- Department of Architecture, Aalto University, Espoo, Finland
The life cycle climate impacts of urban parks are poorly known. Whereas vegetation and soils can be carbon sinks, building products, energy use, and processes cause emissions. Several studies acknowledge the need for further assessment of urban parks, especially regarding vegetation, soil organic carbon, management and design, together with the development of supportive tools for climate-wise planning. To deepen our understanding of carbon flows of urban parks, we applied life cycle assessment (LCA) and studied the carbon dioxide (CO2) emissions and removals of five urban parks in Helsinki, Finland. The components of the parks were divided into four categories: site preparation, covering and surface structures, vegetation and growing media, and systems and installations. According to our findings, CO2 emissions ranged from 27.08 to 61.45 kgCO2e/m2 and CO2 removals from 11.35 to 16.23 kgCO2e/m2 with uncertainty. Planted woody vegetation and existing forested areas had the highest CO2 uptake among the vegetation types. Moreover, growing media caused on average 35% of total CO2 emissions. As significant volumes of growing media remain necessary to support the growth and establishment of plantings, finding less emission intensive alternatives to peat-based growing medium becomes essential. Other main emissions sources included transportation, and replacements of surface materials, but their dominance is highly dependent on the design, use and maintenance of the park. LCA offers a robust assessment framework for the quantification of greenhouse gas emissions and is evolving towards the including of greenhouse gas removals and storages. However, the inclusion of living organisms would require changes in the mindset of LCA. The level of maturity in the assessment methods differs significantly between the park components. Data and methods are especially lacking for nursery production, maintenance and end-of-life phases of vegetation, soils, and mulches. We also identified uncertainties regarding the estimations of CO2 uptake by woody vegetation, lawns, and meadows due to software limitations and lack of data for local context. Simulating dynamic plantings raises additional questions, together with the forecast of accurate meteorological conditions of a changing climate. This research highlights the need for more holistic life cycle assessment of urban parks to inform low-carbon landscape industries.
1 Introduction
Urban parks have multiple recognized benefits in cities. These include various ecosystem services, such as sequestering carbon, supporting biodiversity, managing stormwater, or providing benefits for physical and psychological wellbeing of humans (Haase et al., 2014). The potential of urban green spaces for climate change mitigation is, however, not fully understood and requires further assessment. For instance, the development of a life cycle assessment framework is beneficial to recognize at which stage in the design process of urban parks decisions can be made to decrease greenhouse gas (GHG) emissions and increase carbon sequestration and storage (CSS).
Cities are key contributors in the global share of GHG emissions (United Nations Environment Programme, 2021). Urban areas are growing, and 68% of the global population is expected to live in cities by 2050 (United Nations, Department of Economic and Social Affairs, Population Division, 2019). According to the Intergovernmental Panel on Climate Change (IPCC), this global trend of urbanization can also promote opportunities for climate resilient development (IPCC, 2022). Cities are seeking ways to sequester and store carbon into the built environment (Dhakal, 2010; European Commission, 2013). Therefore, an increasing interest has developed for urban green infrastructure as a potential for urban carbon mitigation (Dhakal, 2010).
Urban parks are part of urban green infrastructure (UGI), a network of natural and semi-natural areas integrated in urban areas such as parks, forests, trees, allotment gardens, and private gardens (European Commission, 2013). UGI has the potential to provide multiple ecosystem services in cities, such as stormwater management, regulation of air quality, removal of pollutant, creation of wildlife habitat, and carbon sequestration and storage. The benefits of this network of green and blue spaces are recognized by the European Commission, where a Green Infrastructure Strategy was implemented to preserve and restore biodiversity as well as to promote nature-based solutions (European Commission, 2013). More recently, the Nature Restoration Law has been proposed to implement the EU Biodiversity Strategy 2030 and tackle the challenges of climate change and biodiversity loss in all ecosystems, also urban areas, and urban green spaces (European Commission, 2023).
Several studies have reported that urban parks can act as urban carbon sinks (Strohbach et al., 2012; Nicese et al., 2021). For instance, urban trees store carbon during their growth by fixing carbon during photosynthesis and can influence local climate and air temperatures (Nowak and Crane, 2002; Nowak et al., 2008). While providing other important ecosystem services, urban soils can store considerable amounts of soil organic carbon (SOC), which is a measurable component of soil organic matter (Pouyat et al., 2006; Dorendorf et al., 2015; Setälä et al., 2016; Vasenev and Kuzyakov, 2018; Lindén et al., 2020; Lu et al., 2021). As these studies illustrate, urban parks can play an important role in sequestering and storing carbon in urban areas. Although urban parks have several climate benefits, they also cause GHG emissions. These emissions are caused, for instance, by the maintenance activities, the construction operation, the production of growing media and vegetation plantings. These impacts are not yet fully understood from a life-cycle perspective, which can lead to undervaluing the environmental impacts (Nicese et al., 2021). Thus, studying urban parks through a life cycle approach and estimating potential GHG emissions and removals become relevant.
Life cycle assessment (LCA) is a methodology for quantifying environmental impacts during the life cycle of a product, a system, or a service, following international standards (CEN, 2011a). It is used broadly for studying various environmental impacts in the built environment. Currently, LCA is making its way to European building regulations (Dodd et al., 2021), and broader statistical surveys on the flows of carbon in the built environment have been carried out (Röck et al., 2020; Stephan et al., 2022). Urban parks are seldomly included in typical LCA studies due to lacking holistic LCA approach (Kuittinen et al., 2021). However, LCA for buildings includes—according to standard EN 15978 (CEN, 2011b)—everything that is within the site. This logically also means built greenery including planted vegetation and soils.
Previous studies have used LCA in the context of urban green to quantify CSS potentials in urban green parks (Strohbach et al., 2012; Nicese et al., 2021), to study the impact of maintenance and design scenarios on CSS of an urban park (Nowak et al., 2002; Strohbach et al., 2012) and to measure carbon emissions during nursery production of ornamental plants (McPherson et al., 2015; Lazzerini et al., 2016; Lazzerini et al., 2018) and trees (Ingram, 2012; Kendall and McPherson, 2012; Strohbach and Haase, 2012; Ariluoma et al., 2021). However, these studies have focused on parts of the life cycle, (e.g., nursery production or maintenance), or on a specific component, such as woody vegetation (Russo et al., 2014; Lind et al., 2023), soil (Canedoli et al., 2020; Richter et al., 2020), or lawn (Kong et al., 2014; Silvenius et al., 2016). The estimation of the carbon balance of an urban park which includes both green and grey infrastructure components, appears to be scarce, so does information that would be compatible with the LCA studies of the built environment. Furthermore, assessing CSS of woody vegetation and soils remains difficult at different urban scales as the growing conditions and maintenance can vary throughout the study assessment period that is set at the beginning of the LCA. Still, for getting a holistic picture of carbon flows in the built environment, the role of urban parks would require more investigations.
For addressing the above-mentioned knowledge gap, we present the LCA of five urban parks in the city of Helsinki, Finland. We seek to identify where gaps in data or scenarios may still become a hindrance for covering all life cycle stages or all components of the parks. The scope of the study includes the following: (1) testing the LCA approach and its applicability to urban parks, (2) determining a suitable life cycle inventory for an urban park, (3) estimating CO2 emissions and removals of an urban park, (4) identifying the available methods, data, and scenarios to implement a complete LCA, and (5) identifying the missing methods, data, and scenarios. Although the case studies presented in this article offer insight into typical urban parks of today, the results cannot be generalized. The emissions are relevant for the studied context only. However, the identified gaps in both methods and data can serve for further development of LCA of green infrastructure.
2 Materials and methods
2.1 The case study parks
The chosen case sites are recently built urban green spaces in Helsinki, Finland. Like many metropolitan cities, Helsinki is undergoing changes with the development of new neighborhood centers and the renovation of its existing urban fabric, including the implementation of new urban green spaces such as urban parks.
We studied five urban parks (UP): Hyväntoivonpuisto park (UP1), Saukonpaadenpuisto park (UP2), Taidemaalarinpuisto park (UP3), Vennynpuisto park (UP4) and Maunula playground and Maunulanpuisto park (UP5). All the parks are new constructions except for UP5, which is a renovation of an existing park. UP5 has been built and modified on several occasions, whereas the other parks are the result of a land use change and city development. The chosen parks represent different urban settings from city centre to new suburban development in addition to an older suburb. These case studies were selected because they all represent recently built and typical public urban green spaces in Helsinki which have been implemented between 2018 and 2022. Their sizes vary between 0.8 ha to 3.4 ha which represents a normal size of a neighborhood park. They all contain typical green elements and materials used in landscape construction and thus form a valid basis for life cycle assessment analysis. The UPs include all or some of the following elements: lawns, meadows, woody vegetation plantings, perennial and annual plantings, urban farming, playgrounds, bike lanes, sport fields, furniture, equipment, and pathways. Figure 1 presents the share of the vegetated land cover types in the parks. More information about the parks can be found in Appendices A, B.
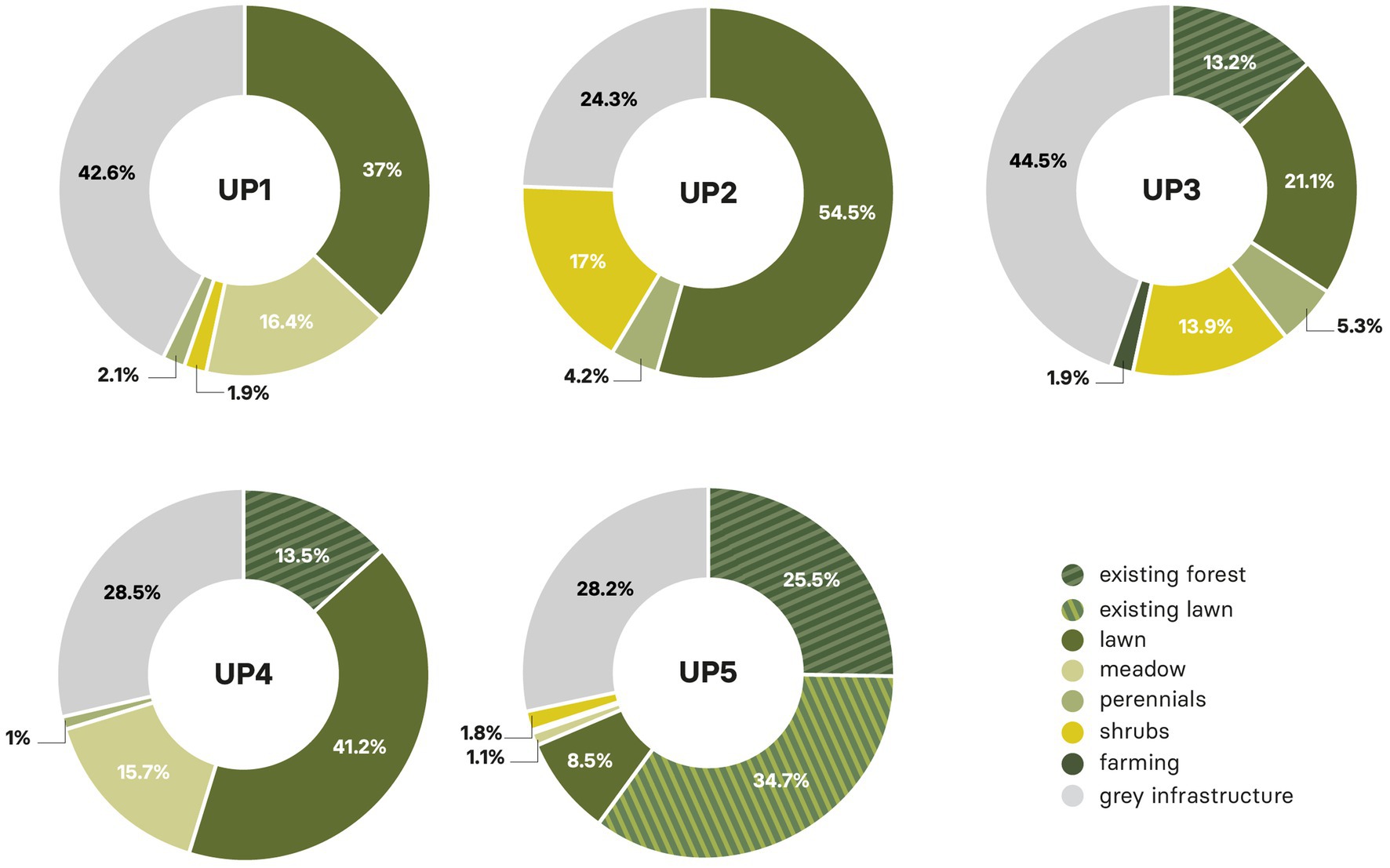
Figure 1. Share of the vegetated land cover types in percentages in Hyväntoivonpuisto (UP1), Saukonpaadenpuisto (UP2), Taidemaalarinpuisto (UP3), Vennynpuisto (UP4) and Maunulanpuisto (UP5). Trees are included within these land cover types.
2.2 Life cycle assessment in this study
This study applied a process based, attributional LCA. As there are no dedicated standards for LCA of parks and green infrastructure, we followed standard EN 15978 (CEN, 2011b) which guides LCA of buildings, in line with ISO 14040 standard series. Hence, our results are methodologically compatible with the LCA studies typically applied to the built environment. This is important for understanding GHG emissions and removals in the built environment and for identifying designer’s possibilities to influence climate-friendly solutions in city planning, landscape design, and building design.
In our study, we chose a limited temporal system boundary, as presented, and explained in section 2.7. We made the assessment for a reference study period (RSP) of 50 years, which is most common in LCA in the built environment, and the default in EU’s joint Level(s) framework (Dodd et al., 2021). For quantifying the climate impacts, we chose to allocate the GHG emissions and removals in relation to the area of the studied parks; in other words, the functional unit was m2 of park.
In this study, we are focusing on one greenhouse gas, specifically carbon dioxide (CO2). Where needed, we have translated carbon into carbon dioxide by multiplying C with the factor of 3.67, according to standard EN16449 (CEN, 2014). However, we present the emissions that are associated to the products and construction in CO2 equivalents (CO2e), as the national generic database does not allow for separation between the GWP of CO2 and other GHGs. The uptakes and removals are modelled only for CO2, again due to the limitations of data and tools. Hence, the relative impact of the emissions is slightly higher than that of the removals, and our findings therefore include a slight underestimation of the full GHG removal potential of the green infrastructure. We present CO2 removals and uptakes as negative values and CO2 emissions as positive values, according to the calculation principles of the abovementioned standards.
2.3 Inventory of utilized resources
We conducted a life cycle inventory (LCI) analysis for each park, considering all materials, planted vegetations and processes occurring during the RSP. The data was retrieved from “as-designed” stage documents and bill of quantities taken from the Fore software (Fore, 2023). Fore is an infrastructure cost management tool commonly used in Finland and it allows to estimate the costs of a construction project after entering quantities of materials. Due to data availability and some parks still being under construction, areas of the parks were excluded from the study (this applies to UP1 and UP2). However, they are still representative of the whole park with the same functionalities and structure types. Site boundaries and structure types of parks are presented in Appendix B.
2.4 Included and excluded components in study
We categorized the components of the parks as follows: (1) Site preparation including removal of soil and vegetation, excavations, embankments, fills, and base structures; (2) Covering and surface structures, with load-bearing and non-bearing layers, surface materials and edges; (3) Vegetation and growing media, with plantings, soils, mulches and supporting structures; and (4) Systems and installations with stormwater drainage systems, lighting poles, fences and additional structures such as pavilion or wooden platforms.
During the inventory, we observed a high degree of ambivalence in assigning components to a certain category, which makes this categorization context and case specific. The applied physical system boundary—i.e., the included and excluded components—is presented in Table 1.
We have excluded some components mostly due to the unavailability of environmental data. Especially street furniture and playground equipment are very varying in their materials and service lives. Environmental information for fertilizers was scarce, and compiling scenarios for their use and shifts in the fertilizer type within the RSP could not be carried out in this study.
2.5 Inventory of CO2 emissions during the life cycle of the parks
2.5.1 Production of building products (modules A1–A3)
The global warming potential (GWP) values for the building products (hereafter “grey infrastructure”) accounted in site preparation, covering and surface structures, vegetation and growing media and systems and installations were taken from the Finnish generic database CO2data.fi (SYKE, 2023) for modules A1–A3, A4 and B4. If a material could not be found in the national database, the emission values were retrieved from LCA tool One Click LCA (2023).
2.5.2 Nursery production (modules A1–A3)
For assessing the impacts of nursery production of woody vegetation and perennials, we divided the plants into six categories that are most common in Finland: container tree, field-grown tree, container shrub, field-grown shrub, perennials, and imported shrubs. We then divided these categories of plants into two main production types: field production and, container production which refers to potted-grown plants. Field-grown plants have a cultivation period of 3…6 years while container-grown plants cultivation is 1-year long for perennials and between 3…4 years for small trees and shrubs. Data to estimate CO2 emissions occurring during the nursery production of woody vegetation was taken from existing literature (Lazzerini et al., 2016, 2018) as there is currently no data available for the Finnish context. The data was distinguished between container and field production. For the cultivation period, we defined an average of 3.5 years for container-grown plants and 5 years for field-grown plants. The emission value to produce perennials was retrieved from CO2data.fi. Estimation of the CO2 uptake during the nursery production of woody vegetation was included in the estimation of the total biomass accumulation of the plants, and hence combined to the results of the use phase (B).
2.5.3 Transportation (modules A4, B4, and C2)
The transportation was estimated with the method from the Ministry of the Environment (2019). The emissions were calculated by considering that the load filling rate was 80% on the outward journey and 0% on the return journey for all materials. The load filling rate for soils and aggregates taken away from the site during the construction process was estimated as 100%. We estimated conservatively that CO2 emissions per tonne-km for road transport in the end-of-life would be 43% of those in the construction stage; assuming conservatively a shift of fuels to renewable diesel instead of electricity or ethanol (Liimatainen et al., 2018).
Transportation distances were estimated based on the bill of quantities provided by the City of Helsinki, whenever applicable. If the distance was no specified, the nearest manufacturer in Finland was considered to calculate the distances.
2.5.4 Construction of the parks (module A5)
The demolition of existing infrastructure on the site, such as municipal water and sewage pipes, asphalt, paving and buildings, were excluded. In conventional LCA, they are considered as part of the end-of-life of the previous project. In the broader picture, however, all actions on the site will cause disturbances to existing carbon storages (in vegetation and soil, Bae and Ryu, 2015), as well as to the overall CO2 sequestration capacity of the area (land use and its changes). Following our intent of testing the suitability of LCA, we considered the removal of soil and existing vegetation for getting better understanding on the flows of CO2 in these elements. Their impacts were estimated based on the forest information provided by Natural Resources Institute of Finland (LUKE, 2023) for vegetation and from existing literature (Lindén et al., 2020) for soils. Earthworks (excavation, fillings, and associated machine work) were estimated with 7 kgCO2e/m2 following the national generic database. Other operations occurring during the construction of the urban parks were not included in the scope of the study due to lack of data.
2.5.5 Maintenance of the parks (module B2)
Maintenance was estimated for the mowing of lawns and meadows and pruning of the woody vegetation. We evaluated four mowing per season for lawns and two mowing for meadows. For the machinery, we considered the use of 5 L/ha per mowing for the first 10 years (Silvenius et al., 2016), 2.5 L the next 10 years and electric machinery for the last 30 years to simulate a scenario of decarbonization. The cuttings were left on the ground after the mowing. Emissions caused by the decomposition of cuttings were not included due to shortage of data. For the woody vegetation, we estimated that 10% of trees and shrubs were pruned during the use of the parks. Half of the pruned biomass was assumed to be used as wood products and other half for firewood. Future studies should include the decomposition of cuttings, application of fertilizers, replacement or addition of new plantings and waste management.
For the grey infrastructure, maintenance would include cleaning (summer) and ploughing of snow and sanding of pavements (winter). Because we encountered a major shortcoming of both data and uncertainty of the planned maintenance classes for the studied parks, the maintenance of grey infrastructure was excluded from the LCA but included in the estimation of the maturity of the assessment methods.
2.5.6 Replacements of paved areas, play areas and traffic areas (module B4)
Replacements of paved areas, play areas and traffic areas were estimated using typical, statistical service lives of their components and products. Data was retrieved from Environmental Product Declarations (EPDs) or from generic national database CO2data.fi.
2.5.7 Operational energy use (module B6)
For the use of energy in the parks, we estimated the CO2 emissions connected to lighting for each park. The annual electricity consumption estimations were retrieved from the lighting planning documents provided by the City of Helsinki. The estimations include the nominal power for each light feature present in the UP but do not consider possible night dimming or shutdowns, for which there were no evidence to make future scenarios. We used One Click LCA software to calculate the CO2 emissions for a 50-year period.
2.5.8 End-of-life phases
The demolition (C1) of the parks was assumed to contain same amount of energy use as the construction (A5) of the parks. However, as fuels would likely decarbonize, we have applied a reduction of the CO2 emissions similarly as for transportation fuels. Transportation of demolition waste and the decarbonization of the fuels are described above in Section 2.5.3.
End-of-life (C3–C4) was estimated with One Click LCA following Level(s) life-cycle carbon tool. For the green infrastructure, we assumed two scenarios, one where there is no land use change and the vegetation and soils would remain, and one where the land use is changing, and the vegetation and soils would be removed.
2.6 Inventory of CO2 removals and temporal storages
We estimated potential CO2 removals and their temporal storages in (whenever applicable) woody vegetation, soils and growing media below all vegetation types, mulches, wood products and concrete paving. We excluded the removals of other GHGs, such as methane, due to lack of data.
2.6.1 Woody vegetation
The CSS potential of trees for 50 years was estimated with i-Tree Eco v6.0.31 software (i-Tree, 2023) developed by the USDA Forest Service. Kumpula, Helsinki was used as the nearest meteorological station available. We chose the latest meteorological profile available from 2021 which did not include pollution data. The diameter at breast height (DBH) at the time of planting and respective tree species name were minimum input requirements in the software. DBH and tree species were retrieved from the landscape design documents. If a tree species could not be found in i-Tree Eco, the nearest taxon in terms of attributes and biomass within the same species family was chosen instead. Full list of trees and shrubs assessed in the study is presented as additional material (Appendices C, D).
To improve the model estimations, general site input fields and tree detail fields were added: status (chosen as planted), land use category (chosen as park or forest according to the maintenance categories of each park), additional tree characteristic (whether it is a street tree or a park tree) and the crown health condition (chosen as 90–95%, equivalent to good condition). The crown light exposure was chosen as default by the software which corresponds to partial sun condition. We utilized the forecast function of i-Tree Eco to simulate the total carbon sequestration of the trees after 50 years. We applied a medium annual mortality rate of 2% to this forecast based on existing literature (Strohbach et al., 2012; Ariluoma et al., 2021). The total carbon sequestration for each park was then translated to carbon dioxide using a constant factor of 3.67 as explained in Section 2.3.
The CSS of shrubs was estimated in the same manner as for the trees whenever applicable. When a species was less than 70–80 cm in height, the software could not forecast the carbon sequestration potential after 50 years as some of these species would most likely have a shorter life. Therefore, all species under 70 cm in height at time of planting were excluded from the simulation.
2.6.2 CO2 uptake into soils, growing media and mulches
Estimation on the CO2 uptake capacity of local soil types was taken from Helsinki Region Environmental Services Authority Report (HSY, 2019), in which annual changes in CO2 uptake in the Helsinki Metropolitan area are presented based on field surveys. According to the report, existing forested areas were found as sinks with 0.17 kgCO2/m2/a for the soils and 0.85 kgCO2/m2/a for the vegetation. Built green spaces were found as emission sources with 0.07 kgCO2/m2/a for the soils. The values were measured as stocks and changes overtime, considering the accumulating organic matter, carbon content baseline in the soil, as well as weather conditions.
As there is currently no data available for mulches, we considered it as a wood product and retrieved the biogenic data from CO2data.fi.
2.6.3 Bio-based products, and carbonation of concrete elements
During the use of the parks, there can be uptake of CO2 also into building products. Carbonation in concrete (a chemical counter-reaction to calcination that happens during the production of cement (Xi et al., 2016)) was estimated following the principles of standard EN 16757 (CEN, 2022).
We assumed biogenic carbon neutrality over the life cycle of wooden construction products according to standard EN 15804 (CEN, 2019). Wooden products were given negative GWP (removal) in the production stage and an equivalent positive GWP (emission) in the end-of-life stage.
2.7 Summary of the applied methods
Table 2 presents a summary of the methods used to estimate CO2 emissions and CO2 removals for the four categories of the UPs.
3 Results
3.1 Life cycle CO2 flows in urban parks
The five urban parks considered in this study showed varying emissions and removal potentials during the 50-year study reference period (see Figures 2, 3 and Table 3). The total balance ranges from 11.93 to 45.22 kgCO2e/m2.
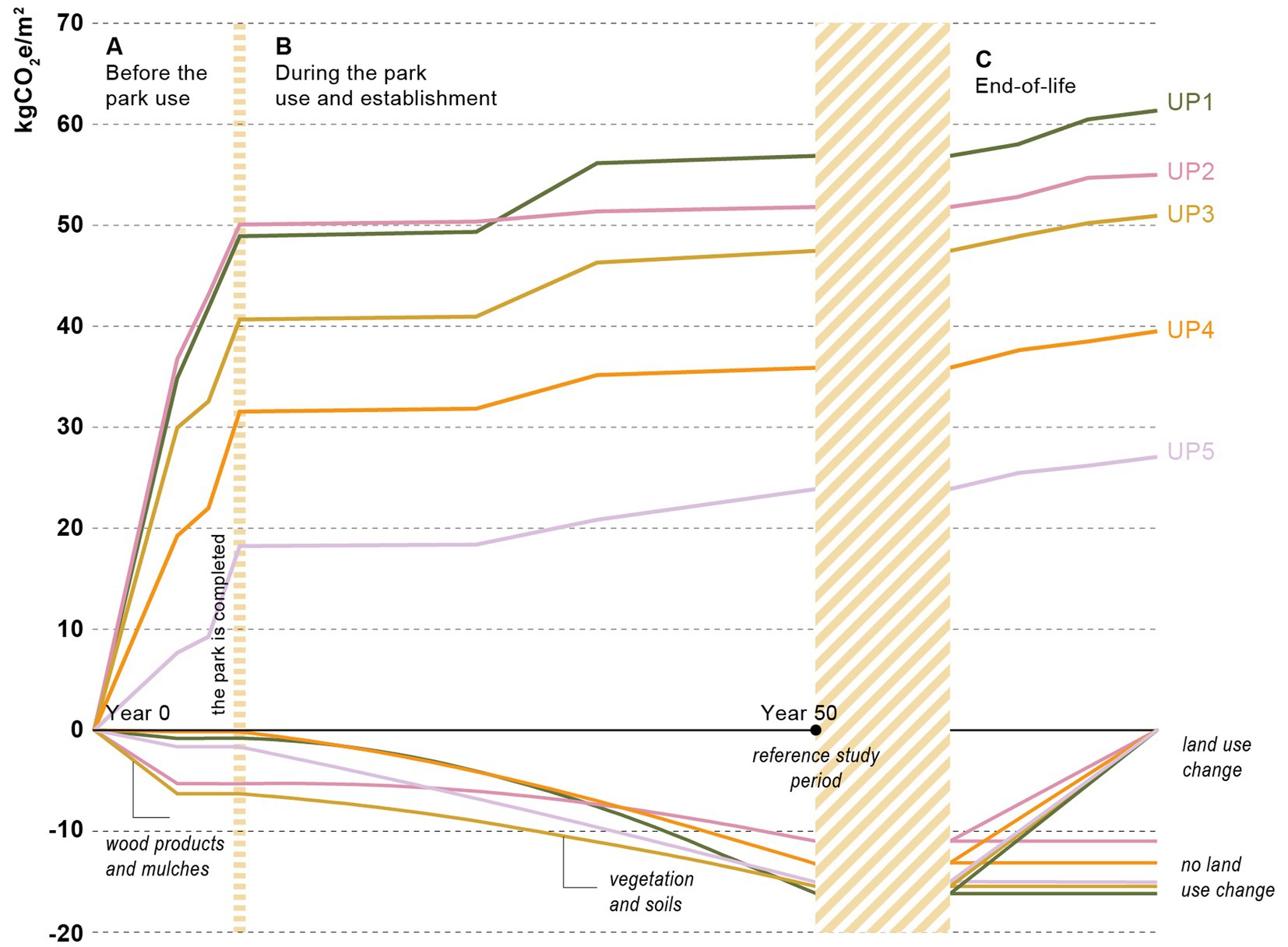
Figure 2. CO2 flows over 50 years. The positive values represent the cumulated emissions, and the negative values display the cumulated removals.
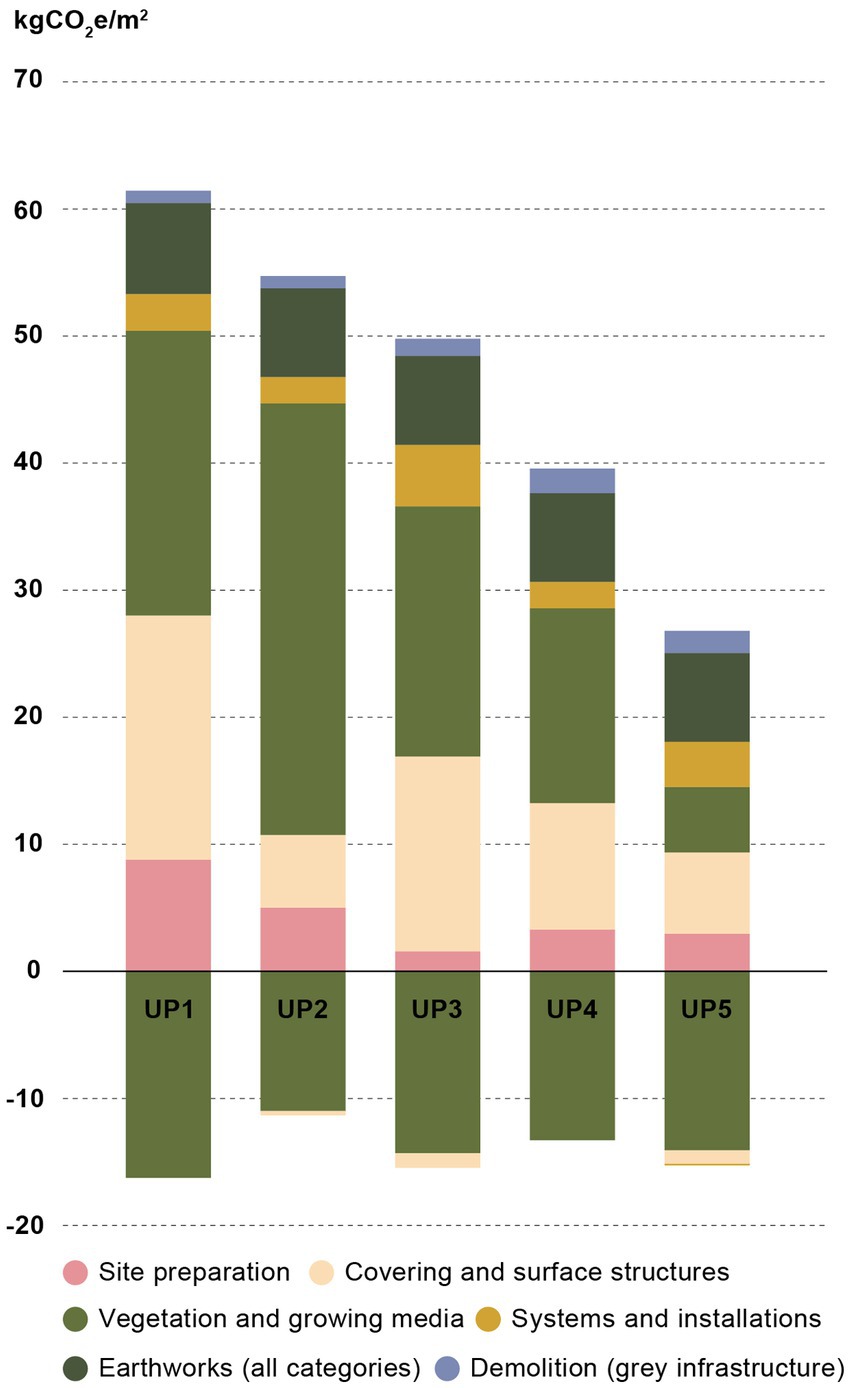
Figure 3. Distribution of CO2 emissions and removals per park category in kgCO2e/m2 in the scenario of no land use change. The negative values refer to removals. Earthworks (A5) and Demolition (C1) could not be differentiated among the park categories and are presented as a combined value for the park.
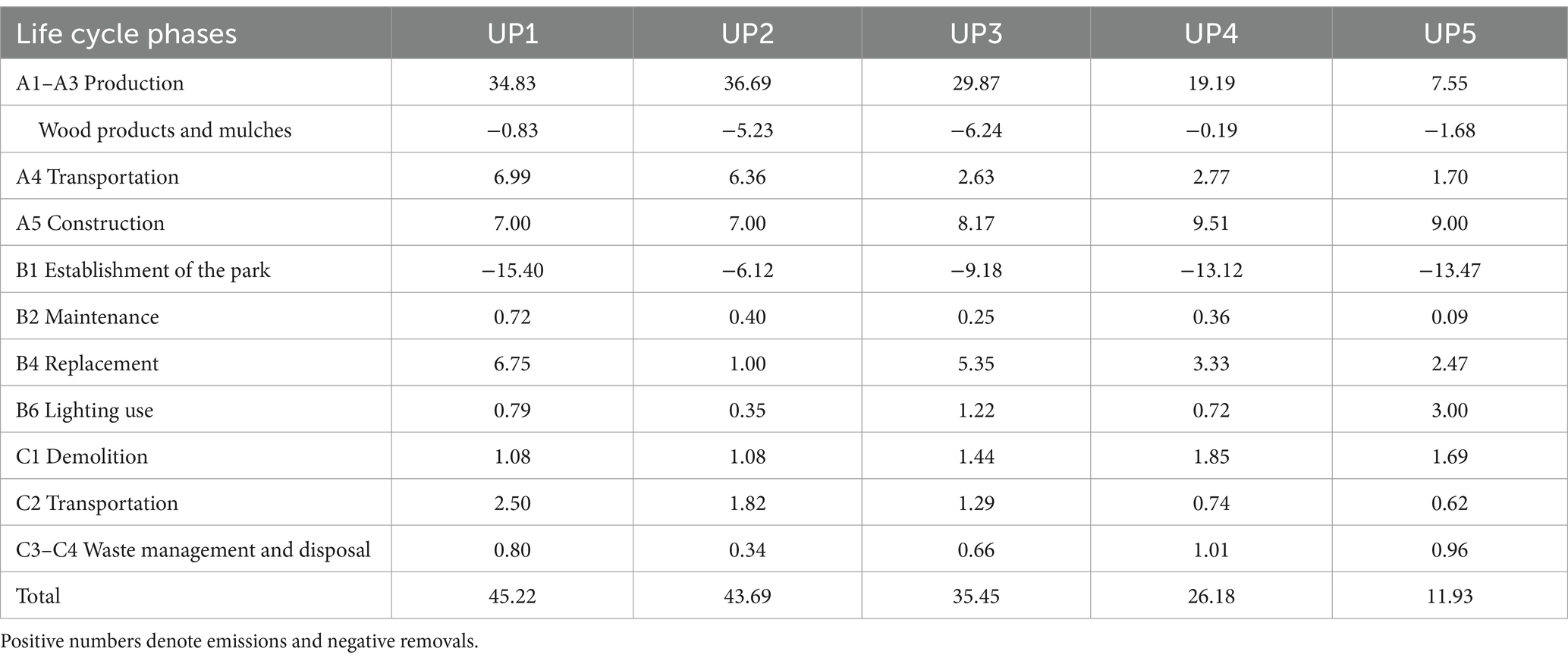
Table 3. CO2 balance of the urban parks over a period of 50 years (kgCO2e/m2) in the scenario of no land use change.
According to our findings growing media, load-bearing and non-bearing layers, removal of soil and vegetation, bounded surface materials (asphalt) and surfaces for sport, play and recreational activities have the highest CO2 emissions during a 50-year RSP (Figure 4). They range very broadly depending on the case, from 2 to 63% of the total emissions. This great variance is explained by the large volumes of growing medium and gravel fills required to build the park and the large areas covered by non-permeable surfaces in some parks. The most contributing park component for all the cases is growing medium due to their peat content. Other contributing components are load-bearing and non-bearing layers which are in significant use in the parks. The parks which reused nearby volumes of aggregates have lower emissions (UP3 and UP4) for this component. The same observation can be made for site preparation and foundation structures.
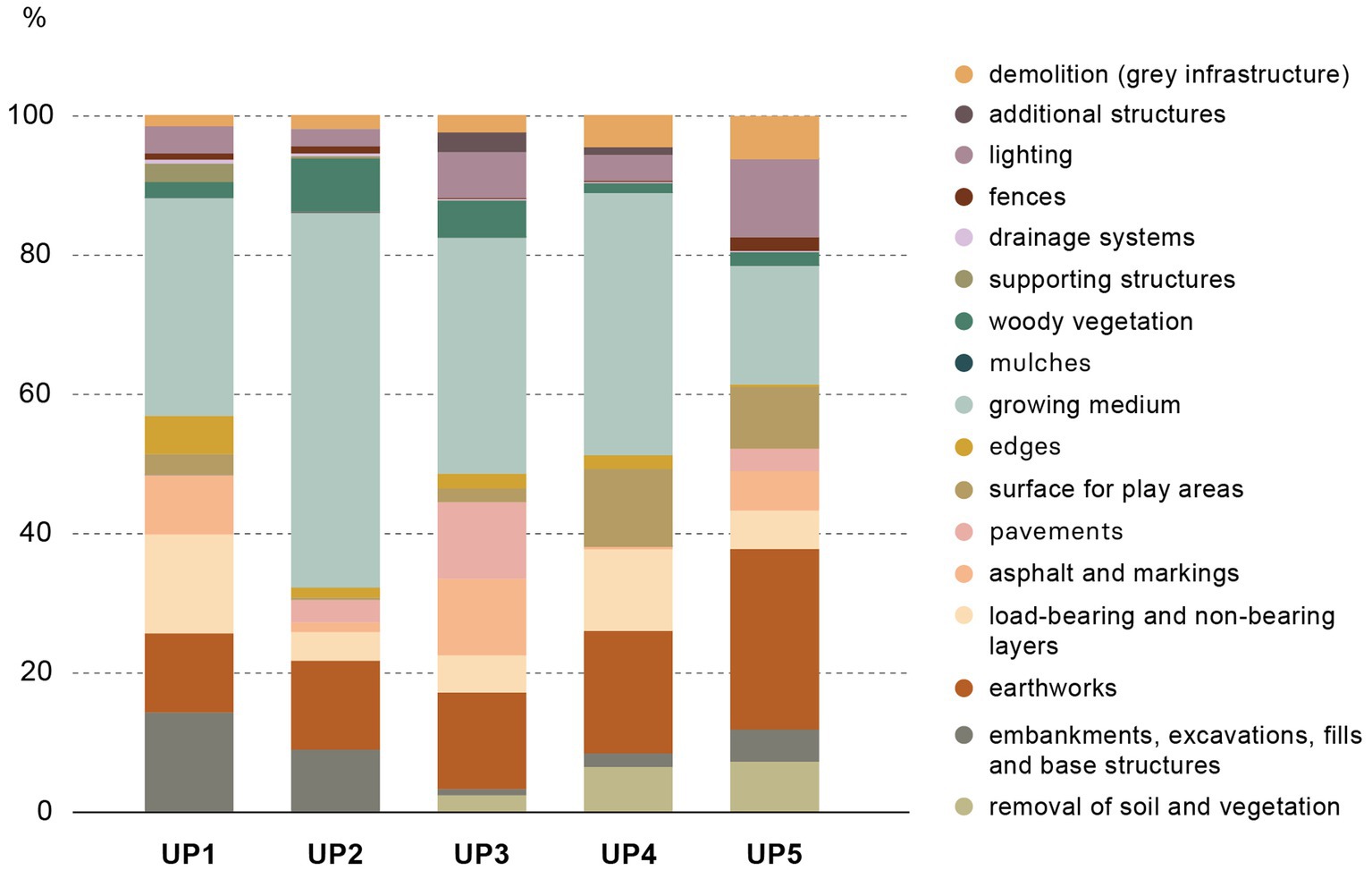
Figure 4. Share of CO2 emissions in percentages between the different components of the parks in the scenario of no land use change. Earthworks (A5) and Demolition (C1) could not be differentiated among the components of the parks and are represented as a combined value for the park.
Preserving existing forested areas and trees has greatest potential for sequestering CO2. Existing forested areas can sequester CO2 that corresponds to 10…48% of the emissions of the parks. Planted woody vegetation can uptake CO2 that corresponds to 8…28% of the emissions. Hence, there is a potential to balance emissions, but the impact varies depending on wood species and climate conditions.
Emissions from production stage range from 7.55 to 34.83 kgCO2e/m2, while CO2 stored into wood products and mulches varies between −6.24 and −0.83 kgCO2e/m2. Vegetation and growing media cause 60 to 86% of the emissions, depending on the composition of the growing medium (see Appendix E1). The second most contributing category is covering and surface structures ranging from 10 to 29% of the total share of emissions for this module. Emissions from transportation vary between 1.70 and 6.99 kgCO2e/m2 (see Appendix E2). The transportation of covering and surface structures are the most contributing factor for UP1, UP3 and UP4, whereas site preparation is the most contributing category for UP2 and UP5.
According to our findings, planted woody vegetation and existing forested areas remove most CO2 from the atmosphere as presented in Table 4. After 50 years, between 6.11 kgCO2e/m2 (1.66 kgC/m2) and 15.40 kgCO2e/m2 (4.20 kgC/m2) could be sequestered into the planted woody vegetation and soils in the studied urban parks. However, CO2 removals through the carbonation of concrete were found to be marginal.
Emissions for maintenance of the vegetation vary between 0.09 and 0.72 kgCO2e/m2. The pruning of trees and shrubs represent the highest share of these emissions with more than 85% for all the parks. Emissions from replacements vary between 1.00 and 6.75 kgCO2e/m2 (see Appendix E3). The most contributing category is covering and surface structures for all parks ranging between 43 and 100% of the total share of emissions. The differences between the parks are explained by the variety in quantity of materials such as asphalt, street markings and surface materials for play areas (rubber flooring and artificial grass flooring) which need to be replaced several times during the 50-year study reference period. Emissions from the production of electricity for lighting vary between 0.35 and 3 kgCO2e/m2. The calculations include a decarbonization scenario for electricity, as described in the national emission database (SYKE, 2023).
3.2 Sensitivity analysis
3.2.1 The role of growing media and transportation distances
We formulated the following scenarios to evaluate the influence of growing medium components and transportation distances: (a) distances for transportation of soils and aggregates are doubled (modules A4 and C2), (b) distances for transportation of soils and aggregates are divided by half (A4 and C2) and (c) no peat is used in the production of growing medium. Table 5 shows the results of this sensitivity analysis.

Table 5. Sensitivity of different transportation distances and peat contents in growing medium (change in percentages).
In scenario a, the increase is highest for the parks where significant volumes of aggregates were brought to the site. Hence, longer distances can significantly increase the CO2 emissions. Same can be observed in scenario b where the decrease is the largest for UP1. In scenario c, replacing the peat in growing medium decreases the emissions by up to 27.9% in UP2.
3.2.2 Reporting of the release of biomass carbon contents
An important part of the sensitivity analysis is the scenario for the reporting of organic carbon contents of vegetation and soils at the end-of-life. During the growth of plants, atmospheric carbon accumulates into biomass. Organic carbon also accumulates into soils over time.
We have considered the uptake of carbon during the growth of plants (module B1) as a reduction to life cycle emissions, according to ISO 14067 (ISO, 2018). In the end-of-life phase, we have excluded the accumulation of organic carbon from our results. It is not an inherent (industrial) material property, but a result of ecosystem services and hence outside of the technosphere. This reporting option is shown as Alternative 1 in Table 6. Also, the carbon uptake potential of vegetation and soils during the use of parks could be excluded, and no emission reductions would be shown in module B1 (Alternative 2). A literal interpretation of EN 15804 could also lead into reporting the end-of-life biomasses and carbon contents of vegetation and soils as emissions at the time of their removal. Using this reporting option and neglecting the uptake of carbon during the use phase gives another picture of life cycle CO2 flows (Alternative 3).

Table 6. Sensitivity of the CO2 flows to different ways of reporting the release of biomass carbon at the end-of-life phase (kgCO2e/m2).
These alternatives would lead into confusingly different results in LCA (Table 6). Alternative 2 neglects fundamental ecosystem services and in Alternative 3, the misleading conclusion is that photosynthesis would cause adverse climate impacts. Therefore, it is necessary to develop instructions for the reporting of accumulated organic carbon in LCA standards. Our proposal is to utilize Alternative 1 and to exclude the accumulation of living biomass and soil organic carbon from the end-of-life release of biogenic carbon.
4 Discussion
4.1 Comparison to other studies
The CO2 uptake by planted woody vegetation after 50 years is within the range of other studies (all units in kgCO2/m2): 3.8–22.6 in an urban green space in Leipzig, Germany (Strohbach et al., 2012), and 2.4 for trees in a residential courtyard in Helsinki, Finland (Ariluoma et al., 2021). Nowak et al. (2013) found a net carbon sequestration average of 0.205 kgC/m2/year per tree cover per year when studying urban trees in the USA. Nicese et al. (2021) found a total of 47 kgCO2/m2 in a park in metropolitan Milan, Italy. The study includes a large part of forested areas which can explain the higher value. The CO2 uptake will vary with the assumptions made during the calculations (mortality rate, growth conditions), the species in study and climate conditions.
In our findings, the CO2 emissions related to construction are higher than in previous studies (all units in kgCO2e/m2): 0.48 in Leipzig, Germany (Strohbach et al., 2012), and 1 in Italy (Nicese et al., 2021). These studies focused only on woody vegetation and included in parts: delivery of trees, excavation, planting, and transportation of workers, equipment, and machinery. In our study we considered the construction of the entire park which leads to higher emissions. In Los Angeles, USA, McPherson and Kendall (2014) estimated total emissions of 185.7 kgCO2e/per tree planted for the 40-year reference study period. They found that street tree represents around 66% of the emissions while park trees and yard trees accounted for, respectively, 13 and 21%. Emissions related to maintenance are within the range as earlier studies (all units in kgCO2e/m2): 0.25–0.47 in Leipzig (Strohbach et al., 2012) and 2.5 in Milan. Zhang et al. (2022) estimated annual emissions of 7.5 MgCO2e/ha in parks in Tiajin, China but with variations in emissions every year during 50 years of maintenance. The study included fertilizer use, pesticide use and irrigation which explains higher emissions. Maintenance practices also vary depending on the cities and climate conditions.
4.2 Assessment methods and their maturity
In this study, our aim was to test a standard based LCA method. It offers a robust assessment framework for the quantification of GHG emissions and is evolving towards the including of GHG removals and storages. However, it has initially been developed for industrial products and processes. The inclusion of living organisms would be a shift in the application and mindset of LCA. Nevertheless, LCA is widely used for agricultural products, and therefore its extension towards plants and soils can be considered.
An alternative to LCA would be material flow analysis (MFA), when adjusted to tracking flows of CO2. However, the same underlying questions of data gaps and uncertainties would follow, and MFA-based approach would be incompatible with the emerging regulation of GHG emissions in the built environment.
Precise measurements, statistics and modelling based on them could also be utilized. These would be especially suitable for measuring soil organic carbon through samples, or the biomass of trees through, e.g., remote sensing. However, measurement-based approaches could not cover the entire park and the diversity of vegetation types and can be only conducted at a single point in time. Hence, building dataset that would allow for, e.g., extrapolation of CO2 flows for future scenarios would require years of observations. Various biogenic CO2 modelling techniques, based on measurements, can also be used to estimate the magnitude and variability of carbon sinks in urban areas (Havu et al., 2024). This can give a valuable knowledge base for developing the datasets that are required for LCA.
As LCA is already making its way to regulations, its application to tracking of the flows of CO2 in the urban context is worth studying further. Main question is the maturity of the process.
Based on the study we formulate a matrix to summarize the maturity level of assessment methods (Figure 5). The availability of methods and data varies widely between the life cycle modules and park components. In modules A1–A3, the lack of knowledge and suitable calculation methods for nursery production plants includes both emissions and sequestration and storage in woody vegetation and soil during the production. There are knowledge gaps also for the maintenance of urban green infrastructure and end-of-life of vegetation, soils, and mulches.
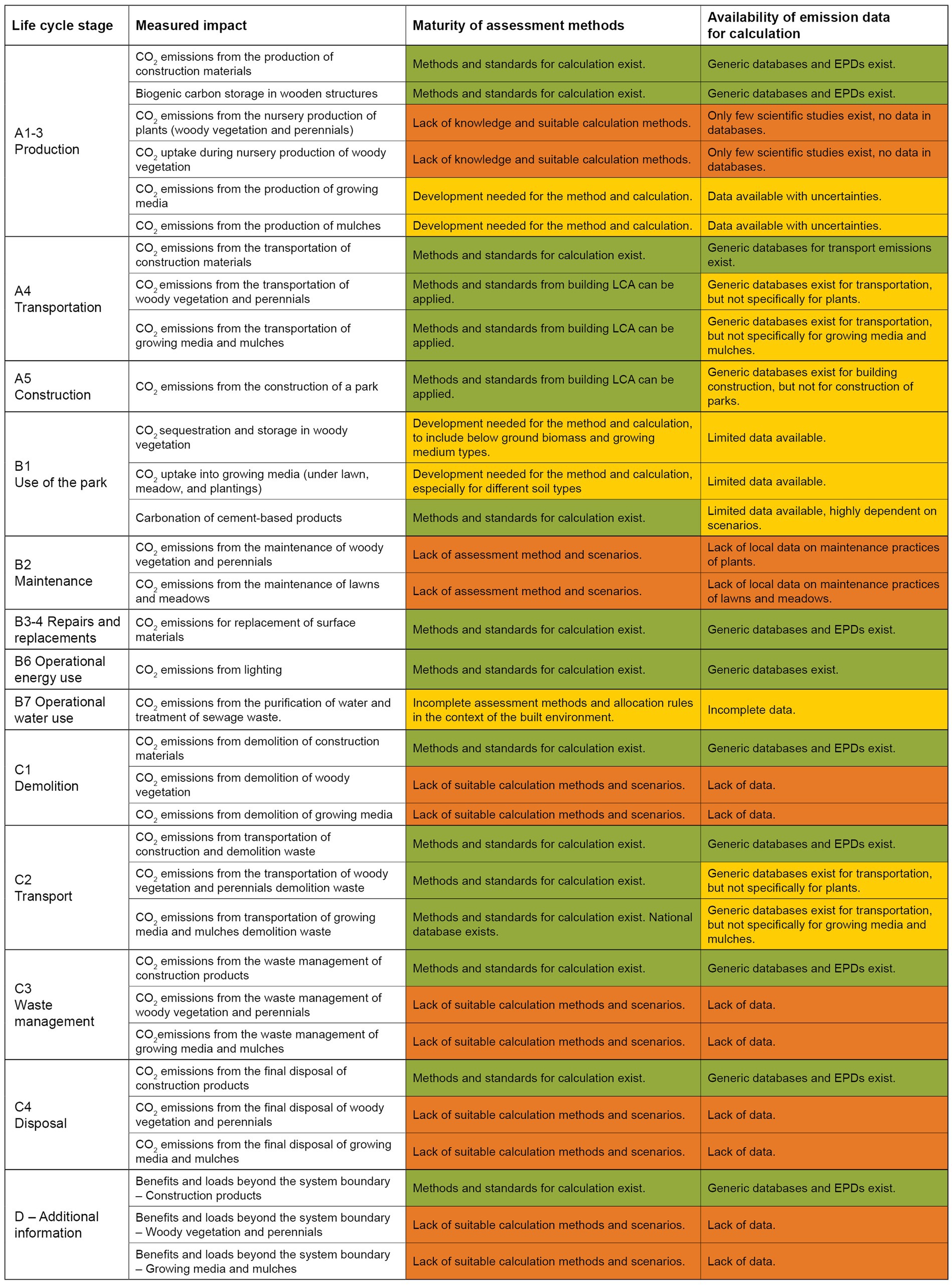
Figure 5. Maturity levels of LCA methods and data. Color coding: green = mature, yellow = available but immature, orange = method unavailable or very uncertain.
4.3 Implications for the design and construction of urban green infrastructure
The study has several implications for the design, construction, and maintenance of urban green infrastructure. Our results show the dominance of the production phase impacts among the studied life cycle stages (Figure 2). This indicates that CO2 emissions are significantly influenced by design choices, functional needs, or by the requirements of the city plan. It also means there are possibilities for reducing these emissions already at the design stage. For example, there are guidance publications for mitigating the production stage impacts in the built environment (World Green Building Council, 2019; LETI, 2023). Many of these principles—low-carbon machinery and logistics, use of local soil and rock to reduce transport, use of recycled and recovered materials and selection of low-carbon concrete and steel as well as wooden materials—could also be applied to the design of parks.
In the early planning phase, the primary means to enhance carbon pools is the preservation of existing vegetation and soil whenever possible. Existing carbon stocks are of primary importance to preserve (Havu et al., 2024). The context of the built park impacts largely pre-construction needs, for example building on brownfield development requires more pre-construction than utilising existing natural elements on greenfield development. In the design and construction phase, a critical question is the growing medium when establishing new plantings (Figure 4). As significant volumes of growing media remain necessary to support the growth and establishment of plantings, finding less emission intensive alternatives to peat-based growing medium becomes essential. For instance, some studies show that the use of recycled and compost or biochar-based products without peat would significantly decrease emissions (Margenot et al., 2018; Ariluoma et al., 2024; Hashemi et al., 2024). However, the quality and additives of the growing medium affect the growth of the vegetation as well as the accumulation of soil organic carbon and this requires further investigation. Balancing these different aspects from a life cycle perspective requires understanding of the interplay of various factors of the UGI.
Regarding other individual components, it would appear relevant to focus on covering and surface materials which have the second highest contribution to CO2 emissions (Figure 3). It is recommendable to use recycled paving materials, e.g., from another construction project and materials with low embodied carbon and long service life. The coordination of the transport and exchange of soil and rock masses during site preparation would decrease emissions in the construction phase. During the maintenance phase, supporting the good growth of the vegetation and ensuring the long-life span of especially urban trees improve CO2 sequestration and storage and support the park to thrive (Ariluoma et al., 2023). It is also important to develop low-emission maintenance practices (Nowak et al., 2002). Questioning the established idea of highly maintained urban green spaces and the use of low-maintenance vegetation types that mimic natural ecosystems (e.g., meadows instead of lawns) would support carbon-smartness and biodiversity of urban green infrastructure (Lerman and Contosta, 2019; Ignatieva et al., 2020).
4.4 The unknowns: discovered uncertainties, gaps, and limitations
In this study, we encountered important shortcomings in the maturity of both data and methods, as presented in Figure 5. The role of maintenance appears to be a major knowledge gap. Environmental impacts of different seasonal maintenance activities are poorly known, and their future scenarios (due to changing weather) are insufficiently modelled. Also, the management of organic waste management is poorly known. Anaerobic decomposition would cause different impacts compared to burning or aerobic decomposition. There is also lack of data on nursery production in the Finnish context.
In our study, newly built lawn and meadow areas were estimated as yearly carbon sources in the module B1 following the available data from HSY (2019), as mentioned in the methods section. It is possible that these areas will become carbon sinks after some years and therefore play a more significant role in sequestering carbon from the atmosphere. The types of growing medium used for the vegetation, lawns and meadows were also not specified in the documents. This is a source of uncertainty. We utilized emission values from the generic database (SYKE, 2023). The growing media composition has a significant impact: for example, recycled soils and using compost or biochar in growing media produce less emissions than standardized soils (Silvenius et al., 2016; Havu et al., 2022).
There is high uncertainty regarding the estimations of removal of existing vegetation and soil. As the data was limited (tree species and size, soil types, waste management information), the actual impact may vary significantly. In addition, data regarding the removal or storage of other GHGs than carbon dioxide appears to be very incomplete, which is why we could not consider them while modelling the removals in our study. Furthermore, exact transportation distances were not known for all the materials.
The overall carbon balance of the urban parks cannot be estimated with sufficient accuracy. In our study, certain components and life cycle modules had to be excluded due to lack of relevant methods and databases. For example, play and sport equipment, or additives (fertilizers and biochar) might have an important impact in parks that have higher amounts of such components.
Finally, there are limitations concerning i-Tree Eco tool (i-Tree, 2023) to model the total CO2 sequestration in the case study parks. The growing medium is not included in the estimations which brings high uncertainties to the CO2 sequestration potential of the woody vegetation. In addition, the DBH was estimated based on the values of the landscape documents (which is measured at 1 m height). Furthermore, the crown light exposure and the mortality rate are difficult to predict for each species on a 50-year period. This makes the forecasted CO2 sequestration uncertain, as the tool applies the mortality rate aleatorily in the urban forest defined.
4.5 Further research needs
To provide a holistic understanding of CO2 flows of urban green infrastructure, several knowledge and methodological gaps need to be addressed. Further research is needed for estimating the GHG emissions during the nursery production of trees, shrubs, perennials, and annuals. There is currently little data about material and energy flows associated to the production of urban street furniture (benches, posts, fences, playgrounds, etc.), which leaves gaps in LCA. There is also a need for holistic, and ideally LCA-compatible methods for estimating CO2 uptake in soils of lawns, meadows, woody vegetation, and plantings. Furthermore, the environmental impacts of different maintenance scenarios for UGI are yet to be documented.
The choice of functional unit (FU) in LCA is also critical. Existing LCA studies of urban parks mostly used per square-metre or per hectare to present the results while studies focusing on woody vegetation use per tree or per tree cover area. For urban parks, a square-metre FU might flatten the complexity of the processes and interactions occurring within and between organisms, vegetation, and soils. Further investigation on suitable functional units to assess urban green spaces is required.
The dynamic nature of urban green infrastructure may also benefit from developing the methodology towards a dynamic LCA. This could be beneficial to integrate temporal and spatial changes happening during the growth of the vegetation together with other changes happening in the parks (Bixler et al., 2019). However, compatibility with a standardized building LCA methodology should be maintained, for ensuring the comparison of results.
5 Conclusion
This study highlights the need to develop assessment methods and design practices for supporting carbon-wise landscape design, construction, and maintenance. Although the studied urban parks have good CO2 uptake potential (6.1–15.4 kgCO2e/m2) from vegetation and soils, the CO2 emissions can be considerably higher (27.1–61.5 kgCO2e/m2). For mitigating the emissions, the use of peat-free growing media or recycled soils would be of primary importance. Other means include the reuse of materials, surfaces with longer service lives, and avoiding long and heavy transportation of fills. Planting of long-living tree species and the preservation of existing vegetation were found to be important for the uptake of CO2. However, planted vegetation would need to sequester CO2 for several decades, to compensate the emissions from the production of materials, construction, and maintenance activities. The CO2 uptake by vegetation and soils will also vary depending on climate conditions, growing conditions, and maintenance.
In addition, there is high demand for a standardized assessment method for urban parks. Emission data are critically missing for plants, growing media, and mulches. Compilation of scenarios for the accumulation and release of organic carbon and its reporting at the end-of-life of parks should be methodologically harmonized.
During the ongoing climate emergency, we would need to design all parts of our built environments to support the goals of the Paris Agreement. The role of urban parks may be significant, but reaching this potential still requires more research, more mature assessment methods and climate-smart design practices.
Data availability statement
The original contributions presented in the study are included in the article/Supplementary material, further inquiries can be directed to the corresponding author.
Author contributions
CM: Data curation, Formal analysis, Investigation, Resources, Software, Visualization, Writing – review & editing, Writing – original draft. MK: Conceptualization, Methodology, Supervision, Writing – review & editing. RH: Conceptualization, Funding acquisition, Supervision, Validation, Writing – review & editing.
Funding
The author(s) declare that financial support was received for the research, authorship, and/or publication of this article. This study has been supported by CO-CARBON project, funded by the Strategic Research Council (SRC) established within the Academy of Finland (grant nos. 358254 and 358255).
Acknowledgments
We thank City of Helsinki for providing us drawings and inventory data of the five urban parks.
Conflict of interest
The authors declare that the research was conducted in the absence of any commercial or financial relationships that could be construed as a potential conflict of interest.
Publisher’s note
All claims expressed in this article are solely those of the authors and do not necessarily represent those of their affiliated organizations, or those of the publisher, the editors and the reviewers. Any product that may be evaluated in this article, or claim that may be made by its manufacturer, is not guaranteed or endorsed by the publisher.
Supplementary material
The Supplementary material for this article can be found online at: https://www.frontiersin.org/articles/10.3389/frsc.2024.1452403/full#supplementary-material
References
Ariluoma, M., Kinnunen, A., Lampinen, J., Hautamäki, R., and Ottelin, J. (2024). Optimizing the co-benefits of biodiversity and carbon sinks in urban residential yards. Front. Sustain. Cities 6:1327614. doi: 10.3389/frsc.2024.1327614
Ariluoma, M., Leppänen, P.-K., Talvonen, O., Hautamäki, R., and Ryymin, A. (2023). A framework for a carbon-based urban vegetation typology – a thematic review. Environ. Dev. 47:100899. doi: 10.1016/j.envdev.2023.100899
Ariluoma, M., Ottelin, J., Hautamäki, R., Tuhkanen, E. M., and Mänttäri, M. (2021). Carbon sequestration and storage potential of urban green in residential yards: a case study from Helsinki. Urban For. Urban Green. 57:126939. doi: 10.1016/j.ufug.2020.126939
Bae, J., and Ryu, Y. (2015). Land use and land cover changes explain spatial and temporal variations of the soil organic carbon stocks in a constructed urban park. Landsc. Urban Plan. 136, 57–67. doi: 10.1016/j.landurbplan.2014.11.015
Bixler, T. S., Houle, J., Ballestero, T., and Mo, W. (2019). A dynamic life cycle assessment of green infrastructures. Sci. Total Environ. 692, 1146–1154. doi: 10.1016/j.scitotenv.2019.07.345
Canedoli, C., Ferrè, C., Khair, D. A., Padoa-Schioppa, E., and Comolli, R. (2020). Soil organic carbon stock in different urban land uses: high stock evidence in urban parks. Urban Ecosyst. 23, 159–171. doi: 10.1007/s11252-019-00901-6
CEN (2011a). EN 15643-2:2011 sustainability of construction works – Sustainability assessment of buildings – framework for the assessment of environmental performance. Brussels: CEN.
CEN (2011b). EN 15978 sustainability of construction works. Assessment of environmental performance of buildings. Calculated method. Brussels: CEN.
CEN (2014). EN 16449 wood and wood-based productsions. Calculation of the biogenic carbon content of wood and conversion to carbon dioxide. Brussels: CEN.
CEN (2019). EN 15804:A2 sustainability of construction works. Environmental product declarations. Core rules for the product category of construction products. Brussels: CEN.
CEN (2022). EN 16757 sustainability of construction works. Environmental production declarations. Product Category Rules for concrete and concrete elements. Brussels: CEN.
Dhakal, S. (2010). GHG emissions from urbanization and opportunities for urban carbon mitigation. Curr. Opin. Environ. Sustain. 2, 277–283. doi: 10.1016/j.cosust.2010.05.007
Dodd, N., Donatello, S., and Cordella, M. (2021). Level(s) – a common EU framework of core sustainability indicators for office and residential buildings. User manual 2: setting up a project to use the level(s) common framework. European Commission, Joint Research Centre.
Dorendorf, J., Eschenbach, A., Schmidt, K., and Jensen, K. (2015). Both tree and soil carbon need to be quantified for carbon assessments of cities. Urban For. Urban Green. 14, 447–455. doi: 10.1016/j.ufug.2015.04.005
European Commission. (2013). Green infrastructure (GI) – Enhancing Europe's natural capital. COM (2013) 249 Final. Brussels.
European Commission. (2023). Biodiversity strategy 2030. Available at: https://environment.ec.europa.eu/strategy/biodiversity-strategy-2030_en (accessed February 29, 2024)
Fore. (2023). Available at: https://portal.fore.fi/ (accessed June 26, 2023)
Haase, D., Larondelle, N., Andersson, E., Artmann, M., Sara Borgström, J. B., Gomez-Baggethun, E., et al. (2014). A quantitative review of urban ecosystem service assessments: concepts, models, and implementation. Ambio 43, 413–433. doi: 10.1007/s13280-014-0504-0
Hashemi, F., Mogensen, L., Smith, A. M., Larsen, S. U., and Knudsen, M. T. (2024). Greenhouse gas emissions from bio-based growing media: a life-cycle assessment. Sci. Total Environ. 907:167977. doi: 10.1016/j.scitotenv.2023.167977
Havu, M., Kulmala, L., Kolari, P., Vesala, T., Riikonen, A., and Järvi, L. (2022). Carbon sequestration potential of street tree plantings in Helsinki. Biogeosciences 19, 2121–2143. doi: 10.5194/bg-19-2121-2022
Havu, M., Kulmala, L., Lee, H. S., Saranko, O., Soininen, J., Ahongshangbam, J., et al. (2024). CO2 uptake of urban vegetation in a warming Nordic city. Urban For. Urban Green. 94:128261. doi: 10.1016/j.ufug.2024.128261
HSY. (2019). Selvitys pääkaupunkiseudun hiilinieluista ja -varastoista [Carbon sinks and storages of the capital region]. Helsinki.
Ignatieva, M., Haase, D., Dushkova, D., and Haase, A. (2020). Lawns in cities: from a globalised urban green space phenomenon to sustainable nature-based solutions. Land 9:73. doi: 10.3390/land9030073
Ingram, D. L. (2012). Life cycle assessment of a field-grown red maple tree to estimate its carbon footprint components. Int. J. Life Cycle Assess. 17, 453–462. doi: 10.1007/s11367-012-0398-7
IPCC (2022). Climate change 2022: Impacts, adaptation, and vulnerability. Contribution of working group II to the sixth assessment report of the intergovernmental panel on climate change. Cambridge, UK and New York, NY, USA: Cambridge University Press.
ISO (2018). ISO 14067. Greenhouse gases – carbon footprint of products – requirements and guidelines for quantification. Geneva: ISO.
i-Tree. (2023). I-tree eco v6. Available at: https://www.itreetools.org/tools/i-tree-eco (accessed June 26, 2023)
Kendall, A., and McPherson, E. G. (2012). A life cycle greenhouse gas inventory of a tree production system. Int. J. Life Cycle Assess. 17, 444–452. doi: 10.1007/s11367-011-0339-x
Kong, L., Shi, Z., and Chu, L. (2014). Carbon emission and sequestration of urban turfgrass systems in Hong Kong. Sci. Total Environ. 473-474, 132–138. doi: 10.1016/j.scitotenv.2013.12.012
Kuittinen, M., Hautamäki, R., Tuhkanen, E.-M., Riikonen, A., and Ariluoma, M. (2021). Environmental product declarations for plants and soils: how to quantify carbon uptake in landscape design and construction? Int. J. Life Cycle Assess. 26, 1100–1116. doi: 10.1007/s11367-021-01926-w
Lazzerini, G., Lucchetti, S., and Nicese, F. (2016). Green house gases (GHG) emissions from the ornamental plant nursery industry: a life cycle assessment (LCA) approach in a nursery district in Central Italy. J. Clean. Prod. 112, 4022–4030. doi: 10.1016/j.jclepro.2015.08.065
Lazzerini, G., Merante, P., Lucchetti, S., and Nicese, F. P. (2018). Assessing environmental sustainability of ornamental plant production: a nursery level approach in Pistoia District, Italy. Agroecol. Sustain. Food Syst. 42, 911–932. doi: 10.1080/21683565.2018.1466755
Lerman, S. B., and Contosta, A. R. (2019). Lawn mowing frequency and its effects on biogenic and anthropogenic carbon dioxide emissions. Landsc. Urban Plan. 182, 114–123. doi: 10.1016/j.landurbplan.2018.10.016
LETI. (2023). LETI unpicker. Retrofit vs rebuild: unpicking the carbon argument. Available at: https://www.leti.uk/retrofitunpicker (accessed August 18, 2023)
Liimatainen, H., Pöllänen, M., and Viri, R. (2018). CO2 reduction costs and benefits in transport: socio-technical scenarios. Eur. J. Futures Res. 6:22. doi: 10.1186/s40309-018-0151-y
Lind, E., Prade, T., Deak, J. S., Levinsson, A., and Sjörman, H. (2023). How green is an urban tree? The impact of species selection in reducing the carbon footprint of park trees in Swedish cities. Front. Sustain. Cities 5:126939. doi: 10.3389/frsc.2023.1182408
Lindén, L., Riikonen, A., Setälä, H., and Yli-Pelkonen, V. (2020). Quantifying carbon stocks in urban parks under cold climate conditions. Urban For. Urban Green. 49:126633. doi: 10.1016/j.ufug.2020.126633
Lu, C., Kotze, D. J., and Setälä, H. M. (2021). Evergreen trees stimulate carbon accumulation in urban soils via high root production and slow litter decomposition. Sci. Total Environ. 774:145129. doi: 10.1016/j.scitotenv.2021.145129
LUKE. (2023). MetsäInfo [Forest information]. Available at: https://metsainfo.luke.fi/fi/hiilivarastot (accessed August 15, 2023)
Margenot, A. J., Griffin, D. E., Alves, B. S., Rippner, D. A., Li, C., and Parikh, S. J. (2018). Substitution of peat moss with softwood biochar for soil-free marigold growth. Ind. Crop. Prod. 112, 160–169. doi: 10.1016/j.indcrop.2017.10.053
McPherson, E. G., and Kendall, A. (2014). A life cycle carbon dioxide inventory of the million trees Los Angeles program. Int. J. Life Cycle Assess. 19, 1653–1665. doi: 10.1007/s11367-014-0772-8
McPherson, E. G., Kendall, A., and Albers, S. (2015). Life cycle assessment of carbon dioxide for different arboricultural practices in Los Angeles, CA. Urban For. Urban Green. 14, 388–397. doi: 10.1016/j.ufug.2015.04.004
Ministry of the Environment (2019). Method for the whole life carbon assessment of buildings. Helsinki: Ministry of the Environment.
Nicese, F., Colangelo, G., Comolli, R., Azzini, L., Luchetti, S., and Marziliano, P. (2021). Estimating CO2 balance through the life cycle assessment prism: a case – study in an urban park. Urban For. Urban Green. 57:126869. doi: 10.1016/j.ufug.2020.126869
Nowak, D., and Crane, D. (2002). Carbon storage and sequestration by urban trees in the USA. Environ. Pollut. B. 116, 381–389. doi: 10.1016/S0269-7491(01)00214-7
Nowak, D. J., Crane, D. E., Stevens, J. C., Hoehn, R. E., Walton, J. T., and Bond, J. (2008). A ground-based method of assessing urban forest structure and ecosystem services. Arboricult. Urban For. 34, 347–358. doi: 10.48044/jauf.2008.048
Nowak, D. J., Greenfield, E. J., Hoehn, R. E., and Lapoint, E. (2013). Carbon storage and sequestration by trees in urban and community areas of the United States. Environ. Pollut. 178, 229–236. doi: 10.1016/j.envpol.2013.03.019
Nowak, D. J., Stevens, J. C., Sisinni, S. M., and Luley, C. J. (2002). Effects of urban tree management and species selection on atmospheric carbon dioxide. J. Arboric. 28, 113–122. doi: 10.48044/jauf.2002.017
One Click LCA. (2023). World's fastest building life cycle assessment software (One Click LCA Ltd). Available at: https://www.oneclicklca.com/ (accessed August 15, 2023)
Pouyat, R. V., Yesilonis, I. D., and Nowak, D. J. (2006). Carbon storage by urban soils in the United States. J. Environ. Qual. 35, 1566–1575. doi: 10.2134/jeq2005.0215
Richter, S., Haase, D., Thestorf, K., and Makki, M. (2020). Carbon pools of Berlin, Germany: organic carbon in soils and aboveground in trees. Urban For. Urban Green. 54:126777. doi: 10.1016/j.ufug.2020.126777
Röck, M., Mendes Saade, M., Balouktsi, M., Nygaard Rasmussen, F., Birgisdottir, H., Frischknecht, R., et al. (2020). Embodied GHG emissions of buildings – the hidden challenge for effective climate change mitigation. Appl. Energy 258:114107. doi: 10.1016/j.apenergy.2019.114107
Russo, A., Escobedo, F. J., Timilsina, N., Otto, S. A., Varela, S., and Zerbe, S. (2014). Assessing urban tree carbon storage and sequestration in Bolzano, Italy. Int. J. Biodivers. Sci. Ecosyst. Serv. Manag. 10, 54–70. doi: 10.1080/21513732.2013.873822
Setälä, H. M., Francini, G., Allen, J., Hui, N., Jumpponen, A., and Kotze, D. J. (2016). Vegetation type and age drive changes in soil properties, nitrogen, and carbon sequestration in urban parks under cold climate. Front. Ecol. Evol. 4:93. doi: 10.3389/fevo.2016.00093
Silvenius, F., Niemeläinen, O., and Kurppa, S. (2016). LCA case study on lawn establishment and maintenance with various peat and compost contents in substrates. Integr. Environ. Assess. Manag. 12, 459–464. doi: 10.1002/ieam.1789
Stephan, A., Crawford-, R. H., Bunster, V., Warren-Myers, G., and Moosavi, S. (2022). Towards a multiscale framework for modeling and improving the life cycle environmental performance of built stocks. J. Ind. Ecol. 26, 1195–1217. doi: 10.1111/jiec.13254
Strohbach, M. W., Arnold, E., and Haase, D. (2012). The carbon footprint of urban green space a life cycle approach. Landsc. Urban Plan. 104, 220–229. doi: 10.1016/j.landurbplan.2011.10.013
Strohbach, M. W., and Haase, D. (2012). Above-ground carbon storage by urban trees in Leipzig, Germany: analysis of patterns in a European city. Landsc. Urban Plan. 104, 95–104. doi: 10.1016/j.landurbplan.2011.10.001
SYKE. (2023). Emissions database for construction. Available at: co2data.fi (accessed June 26, 2023)
United Nations, Department of Economic and Social Affairs, Population Division (2019). World urbanization prospects: The 2018 revision. New York: United Nations.
Vasenev, V., and Kuzyakov, Y. (2018). Urban soils as hot spots of anthropogenic carbon accumulation: review of stocks, mechanisms and driving factors. Land Degrad. Dev. 29, 1607–1622. doi: 10.1002/ldr.2944
World Green Building Council. (2019). Bringing embodied carbon upfront. Coordinated action for the building and construction sector to tackle embodied carbon. Available at: https://worldgbc.org/article/bringing-embodied-carbon-upfront/
Xi, F., Davis, S., Ciais, P., Crawford-Brown, D., Guan, D., Pade, C., et al. (2016). Substantial global carbon uptake by cement carbonation. Nat. Geosci. 9, 880–883. doi: 10.1038/ngeo2840
Keywords: greenhouse gas, carbon, life cycle assessment, urban park, climate change mitigation, urban design
Citation: Moinel C, Kuittinen M and Hautamäki R (2024) Estimating CO2 flows in urban parks: knowns and unknowns. Front. Sustain. Cities. 6:1452403. doi: 10.3389/frsc.2024.1452403
Edited by:
Maria De Fatima Andrade, University of São Paulo, BrazilReviewed by:
Galina Churkina, Berlin University of Technology (TU Berlin), GermanyMaria Chiara Pastore, Polytechnic University of Milan, Italy
Copyright © 2024 Moinel, Kuittinen and Hautamäki. This is an open-access article distributed under the terms of the Creative Commons Attribution License (CC BY). The use, distribution or reproduction in other forums is permitted, provided the original author(s) and the copyright owner(s) are credited and that the original publication in this journal is cited, in accordance with accepted academic practice. No use, distribution or reproduction is permitted which does not comply with these terms.
*Correspondence: Caroline Moinel, Y2Fyb2xpbmUubW9pbmVsQGFhbHRvLmZp