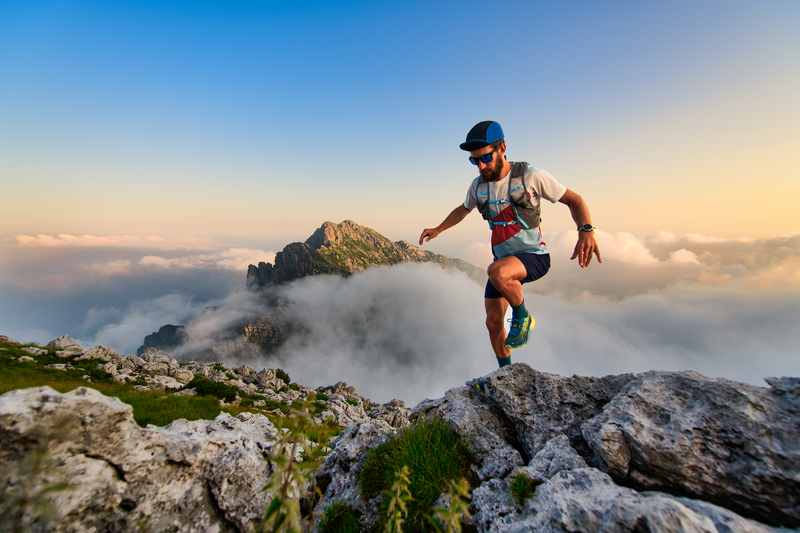
95% of researchers rate our articles as excellent or good
Learn more about the work of our research integrity team to safeguard the quality of each article we publish.
Find out more
ORIGINAL RESEARCH article
Front. Sustain. Cities , 12 June 2023
Sec. Urban Greening
Volume 5 - 2023 | https://doi.org/10.3389/frsc.2023.1182408
Introduction: Planting trees in urban areas can mitigate some of the emissions generated in cities by carbon sequestration (annual uptake of CO2 through the process of photosynthesis) and carbon storage (amount of carbon stored in the tree's biomass throughout its lifespan). The aim of this study was to calculate the carbon footprint from nursery production to final establishment of different tree species grown for planting in urban parks in a northern European context.
Material and methods: The analysis included a cradle-to-gate approach and investigated the amount of carbon the adult trees needed to sequester in order to compensate for initial carbon emissions and which temporal perspectives are of concern. Greenhouse gas emissions were estimated based on an inventory of consumption of fuels, energy, materials and other production inputs during cultivation, delivery, planting and establishment of three different tree species in three different locations in Sweden. The tree species considered in the analysis (Salix alba, Quercus rubra, Pinus sylvestris) were selected due to significant differences in their growth rates. Salix alba is a competitive strategist in resource-rich habitats, and is proficient at converting these resources into vigorous growth. Pinus sylvestris is a pronounced stress strategist with good ability to handle resource-limited habitats, and invests in traits accordingly, resulting in significantly slower development. Quercus rubra has its main distribution in cool and moderately resource-rich habitats, but has relatively high stress tolerance and can be considered intermediate between the other two species in terms of growth rate.
Results and discussion: The results showed that within 16 years of planting, all species in all three cities, except Pinus sylvestris planted in Umeå, compensated for initial carbon emissions, i.e. showed net absorption of CO2 after emissions from cultivation, delivery, planting and establishment of the trees had been deducted. There was a clear link between the time by which compensation of initial carbon emissions was achieved and growth rate of the different species, with the fast-growing Salix alba showing the best results. The single largest source of emissions among all activities carried out during cultivation, delivery and planting of all species, regardless of the city in which they were planted, was fuel consumption during tree planting.
Global warming as a result of emissions of greenhouse gases and associated climate change is widely known and scientifically documented. Climate change is predicted to involve higher frequencies of extreme weather events, natural disasters and drought, with major consequences for human living conditions, ecosystems and biodiversity (IPCC et al., 2018). Compared with mean global temperature in the period 1850–1900, there has been an increase of almost 1.0°C. Past emissions and the current increase in emissions globally are leading to an increase in mean global temperature of around 0.2°C per decade. However, Europe has experienced an average increase of 0.5°C per decade in the past 30 years, i.e., a more rapid rate of global warming than previously predicted (WMO, 2022).
Minimizing greenhouse gas emissions and associated temperature rises is a major concern and many countries have developed climate targets to reduce the carbon footprint of different sectors. Cities alone contribute around 70% of the global carbon footprint, by generating direct (within city boundary) and indirect (outside city boundary) greenhouse gas emissions, so mitigation efforts need to include urban areas (Lombardi et al., 2017; Moran et al., 2018). Within cities, the construction industry is a major contributor to urban carbon footprint and its energy-related carbon dioxide (CO2) emissions are currently rising. According to a press release from the COP27 conference, investments in building energy efficiency have increased but with an expected divergence too great to meet the decarbonization targets for 2050 (UNEP, 2022).
From an urban planning point of view, it has long been apparent that construction and management of low-carbon cities is a pressing concern (e.g., Selman, 2010). However, there is still only limited recognition of this within the landscape industry and there is insufficient guidance on how design principles, material selection, construction and maintenance can contribute to low/high carbon footprint (Kuittinen et al., 2021; Nikologianni et al., 2022). A tangible approach to the carbon dynamics of urban outdoor space requires creation of new policies and tools. On a tactical and operational level, this means clear Environmental Product Declarations (EPDs) (as described in Kuittinen et al., 2021), which need to be made available to producers and consumers. Urban trees as part of green infrastructure (GI) and associated positive benefits of carbon storage and sequestration have long been recognized (Dhakal, 2010). However, while planting trees is seen as mitigating aspects of climate change, systematic inclusion of the effects will require a life cycle assessment approach to GI (Kuittinen et al., 2021) and reliable data for species- and scenario-specific obtainable impacts and benefits, e.g., expressed as carbon footprint.
The quality and performance of urban trees derive partly from the individual characteristics and traits of the individual tree and partly from the management regime and the spatial context in which the tree is growing. Generally, carbon sequestration and storage are greater in larger trees with abundant biomass, such as those found in parks and recreation areas (Richter et al., 2020). In order to develop these environments into systematic and continuous carbon sinks, the carbon dynamics occurring in urban parks require strategic planning and management. Clear EPDs are needed for design and construction, while tangible tools for decision making and management are of equal importance for implementation (Hagemann et al., 2020).
Planting trees in urban areas can mitigate some of the emissions generated in these areas by carbon sequestration (annual uptake of CO2 through the process of photosynthesis) and carbon storage (amount of carbon stored in the tree's biomass throughout its lifespan). Research on carbon sinks and sequestration of CO2 in urban tree populations has been conducted since the 1990s, but has mainly focused on cities in the USA (Rahman and Ennos, 2016). For example, McPherson (1998) mapped CO2 storage and uptake in biomass of the urban tree population throughout Sacramento County, California, and estimated that the county's roughly 6 million trees absorb ~238,000 tons of CO2 annually, while Nowak and Crane (2002) estimated that trees in urban settings in the continental United States collectively store ~700 million tons of carbon. If all this stored carbon were to be released and converted to CO2, it would correspond to ~2.5 billion tons of CO2. The average amount of carbon stored per unit area of tree canopy in American cities is estimated to be 7.69 kg/m2 and the maximum amount is 14.1 kg C/m2 (Nowak et al., 2013). Similar values have been reported in other studies, e.g., 4.45 kg C/m2 in Barcelona, Spain (Chaparro and Terrasdas, 2009); 4.28 kg C/m2 in Hangzhou, China (Zhao et al., 2010); 6.82 kg C/m2 in Leipzig, Germany (Strohbach et al., 2012); and 10.6 kg C/m2 in Boston, USA (Raciti et al., 2012). According to Nowak et al. (2013), the differences in values between studies derive from differences in tree structure and composition, i.e., size and species distribution.
Crucial traits for carbon sequestration and storage include long life span, high wood density and high tolerance to various urban stress factors (Scharenbroch, 2012). In cities, preconditions for development of these traits can be found in, e.g., parks and woodlands, where rooting space and microclimate conditions are favorable for tree development, whereas high-density areas with impermeable surfaces pose greater challenges. Different growth rates may also affect carbon sequestration capacity, with different species sequestering different amounts depending on their life cycle stage (Kaul et al., 2010). For most fast-growing species (e.g., Populus spp. and Salix spp.), establishment is more rapid and management inputs are lower than for slower-growing species (e.g., Tsuga spp., Sciadopitys sp., and Quercus ilex). This means that biomass production rate is higher for fast-growing species than for slower-growing species. Stephenson and MacKay (2014) analyzed 403 different tropical and temperate tree species in forest stands worldwide and found that biomass growth increased with tree size for almost all species. This suggests that large trees not only act as major carbon stores, but also actively sequester large amounts of CO2 even when they have grown large and old. Climate and growing conditions can also affect CO2 sequestration by urban trees through affecting growth, which is slower in colder climates or in more stressful environments. Drought, high salt concentration, lack of nutrients, soil compaction and limited sunlight are some of the stressors that must be taken into account when evaluating the capacity of urban trees to sequester carbon (Wang et al., 2019).
Although urban trees can mitigate the effects of urban heat and help reduce carbon emissions, their early cultivation contributes to climate change. For example, emissions of greenhouse gases from the use of fossil-powered vehicles and machinery during seedling cultivation in the nursery and during tree establishment can affect the overall carbon offset. In fact, it can take many years before trees are climate-neutral (McPherson et al., 2015; Petri et al., 2016). Previous studies on carbon emissions and tree cultivation in nurseries, mainly in the USA, have compared different cultivation techniques and species in order to identify the cumulative carbon footprint of the nursery production stage (e.g., Kendall and McPherson, 2012; Ingram, 2013; Ingram and Hall, 2013, 2016; Ingram et al., 2019). Corresponding studies have not been carried out in a northern European context (i.e., Scandinavia), where climate conditions differ greatly from those covered by existing studies, affecting species availability and growth rate.
The aim of this study was therefore to calculate the carbon footprint from nursery production to final establishment of different tree species grown for planting in urban parks in a northern European context. The analysis includes a cradle-to-gate approach and investigated the amount of carbon the adult trees needed to sequester in order to compensate for initial carbon emissions and which temporal perspectives are of concern.
The approach used for analyzing the impacts of urban trees in this study was largely guided by the specifications and guidelines in PAS 2050, a document from the British Standards Institution (BSI) that specifies the procedure for greenhouse gas emissions for products and services (BSI, 2008). PAS 2050 is based on ISO 14044:2006 (the international standard for life cycle analysis), but is specifically aimed at, and offers a concrete quantitative description of assessing greenhouse gases (BSI, 2008).
Greenhouse gas emissions were estimated based on an inventory of consumption of fuels, energy, materials and other production inputs during cultivation, delivery, planting and establishment of three different tree species, Salix alba (white willow), Quercus rubra (red oak), and Pinus sylvestris (Scots pine). The functional unit (FU) was one tree planted in its final location. The trees were assumed to be produced for planting and establishment in park environments in the cities of Helsingborg, Stockholm and Umeå in Sweden (Figure 1). Production was assumed to take place at two nurseries located in southwestern Sweden. Nursery A1 produces seedlings as 1- or 2-year-old individuals. Nursery B2 obtains these seedlings and raises them until the point of sale, when all individual trees are 30–35 cm in girth and are balled and burlapped before sale. Girth was taken as distance around the trunk measured perpendicular to the axis of the trunk at breast height (1.4 m). A description of all activities performed during tree cultivation was obtained through direct contact with the cultivation managers at the nurseries. The analysis also included unsold seedlings and trees lost during production of the different species, which added to the carbon footprint of trees sold.
Figure 1. Geographical location of the nurseries and city destinations for the three tree species investigated.
Emissions from handling procedures and fuel consumption during transport from Nursery B to planting in Helsingborg, Stockholm and Umeå were estimated in consultation with a major transportation company in the city of Trelleborg with experience of shipping trees within Sweden. Fuel consumption was estimated based on the assumption that a fully loaded truck trailer delivered only Salix alba, Quercus rubra, or Pinus sylvestris for planting in open and easily accessible park environments in the three selected cities. Specific planting sites within the cities were not assumed. The search engine in Google Maps was used to determine the distance and direction between Nursery B and each city, i.e., without a specific street location, leading to final destinations in the city center. Establishment procedure included unloading of the trees from the truck trailer, wedding and preparation of the planting bed as well as soil improvement with green compost, and establishment care including watering. Information on procedures and data on fuel consumption and material use when planting trees were obtained through consultation with an experienced construction contractor of public environments.
The final inventory, including cultivation, delivery and planting, is presented in Appendix A1, while Figure 2 illustrates the process and consumption and supply of materials included in calculations of greenhouse gas emissions for the different tree species.
Figure 2. Process flow charts for production of the three tree species: (Top panel) Salix alba, (center panel) Quercus rubra and (lower panel) Pinus sylvestris. The system boundaries are marked with a dashed-dotted line.
The tree species considered in the analysis (Salix alba, Quercus rubra, and Pinus sylvestris) were selected due to significant differences in their growth rates (Reich, 2014). Salix alba is a competitive strategist and pioneer species in resource-rich habitats, and is proficient at converting these resources into vigorous growth in order to gain an advantage over other tree species (Newsholme, 2002; Grime and Pierce, 2012). Pinus sylvestris, an evergreen species, is a pronounced stress strategist with good ability to handle resource-limited habitats, such as wet, dry or hot growing environments (Farjon, 2021), and invests in traits accordingly, resulting in significantly slower and more defensive development (Grime and Pierce, 2012). Quercus rubra has its main distribution in cool and moderately resource-rich habitats, but has relatively high stress tolerance (Eyre, 1980) and can be considered intermediate between the other two species in terms of growth rate.
Carbon dioxide uptake by the trees during cultivation and when planted in the cities of Helsingborg, Stockholm and Umeå was estimated using the i-Tree Eco model (USDA Forest Service, 2020). Model inputs comprised estimates of tree height, trunk height, crown width and diameter at breast height (dbh), made using a method described by Strohbach et al. (2012). Dimensions were estimated for the three tree species at the time of planting (assumed girth 30–35 cm) and every 2 years for 16 years of tree growth in park environments in Helsingborg, Stockholm and Umeå. Dimensions were also estimated for the three species after 50 years of growth in park environments in the three cities. All park environments were assumed to have permeable site conditions with sufficient space above and below ground for biomass development (i.e., roots, trunk, branches, and leaves) and surrounding soil and microclimate conditions providing favorable growing conditions. The estimates obtained (see Appendix A2) were used in the i-Tree Eco model to calculate the amount of carbon sequestered in tree trunk, branches and roots, based on allometric equations for each tree species. For Pinus sylvestris, carbon sequestered in the needles was also included. The amount of carbon sequestered was then multiplied by a constant factor of 3.67 (which is the molecular weight of CO2) to convert to the amount of CO2 taken up from the atmosphere (McPherson et al., 2016). The estimations in our study were concluded when the tree's total CO2 uptake exceeded total combined CO2 emissions during cultivation, delivery, planting, and establishment.
Emissions of greenhouse gases estimated for each part of the production process and for the overall process are shown in Figure 3. Total emissions and accumulated carbon were estimated for the three tree species when planted in a park environment in Helsingborg, Stockholm, and Umeå.
Figure 3. Greenhouse gas emissions during seedling cultivation of the three tree species investigated (Salix alba, Quercus rubra and Pinus sylvestris). FU, functional unit.
Total emissions during seedling cultivation in Nursery A, including an allowance for 20% mortality, were similar for Salix alba and Quercus rubra, while emissions during cultivation of Pinus sylvestris seedlings were noticeably lower (Figure 3). This was mainly due to cultivation in 1-L pots, compared with 2-L pots for the other two species. The greatest contributor to within-nursery emissions for all three species was peat, which accounted for just over 50% of emissions. Aggregated emissions linked to growth substrates comprised around 70% of emissions for all three species.
Emissions from fuel and electricity consumption, use of pesticides, seed for green manure crops, fertilizer and nitrous oxide emissions as a result of nitrogen fertilization during cultivation of Salix alba, Quercus rubra and Pinus sylvestris trees at Nursery B were estimated to be 7.62, 11.18, and 12.19 kg CO2-equivalents (CO2e) per FU, respectively (Appendix A1). These estimates were based on the assumption that Salix alba was grown for 7.5 years at Nursery B, Quercus rubra for 11 years and Pinus sylvestris for 12 years before reaching sellable size (Figure 4). Additional emissions that could not directly be linked to a specific species were allocated based on total annual greenhouse gas emissions from production of 150,000 trees grown at Nursery B (all tree species and sizes included). Emissions per average tree in cultivation at Nursery B were calculated to be ~0.7 kg CO2e per year (Figure 4). Furthermore, an allowance of 0.01 kg CO2e per tree and year was made for 2% mortality of plants during cultivation and an allowance of 0.3 kg CO2e per tree and year for the average 30% of tree seedlings not sold.
Figure 4. Greenhouse gas emissions from Nursery B for the three tree species investigated. FU, functional unit.
Total emissions of greenhouse gases during the entire cultivation process from seed/acorn/cutting to ready-to-sell tree (30–35 cm girth) were similar for Quercus rubra and Pinus sylvestris, but ~25% lower for Salix alba (Figure 5). On assuming 0% mortality rate during cultivation and that 100% of trees were sold, the emissions were ~28% lower.
Figure 5. Accumulated greenhouse gas emissions during the whole production process for the three tree species investigated. FU, functional unit.
The truck assumed to deliver the trees emitted an estimated total of 64.8 kg CO2e, 417.6 kg CO2e, and 956.5 kg CO2e on a one-way trip from Nursery B to Helsingborg, Stockholm and Umeå, respectively. Assuming a fully loaded trailer with balled and burlapped Salix alba or Quercus rubra with 30–35 cm girth (22 trees), emissions per tree were 3.24 kg CO2e/FU to Helsingborg, 20.9 kg CO2e/FU to Stockholm and 47.8 kg CO2e/FU to Umeå (Figure 6). Corresponding emissions per tree when delivering balled and burlapped Pinus sylvestris with 30–35 cm girth (28 trees) in a fully loaded trailer were 2.55 kg CO2e/FU to Helsingborg, 16.4 kg CO2e/FU to Stockholm and 37.6 kg CO2e/FU to Umeå. A higher number of FU per delivery, as for Pinus sylvestris compared with the other two tree species, gave lower transport emissions per tree and the effect of this increased with longer transport distance (Figure 6).
Figure 6. Emissions during delivery of the respective functional units (FU, Salix alba, Quercus rubra and Pinus sylvestris trees) to Helsingborg, Stockholm and Umeå. Note that transport distance becomes increasingly important when delivering a smaller number of FU.
Plantation of the trees led to emissions of over 100 kg CO2e/FU for the three tree species (Figure 7). The single largest source of emissions during planting (58%) was the fossil-powered equipment used, which emitted ~55 kg CO2e/FU. The second largest source of emissions during planting (42%) was use of green compost in the plant bed, production of which emitted methane and nitrous oxide emissions from composting of waste biomass. The minor difference in emissions for planting the different species arose from the number of trees estimated to be available for planting at the same time (22 FU for Salix alba and Quercus rubra, 28 FU for Pinus sylvestris).
Figure 7. Accumulated emissions of greenhouse gases during establishment of the three tree species investigated (Salix alba, Quercus rubra, and Pinus sylvestris).
Irrigation during plantation of Salix alba (a total of 20 occasions) generated emissions of 8.8 kg CO2e/FU (Figure 7). Corresponding emissions for Quercus rubra (a total of 27 occasions) and Pinus sylvestris (a total of 41 occasions) were 11.9 and 18 kg CO2e/FU, respectively. Of these emissions, the pickup truck used for irrigation accounted for 31%, while the petrol-fuelled pump used to pump the water accounted for 69%.
Total emissions of greenhouse gases during cultivation, delivery and planting of Salix alba in Helsingborg, Stockholm and Umeå were calculated to be 120, 138, and 165 kg CO2e/FU, respectively (Figure 8). Corresponding emissions for Quercus rubra were 128, 146, and 173 kg CO2e/FU, respectively, and for Pinus sylvestris 134, 148, and 169 kg CO2e/FU, respectively. Regardless of city and tree species, planting (including establishment) accounted for the largest share of total emissions (Figure 8). Emissions from cultivation and planting of each tree species were similar regardless of the city in which they were planted. For planting in Helsingborg and Stockholm, this meant that Pinus sylvestris had the greatest total emissions of the three species, mainly due to greater emissions from planting (where the longer period of establishment irrigation was a decisive factor) (Figure 8). When planting in Umeå, on the other hand, Quercus rubra caused the greatest emissions. Compared with Pinus sylvestris, Quercus rubra is a larger tree in terms of total volume when delivered, which means that fewer trees (FU) fit into a trailer for delivery. With the longer transport distance to Umeå, the higher transport-related emissions for Quercus rubra meant that its total emissions exceeded those of Pinus sylvestris. Salix alba had the lowest total emissions in all three cities. However, the differences between the species decreased as the transport distance increased (Figure 8). In Helsingborg, the difference between planting Salix alba and Pinus sylvestris was 14 kg CO2e. In Stockholm this difference decreased to 10 kg CO2e and in Umeå it decreased further to 4 kg CO2e. This was again due to the difference in volume between the species at the size when delivered, which meant that more Pinus sylvestris could fit in the trailer, resulting in lower emissions per FU during delivery (Figure 8).
Figure 8. Total accumulated greenhouse gas emissions for the three tree species investigated (Salix alba, Quercus rubra and Pinus sylvestris) when established in the city of (left) Helsingborg, (center) Stockholm, and (right) Umeå.
Salix alba reached ready-to-sell size of 30–35 cm in girth after 8 years in Nursery B, by which time it was estimated to have sequestered 52.8 kg CO2. Quercus rubra required 13 years of cultivation and sequestered 56.8 kg CO2 during that period, while Pinus sylvestris required 14 years of cultivation and sequestered 27.9 kg CO2.
After 10 years, a Salix alba tree planted in an open park environment in Helsingborg, Stockholm and Umeå had sequestered 394, 320, and 234 kg CO2, respectively. Net absorption of CO2 by that time, i.e., with emissions from cultivation, delivery, planting and establishment deducted, was 274, 182, and 69 kg CO2, respectively. By 16 years after planting, a Salix alba tree had sequestered 832, 633, and 422 kg CO2 in Helsingborg, Stockholm and Umeå, respectively, and net absorption then was 712, 495, and 257 kg CO2, respectively (Figure 9). In Helsingborg, Salix alba had compensated the initial carbon emissions after 4 years of planting, while in Stockholm and Umeå this occurred in year 5 and 8, respectively (Figure 9).
Figure 9. The number of years from establishment until the three tree species could compensate the initial carbon emissions in each of the three cities investigated.
A Quercus rubra tree planted in an open park environment in Helsingborg, Stockholm and Umeå had sequestered 347, 276, and 178 kg CO2, respectively, after 10 years and 693, 518, and 287 kg CO2, respectively, after 16 years (Figure 9). Net absorption of CO2 in these cities was 218, 130, and 5 kg CO2, respectively, after 10 years and 565, 372, and 114 kg CO2, respectively, after 16 years (Figure 9).
A Pinus sylvestris tree planted in an open park environment in Helsingborg, Stockholm and Umeå had sequestered 113, 89, and 77 kg CO2, respectively, by 10 years after planting, and 205, 150, and 123 kg CO2, respectively, by 16 years after planting (Figure 9). Net absorption of CO2 in these cities was −20, −58, and −91 kg CO2, respectively, after 10 years and 71, 2, and −46 kg CO2, respectively, after 16 years. In Helsingborg and Stockholm, Pinus sylvestris had compensated the initial carbon emissions after 12 and 16 years. In Umeå, this did not occur within the time frame of 16 years after planting, and the Pinus sylvestris needed to sequester an additional 46 kg CO2 in order to compensate the initial carbon emissions.
After 50 years, a Salix alba, Quercus rubra, and Pinus sylvestris had sequestered 8,191, 6,072, and 1,312 kg CO2, respectively, when established in Helsingborg (Figure 10). The corresponding values for Stockholm were 5,748, 4,047, and 983 kg CO2, respectively, and for Umeå 3,048, 1,545, and 711 kg CO2, respectively. Net uptake of CO2 by Salix alba trees after 50 years, i.e., with emissions from cultivation, delivery, planting and establishment deducted, was 8,071, 5,610, and 2,883 kg CO2 in Helsingborg, Stockholm and Umeå, respectively. The corresponding net uptake values for Quercus rubra were 5,944, 3,901, and 1,372 kg CO2, respectively, and for Pinus sylvestris 1,179, 836, and 542 kg CO2, respectively (Figure 10).
Figure 10. Net carbon budget 50 years after establishment (green color) for the three tree species studied (Salix alba, Quercus rubra, and Pinus sylvestris) when growing in (left) Helsingborg, (center) Stockholm, and (right) Umeå and accumulated emissions during production and establishment (blue color).
Apart from Pinus sylvestris planted in Umeå, the tree species considered in this study compensated the initial carbon emissions in the three cities within 16 years. There was a clear connection between the time of compensation and the growth rate of the different species. The rapid biomass growth of Salix alba led to a rapidly increasing rate of sequestration of CO2 in the first 16 years after planting, while the slow biomass growth of Pinus sylvestris led to a much more slowly increasing sequestration rate. Quercus rubra showed a sequestration rate that was much faster than for Pinus sylvestris, but not as fast as for Salix alba.
As expected, there was also a clear connection between CO2 sequestration by all three tree species and estimated biomass growth in the three different cities. In general, the results indicated that it is more difficult to contribute to negative emissions by using urban trees and that it may be important to select fast-growing and large-growing tree species for park environments in colder climates. Overall, the results showed that rapid growth rate of an urban tree is very positive from the view of achieving negative emissions, at least within a time horizon of 50 years, which is in line with findings in previous studies (e.g., Nowak et al., 2002; Jo et al., 2019). However, our conclusions are based on trees compensating initial carbon emissions in a Swedish climate whereas trees growing in milder temperate climates often become carbon neutral in shorter time span (Loehle, 1998). This also relates to nursery productions where trees produced in milder temperate climates reach a final size much faster compared to trees produced in a cooler Swedish climate, thereby producing less carbon baggage. Still, these initial benefits will provide a negative trade off due to emissions from tree transportation between nurseries in continental Europe to Swedish cities.
The single largest source of emissions for establishing an urban tree, independent of location, was the machinery used for excavation and planting bed preparation. If these operations could be shortened with unchanged engine load, to, e.g., 80 min, emissions would be reduced by −11 kg CO2e. This hotspot would be an interesting starting point for decarbonization of the production chain, e.g., by using electric excavators when low-carbon electricity is available to fuel the machine, which is already the case in Sweden and some other countries. An emissions cut of 50% for these operations would mean, e.g., that a Salix alba planted in Stockholm would compensate for its initial carbon emissions 1 year earlier. A study by Ingram (2012) found that smaller tree sizes that can be handled and planted by hand would result in lower greenhouse gas emissions than larger tree seedlings requiring machines for handling and planting.
The footprint deriving from nursery cultivation accounted for only a small proportion of total emissions for all three tree species, and these emissions were of a similar order of magnitude to those reported in similar life cycle analyses by, e.g., Kendall and McPherson (2012) and Ingram and Hall (2016). In those studies, however, the FU was a smaller tree size grown for a shorter time. For example, Ingram and Hall (2016) based their calculations on a tree grown in the field at a nursery in the USA to a size of 5 cm dbh, which is roughly half the trunk diameter of the trees analyzed in this study, and reported total emissions of 17.1 kg CO2e during cultivation for 6 years. It can of course be debated whether it is justifiable to choose such large trees in our study, but it echoes a current planting trend in Swedish cities. However, it involves an extensive use of machinery compared to smaller trees that can be handled by hand. A common explanation for new plantings of large trees is robustness—the ability of trees to handle wear and tear in often intensively utilized environments. In a study by Hilbert et al. (2019) it appears that tree mortality for trees in public environments is greatest in the first 5 years after planting where smaller and younger plants signify the highest mortality (e.g., Nowak et al., 1990; Koeser et al., 2014; Roman et al., 2015; Widney et al., 2016). This also highlights the link between successful establishment and successful development with subsequent benefit to their function as carbon sinks. Species-specific establishment also becomes central to this perspective, where slower-growing species (e.g., Pinus sylvestris) need to receive extended establishment care with special attention to irrigation compared to the fast-growing and more quickly established tree species (e.g., Salix alba) where the chances are greater for a successful establishment through its rapid establishment. Furthermore, species-specific establishment and management are context dependent on varying climate conditions, as growth and the rate of establishment will differ greatly in a cool vs. a hot summer climate (e.g., Loehle, 1998).
We focused on the establishment of trees in parks, but if paved street environments were included, the use of more fast-growing and competitive strategy species such as Salix alba (Grime and Pierce, 2012) would not be as useful due to their limited capacity to handle periods of drought (in comparison to, e.g., Pinus sylvestris) (Shaban et al., 2009; Farjon, 2021). Hence the ability of trees as carbon sinks will differ greatly depending on both species selection (in terms of slow and fast growing strategies) and planting location (in terms of water stress).
The results would also most likely differ if a street environment were assumed for our study since carbon emissions from materials such as asphalt and concrete would probably result in greater carbon expenditure during bed preparation and establishment. In contrast to studies by Richter et al. (2020) and Kuittinen et al. (2021), the present study did not include the impact from manufactured soils and mulches already present in parks or added during the final phase of tree establishment, although this may be of relevance for tree planting in park environments. Our study focused on the cultivation, transportation and establishment of the trees leaving additional research toward long-term evaluations of emissions associated with maintenance, decommissioning and disposal. The estimated climate footprint values presented for the trees in this study are therefore only valid provided that interventions taking place after the trees are established, e.g., maintenance and safety pruning, do not result in additional greenhouse gas emissions. Safety pruning at 5-year intervals over 50 years (using fossil-powered equipment) can result in estimated emissions of 28 kg CO2e (McPherson et al., 2015), while felling and disposal of trees grown in good site conditions for 60 years can involve emissions of 214 kg CO2e (Ingram and Hall, 2016). Assuming that equivalent emissions occur during pruning and removal and disposal of, e.g., a Quercus rubra tree, it would take another 7 years in Helsingborg, 8 years in Stockholm and over 16 years in Umeå before the tree compensates its initial carbon emissions. In the latter case, a Quercus rubra tree in Umeå would not have sequestered sufficient amounts of CO2e to compensate initial carbon emissions within 16 years. It is also clear that during the production phase, nurseries can play an important role in minimizing CO2 emissions. For example, peat was a major contributor to the carbon footprint of the nurseries considered in the present study, indicating a need for future research to find alternative growing media. Today, extensive development is underway to find alternatives to sphagnum peat moss such as compost from sweet corn tassels or mixing in biochar (e.g., Vaughn et al., 2011; Margenot et al., 2018). As transport is a large source of emissions in connection with delivering growing substrate to nurseries, it is crucial to use local products or components in the production of peat free composts and thereby reduce the carbon footprint in the production of trees (Fascella, 2015).
Trees in urban parks are a vital asset for the ecosystem functioning of cities and for urban dwellers. Multi-layered dense tree plantings have the greatest carbon sink capacity of all urban vegetation types (Strohbach et al., 2012; Li et al., 2018; Wilkes et al., 2018; Jo et al., 2019), and help support additional ecosystem services of, e.g., biodiversity and for wildlife habitats (Nielsen et al., 2013). However, the potential carbon footprint of newly planted park trees and the time at which compensation is achieved may differ between species. It is therefore essential to develop a species-appropriate management strategy (i.e., planning, design, construction, and maintenance) (Jansson et al., 2019). For instance, Salix alba showed very rapid growth and establishment, which means it is a suitable species for quick compensation of initial carbon emissions, while Quercus rubra and Pinus sylvestris can provide long-term robustness due to their longer life span compared with Salix alba. This information can help, e.g., municipal tree caretakers to plan, design and allocate different maintenance strategies diversely and more site-specifically in relation to location, function and the selection of species with resilience to longer periods of heat and drought (Sjöman et al., 2018). For instance, slower-growing trees with appropriate stress-coping strategies for inner-city environments, such as Pinus sylvestris in this study, may compensate for the initial carbon footprint with a long-term approach of stress tolerance and longevity. Recognizing the unavoidable carbon footprint of, e.g., 5 years of irrigation during establishment (which may be the case for slow-growing species) can help limit tree mortality and secure more stable carbon sinks in the urban landscape (Roman et al., 2014). An urban tree with high vitality will most likely also result in lower greenhouse gas emissions by requiring fewer maintenance efforts (Petri et al., 2016). Careful evaluation of the planting location and choosing an appropriate species to match these conditions, followed by appropriate establishment management, is thus crucial to obtaining long-term sustainable tree plantings and thereby effective carbon storage.
In this study we analyzed three common tree species with differing growth characteristics. In future research, assessments should be performed for a greater range of urban trees and extend the scope beyond a cradle to gate approach. Future studies should also consider the biophysical qualities of soils (Richter et al., 2020), wood density (Scharenbroch, 2012), maintenance operations occurring after establishment and options for final tree disposal. Taking all these factors into account would allow for more realistic assessments of the carbon footprint of urban trees and the time it takes for compensation of initial carbon emissions.
This study examined the climate benefits of planting trees of three species (Salix alba, Quercus rubra, and Pinus sylvestris) in park environments in three Swedish cities (Helsingborg, Stockholm, and Umeå). The results showed that within 16 years of planting, all species in all three cities, except Pinus sylvestris planted in Umeå, had compensated initial carbon emissions, i.e., showed net absorption of CO2 after emissions from cultivation, delivery, planting and establishment of the trees had been deducted. There was a clear link between the time by which compensation of initial carbon emissions was achieved and growth rate of the different species, with the fast-growing Salix alba showing the best results. The single largest source of emissions among all activities carried out during cultivation, delivery and planting of all species, regardless of the city in which they were planted, was fuel consumption during tree planting. The largest contributor to within-nursery emissions for all three species was use of peat, which accounted for just over 50% of emissions.
The original contributions presented in the study are included in the article/Supplementary material, further inquiries can be directed to the corresponding author.
All authors listed have made a substantial, direct, and intellectual contribution to the work and approved it for publication.
This study was funded by Swedish Research Council Formas [2021-02398].
The authors declare that the research was conducted in the absence of any commercial or financial relationships that could be construed as a potential conflict of interest.
All claims expressed in this article are solely those of the authors and do not necessarily represent those of their affiliated organizations, or those of the publisher, the editors and the reviewers. Any product that may be evaluated in this article, or claim that may be made by its manufacturer, is not guaranteed or endorsed by the publisher.
The Supplementary Material for this article can be found online at: https://www.frontiersin.org/articles/10.3389/frsc.2023.1182408/full#supplementary-material
1. ^Ranviks Garden; ~120 km north of Malmö. http://www.ranvik.se/.
2. ^Tönnersjö Nursery; ~140 km north of Malmö, Sweden. https://tonnersjo.se/.
BSI. (2008). PAS 2050. Available online at: http://www.carbonconstruct.com/pdf/pas_2050.pdf (accessed December 16, 2022).
Chaparro, L., and Terrasdas, J. (2009). Ecological Services of Urban Forest in Barcelona. Bellaterra: Centre de Recerca Ecologica i Aplicacions Forestals, Universitat Autonoma de Barcelona.
Dhakal, S. (2010). GHG emissions from urbanization and opportunities for urban carbon mitigation. Curr. Opin. Environ. Sustain. 2, 277–283. doi: 10.1016/j.cosust.2010.05.007
Fascella, G. (2015). Growing Substrates Alternative to Peat for Ornamental Plants. Soilless Culture-Use of Substrates for the Production of Quality Horticultural Crops. p. 47–67.
Grime, J. P., and Pierce, S. (2012). The Evolutionary Strategies That Shape Ecosystems. John Wiley and Sons.
Hagemann, F. A., Randrup, T. B., and Ode Sang, Å. (2020). Challenges to implementing the urban ecosystem service concept in green infrastructure planning: a view from practitioners in Swedish municipalities. Socio-Ecol. Pract. Res. 2, 283–296. doi: 10.1007/s42532-020-00054-3
Hilbert, D. R., Roman, L. A., Koeser, A. K., Vogt, J., and van Doorn, N. S. (2019). Urban tree mortality: A literature review. Arboricult. Urban Forest. 45, 167–200. Available online at: https://www.fs.usda.gov/nrs/pubs/jrnl/2019/nrs_2019_hilbert_001.pdf
Ingram, D. L. (2012). Life cycle assessment of a field-grown red maple tree to estimate its carbon footprint components. Int. J. Life Cycle Assess. 17, 453–462. doi: 10.1007/s11367-012-0398-7
Ingram, D. L. (2013). Life cycle assessment to study the carbon footprint of system components for colorado blue spruce field production and use. J. Am. Soc. Horticul. Sci. 138, 3–11. doi: 10.21273/JASHS.138.1.3
Ingram, D. L., and Hall, C. R. (2013). Carbon footprint and related production costs of system components of a field-grown Cercis canadensis L. “Forest Pansy” Using Life Cycle Assessment 1. I J. Environ. Hort. 31, 169. doi: 10.24266/0738-2898.31.3.169
Ingram, D. L., and Hall, C. R. (2016). Comparison of carbon footprint and variable costs of selected nursery production systems for a 5-cm-caliper red maple. HortScience, 51, 383–387. doi: 10.21273/HORTSCI.51.4.383
Ingram, D. L., Hall, C. R., and Knight, J. (2019). Understanding carbon footprint in production and use of landscape plants. HortTechnology, 29, 6–10. doi: 10.21273/HORTTECH04220-18
IPCC. (2018). “Summary for policymakers,” in Global Warming of 1.5°C. An IPCC Special Report on the Impacts of Global Warming of 1.5°C Above Pre-Industrial Levels and Related Global Greenhouse Gas Emission Pathways, in the Context of Strengthening the Global Response to the Threat of Climate Change, Sustainable Development, and Efforts to Eradicate Poverty, eds V. Masson-Delmotte, P. Zhai, H. -O. Pörtner, D. Roberts, J. Skea, P. R. Shukla, A. Pirani, W. Moufouma-Okia, C. Péan, R. Pidcock, S. Connors, J. B. R. Matthews, Y. Chen, X. Zhou, M. I. Gomis, E. Lonnoy, T. Maycock, M. Tignor, and T. Waterfield (Cambridge; New York, NY: Cambridge University Press), 3–24. doi: 10.1017/9781009157940.001
Jansson, M., Vogel, N., Fors, H., and Randrup, T. B. (2019). The governance of landscape management: new approaches to urban open space development. Landscape Res. 44, 952–965. doi: 10.1080/01426397.2018.1536199
Jo, H. K., Kim, J. Y., and Park, H. M. (2019). Carbon reduction and planning strategies for urban parks in Seoul. Urban Forest. Urban Green. 41, 48–54. doi: 10.1016/j.ufug.2019.03.009
Kaul, M., Mohren, G. M. J., and Dadhwal, V. K. (2010). Carbon storage and sequestration potential of selected tree species in India. Mitig. Adapt. Strat. Global Change 15, 489–510. doi: 10.1007/s11027-010-9230-5
Kendall, A., and McPherson, G. E. (2012). A life cycle greenhouse gas inventory of a tree production system. Int. J. Life Cycle Assess. 17, 444–452. doi: 10.1007/s11367-011-0339-x
Koeser, A. K., Gilman, E. F., Paz, M., and Harchick, C. (2014). Factors influencing urban tree planting program growth and survival in Florida, United States. Urban Forest. Urban Green. 13, 655–661. doi: 10.1016/j.ufug.2014.06.005
Kuittinen, M., Hautamäki, R., Tuhkanen, E.-M., Riikonen, A., and Ariluoma, M. (2021). Environmental Product Declarations for plants and soils: how to quantify carbon uptake in landscape design and construction? Int. J. Life Cycle Assess. 26, 1100–1116. doi: 10.1007/s11367-021-01926-w
Li, F. X., Shi, H., Zhao, J. S., Feng, X. G., and Li, M. (2018). Carbon sequestration and spatial differentiation characteristics of urban forest in China. Appl. Ecol. Environ. Res. 16, 1563–1580. doi: 10.15666/aeer/1602_15631580
Loehle, C. (1998). Height growth rate tradeoffs determine northern and southern range limits for trees. J. Biogeogr. 25, 735–742. doi: 10.1046/j.1365-2699.1998.2540735.x
Lombardi, M., Laiola, E., Tricase, C., and Rana, R. (2017). Assessing the urban carbon footprint: an overview. Environ. Impact Assess. Rev. 66, 43–52. doi: 10.1016/j.eiar.2017.06.005
Margenot, A. J., Griffin, D. E., Alves, B. S., Rippner, D. A., Li, C., and Parikh, S. J. (2018). Substitution of peat moss with softwood biochar for soil-free marigold growth. Indust. Crops Prod. 112, 160–169. doi: 10.1016/j.indcrop.2017.10.053
McPherson, E. G. (1998). Atmospheric carbon dioxide reduction by Sacramento's urban forest. J. Arboricult. 24, 215–223 doi: 10.48044/jauf.1998.026
McPherson, E. G., Kendall, A., and Albers, S. (2015). Life cycle assessment of carbon dioxide for different arboricultural practices in Los Angeles, CA. Urban Forest. Urban Green. 14, 388–397. doi: 10.1016/j.ufug.2015.04.004
McPherson, E. G., van Doorn, N. S., and Peper, P. J. (2016). Urban tree database and allometric equations. Gen. Tech. Rep. PSW-GTR-253. Albany, CA: US Department of Agriculture, Forest Service. Pacif. Southwest Res. Stat. 86, 253. doi: 10.2737/PSW-GTR-253
Moran, D., Kanemoto, K., Jiborn, M., Wood, R., Többen, J., and Seto, K. C. (2018). Carbon footprints of 13,000 cities. Environ. Res. Lett. 13, 064041. doi: 10.1088/1748-9326/aac72a
Nielsen, A. B., van den Bosch, M., Maruthaveeran, S., and Konijnendijk van den Bosch, C. (2013). Species richness in urban parks and its drivers: a review of empirical evidence. Urban Ecosyst. 17, 305–327. doi: 10.1007/s11252-013-0316-1
Nikologianni, A., Plowman, T., and Brown, B. (2022). A review of embodied carbon in landscape architecture. Pract. Policy J. Carb. Res. 8, 22. doi: 10.3390/c8020022
Nowak, D. J., and Crane, D. E. (2002). Carbon storage and sequestration by urban trees in the USA. Environ. Pollut. 116, 381–389. doi: 10.1016/S0269-7491(01)00214-7
Nowak, D. J., Greenfield, E. J., Hoehn, R. E., and Lapoint, E. (2013). Carbon storage and sequestration by trees in urban and community areas of the United States. Environ. Pollut. 178, 229–236. doi: 10.1016/j.envpol.2013.03.019
Nowak, D. J., McBride, J. R., and Beatty, R. A. (1990). Newly planted street tree growth and mortality. J. Arboricult. 16, 124–129.
Nowak, D. J., Stevens, J. C., Sisinni, S. M., and Luley, C. J. (2002). Effects of urban tree management and species selection on atmospheric carbon dioxide. J. Arboricult. 28, 113–122. doi: 10.48044/jauf.2002.017
Petri, A. C., Koeser, A. K., Lovell, S. T., and Ingram, D. (2016). How green are trees? Using life cycle assessment methods to assess net environmental benefits. J. Environ. Hort. 34, 101–110. doi: 10.24266/0738-2898-34.4.101
Raciti, S. M., Hutyra, L. R., Rao, P., and Finzi, A. C. (2012). Inconsistent definitions of “urban” result in different conclusions about the size of urban carbon and nitrogen stocks. Ecol. Appl. 22, 1015–1035. doi: 10.1890/11-1250.1
Rahman, M. A., and Ennos, A. R. (2016). What We Know and Don't Know About the Carbon Storage and Sequestration of Urban Trees. Technical Report.
Reich, P. B. (2014). The world-wide ‘fast–slow'plant economics spectrum: a traits manifesto. J. Ecol. 102, 275–301. doi: 10.1111/1365-2745.12211
Richter, S., Haase, D., Thestorf, K., and Makki, M. (2020). Carbon pools of Berlin, Germany: organic carbon in soils and aboveground in trees. Urban Forest. Urban Green. 54, 126777. doi: 10.1016/j.ufug.2020.126777
Roman, L. A., Battles, J. J., and McBride, J. R. (2014). The balance of planting and mortality in a street tree population. Urban Ecosyst. 17, 387–404. doi: 10.1007/s11252-013-0320-5
Roman, L. A., Walker, L. A., Martineau, C. M., Muffly, D. J., MacQueen, S. A., and Harris, W. (2015). Stewardship matters: Case studies in establishment success of urban trees. Urban Forest. Urban Green. 14, 1174–1182. doi: 10.1016/j.ufug.2015.11.001
Scharenbroch, B. C. (2012). “Urban trees for carbon sequestration,” in Carbon Sequestration in Urban Ecosystems, eds. R. Lal and B. Augustin (New York, NY: Springer).
Selman, P. (2010). Learning to Love the Landscapes of Carbon-Neutrality. Landscape Res. 35, 157–171. doi: 10.1080/01426390903560414
Shaban, M., Khajeddin, S. J., Karimzade, H. R., and Panahpoor, E. (2009). The investigation of drought resistance in wood species for Isfahan greenery development. J. Res. Agricul. Sci. 5, 57–67.
Sjöman, H., Hirons, A. D., and Bassuk, N. L. (2018). Improving confidence in tree species selection for challenging urban sites: a role for leaf turgor loss. Urban Ecosyst. 21, 1171–1188. doi: 10.1007/s11252-018-0791-5
Stephenson, A. L., and MacKay, D. J. (2014). Life Cycle Impacts of Biomass Electricity in 2020. UK Department of Energy and Climate Change.
Strohbach, M. W., Arnold, E., and Haase, D. (2012). The carbon footprint of urban green space-A life cycle approach. Landscape Urban Plann. 104, 220–229. doi: 10.1016/j.landurbplan.2011.10.013
UNEP (2022). CO2 Emissions from Buildings and Construction Hit New High, Leaving Sector off Track to Decarbonize by 2050. UN Environment Programme. Available online at: https://www.unep.org/news-and-stories/press-release/co2-emissions-buildings-and-construction-hit-new-high-leaving-sector (accessed 9, November).
USDA Forest Service (2020). i-Tree. Available online at: https://www.itreetools.org/about (accessed December 21, 2022).
Vaughn, S. F., Deppe, N. A., Palmquist, D. E., and Berhow, M. A. (2011). Extracted sweet corn tassels as a renewable alternative to peat in greenhouse substrates. Indust. Crops Prod. 33, 514–517. doi: 10.1016/j.indcrop.2010.10.034
Wang, X. M., Wang, X. K., Su, Y. B., and Zhang, H. X. (2019). Land pavement depresses photosynthesis in urban trees especially under drought stress. Sci. Total Environ. 653, 120–130. doi: 10.1016/j.scitotenv.2018.10.281
Widney, S., Fischer, B. C., and Vogt, J. (2016). Tree mortality undercuts ability of tree-planting programs to provide benefits: results of a three-city study. Forests. 7, 65. doi: 10.3390/f7030065
Wilkes, P., Disney, M., Vicari, M. B., Calders, K., and Burt, A. (2018). Estimating urban above ground biomass with multi-scale LiDAR. Carbon Bal. Manag. 13, 1–20. doi: 10.1186/s13021-018-0098-0
WMO (2022). Temperatures in Europe Increase More Than Twice Global Average. World Meteorological Organization (2022). Available online at: https://public.wmo.int/en/media/press-release/temperatures-europe-increase-more-twice-global-average (acessed November 7, 2022).
Keywords: climate change, tree selection, carbon sequestration, carbon storage, urban forestry
Citation: Lind E, Prade T, Sjöman Deak J, Levinsson A and Sjöman H (2023) How green is an urban tree? The impact of species selection in reducing the carbon footprint of park trees in Swedish cities. Front. Sustain. Cities 5:1182408. doi: 10.3389/frsc.2023.1182408
Received: 08 March 2023; Accepted: 17 May 2023;
Published: 12 June 2023.
Edited by:
Peng Fu, Harrisburg University of Science and Technology, United StatesReviewed by:
Tenley M. Conway, University of Toronto Mississauga, CanadaCopyright © 2023 Lind, Prade, Sjöman Deak, Levinsson and Sjöman. This is an open-access article distributed under the terms of the Creative Commons Attribution License (CC BY). The use, distribution or reproduction in other forums is permitted, provided the original author(s) and the copyright owner(s) are credited and that the original publication in this journal is cited, in accordance with accepted academic practice. No use, distribution or reproduction is permitted which does not comply with these terms.
*Correspondence: Henrik Sjöman, aGVucmlrLnNqb21hbkBzbHUuc2U=
†These authors have contributed equally to this work
Disclaimer: All claims expressed in this article are solely those of the authors and do not necessarily represent those of their affiliated organizations, or those of the publisher, the editors and the reviewers. Any product that may be evaluated in this article or claim that may be made by its manufacturer is not guaranteed or endorsed by the publisher.
Research integrity at Frontiers
Learn more about the work of our research integrity team to safeguard the quality of each article we publish.