) demonstrated that implementing LCA in the early design stage of the building has a higher potential of reducing the overall environmental impact of the building than conducting the LCA at later design stages, where it has little effect on the decision-making process. Ben-Alon et al. (2021) conducted an LCA of buildings made of natural materials compared to conventional building materials in six different climates and found that natural materials have superior energy conservation compared to conventional materials in all six climates (Günkaya et al., 2021) explored how energy-saving measures such as exterior insulation and double glazing applications can significantly reduce a building’s environmental impact by minimizing the energy consumption during the operational phase, while Arehart et al. (2020) discussed the theoretical carbon storage potential of hempcrete, highlighting innovative approaches for construction materials that have a net negative carbon footprint over its lifecycle.
In the Middle East, LCA studies on buildings are also gaining traction due to the region’s unique climatic and environmental conditions. For example, Asif et al. (2017) conducted an LCA of a residential building in Saudi Arabia, focusing on the energy consumption associated with different building materials. Their findings revealed that concrete and steel account for 62.6% of the embodied energy and the bulk of the environmental impact of the house, emphasizing the need for sustainable material choices in the region. Similarly, al-Omari et al. (2023) explored the LCA of residential buildings in Jordan, comparing traditional concrete structures with more sustainable alternatives. Their study highlighted that using locally sourced materials like bricks instead of cement concrete and energy-efficient designs could substantially reduce the environmental impacts of residential buildings. They also found that the operational phase was the major contributor to carbon emissions. The findings from these studies emphasize the importance of regional specificity in LCA methodologies to achieve accurate and actionable results.
In this study, the environmental impacts of a tiny house, built on the American University of Sharjah (AUS) campus were evaluated using a life cycle impact assessment. This study focuses on 18 impact categories and one life cycle indicator: climate change, ozone depletion, terrestrial acidification, freshwater eutrophication, marine eutrophication, human toxicity, photochemical oxidant formation, particulate matter formation, terrestrial ecotoxicity, freshwater ecotoxicity, marine ecotoxicity, ionizing radiation, agricultural land occupation, urban land occupation, natural land transformation, water depletion, metal depletion, and fossil depletion. Standard methodologies were used based on Simapro software.
The novelty of this study is that it adapts and applies LCA methodologies to tiny houses, a subject rarely explored in existing literature. Moreover, it contextualizes these findings within the UAE’s specific environmental and climatic conditions. As tiny houses are inherently designed to minimize resource use, they present a promising alternative to traditional housing, especially in regions facing sustainability challenges. By conducting the first comprehensive LCA of a tiny house in the UAE, this study provides novel insights into the environmental performance of such housing solutions in arid climates.
The goal and scope are defined with respect to the intended applications and assumptions of the study. The goal of this study is to estimate the life cycle environmental impacts of a tiny house built in the AUS campus located in Sharjah, United Arab Emirates. The results are then used to assess the significance of each material, process, and phase in contributing to various impact categories. The system boundaries and functional units are defined during this stage in accordance with ISO 14040 and ISO 14044.
The working hypotheses of this study are as follows: (1) Tiny houses in arid climates offer significant environmental benefits and reduced resource consumption due to their small area. (2) Applying Life LCA methodologies to tiny houses will reveal lower overall carbon emissions and resource consumption when compared to conventional homes in the UAE. (3) The choice of construction materials heavily affects the environmental impact of the tiny house. These hypotheses guide the structure and focus of our study, ensuring that we can assess the environmental implications of adopting tiny houses in the UAE context.
2 Materials and methods
The LCA methodology was used to evaluate the impacts of the tiny house built in AUS. It follows the International Organization for Standardization (ISO) 14,040 and ISO 14044. Simapro software version 8 was used to model the LCA. Simapro is a product system and modeling assessment software based in the Netherlands (Herrmann and Moltesen, 2015). The main reason Simapro was chosen instead of other available softwares such as OpenLCA, GaBi, and Umberto, is because of its Ecoinvent database. In LCA studies, Ecoinvent is one of the most comprehensive global databases, and its customaizable with third party databases. In addition, Simapro allows for custom impact assessment methods along with a smooth integration of datasets (Silva et al., 2017). While OpenLCA is a free and open access tool, it has a lack of freely available datasets, some of which are also poorly documented (Silva et al., 2017). A major issue with Umberto is that it does not have the option to import/export individual datasets and they have a limited number of datasets available (Silva et al., 2017). GaBi is the closest software tool option to Simapro, but GaBi is generally applied more using industry-specific databases. Overall, Simapro is a well-rounded tool with extensive database access and strong reporting capabilities.
The Ecoinvent v3 database was used from the Simapro software. Table 1 summarizes the included items in the LCA inventory, in the material, production transportation, construction, operation, and disposal stages. The maintenance phase was not considered in the study.
2.1 Description of the tiny house characteristics
Figure 1 shows the floor plan of the single-story tiny house in centimeters (cm) developed using ArchiCAD. Figure 2 illustrates the 3D ArchiCAD models of the exterior and interior of the tiny house. The outer width of the house is approximately 300 cm and the outer length of the house is 600 cm, so the area of the tiny house in this study is 18 m2. The main structural system, comprising of beams and columns, is classified as an ordinary moment frame (OMF). The structural system of the tiny house is composed of locally available steel frames, while the walls, roof, and flooring are made using sustainable, lightweight concrete panels known as ‘SAF’ panels. In addition, the fully butt-welding method was used. The steel frames were manufactured at a location off-site and then transported to the university.
The tiny house located in the AUS campus was selected as a representative model for the sustainable structures in the UAE, due to its resource-efficient construction methods and use of locally sourced materials like steel and lightweight concrete panels. Its design adapts to the UAE’s arid climate, where energy efficiency is crucial, especially during the operational phase, which heavily relies on cooling systems to mitigate extreme temperatures.
2.1.1 System boundaries
A cradle-to-grave evaluation was conducted for the LCA of the tiny house, as shown in Figure 3. The phases before the operation are the building materials extraction/production, transportation, and construction/installation phases, also referred to as modules of A1-A5 according to UNE15804 (Yang et al., 2018). During the operational stage (use stage), the operational use of electricity and water, as well as GHG emissions are considered, following modules B6 and B7. At the end-of-life phase, the tiny house is sent to be disposed of at the nearest landfill, C4 of UNE15804.
2.1.2 Functional unit
The FU is used as the basis of comparing environmental impact of products or services, and the inputs of the system are determined based on the chosen FU. Some studies (Cuéllar-Franca and Azapagic, 2012; Yang et al., 2018) use the gross area of the residential area as a FU, while other studies (Dekker et al., 2020; Abdalla et al., 2021) consider 1 m2 of the house as its FU. In this study, the FU used is per 1 m2 of the tiny house, as it is easier to compare the results to other studies when presented as per 1 m2 instead of the total usable floor area.
2.2 Life cycle inventory
The LCI stage is critical as it quantifies the inputs of the system, including material consumption as well as the emissions related to the processes and activities that are part of the system boundary. The inputs used to calculate the environmental impact per 1 m2 of the tiny house were compiled using the tiny house bill of materials. Detailed process data were considered in this LCA in the production, transportation, and operation phases.
2.2.1 Building materials stage
The building materials were allocated in the inventory data by using the bill of quantities (BOQ) shown in Table 2 using the Ecoinvent database.
2.2.2 Transportation stage
All the different building materials were brought to AUS from different suppliers, so the distance of every supplier’s location to AUS was calculated. This distance was multiplied by the mass of material per m2 of the house.
Table 3 shows the material inventory for the transportation stage in tons-kilometers.
2.2.3 Construction stage
In the construction stage, the waste material was added as an input under the assumption that 10% of the initial building materials in the BOQ were construction waste. This assumption is based off the general limits for structural steel wastages via cutting and bending steel plates or sections at fabrication. While tiny houses can built on foundation and be made fixed, they can also be fully mobile on a trailer base or semi-mobile on a non-permanent foundation (Shearer and Burton, 2023). The tiny house in this study does not require any excavation or soil preparation, so these processes were not considered in the inventory data for this stage.
2.2.4 Operational stage
The tiny house is assumed to last for 50 years, based on the materials as well as the maintenance and services. The water demand, energy demand, and CO2 emissions per m2 of the house in 50 years are illustrated in Table 4.
2.2.5 End-of-life stage
In the UAE, the common practice for construction waste is to be disposed of at the end of its service life. So, it was assumed that the tiny house was transported by truck to be disposed of in a nearby landfill in Bee’ah Waste Management Complex, located 23 km away from AUS campus.
2.3 Life cycle impact assessment
In this stage, the potential environmental impacts are assessed by analyzing the life cycle inventory results and linking the inventory data to specific impacts following ISO standards. While many papers have followed the CML methodology (Cuéllar-Franca and Azapagic, 2012; Dekker et al., 2020; Alhazmi et al., 2021), this study utilizes the ReCiPe 2016 V1.03 midpoint method to evaluate the 18 potential impacts of the tiny house. CML is a midpoint method that assesses several impact categories, normalization is provided but there is neither weighing or addition (Goedkoop, 2022). The ReCiPe framework was chosen as it has a wider set of impact categories than CML and its impact mechanisms have a global scope, instead of a European scope (Dekker et al., 2020). The ReCiPe 2016 V1.03 midpoint method uses 18 unique midpoint impact categories, including climate change (kg CO2 eq), ozone depletion (kg CFC-11 eq), terrestrial acidification (kg SO2 eq), freshwater eutrophication (kg P eq), marine eutrophication (kg N eq), human toxicity (kg 1,4-DB eq), photochemical oxidant formation (kg NMVOC), particulate matter formation (kg PM10 eq), terrestrial ecotoxicity (kg 1,4-DE eq), freshwater ecotoxicity (kg 1,4-DE eq), marine ecotoxicity (kg 1,4-DB eq), ionizing radiation (kBq U235 eq), agricultural land occupation (m2 a), urban land occupation (m2 a), natural land transformation (m2), water depletion (m3), mineral resource depletion (kg Fe eq), and fossil fuel depletion (kg oil eq; Dekker et al., 2020; Dekker et al., 2020). This study, like the one by Alhazmi et al. (2021) adapts the global Ecoinvent dataset to reflect local conditions, such as the energy consumption patterns specific to the UAE. These adaptations highlight the robustness of the LCA methodology and its flexibility across different regions and housing types.
3 Results
3.1 Cradle-to-grave results
The LCA impact assessment results provide an estimation of the environmental impact per square meter of the internal area of a tiny house. The impacts considered in this study span across various stages, including the building materials production stage, transportation of the building materials stage, construction stage, operational stage for a 50-year life span, and end-of-life disposal to landfills.
Table 5 provides detailed results of the life cycle assessment of the tiny house from cradle to grave for the FU (m2 of the internal area of the tiny house). Figure 4 depicts the percentage contribution of each life cycle stage to each of the individual impact categories. The results show that the raw materials production stage and operation stage have the most effect on the tiny house. On the other hand, materials transportation stage and disposal at the end of life have no significant environmental effect across all the impact categories. The construction process only has a notable environmental effect on the particulate matter formation, which is expected since this process involves activities such as cutting building elements, sanding, and painting.
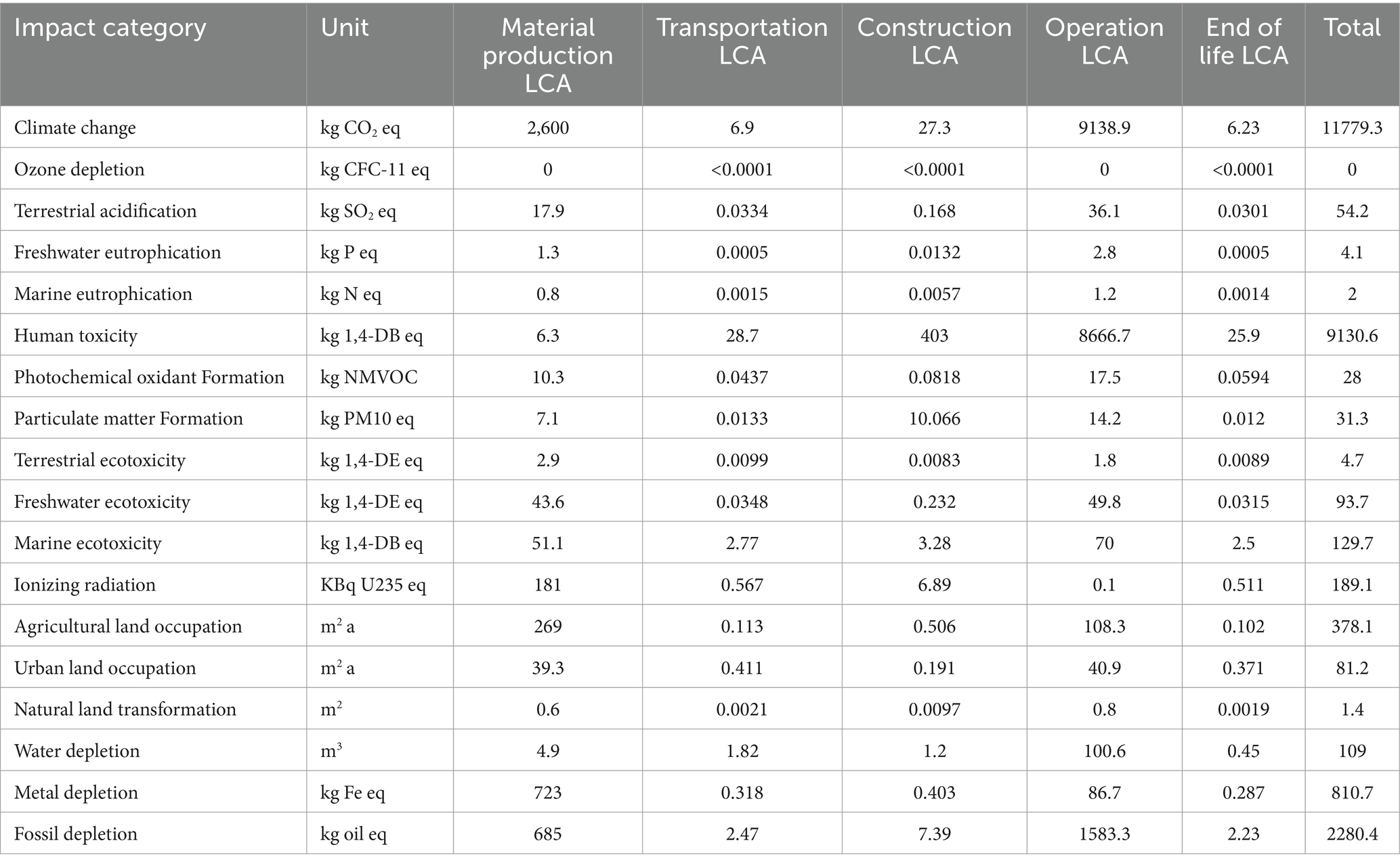
Table 5. Life cycle impact assessment results for a tiny house during a 50-year life span per functional unit.
A total of 11.78 tons CO2—eq per m2 (equivalent to 212 tons CO2—eq for the whole tiny house) is emitted throughout the tiny house’s lifetime. 77.6% of this emission potentially occurs during the operation stage, while 22% happens during the material production stage. This emission rate is relatively high compared to the 50-year operation stage. The operation stage contributes to 62% of ozone depletion, whereas the material production stage accounts for 37% of this category. Almost two-thirds of terrestrial, freshwater, and marine acidification results from the operational phase, with the remaining third originating from the material production phase. The Human Toxicity potential from the tiny house is estimated to be 9,130 kg 1,4-DB eq. 95% of this toxicity is due to the operation phase, based on electricity and water use estimations from the reference report. Terrestrial, freshwater, and marine ecotoxicities are similarly distributed between the material production and operation phases. However, the total ecotoxicity amounts to 228 kg of NMVOC over the tiny house’s lifetime per the FU, which is relatively high.
Ionizing radiation from radioactive materials is estimated to be 189.1 kBq U235 eq, with almost 95.7% produced during the material production phase. This emphasizes the hazardous nature of raw materials extraction and manufacturing processes. The material production stage also contributes significantly to metal depletion (89.2%), and agricultural land depletion (71.2%). The remaining portion is primarily attributed to the operation phase. While the metals are mainly used during material production, the water used during the operation stage exceeds the amount consumed during the material production stage by more than 20 times. The operation phase accounts for more than 92.3% of water depletion over the house’s lifetime.
The urban area occupation is estimated to be 81.22 m2 per year, evenly distributed between material production and operation phases. The natural land transformation category covers only 1.38 m2 over the tiny house’s life cycle per the FU, equivalent to 24.84 m2 for the whole house. Finally, 2280.5 kg oil eq of fossil fuel is expected to be depleted over the project’s lifetime, with 69.4% of it estimated to occur during the operation stage, primarily due to electricity and energy consumption.
3.2 Comparison with other studies
To gain deeper insights into the LCA results of the tiny house, they were compared with LCA studies of residential buildings having similar functional units and lifespans. Specifically, the LCA results of the tiny house were juxtaposed against the findings from Alhazmi et al. (2021) and Cuéllar-Franca and Azapagic (2012) as these studies provided relevant benchmarks for comparison. This comparison helps to validate the findings by situating them within the broader body of knowledge on sustainable housing and LCA. By identifying where the results align with or differ from established research, both the robustness and reliability of the conclusions of this study are ensured. Additionally, such comparisons highlight the novelty of this study, especially in terms of applying LCA to tiny houses in the UAE, an area that remains underexplored. This comparison also helps to contextualize the findings, showing how tiny house models in extreme climates may offer different sustainability benefits than those observed in temperate regions.
Cuéllar-Franca et al. conducted a comprehensive LCA of three prevalent house types in the UK: detached, semi-detached, and terraced houses. Given that the terraced house closely matches the tiny house in terms of size, it was selected for comparison. However, since the FU in the Cuéllar-Franca study is the entire house, their results were normalized by the area of the terraced house to align with the tiny house study’s FU (m2). Meanwhile, H. Alhazmi et al. assessed the environmental impact of constructing a villa in Saudi Arabia over a 50-year lifespan, employing the same FU as the tiny house study. This similarity in methodology and assumptions rendered the Saudi villa study a valuable point of comparison, especially since it represents one of the few LCA studies for residential buildings in the region.
Our study’s comparisons focused on the total environmental impact across all life cycle stages, including the end-of-life phase, over 50 years. Notably, Cuéllar-Franca and Azapagic (2012) and Alhazmi et al. (2021) utilized the CML method for impact estimation, whereas our tiny house LCA employed the ReCiPe method (Cuéllar-Franca and Azapagic, 2012; Abdalla et al., 2021). Due to the differences in impact assessment methods and terminologies, the comparison was confined to the five common impact categories: climate change, ozone depletion, photochemical oxidant formation, acidification, and eutrophication. Additionally, human toxicity was included in the comparison due to its critical implications for human health, despite its exclusion from the KSA villa study.
Figure 5 presents the summarized total impact results for each house type from material production to disposal. The results were scaled to fit in the same graph. The original (un-scaled) values can be obtained by multiplying the value shown on the y-axis by the scaling factor given before the unit of each impact category. The terraced house exhibits the highest CO2 equivalent emissions at 17,166.67 kg CO2 eq, followed by the tiny house at 11,779.32 kg CO2 eq, and the villa with the lowest emissions at 7,255.66 kg CO2 eq. This indicates that the terraced house has the most significant impact on climate change, while the villa has the least. The tiny house demonstrates the lowest ozone depletion potential at 0.000580846 kg CFC-11 eq, significantly lower than the villa’s 0.000911 kg CFC-11 eq and the terraced house’s 0.00272778 kg CFC-11 eq. This suggests the tiny house is more environmentally friendly concerning ozone layer protection. The tiny house has the lowest photochemical oxidant formation at 0.893 kg NMVOC, compared to the terraced house at 3.7611 kg NMVOC and the villa at 2.021 kg NMVOC, indicating lesser smog formation potential. Interestingly, the tiny house has the highest acidification potential at 54.187 kg SO2 eq, followed by the villa at 47.23 kg SO2 eq, and the terraced house at 34.722 kg SO2 eq. This highlights a significant environmental concern for the tiny house. The eutrophication potential of the tiny house (4.108 kg P eq) is nearly equivalent to that of the villa (4.076 kg P eq), both lower than the terraced house (4.511 kg P eq), suggesting similar environmental performance in terms of nutrient pollution. The human toxicity impact of the tiny house is notably high at 9,130.61 kg 1,4-DB eq, which is approximately five times greater than the terraced house at 1,871.11 kg 1,4-DB eq. The KSA villa study did not report on this category. The disparity in human toxicity could stem from differences in building materials, geographical locations, and specific LCA methodologies employed.
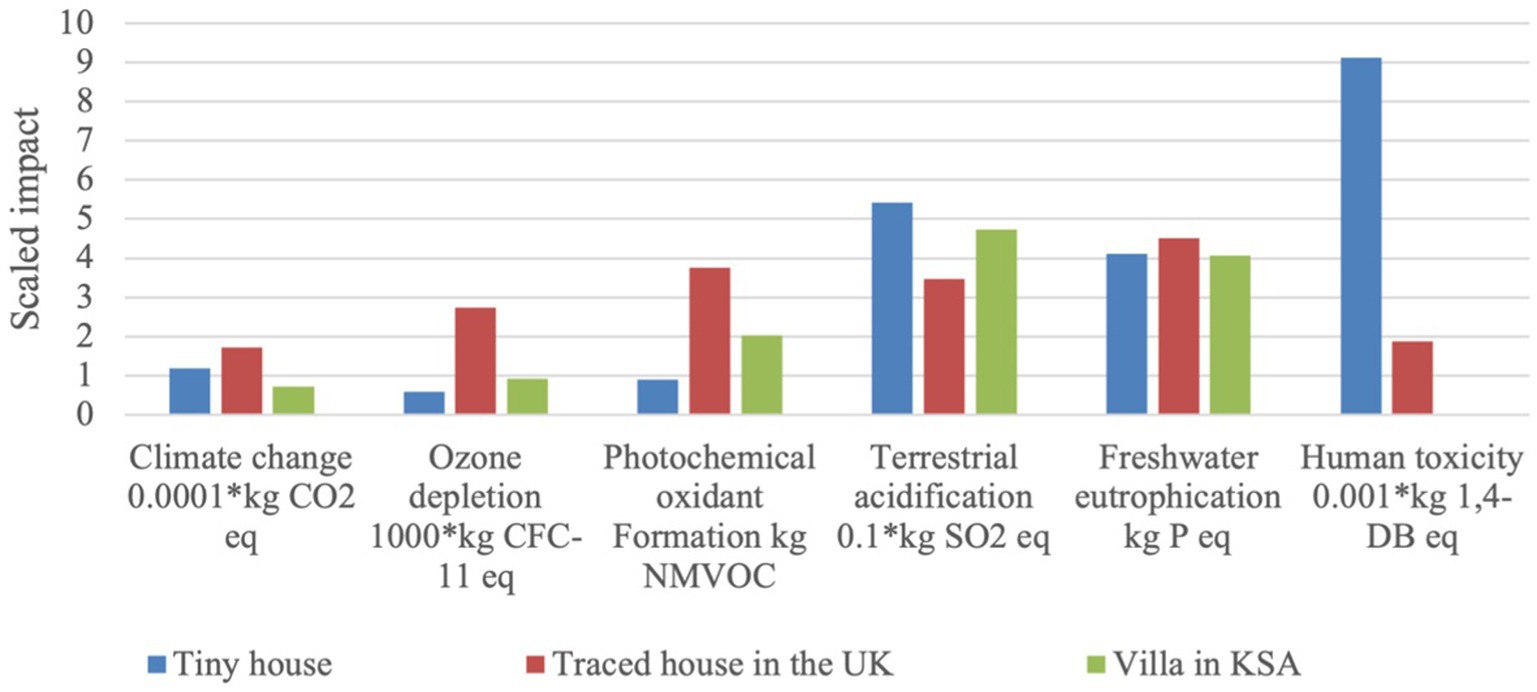
Figure 5. Comparison between LCA of the tiny house, terraced house in the UK, and a villa in KSA (Cuéllar-Franca and Azapagic, 2012; Alhazmi et al., 2021).
The results of this study are comparable to an LCA assessment evaluating conventional and sustainable villas in the UAE (Elgzeary et al., 2024). Both studies are based in the UAE and use the ReCiPe midpoint impact method, with the same 18 indicators; however, the study by Elgzeary et al. did not consider the villas’ end of life phase. The results of the LCA found that the concrete and masonry works are the main contributors to the environmental impacts (Elgzeary et al., 2024). Similarly, the results of this LCA also found the production and installment of the lightweight concrete panels has one of the most significant impacts to the environment. These findings demonstrate the adverse impacts of concrete on climate change, fossil fuel depletion, and human toxicity exposure. The traditional villa has high electricity and water consumption in comparison to the tiny house, particularly due to its larger size and energy requirements. The average UAE household consumes 500 L of water per day, while the tiny house consumes 100 L per day (Elgzeary et al., 2024). The study by Elgzeary et al. did not provide a detailed breakdown of the total contribution of the conventional villa across the 18 impact categories. For this reason, a comparison of the environmental performance between this study and the traditional UAE villa cannot be feasible.
In summary, while the tiny house shows advantages in categories such as ozone depletion and photochemical oxidant formation, it falls short in terms of acidification and human toxicity impacts. These variations can be attributed to the differing LCA methods, material choices, and regional contexts of the studies compared.
3.3 Materials production stage LCA results
To gain a comprehensive understanding of the environmental impact associated with the construction of a tiny house, an in-depth analysis of the building material production phase was conducted. This phase is crucial as it encompasses the manufacturing processes of various materials used in the construction of the tiny house. These materials were categorized into seven distinct groups: metal frames, insulation materials, concrete, wood, paint, glass windows along with their frames, and mechanical, electrical, and plumbing (MEP) materials.
The production of raw materials for concrete was found to be a significant contributor to potential climate change impacts during the material production phases, accounting for 42.2% of the total impact. Concrete production was not only a major contributor to climate change but also had a substantial impact on seven additional environmental impact categories. These categories include freshwater eutrophication (54.1%), marine eutrophication (40.3%), human toxicity (45.9%), ionizing radiation (40.4%), water depletion (44%), metal depletion (65.4%), and fossil depletion (45%).
In addition to concrete, the production process of the metal frames also had a significant environmental impact during this stage. It accounted for 81% of ozone depletion, 37.8% of terrestrial ecotoxicity, 60.2% of freshwater ecotoxicity, 87.5% of agricultural land occupation, and 43.5% of natural land transformation. On the other hand, the production of aluminum windows was found to contribute to 78.8% of terrestrial eutrophication.
Upon evaluating all the material production processes, it was clear that the production of concrete exhibited the highest environmental impact. This was closely followed by the production of metal frames. After these, the production processes of aluminum window materials, insulation materials, paint, MEP materials, wood materials, and ceramic materials were identified as the next most significant contributors to environmental impact, in that order.
Comparing our detailed LCA results for the tiny house building materials production with the broader findings from Abdalla et al. (2021), where they performed a detailed LCA for the materials used in the construction of a conventional home, reveals consistent and critical insights into the environmental impacts of housing material production. Both our study, and the one by Abdalla et al. (2021) highlight the significant environmental burden of concrete and metal production. In our study, concrete production contributes 42.2% to climate change impacts and has substantial effects on freshwater eutrophication, marine eutrophication, and human toxicity. Similarly, the Abdalla et al. (2021) study identifies cement production as a major contributor across all impact categories due to high raw material consumption, energy use, and waste emissions. Moreover, our findings show that metal frames significantly impact ozone depletion (81%) and various ecotoxicity categories, which aligns with the findings of Abdallah et al.’s study, where reinforcing steel production heavily influences freshwater eutrophication (99%), land occupation (98%), and ecotoxicity (93%). This study and Abdallah et al.’s also agree that electricity production has relatively low impacts, except in ionizing radiation. This comparison underscores the necessity of targeting concrete and metal production for reducing the environmental footprint of residential construction.
In conclusion, the study provides a detailed comparison of the environmental impacts associated with the production of different materials used in the construction of a tiny house. The results, summarized in Figure 6, offer valuable insights into the LCA of the tiny house per the FU. These comprehensive analysis aids are crucial for developing sustainable building practices and materials, highlighting areas for potential improvement in the construction of both conventional and tiny houses.
4 Discussion
The LCA results from the study on tiny house construction revealed critical insights when interpreted considering previous studies and our working hypotheses. The primary finding is the substantial environmental impact associated with the production and operational phases, aligning with similar studies on residential buildings. Specifically, our analysis indicates that the material production stage, particularly concrete and metal frames, contributes significantly to various impact categories such as climate change, eutrophication, and human toxicity. This is consistent with findings from previous studies, including those by Cuéllar-Franca and Azapagic (2012) and Alhazmi et al. (2021), which identified concrete and steel production as major environmental burdens in house construction (Dekker et al., 2020; Abdalla et al., 2021). However, it’s crucial to interpret these values with caution, considering the tiny house’s very small size compared to other housing types. The total impact of the whole tiny house, when compared to the environmental impact of other residential house types, may appear less significant.
The operational phase of the tiny house, primarily driven by energy consumption for heating, cooling, and electricity, was found to be the dominant contributor to climate change impacts, accounting for 77.6% of total CO2 emissions over the 50-year lifespan. This mirrors the patterns observed in other LCA studies, where the operational energy demand often overshadows the impacts of other life cycle stages. For instance, our results show a higher climate change impact compared to the terraced house in the UK but lower than that of a villa in Saudi Arabia, reflecting regional variations in energy use and efficiency.
Additionally, while the tiny house shows a lower potential for ozone depletion and photochemical oxidant formation compared to other residential types, it exhibits higher acidification and human toxicity potential. This discrepancy can be attributed to the specific materials used and the geographic and methodological differences in the LCA studies compared. For example, the significant human toxicity impact is primarily due to the extensive use of certain construction materials and the high electricity consumption during the operational phase, which emphasizes the need for improving energy efficiency and material selection in tiny house construction.
The tiny house is more recyclable and has higher reuse potential than a conventional villa in the UAE as its structure is made of locally available steel, which is easily recyclable. In addition, the walls and flooring consist of lightweight concrete panels, which are easy to handle for reuse due to their modular design. The structure framework of traditional villas and buildings in the UAE primarily consists of reinforced concrete blocks, which account for 44.6% of the construction and demolition waste disposed into landfills (Belpoliti et al., 2018). It is very difficult to separate the structure into its original parts during maintenance or demolition, meaning it cannot be repurposed into its original state (Belpoliti et al., 2018). However, Bee’ah construction and demolition waste recycling facility has a 95–97% recovery rate and diverts all construction waste from landfills (AlHaj Ali et al., 2022). The facility repurposes concrete waste by grinding concrete and debris to create recycled aggregates or a base for roads (Belpoliti et al., 2018; Alzard et al., 2021). While this greatly reduces landfill dependency, the continuous process of breaking down concrete is energy-intensive and contributes to carbon emissions. Since steel is completely recyclable, an estimated amount of 95% of the steel in the tiny house can be recycled, while approximately 80% of the lightweight concrete SAF panels will be recycled (Broadbent, 2016). The estimation of the reusability of the SAF panels is based on its modular design. Traditional villas in the UAE are significantly larger than tiny houses, resulting in substantially greater material waste generated during their construction compared to the minimal waste produced by tiny houses. Moreover, the materials used in the tiny house (steel frames and SAP panels) are inherently recyclable, unlike traditional villas that require extensive energy for reuse. This underlines the importance of material choice as well as waste recycling infrastructure in minimizing environmental impact. Overall, the tiny house produces a lot less waste and includes a higher proportion of easily reusable materials compared to conventional housing in the UAE, making it more aligned with sustainable practices.
The comparison with the study by Abdalla et al. (2021) conventional home materials underscore the importance of focusing on concrete and metal production to mitigate environmental impacts (Dekker et al., 2020). Both studies highlight the high environmental footprint of these materials, suggesting that innovations in sustainable material alternatives and recycling could be crucial for reducing the overall impacts. Several studies have examined the potential environmental benefits of using wood and timber products, providing insights to the advantages of wood as a renewable resource (Rai et al., 2022; Barbhuiya and Das, 2023; Ding et al., 2023). Furthermore, a book chapter (Woodard and Milner, 2016), outlined the embodied energy and net carbon impacts of multiple building materials over all life stages, including sawn timber, red brick, light concrete blocks, rigid PVC, recycled steel, steel, and aluminum. This chapter illustrated that sawn timber has negative CO2 production per cubic meter of material. They also summarized case studies demonstrating the embodied carbon benefits of wood, emphasizing its carbon storage capacity and the GHG emissions avoided when substituting wood for steel or concrete (Woodard and Milner, 2016). In addition, wood can be recycled directly or indirectly, and the recycling of timber products is highly efficient compared to alternative materials such as aluminum and steel (Woodard and Milner, 2016). Therefore, it is worthwhile to evaluate wood as a viable alternative building material, not only for its renewable and carbon storage properties, but for its recycling potential, which can further reduce environmental impacts and promote circular construction practices.
One of the key strengths of this study is its focus on a critical global issue—sustainable housing in regions facing both resource scarcity and high energy demand. By applying a detailed LCA to a tiny house model within the UAE’s unique climatic context, this research addresses gaps in previous studies that often overlook arid climates using localized data, to ensure that the findings are directly relevant to regional needs, while contributing to global discussions on sustainable building practices (Johst et al., 2024).
5 Study limitations
While the findings align with previous studies on residential buildings, it’s essential to note the limitations of our data source. The analysis primarily relies on the bill of quantities, suppliers’ information, and resource consumption patterns from the project. In future research, obtaining more detailed information, perhaps through direct measurements and comprehensive surveys, would enhance the accuracy and comprehensiveness of the results. In addition, the maintenance phase being excluded from the analysis may affect the overall environmental footprint of the tiny house. Also, since the case study of this paper is specific to the UAE’s conditions and may not be applicable to regions with different climates, resources, or construction practices.
6 Conclusion
This study provides a comprehensive LCA of a tiny house, revealing critical environmental impacts primarily during the material production and operational stages. The findings are consistent with previous studies, affirming the significant contributions of concrete and metal frames to various environmental impacts. The operational phase, driven by energy consumption, is the major contributor to climate change impacts, emphasizing the need for energy-efficient designs and renewable energy sources in tiny house construction.
The higher impacts in categories such as acidification and human toxicity compared to other residential types highlight areas for potential improvement. Future research should focus on exploring sustainable material alternatives, enhancing energy efficiency, and incorporating renewable energy technologies to reduce the environmental footprint of tiny houses.
Overall, this study highlights the importance of a holistic approach to LCA for a tiny house, considering all the phases from material production to disposal to landfills. The impact patterns of tiny houses are similar to other house types when comparing them based on the unit area. However, the significance of a tiny house as a potential solution for reducing the environmental impact of residential buildings, is its very tiny size, creating less impact than conventional houses. By addressing the identified hotspots, significant strides can be made toward more sustainable building practices, contributing to the broader goal of reducing the environmental impact of housing.
The findings of this study support the UAE’s sustainability goals, particularly in the context of COP28 and the UAE Green Agenda 2030. By demonstrating the environmental benefits of tiny houses, this research can guide policymakers in implementing housing solutions that align with national objectives for carbon reduction and resource efficiency. Projects like Masdar City represent the UAE’s commitment to sustainable urban development, and integrating tiny houses within such frameworks can further enhance the nation’s efforts to achieve Net Zero by 2050. Overall, this study provides a viable model for sustainable living that aligns with the UAE’s vision for a greener future.
Data availability statement
The original contributions presented in the study are included in the article/supplementary material, further inquiries can be directed to the corresponding author.
Author contributions
LS: Conceptualization, Formal analysis, Investigation, Methodology, Writing – original draft, Writing – review & editing. RA: Conceptualization, Formal analysis, Methodology, Software, Writing – original draft, Writing – review & editing. LA: Formal analysis, Writing – original draft, Writing – review & editing. SA: Conceptualization, Methodology, Resources, Supervision, Writing – review & editing. MM: Methodology, Validation, Visualization, Writing – review & editing. TA: Validation, Writing – review & editing. AT: Data curation, Validation, Writing – review & editing.
Funding
The author(s) declare financial support was received for the research, authorship, and/or publication of this article. This manuscript was supported by the American University of Sharjah, United Arab Emirates, through grants FRG22-C-E15.
Acknowledgments
The authors would like to express their gratitude to the American University of Sharjah for providing resources for this research. In addition, the authors would like to acknowledge that the data collected to conduct the LCA was derived from the Spring 2018 senior design project in the American University of Sharjah.
Conflict of interest
The authors declare that the research was conducted in the absence of any commercial or financial relationships that could be construed as a potential conflict of interest.
The author(s) declared that they were an editorial board member of Frontiers, at the time of submission. This had no impact on the peer review process and the final decision.
Publisher’s note
All claims expressed in this article are solely those of the authors and do not necessarily represent those of their affiliated organizations, or those of the publisher, the editors and the reviewers. Any product that may be evaluated in this article, or claim that may be made by its manufacturer, is not guaranteed or endorsed by the publisher.
References
Abdalla, H., Fattah, K. P., Abdallah, M., and Tamimi, A. K. (2021). Environmental footprint and economics of a full-scale 3D-printed house. Sustainability 13:1978. doi: 10.3390/su132111978
Abdulmaksoud, S., and Beheiry, S. (2023). Perceptions governing sustainability in the UAE construction sector. Buildings 13:683. doi: 10.3390/buildings13030683
Abu-Hijleh, B., and Jaheen, N. (2019). Energy and economic impact of the new Dubai municipality green building regulations and potential upgrades of the regulations. Energ. Strat. Rev. 24, 51–67. doi: 10.1016/j.esr.2019.01.004
Al-Alili, A., and Al Qubaisi, A. (2018). Efficient residential buildings in hot and humid regions: the case of Abu Dhabi, UAE. Int. J. Thermal Environ. Eng. 17, 29–40. doi: 10.5383/ijtee.17.01.004
AlHaj Ali, S., Kawaf, L., Masadeh, I., Saffarini, Z., Abdullah, R., and Barqawi, H. (2022). Predictors of recycling behavior: a survey-based study in the city of Sharjah, United Arab Emirates. J. Health Res. 36, 552–560. doi: 10.1108/JHR-09-2020-0431
Al-Hajj, A., and Hamani, K. (2011). Material waste in the UAE construction industry: Main causes and minimization practices. Archit. Eng. Des. Manag. 7, 221–235. doi: 10.1080/17452007.2011.594576
Alhazmi, H., Alduwais, A. K., Tabbakh, T., Aljamlani, S., Alkahlan, B., and Kurdi, A. (2021). Environmental performance of residential buildings: a life cycle assessment study in Saudi Arabia. Sustainability 13:3542. doi: 10.3390/su13063542
al-Omari, O., Alkhdor, A., al-Rawashdeh, M. A., al-Ruwaishedi, M. R., and al-Rawashdeh, S. B. (2023). Evaluating carbon footprint in the life cycle design of residential concrete structures in Jordan. Civ. Eng. J. 9, 1646–1659. doi: 10.28991/CEJ-2023-09-07-07
Alzard, M. H., El-Hassan, H., and El-Maaddawy, T. (2021). Environmental and economic life cycle assessment of recycled aggregates concrete in the United Arab Emirates. Sustainability 13:348. doi: 10.3390/su131810348
Arehart, J. H., Nelson, W. S., and Srubar, W. V. (2020). On the theoretical carbon storage and carbon sequestration potential of hempcrete. J. Clean. Prod. 266:121846. doi: 10.1016/j.jclepro.2020.121846
Asif, M., Dehwah, A., Ashraf, F., Khan, H., Shaukat, M., and Hassan, M. (2017). Life cycle assessment of a three-bedroom house in Saudi Arabia. Environments 4:52. doi: 10.3390/environments4030052
Bande, L., Manandhar, P., Ghazal, R., and Marpu, P. (2020). Characterization of local climate zones using ENVI-met and site data in the city of Al-Ain, UAE. Int. J. Sustain. Dev. Plan. 15, 751–760. doi: 10.18280/ijsdp.150517
Barbhuiya, S., and Das, B. B. (2023). Life cycle assessment of construction materials: methodologies, applications and future directions for sustainable decision-making. Case Stud. Const. Mat. 19:e02326. doi: 10.1016/j.cscm.2023.e02326
Belpoliti, V., Abbas, H. S., Abu Ali, M. A., and Khulaifi, A. M. (2018) “Design from waste: review of the UAE waste management sector and experimentation on recycled materials to support the development of a sustainable and energy-efficient building market.” in 2018 Advances in Science and Engineering Technology International Conferences (ASET), pp. 1–6.
Ben-Alon, L., Loftness, V., Harries, K. A., and Cochran Hameen, E. (2021). Life cycle assessment (LCA) of natural vs conventional building assemblies. Renew. Sust. Energ. Rev. 144:110951. doi: 10.1016/j.rser.2021.110951
Broadbent, C. (2016). ‘Steel’s recyclability: demonstrating the benefits of recycling steel to achieve a circular economy’, the. Int. J. Life Cycle Assess. 21, 1658–1665. doi: 10.1007/s11367-016-1081-1
Cao, C. (2017). “Sustainability and life assessment of high strength natural fibre composites in construction” in Advanced high strength natural fibre composites in construction. eds. M. Fan and F. Fu (Duxford, United Kingdom: Elsevier Inc.), 529–544.
Clune, S., Morrissey, J., and Moore, T. (2012). Size matters: house size and thermal efficiency as policy strategies to reduce net emissions of new developments. Energy Policy 48, 657–667. doi: 10.1016/j.enpol.2012.05.072
Coolen, J. W. P., Vanaverbeke, J., Dannheim, J., Garcia, C., Birchenough, S. N. R., Krone, R., et al. (2022). Generalized changes of benthic communities after construction of wind farms in the southern North Sea. J. Environ. Manag. 315:115173. doi: 10.1016/j.jenvman.2022.115173
Crawford, R. H., and Stephan, A. (2020). Tiny house, tiny footprint? The potential for tiny houses to reduce residential greenhouse gas emissions. IOP Conf. Ser. Earth Environ. Sci. 588:022073. doi: 10.1088/1755-1315/588/2/022073
Cruz Rios, F., Grau, D., and Chong, W. K. (2019). Reusing exterior wall framing systems: a cradle-to-cradle comparative life cycle assessment. Waste Manag. 94, 120–135. doi: 10.1016/j.wasman.2019.05.040
Cuéllar-Franca, R. M., and Azapagic, A. (2012). Environmental impacts of the UK residential sector: life cycle assessment of houses. Build. Environ. 54, 86–99. doi: 10.1016/j.buildenv.2012.02.005
Curran, M. A. (2013). Life cycle assessment: a review of the methodology and its application to sustainability. Curr. Opin. Chem. Eng. 2, 273–277. doi: 10.1016/j.coche.2013.02.002
Dekker, E., Zijp, M. C., van de Kamp, M. E., Temme, E. H. M., and van Zelm, R. (2020). A taste of the new ReCiPe for life cycle assessment: consequences of the updated impact assessment method on food product LCAs. Int. J. Life Cycle Assess. 25, 2315–2324. doi: 10.1007/s11367-019-01653-3
Ding, Y., Pang, Z., Lan, K., Yao, Y., Panzarasa, G., Xu, L., et al. (2023). Emerging engineered wood for building applications. Chem. Rev. 123, 1843–1888. doi: 10.1021/acs.chemrev.2c00450
Elgzeary, M., Tatan, B., Ibrahim, O., and Gouda, H. (2024) “Life cycle assessment of conventional and sustainable villas in the United Arab Emirates: a comparative case study.”, in Proceedings of the world congress on new technologies. Avestia Publishing.
Fuller, R. J., and Crawford, R. H. (2011). Impact of past and future residential housing development patterns on energy demand and related emissions. J. Housing Built Environ. 26, 165–183. doi: 10.1007/s10901-011-9212-2
Giusti, L., and Almoosawi, M. (2017). Impact of building characteristics and occupants’ behaviour on the electricity consumption of households in Abu Dhabi (UAE). Energ. Build. 151, 534–547. doi: 10.1016/j.enbuild.2017.07.019
Guerra Santin, O., Itard, L., and Visscher, H. (2009). The effect of occupancy and building characteristics on energy use for space and water heating in Dutch residential stock. Energ. Build. 41, 1223–1232. doi: 10.1016/j.enbuild.2009.07.002
Günkaya, Z., Özkan, A., and Banar, M. (2021). The effect of energy-saving options on environmental performance of a building: a combination of energy audit–life cycle assessment for a university building. Environ. Sci. Pollut. Res. 28, 8822–8832. doi: 10.1007/s11356-020-11141-z
Heijungs, R., Huppes, G., and Guinée, J. B. (2010). Life cycle assessment and sustainability analysis of products, materials and technologies. Toward a scientific framework for sustainability life cycle analysis. Polym. Degrad. Stab. 95, 422–428. doi: 10.1016/j.polymdegradstab.2009.11.010
Herrmann, I. T., and Moltesen, A. (2015). Does it matter which life cycle assessment (LCA) tool you choose? – a comparative assessment of SimaPro and GaBi. J. Clean. Prod. 86, 163–169. doi: 10.1016/j.jclepro.2014.08.004
Hoogmartens, R., van Passel, S., van Acker, K., and Dubois, M. (2014). Bridging the gap between LCA, LCC and CBA as sustainability assessment tools. Environ. Impact Assess. Rev. 48, 27–33. doi: 10.1016/j.eiar.2014.05.001
Jin, T., and Kim, J. (2018). What is better for mitigating carbon emissions – renewable energy or nuclear energy? A panel data analysis. Renew. Sust. Energ. Rev. 91, 464–471. doi: 10.1016/j.rser.2018.04.022
Johst, P., Chatzipanagiotou, K. R., Kucher, M., Zschiebsch, W., Voigt, P., Breinl, D., et al. (2024). Concept and life cycle assessment of a tiny house made from root section structures of a decommissioned large-scale wind turbine blade as a repurposed application. Mat. Circ. Econ. 6:5. doi: 10.1007/s42824-023-00093-7
Kilman, C. (2010). Small house, big impact: the effect of tiny houses on community and environment. Available at: https://api.semanticscholar.org/CorpusID:43231304 (Accessed May 24, 2024).
Lin, M., Afshari, A., and Azar, E. (2018). A data-driven analysis of building energy use with emphasis on operation and maintenance: a case study from the UAE. J. Clean. Prod. 192, 169–178. doi: 10.1016/j.jclepro.2018.04.270
Mourão, J., Gomes, R., Matias, L., and Niza, S. (2019). Combining embodied and operational energy in buildings refurbishment assessment. Energ. Build. 197, 34–46. doi: 10.1016/j.enbuild.2019.05.033
Mukhopadhyay, J. (2020). Observations of energy consumption and IEQ in a “tiny house”. Build. Res. Inf. 48, 613–631. doi: 10.1080/09613218.2019.1661765
Nwodo, M. N., and Anumba, C. J. (2019). A review of life cycle assessment of buildings using a systematic approach. Build. Environ. 162:106290. doi: 10.1016/j.buildenv.2019.106290
Preethi Kavitha, S., Rajesh Banu, J., Arulazhagan, P., and Gunasekaran, M. (2020). “Chapter 17 - environmental impacts and sustainability assessment of food loss and waste valorization: value chain analysis of food consumption” in Food waste to valuable resources. eds. J. R. Banu, et al. (London, United Kingdom: Academic Press), 359–388.
Rahman, I. A., al Ameri, A. E. S., Memon, A. H., al-Emad, N., and Alhammadi, A. S. A. M. (2022). Structural relationship of causes and effects of construction changes: case of UAE Construction. Sustainability 14:596. doi: 10.3390/su14020596
Rai, R., Ranjan, R., and Dhar, P. (2022). Life cycle assessment of transparent wood production using emerging technologies and strategic scale-up framework. Sci. Total Environ. 846:157301. doi: 10.1016/j.scitotenv.2022.157301
Rauf, A., Attoye, D. E., and Crawford, R. (2022). Embodied and operational energy of a case study Villa in UAE with sensitivity analysis. Buildings 12:1469. doi: 10.3390/buildings12091469
Rauf, A., Attoye, D., and Crawford, R. (2023). Material service life and embodied energy: a residential Villa case study in the United Arab Emirates. SSRN Electronic Journal [Preprint].
Rauf, A., Shafiq, M. T., Khalfan, M. M. A., and Ulhaq, I. (2024). Sustainable water management in construction: life-cycle embodied water assessment of residential buildings. Built Environ. Project Asset Manag. 14, 682–696. doi: 10.1108/BEPAM-06-2023-0102
Roberts, M., Allen, S., and Coley, D. (2020). Life cycle assessment in the building design process – a systematic literature review. Build. Environ. 185:107274. doi: 10.1016/j.buildenv.2020.107274
Sadar Din, K. M., and Ishak, M. S. (2024). Sustainable building construction materials in the United Arab Emirates: a review. Sustainability 16:6565. doi: 10.3390/su16156565
Salameh, T., Alkhalidi, A., Hussien Rabaia, M. K., al Swailmeen, Y., Alroujmah, W., Ibrahim, M., et al. (2022). Optimization and life cycle analysis of solar-powered absorption chiller designed for a small house in the United Arab Emirates using evacuated tube technology. Renew. Energy 198, 200–212. doi: 10.1016/j.renene.2022.07.121
Shearer, H., and Burton, P. (2019). Towards a typology of tiny houses. Hous. Theory Soc. 36, 298–318. doi: 10.1080/14036096.2018.1487879
Shearer, H., and Burton, P. (2023). Tiny houses: movement or moment? Hous. Stud. 38, 360–382. doi: 10.1080/02673037.2021.1884203
Silva, D., Nunes, A., Moris, V., Piekarski, C., and Rodrigues, T. (2017). How important is the LCA software tool you choose comparative results from GaBi, openLCA, SimaPro and Umberto.
Stratton, M. J., and Corneal, L. M. (2023). Development of a tiny house design tool to increase safety, efficiency, and cost-effectiveness. Innov. Green Dev. 2:100052. doi: 10.1016/j.igd.2023.100052
Woodard, A. C., and Milner, H. R. (2016). “7 - sustainability of timber and wood in construction” in Sustainability of construction materials. ed. J. M. Khatib. Second ed (Duxford, United Kingdom: Woodhead Publishing), 129–157.
Keywords: tiny house, materials, life cycle assessment, environmental impact, energy
Citation: Sabobeh L, Al Hassani R, Alomar L, Atabay S, Mortula MM, Ali TA and Taher AM (2024) Life cycle assessment of the tiny house initiative in the United Arab Emirates. Front. Sustain. Cities. 6:1488269. doi: 10.3389/frsc.2024.1488269
Edited by:
Roberto Alonso González-Lezcano, CEU San Pablo University, SpainReviewed by:
Sofia Melero-Tur, CEU San Pablo University, SpainTamar Awad, Polytechnic University of Madrid, Spain
Copyright © 2024 Sabobeh, Al Hassani, Alomar, Atabay, Mortula, Ali and Taher. This is an open-access article distributed under the terms of the Creative Commons Attribution License (CC BY). The use, distribution or reproduction in other forums is permitted, provided the original author(s) and the copyright owner(s) are credited and that the original publication in this journal is cited, in accordance with accepted academic practice. No use, distribution or reproduction is permitted which does not comply with these terms.
*Correspondence: Serter Atabay, c2F0YWJheUBhdXMuZWR1