- 1School of Environmental Sciences, Jawaharlal Nehru University, New Delhi, India
- 2Department of Environmental Studies, Zakir Husain Delhi College, University of Delhi, New Delhi, India
- 3Department of Environmental Sciences, Hindu College, University of Delhi, New Delhi, India
- 4Department of Chemistry, Hindu College, University of Delhi, New Delhi, India
The variability of organic carbon (OC), elemental carbon (EC), and secondary organic aerosol (SOA) and their relationship with meteorological parameters have been studied during foggy and non-foggy days in the peak winter months (December–January) from 2015 to 2016 in Delhi, India. Different sectoral locations were chosen based on predominant industrial, traffic, and residential activities with a background location. The average level of OC, EC, and SOA was found to be 7.47 ± 7.74, 0.69 ± 0.7, and 10.46 ± 10.76 μg/m3, respectively, during the foggy period and 6.1 ± 6.8, 0.9 ± 1.1, and 9.1 ± 10.6 μg/m3, respectively, during the non-foggy period in Delhi. A relatively higher SOA level was observed at industrial and traffic intersection sites, which indicates the proximity of the dominant source of OC that play a significant role in SOA formation. It was also found that SOA production is associated with the OC/EC ratio and may vary from site to site. Correlation analysis has confirmed that OC is having a significant strong positive correlation with EC and SOA, while EC is showing a significant moderate positive correlation with SOA. Ambient temperature (AT) shows a significant negative moderate correlation with OC levels and SOA and formation. Due to hydrophilicity (hydrophobicity) of OC (EC), its average concentration was found high (less) due to its high (less) scavenging during foggy days in comparison to non-foggy days. The study further suggests the significant impact of source variability on SOA formation due to the different nature of sector-wise sites during foggy days in Delhi.
Introduction
Airborne particulate matter (PM) is composed of a variety of inorganic and organic species including carbonaceous aerosol. Carbonaceous aerosol is composed of organic carbon (OC) and elemental carbon (EC); due to radiative properties, EC is also known as black carbon (BC) (Dutkiewicz et al., 2009). The carbonaceous aerosol is produced from the incomplete combustion of organic materials such as coal, petroleum, and biomass (Seinfeld and Pandis, 2006). The OC is directly released into the atmosphere in the particle phase (primary organic aerosol) or the gas phase. OC species in the gas phase frequently undergo oxidation reactions that lead to the formation of secondary organic aerosol (SOA) (Odum et al., 1997). Another important component of carbonaceous aerosol is EC, which is released into the atmosphere from incomplete combustion of carbonaceous fuels (Sonwani et al., 2021). Over the last decade, a number of studies of carbonaceous aerosols have been carried out especially related to their characterization and source apportionment in India and the world (Bisht et al., 2015a; Begum, 2017; Saxena et al., 2020b). Several studies in China identified the composition and distribution of organic aerosol (OA) and found that the primary organic aerosol (POA) and secondary organic aerosol (SOA) contributed 52 and 48% of total OA, respectively, in eastern China (Han et al., 2016). Huang et al. (2014) conducted a study over different regions of China and mentioned that the SOA contributes 44–71% of OA. Residential emissions, biomass burning, industrial and traffic emissions, and coal combustion are also considered major sources of OA in Beijing and South China (Cao et al., 2006; Song et al., 2006). Indian studies also identified several sources of carbonaceous components such as industrial and traffic emission sources as important sources in Delhi (Saxena et al., 2020b; Goel et al., 2021; Sonwani et al., 2021, 2022); biofuel/biomass burning and fossil fuel combustion are the important sources of the Mumbai (Aggarwal et al., 2013), while other studies mentioned that fossil fuels combustion, biomass burning, and vehicular emission sources are the important sources in Indo-Gangetic plain (one of the most popular and highly polluted regions in the world) in India (Rajput et al., 2016; Bikkina et al., 2017; Saxena et al., 2021; Ravindra et al., 2022).
Organic aerosol (OA), a major fraction of fine particulate matter, consists of primary organic aerosol (POA) and secondary organic aerosol (SOA) (Ho et al., 2006). POA is directly emitted to the atmosphere in condensed particle form (e.g., Schauer et al., 2001) after which it may partially evaporate depending on ambient conditions (Subramanian et al., 2007). Atmospheric oxidation reactions of the volatile organic compounds (VOCs) with O3, OH, and radicals result in the formation of semi-volatile VOCs (SVOCs) that are further oxidized to form SOA in the atmosphere. It was identified that most of the SOA are formed from isoprene and monoterpenes emitted from biogenic sources (Kroll et al., 2006). However, some studies also mentioned that sesquiterpenes, whose emission rate is only 10–20% of that of monoterpenes, could also be important contributors to SOA formation (Helmig et al., 2007; Sonwani et al., 2016). Oxidation of higher alkanes and aromatic compounds is an important process involved in the formation of most of the anthropogenic SOA (Kleeman et al., 2007; Chen et al., 2010).
It was identified that the water-soluble organic carbon (WSOC) in ambient aerosol is responsible for the formation of SOA (Seinfeld and Pankow, 2003; Weber et al., 2007). However, a part of water-insoluble OC (WIOC) can also be SOA (Sonwani and Saxena, 2021). The importance of SOA has been recognized and modeled extensively during photochemical events (Schell et al., 2001; Griffin et al., 2002; Pun et al., 2003; Johnson et al., 2006).
Several studies in the urban area reported SOA with higher concentration, especially in the winter months (Cao et al., 2003; Saxena et al., 2020b; Sonwani et al., 2021). A high concentration of SOA in the atmosphere is responsible for regional air quality, climate change, and human health (Pöschl, 2005; Chen et al., 2010; Saxena et al., 2020b). Thus, the monitoring and control of the SOA are important to the establishment of the air quality standard for fine PM due to its role in haze formation, visibility reduction, and negative health effects. SOA also affects climate forcing directly by altering the scattering properties of the atmosphere and indirectly by changing cloud properties.
The significance of SOA has been documented and modeled widely (Griffin et al., 2002; Johnson et al., 2006; Sonwani and Kulshrestha, 2019; Sonwani et al., 2021). Generally, OC/EC ratios are in the range of 2–3 for urban sites (Turpin and Huntzicker, 1995), and OC/EC ratios exceeding 2 represents SOA formation (Chow et al., 1993); it also represents the contribution of several sources (Sonwani et al., 2021). A ratio between 2.5 and 10.5 represents residential coal burning, 7.7 symbolizes biomass burning, 0.8 indicates the predominance of heavy-duty diesel vehicles, and 2.2 indicates gasoline exhaust as a major emission source for carbonaceous aerosol (Cheng et al., 2006; Saarikoski et al., 2008). Another study with a higher OC/EC ratio such as 5.8 in Lahore (urban location), Pakistan (Husain et al., 2007) reported the formation of SOA. However, such high OC/EC ratios cannot be explained only in terms of increased contribution from SOA, rather it can also be credited to the majority of biomass burning sources (Ram and Sarin, 2010). Nevertheless, there are very few studies that have reported SOA formation during the foggy/winter season (Chen et al., 2010; Kaul et al., 2011; Saxena et al., 2020b). Due to meteorological conditions such as low temperature, high humidity, and stagnant air favors the formation of condensable water-soluble SOA during foggy days (Kaul et al., 2011), it was also reported that the fog enhances the SOA formation, and aqueous phase chemistry in fog drops is responsible for the SOA formation (Saxena et al., 2020b). Studies in Indo-Gangetic Plain (IGP) also reported the enhanced formation of SOA due to aqueous phase chemistry. Thus, fogs also act as the active at cleansing the atmosphere of carbonaceous aerosols (Saxena et al., 2020a). The removal of the carbonaceous aerosol through sedimentation is efficient with smaller fog droplets as compared to the large droplets (Kaul et al., 2011). The OC is more effectively removed from the atmosphere through the wet scavenging process due to its high hydrophilicity as compared to EC (Sonwani et al., 2021).
The present study examined the OC and EC in ambient aerosol samples collected at different locations during the foggy and non-foggy periods in the year 2015–2016 in Delhi, India. The main goal of the present study is to identify the variability in SOA levels according to changing anthropogenic sources of carbonaceous aerosol during foggy days. Thus, the objectives include the source identification by the OC/EC ratio, estimation of SOA using the EC tracer technique, variability of carbonaceous fractions during foggy and non-foggy days, and relationship of SOA production according to the source's predominance of carbonaceous fractions.
Methodology
Study area and sampling locations
Delhi, India (28.38°N, 77.10°E), constitutes an area of 1,483 km2 with one of the highest polluted regions in the world. Delhi is also facing a problem of rapid industrial growth and urbanization resulting in the immigration of people from different cities in India and daily up and down schedules of the population from the National Capital Region (NCR) of Delhi resulting in high traffic density in the city. The city has several small, medium/large scale industries, several coal-based thermal power plants with combined capacity of ~1,560 MW and three gas-based power plants with a combined capacity of ~1,938 MW (CEA 2016). According to the Delhi transport department, the total number of registered vehicles up to June 30, 2014 was 80, 52,508 (Government of Delhi, 2014). Among all the megacities of the world, Delhi is also identified as the world's most polluted city in terms of fine particulate matter (World Health Organization, 2021). In several research studies, it was identified that the PM2.5 concentration in the Delhi region exceeds the National Ambient Air Quality Standard (annual NAAQS) of 40 μg/m3 (it is four times higher than the WHO's standard limit) (Dey et al., 2012; Pal et al., 2018; Sonwani and Kulshrestha, 2018; Singh et al., 2021; Sonwani et al., 2022). Thus, Delhi is characterized by several man-made emission sources such as fossil fuels, vehicular emissions, and agricultural residue burning (Saxena et al., 2020a; Sonwani et al., 2021). Delhi experiences three main seasons, viz., summer, monsoon, and winter. It experiences winter from November to February with several foggy days. The ambient temperature ranges between 3 and 22°C, humidity ranges from 53 to 98%, and wind speed is 2–6 m/s in winter months. UB is the Jawaharlal Nehru University campus (latitude 28.54°N and longitude 77.17°E) located at the ridge of the Aravalli hills in South Delhi. It is considered the urban background location in Delhi as it receives citywide emissions most of the time in a year (Sonwani et al., 2021). This site comprises academic buildings, staff quarters, a mini shopping area, and hostels. TI site is a traffic intersection area (latitude 28.63°N and longitude 77.09°E) and very near to commercial areas such as Mukherjee Nagar and Kamla Nagar. This site has high congestion at all times because of a large number of eating points, proximity to the metro station, and close to the University of Delhi North Campus area. The ID site is an industrial site (latitude 28.71°N and longitude 77.17°E) located in North-West Delhi and in close proximity to another industrial area Jahangirpuri. It is also close to the outer ring road and contains a high traffic volume (Saxena et al., 2020b). This site is also having a metro station nearby, a bus depot, some slum areas, and one of the biggest vegetable markets in Delhi, Azadpur mandi, which makes this site more congested. The location of these three sites is shown in Figure 1.
Sample collection, analysis, and quality control
Fine aerosol samples (PM2.5) were collected on quartz micro-fiber filters using a low volume sampler (flow rate = 30 LPM) on foggy and non-foggy days during the winter season in early morning hours, i.e., 04:00–07:00 h on the terrace of each site's building in the year 2015–2016 (Saxena et al., 2020b). The meteorological parameters, viz., ambient temperature (AT), relative humidity (RH), and wind speed (WS) data were procured from www.wounderground.com in the Delhi region. The foggy and non-foggy days were identified with the help of a weather forecast from the Indian Meteorological Department (IMD) (http://city.imd.gov.in) website. Before starting sampling, the filters were baked at 550°C for 8 h and then stored in aluminum foils that were also pre-baked. These samples were kept in airtight boxes in the refrigerator at about 4°C to prevent the evaporation of volatile components.
A punch of ~0.5 cm2 area of QFFs was analyzed for OC and EC fractions by using an OC-EC analyzer (DRI Model, 2001; Cao et al., 2013). The IMPROVE_A protocol was used for the analysis of carbon in PM2.5 collected on a quartz fiber filter. The sample is heated to four temperature plateaus (140, 280, 480, and 580°C) in pure helium and three temperature plateaus (580, 740, and 840°C) in 98% helium and 2% oxygen. During the analysis, OC is vaporized from a sample in the presence of inert helium and an oxidizer is used to combust EC. Pyrolyzed carbon (OP) is formed when reflectance reached its initial intensity and when O2 is added to the combustion atmosphere at 580°C. The relative standard deviation of duplicate samples was in the range of 10% for organic species, OC and EC. The calibration of this instrument can be done by different known samples of CH4. A blank is also analyzed to obtain the correct value of the samples by subtracting blank values from the samples. The magnitude of the blank was 1.77 μg/m3 for OC and 0.04 μg/m3 for EC. The analyzer was also calibrated each month by using a preheated blank quartz fiber filter and standard sucrose solution. Several studies mention a similar process of quality control for atmospheric OC and EC determination (Srinivas and Sarin, 2014; Sonwani and Kulshrestha, 2019; Sonwani et al., 2021).
Determination of SOA
There is no direct analysis method available to quantify SOA in total organic mass. So, for this, an indirect method for SOC estimation is used to take EC as a tracer for POC into consideration for the evaluation of SOA (Turpin and Huntzicker, 1995). EC is mainly emitted from combustion sources along with POC (Cao et al., 2003). The equations used to estimate SOC are as follows:
where [OC/EC]min is the minimum value of OC/EC ratios observed for the samples. SOA estimation was done by the EC tracer method. In this case, SOC is multiplied by 1.4 to give SOA. This method was adopted by Turpin and Huntzicker (1995) and Kaul et al. (2011).
Results and discussion
Level distribution of carbonaceous components
Foggy days during the winter season (November–January) repeatedly affect the pollutants load in northern India (Saxena et al., 2020b). Descriptive statistics of mass concentration (average ± SD) of OC, EC, SOA, and OC/EC are shown in Table 1 for the foggy and non-foggy periods over selected locations in Delhi, India. Figures 2A,B show day-to-day variations of the different species during both seasons. The average level of OC, EC, SOA, and OC/EC was found to be 7.47 ± 7.74, 0.69 ± 0.7, 10.46 ± 10.76, and 18.62 ± 20.66 μg/m3, respectively, during the foggy period and 6.1 ± 6.8, 0.9 ± 1.1, 9.1 ± 10.6, and 14.84 ± 18.99 μg/m3, respectively, during the non-foggy period in Delhi. The relatively lower carbonaceous concentration may be due to the presence of fog in the winter season (Saxena et al., 2020b). Among all three selected sites, the highest level of carbonaceous components was found at the ID site during foggy days, where OC and EC were found about two times and SOA was 1.7 times higher than Delhi's average. The UB site was identified with lower average OC and SOA levels and was found about two and 2.4 times lower than Delhi's average. Similarly, in the case of the non-foggy period, carbonaceous components were found to be highest at the ID site and OC, EC, and SOA were observed to be about two times higher than Delhi's average. Moreover, the UB site was again identified with the lowest OC and SOA concentration and observed with about two times lower concentration on non-foggy days. The higher level of the OC in comparison to EC indicated the more abundance of OC with fog droplets due to OC's hydrophilicity and EC's hydrophobicity. Thus, the average OC and EC concentrations were found in the order of ID > UB > TI; whereas for SOA concentration, the observed order was ID > TI > UB during foggy and non-foggy days. The higher levels of carbonaceous components at the ID site during both periods indicated the high combustion and emission activities, resulting in high levels of ambient carbonaceous components (Sonwani et al., 2021). Whereas, the low concentration of OC and SOA at UB indicated less number of immediate sources as it is a university campus area with limited combustion-related sources, especially during the sampling period. The observed SOA levels were found lesser than in the earlier reported studies in Delhi (Saxena et al., 2020b) and Kanpur (Kaul et al., 2011). In the case of EC, it was found to be lowest at the TI site with about four times and six times lower than Delhi's average during foggy and non-foggy periods, respectively. The low level of EC at the traffic site may be representative of freshly emitted EC from vehicular sources and less abundance of aged EC particles during both periods. It was also observed that the average levels of OC/EC ratios were found significantly higher at the TI site (30.56 ± 26.83 and 30.76 ± 26.04, respectively) as compared to UB (8.2 ± 5.46 and 6.32 ± 4.01) and ID site (14.25 ± 15.37 and 8.07 ± 5.93) during foggy and non-foggy periods, respectively. This significantly higher OC/EC ratio at the TI site was due to the emission released from the several restaurants located very near to the TI sites and involved with the different cooking activities, namely, meat roasting, cafeteria frying, fish roasting, and snack-street boiling. Similar observations were also reported by different authors characterizing different cooking-related emissions (Schauer et al., 2002; See and Balasubramanian, 2008; Li et al., 2015).
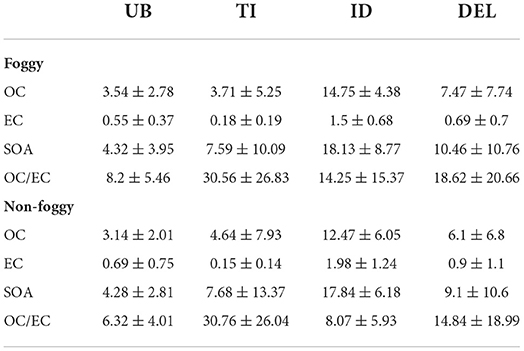
Table 1. Carbonaceous aerosol fractions during foggy and non-foggy days at respective sites over Delhi.
Table 2 shows a comparison of particulate bound average concentrations of OC, EC, and SOA/SOC/WSOC with their predominant source type at different selected locations in Delhi, India and in the different regions of the world. Several studies on carbonaceous aerosol have been performed across the world but very limited studies were carried out for the determination of carbonaceous species during foggy days in comparison to non-foggy days. Some of the recent studies that reported their OC, EC, and SOA/SOC/WSOC concentrations during foggy days have been listed along with the other studies that reported concentrations of such carbonaceous species in PM2.5. Saxena et al. (2020b) reported OC, EC, and SOA levels as 35.48, 10.34, 17.84 and 54.74, 11.86, and 40.12 μg/m3 at residential and industrial sites, respectively, during foggy days in Delhi. Such levels of the carbonaceous species are relatively higher as compared to our study and that may be due to the selection of the nearness of sampling location to the source and another reason is due to the selection of the time period and that is the time of Diwali (firework) festival and a season of crop residue burning in nearby states in Delhi. Similarly, Ali et al. (2019) identified the OC, EC, and SOA levels in Delhi in PM1.0 mass concentrations and found OC (24.7 ± 9.4), EC (11.7 ± 4.7), and SOC (11.3–16.3) μg/m3 at airport and traffic sites, i.e., also higher than our studies values due to the fine size of particulate matter and predominance of traffic and airport-related emissions. Kaul et al. (2011) identified the OC, EC, and SOA concentrations in foggy and non-foggy periods in the Kanpur site with a predominance of industrial, traffic, and domestic fuel combustion sources. The levels of carbonaceous species were found significantly higher than in the present study during foggy and non-foggy periods. These significantly higher levels of OC, EC, and SOA might be due to the presence of a variety of emission sources near the sampling location in Kanpur. Jain et al. (2020) identified Delhi's annual average levels of OC and EC as 15.73 ± 12.72 and 7.31 ± 6.17 μg m−3, respectively, for PM2.5. Jain et al. (2021) also noticed PM2.5 bound OC and EC levels as 15.09 ± 8.72 and 6.54 ± 3.27 μg m−3, respectively, in Varanasi. While in Kolkata, OC and EC levels were observed as 17.91 ± 9.72 and 9.43 ± 4.11 μg m−3, respectively. Similarly, other studies also reported the carbonaceous species in PM2.5 and PM1.0 samples collected at different locations in India and the world as listed in Table 2 (Pio et al., 2011; Zhang et al., 2011, 2014; Du et al., 2014; Bisht et al., 2015a; Gao et al., 2016; Sharma et al., 2016).
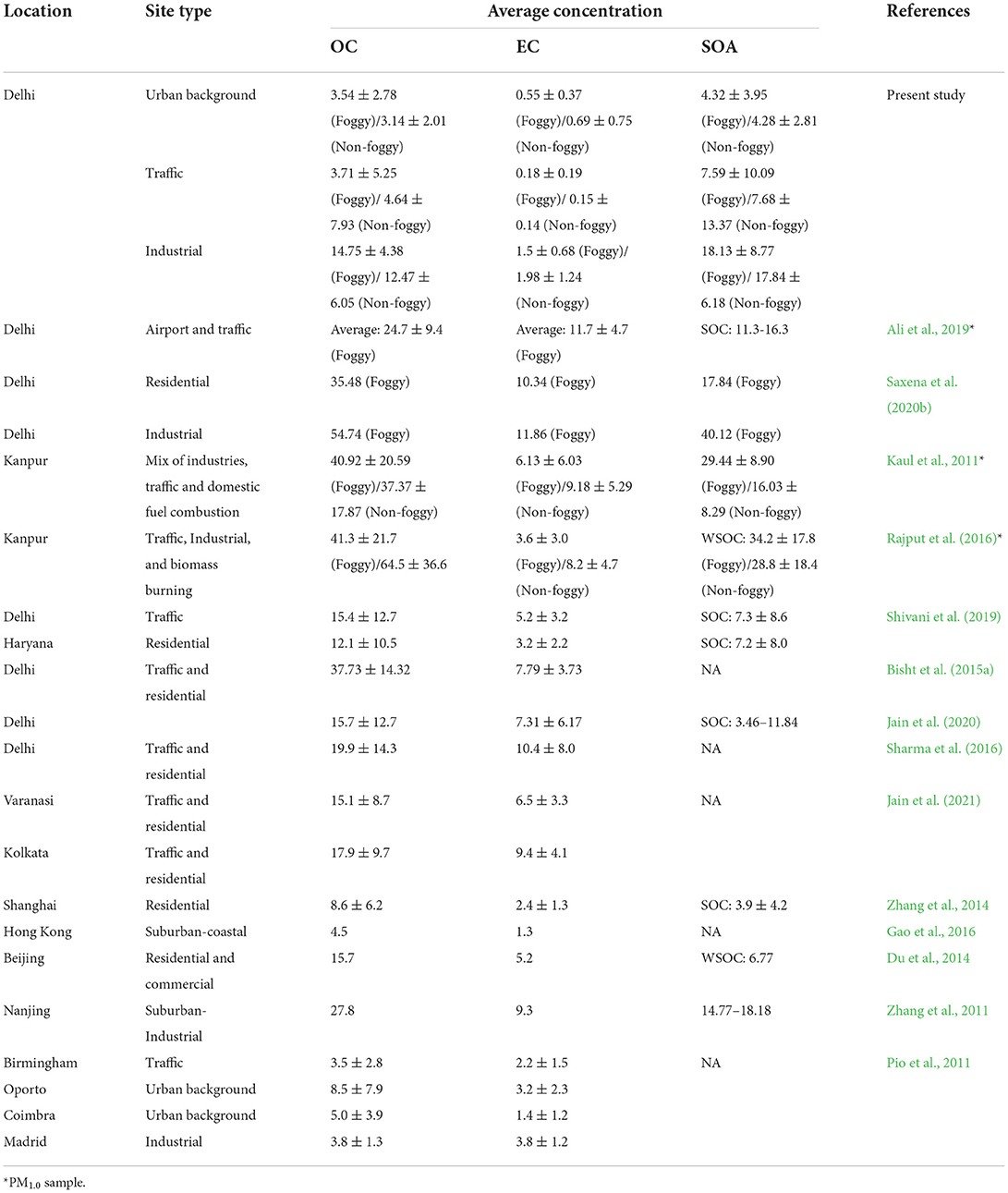
Table 2. Statistics for carbonaceous aerosol mass concentrations in PM2.5 measured in the major cities of India and the world (μg/m3).
Time series of carbonaceous components
Figures 2A,B represent the time series of carbonaceous components at selected sites over Delhi during foggy days and non-foggy days, respectively. The time series of OC and SOA have been presented on the primary y-axis, while EC has been presented on the secondary y-axis in both the figures. Some of the samples were discarded due to contamination, and electricity failure/operational failure of the sampler at the respective sites. Fog is an effective scavenger of OC and is observed with varying scavenging efficiencies that range between 5 and 90% for EC or black carbon (BC) (Gundel et al., 1994; Collett et al., 2008). Carbonaceous species (OC, EC, and SOA) were found to be higher in the peak winter period during both foggy and non-foggy days. Due to a predominant industrial emission, significantly high OC and SOA levels were observed in the case of ID sites during both periods. SOA concentrations on both foggy and non-foggy days were found to be high; however, the SOA concentrations on foggy days are comparatively higher, on average, than on non-foggy days. A direct comparison of SOA concentrations is, however, perhaps not especially revealing, since fog actively deposits scavenged organic matter as enhanced dispersion on non-foggy days is estimated to decrease SOA levels under such atmospheric conditions. A higher OC and SOA formation was also noticed in the case of the TI site, especially during the peak winter period, which may be due to the traffic congestion due to visibility degradation in the peak winter period (Saxena et al., 2020a; Sonwani et al., 2022). Similarly, EC levels were also found to be higher on foggy and non-foggy days both at the ID site. In case of non-foggy days, OC at ID and TI sites was found higher in comparison to OC at the UB site. Moreover, SOA formation was significantly higher at industrial and traffic sites in comparison to the UB site. The higher SOA formation at ID and TI sites indicated toward the immediate source of OC that is further responsible for the anthropogenic origin of SOC formation and play a significant role in anthropogenic pollutants for SOA formation in the urban area (Lee et al., 2010). In comparison to OC and SOA, EC remains less affected during foggy days, as freshly emitted EC particles (hydrophobic) remain unaffected by fog, hence less scavenged by fog droplets, whereas aged EC particles (hydrophilic) are easily scavenged from the atmosphere during foggy days. In the present study, EC particles effectively scavenged through the fog at ID followed by UB in comparison to the TI site. It indicates the abundance of EC particles at the ID site and the predominance of aged particles at the UB site (as the site is located at the urban background location and behaves like a sink of the city). The low EC concentration during foggy and non-foggy days at the TI site represents the predominance of freshly released EC particles (hydrophobic) through vehicular emission.
Correlation analysis
Correlation analysis is performed between OC, EC, and SOA concentrations (μg/m3) and meteorological parameters, i.e., AT (ambient temperature), RH (relative humidity), and WS (wind speed) in Delhi (Supplementary Table 1). Pearson's correlation coefficient (r) assumes an inherent linear relationship in the data (Jain et al., 2017) and the correlation ranges from strong (1.00–0.50) to moderate (0.49–0.30) to weak (0.29–0.00) (Xie et al., 2015). As per correlation analysis, OC is having a significant strong positive correlation with EC and SOA, while EC is showing a significant moderate positive correlation with SOA. Moreover, OC shows a significant negative moderate correlation with AT. OC and EC's strong positive correlation indicates that both are emitted from a common source. The selected sites, TI and ID, have immediate combustion sources like industries and vehicles which are strong sources of OC and EC; however, UB is an urban background site and acts as a sink of emissions coming from combustible sources that again confirms the presence of OC and EC. Moreover, OC was also found to be strongly positively correlated with SOA, and EC was found to be significantly moderately positively correlated with SOA. Primary OC is the main precursor of SOA and actively participates in the formation of SOA. OC species in the gas phase often undergo oxidation reactions that lead to the formation of secondary organic aerosol (SOA) (Odum et al., 1997). The pre-existing seed aerosols are responsible for the formation of SOA under the influence of homogeneous or heterogeneous nucleation (Sonwani and Kulshrestha, 2019). Moreover, since OC and EC are significantly strongly positively correlated, therefore, EC also has some minor role in the formation of SOA (Bhowmik et al., 2021). The significant moderate negative correlation of OC with AT justifies the fact that with a decrease in temperature, OC concentrations will increase. Generally, during winters (selected period of study), lower temperatures favor the low mixing heights of carbonaceous aerosols resulting in their higher deposition in less air mass. Lower temperatures also favor more SOA due to the shifting of gas-to-particles conversion toward the particle phase in the atmosphere (Sonwani and Kulshrestha, 2019; Sonwani and Saxena, 2021). Moreover, EC is having a negative weak correlation with AT. In addition to that, SOA is showing a significant negative moderate correlation with AT which favors the fact that during low temperatures, SOA formation will be more (Rengarajan et al., 2007).
SOA formation and OC/EC ratios during foggy and non-foggy days
Secondary organic aerosol (SOA) is a significant component of atmospheric fine particulate matter and formed through reactions in atmospheric waters (i.e., clouds, fogs, and aerosol water) (Aiken et al., 2008), and responsible for the adverse impacts on both climate and human health (Seinfeld and Pandis, 1998). Formally, SOA forms through the partitioning of semi-volatile compounds of gas-phase photochemical reactions relating to volatile organic compounds (VOCs) and atmospheric oxidants (Seinfeld and Pankow, 2003). Thus, the partitioning theory is an essential tool for SOA modeling (Donahue et al., 2006). In the winter months, the shifting of gas to particle toward the particle phase is one of the significant factors for the formation of SOA that ultimately increase the organic aerosol in the winter season. It was reported that a large fraction of the OC concentration is estimated to be secondary in origin (Cabada et al., 2004; Bisht et al., 2015b). The EC tracer method is one of the well-known SOA measurement tools that are now widely used in carbonaceous aerosol-related studies. The primary OC/EC ratio estimated by the EC tracer method is subject to uncertainties due to the influence of several emission sources with variable meteorological factors that ultimately effects the measurement of accurate SOC and SOA levels. Several studies recently suggested that multiphase chemistry can play an important role in understanding the SOA formation, and the SOA formation mechanism has been explained through wet aerosol and in fogs and clouds (Carlton et al., 2008; Virtanen et al., 2010). Multiphase chemistry involves chemical reactions, transportation, and transformations between gaseous, liquid, and solid matter. Such processes are important for climate and Earth science research as well as for life and health sciences (Poschl and Shiraiwa, 2015). The SOA formation and evolution is a complex multiphase process, involving a series of chemical reactions and mass transport in the gas phase, at the gas–particle interface, and in the particle phase. In the atmosphere, a mixture of organic aerosol with some inorganic salts or acids and water may follow complex, non-ideal behavior including liquid–liquid or liquid–solid phase separation identified in some thermodynamic models and also demonstrated in laboratory and field studies (Pöhlker et al., 2012; Shiraiwa et al., 2013; Ganbavale et al., 2015). Several studies also mentioned that the oxidation of α-pinene and isoprene play an important role in the formation of the SOA particles formed that are liquid at high RH and amorphous semisolid or glassy at low RH and ambient temperature (Renbaum-Wolff et al., 2013; Song et al., 2015). A large number of primary particles from a variety of anthropogenic sources (e.g., fly ash, metal, primary organic matter, and soot particles) are the major aerosols in winter hazes (Chen et al., 2017; Wang et al., 2019). Rapid production of secondary aerosols (e.g., sulfates, nitrates, and secondary organics) via heterogeneous reactions under high relative humidity (RH) mainly elevates haze levels and causes regional hazes (Wang et al., 2016; Murphy et al., 2017; Li et al., 2019; Xing et al., 2019). In the early winter season (October–mid-November), crop residue burning activities are very common and release a huge amount of air pollutants that undergo long-range transport and disturb Delhi's air quality significantly (Saxena et al., 2021). Firework activity-related festival, “Diwali,” is associated with the early winter months that create air pollution load in the Delhi region (Sarkar et al., 2010; Saxena et al., 2020a,b). Other studies, also reported that the regional haze and SOA levels are also enhanced by long-range transport of emissions from distant locations (Zhang et al., 2020, 2021). The dissolution of soluble organic gases into the fog droplets results in oxidation and yields low volatile products that remain along with the particles released by evaporating fog droplets. The mechanism of SOA production is still poorly understood partly due to the complex nature of multiphase processes likely involved in SOA production (Virtanen et al., 2010); while in the case of surface-based fogs, significant scavenging and removal of organic aerosol also occur as droplets that are deposited to surfaces Turpin and Huntzicker (1995). In addition, recent studies during winter months mentioned the anthropogenic emission's dominant role in SOA formation during foggy days (Kaul et al., 2011; Chakraborty et al., 2016; Satish et al., 2017; Saxena et al., 2020b) and observed that the SOA formation is also affected by several factors such as AT, RH, fog, and organic aerosol loading (Frank et al., 1998; Sonwani et al., 2021).
In the present study, the average OC/EC ratio of Delhi during foggy days was comparatively higher with an average value ± standard deviation of 18.62 ± 20.66 to its value on non-foggy days with an average value ± standard deviation of 14.84 ± 18.99. As fog is most often present during the early morning hours; the enhanced OC/EC ratio during this time period on foggy days could be an indication of aqueous phase SOA production (Kaul et al., 2011). Another explanation for this observation could be an increased occurrence of biomass burning at night. The study occurred during winter when overnight heating by fires is likely to be important. In the case of SOA formation, it was found to be higher with a concentration of 10.46 ± 10.76 μg/m3 on foggy days in comparison to non-foggy days with a concentration of 9.1 ± 10.6 μg/m3 over Delhi. The relationship between SOA and OC/EC ratio is shown in Figure 3 and it represents the scatter plot between the SOA and OC/EC ratio during foggy and non-foggy days. Fig 3a shows the OC/EC and SOA relationship at all three sites during foggy days. It was observed that the TI site showed a moderate positive correlation (R = 0.57), whereas ID and UB sites showed a poor correlation with R = 0.08 and R = 0.19, respectively, between SOA and OC/EC ratio during foggy days. Similarly, during non-foggy days, SOA and OC/EC ratios were observed with moderate positive correlation (R = 0.62) at the TI site and poor correlation at ID (R = 0.17) and UB sites (R = 0.34). Thus, the most significant relationship between SOA and OC/EC ratios was observed at the TI site on both days.
On comparing the OC/EC ratio at different sites, the highest average level was observed in decreasing order of TI >ID>UB during foggy and non-foggy days. It represents that the comparatively higher amount of freshly emitted OC and EC particles at TI and ID sites in comparison to UB sites were observed with mixed primary as well secondary OC and EC particles. Aged OC and EC particles are also the characteristic features of the site located at a downstream location (Sonwani and Kulshrestha, 2019). It was also observed that a higher average value of OC/EC ratio (≥10) was found to be 68% at the TI site, 57% at the ID site, and 38% at the UB site during foggy days. Similarly, in the case of non-foggy days, a higher OC/EC ratio was 73% at the TI site, 17% at the ID site, and 6% at the UB site. The higher OC/EC ratio during foggy and non-foggy days represents the biomass burning activities on winter nights. Apart from traffic emission at the TI site, the high percentage of samples with a higher OC/EC ratio also indicated the contribution from residential heating, as this site is very close to the residential and commercial area. Previous studies also mentioned that a high OC/EC ratio indicates a higher contribution of OC, indicating lower traffic emissions or a higher non-traffic contribution (e.g., biomass burning) at the same time as a low OC/EC ratio indicates a high contribution from traffic emissions (Pant et al., 2017). The result shows that there is no significant difference in the SOA formation between foggy and non-foggy days. However, there was a significant difference in SOA formation between different sites, where, the ID site was predominantly contributing to SOA formation over Delhi and followed by TI and UB sites during both days. In addition, recent studies in wintertime also mentioned biomass burning emission as a dominant contributor to the SOA formation during winter months (Chakraborty et al., 2016; Saxena et al., 2020a).
Conclusion
The present study hypothesized that the fog episodes in Delhi were associated with enhanced production of SOA. The findings of the study may be summarized as follows:
• The enhanced SOA production in Delhi is due to aqueous phase chemistry, hence high SOA production was observed during foggy days as compared to the non-foggy period. Results also indicate the higher SOA formation at industrial and traffic intersection sites in comparison to the urban background site in Delhi.
• The higher levels of carbonaceous components at the ID site during both periods indicated the high carbonaceous aerosol emissions due to industrial activities. The ID site was also identified with high SOA production under the influence of high OC at the industrial site.
• Correlation observations found that SOA was strongly positively correlated with OC and moderately positively correlated with EC. The lower temperature also favors SOA formation due to the shifting of gas-to-particles conversion toward the particle phase. However, relative humidity had no detectable influence on SOA production. AT was observed with a significant moderate negative correlation with OC and a negative weak correlation with EC. Scatter plots between SOA and OC/EC ratio indicated that the TI site was observed with a moderate positive correlation, while ID and UB sites were observed with a poor correlation on foggy and non-foggy days both.
• Thus, the results mentioned that the SOA production may be associated with the OC/EC ratio during foggy and non-foggy days. However, it may vary from site to site, and their levels are influenced by the immediate carbonaceous aerosol emission sources.
• The high average OC concentration during foggy days is due to its hydrophilicity, due to which OC aerosol is easily scavenged from the atmosphere during foggy days; however, due to the hydrophobicity, EC is less effectively scavenged with fog and was observed with relatively less average concentration during foggy days in comparison to non-foggy days.
• Thus, the understanding of the atmospheric chemistry of carbonaceous aerosol during foggy days is important to develop effective air quality management and technological advancements in the region.
Data availability statement
The raw data supporting the conclusions of this article will be made available by the authors, without undue reservation.
Author contributions
SS wrote the full text of manuscript. SS and PS involved in conceptualization and design of the work. PS contributed in acquisition, analysis and interpretation of data for the work, helped in manuscript writing, and coordinated and supervised the entire team. AS involved in acquisition and interpretation of data and critically reviewed the manuscript. All authors contributed to the article and approved the submitted version.
Acknowledgments
The authors pay sincere thanks to Central Instrumentation Facility, SES, JNU and SERB (SR/FTP/ES-74/2013) to facilitate sampling and analysis of samples.
Conflict of interest
The authors declare that the research was conducted in the absence of any commercial or financial relationships that could be construed as a potential conflict of interest.
Publisher's note
All claims expressed in this article are solely those of the authors and do not necessarily represent those of their affiliated organizations, or those of the publisher, the editors and the reviewers. Any product that may be evaluated in this article, or claim that may be made by its manufacturer, is not guaranteed or endorsed by the publisher.
Supplementary material
The Supplementary Material for this article can be found online at: https://www.frontiersin.org/articles/10.3389/frsc.2022.951340/full#supplementary-material
References
Aggarwal, S. G., Kawamura, K., Umarji, G. S., Tachibana, E., Patil, R. S., and Gupta, P. K. (2013). Organic and inorganic markers and stable C-, N-isotopic compositions of tropical coastal aerosols from megacity Mumbai: sources of organic aerosols and atmospheric processing. Atmos. Chem. Phys. 13, 4667–4680. doi: 10.5194/acp-13-4667-2013
Aiken, A. C., DeCarlo, P. F., Kroll, J. H., Worsnop, D. R., Huffman, J. A., Cocherty, K., et al. (2008). O/C and OM/OC ratios of primary, secondary, and ambient organic aerosols with high resolution time-of-flight aerosol mass spectrometry. Environ. Sci. Technol. 42, 4478–4485. doi: 10.1021/es703009q
Ali, K., Acharja, P., Trivedi, D. K., Kulkarni, R., Pithani, P., Safai, P. D., et al. (2019). Characterization and source identification of PM2. 5 and its chemical and carbonaceous constituents during winter fog experiment 2015–16 at Indira Gandhi International Airport, Delhi. Sci. Total Environ. 662, 687–696. doi: 10.1016/j.scitotenv.2019.01.285
Begum, B. A. (2017). Validation of some air quality study methods for measurement of black carbon and organic carbon and identification of their sources. Nucl. Sci. Appl. 26, 1–5.
Bhowmik, H. S., Naresh, S., Bhattu, D., Rastogi, N., Pr?v?t, A. S., and Tripathi, S. N. (2021). Temporal and spatial variability of carbonaceous species (EC; OC; WSOC and SOA) in PM2. 5 aerosol over five sites of Indo-Gangetic Plain. Atmos. Pollut. Res. 12, 375–390. doi: 10.1016/j.apr.2020.09.019
Bikkina, S., Andersson, A., Ram, K., Sarin, M. M., Sheesley, R. J., Kirillova, E. N., et al. (2017). Carbon isotope-constrained seasonality of carbonaceous aerosol sources from an urban location (Kanpur) in the Indo-Gangetic Plain. J. Geophys. Res. Atmos. 122, 4903–4923. doi: 10.1002/2016JD025634
Bisht, D. S., Dumka, U. C., Kaskaoutis, D. G., Pipal, A. S., Srivastava, A. K., Soni, V. K., et al. (2015a). Carbonaceous aerosols and pollutants over Delhi urban environment: temporal evolution, source apportionment and radiative forcing. Sci. Total Environ. 521, 431–445. doi: 10.1016/j.scitotenv.2015.03.083
Bisht, D. S., Srivastava, A. K., Pipal, A. S., Srivastava, M. K., Pandey, A. K., Tiwari, S., et al. (2015b). Aerosol characteristics at a rural station in southern peninsular India during CAIPEEX-IGOC: physical and chemical properties. Environ. Sci. Pollut. Res. 22, 5293–5304. doi: 10.1007/s11356-014-3836-1
Cabada, J. C., Pandis, S. N., Subramanian, R., Robinson, A. L., Polidori, A., and Turpin, B. (2004). Estimating the secondary organic aerosol contribution to PM2. 5 using the EC tracer method special issue of aerosol science and technology on findings from the fine particulate matter supersites program. Aerosol Sci. Technol. 38, 140–155. doi: 10.1080/02786820390229084
Cao, G., Zhang, X., and Zheng, F. (2006). Inventory of black carbon and organic carbon emissions from China. Atmos. Environ. 40, 6516–6527. doi: 10.1016/j.atmosenv.2006.05.070
Cao, J. J., Lee, S. C., Ho, K. F., Zhang, X. Y., Zou, S. C., Fung, K. K., et al. (2003). Characteristics of carbonaceous aerosol in Pearl River Delta Region, China during 2001 winter period. Atmos. Environ. 37, 1451–1460. doi: 10.1016/S1352-2310(02)01002-6
Cao, J. J., Zhu, C. S., Tie, X. X., Geng, F. H., Xu, H. M., Ho, S. S. H., et al. (2013). Characteristics and sources of carbonaceous aerosols from Shanghai, China. Atmos. Chem. Phys. 13, 803–817. doi: 10.5194/acp-13-803-2013
Carlton, A. G., Turpin, B. J., Altieri, K. E., Seitzinger, S. P., Mathur, R., Roselle, S. J., et al. (2008). CMAQ model performance enhanced when in-cloud secondary organic aerosol is included: comparisons of organic carbon predictions with measurements. Environ. Sci. Technol. 42, 8798–8802. doi: 10.1021/es801192n
Chakraborty, A., Gupta, T., and Tripathi, S. N. (2016). Combined effects of organic aerosol loading and fog processing on organic aerosols oxidation, composition, and evolution. Sci. Total Environ. 573, 690–698. doi: 10.1016/j.scitotenv.2016.08.156
Chen, J., Li, C., Ristovski, Z., Milic, A., Gu, Y., Islam, M. S., et al. (2017). A review of biomass burning: emissions and impacts on air quality, health and climate in China. Sci. Total Environ. 579, 1000–1034. doi: 10.1016/j.scitotenv.2016.11.025
Chen, J., Ying, Q., and Kleeman, M. J. (2010). Source apportionment of wintertime secondary organic aerosol during the California regional PM10/PM2. 5 air quality study. Atmos. Environ. 44, 1331–40. doi: 10.1016/j.atmosenv.2009.07.010
Cheng, Y., Lee, S. C., Ho, K. F., and Louie, P. K. K. (2006). On-road particulate matter (PM2. 5) and gaseous emissions in the Shing Mun Tunnel, Hong Kong. Atmos. Environ. 40, 4235–4245. doi: 10.1016/j.atmosenv.2006.04.002
Chow, J. C., Watson, J. G., Pritchett, L. C., Pierson, W. R., Frazier, C. A., and Purcell, P. G. (1993). The DRI thermal/optical reflectance carbon analysis system: Description, evaluation and applications in US air quality studies. Atmos. Environ. Part A 27, 1185–1201. doi: 10.1016/0960-1686,90245-T
Collett, J. L. Jr, Herckes, P., Youngster, S., and Lee, T. (2008). Processing of atmospheric organic matter by California radiation fogs. Atmos. Res. 87, 232–241. doi: 10.1016/j.atmosres.2007.11.005
Dey, S., Di Girolamo, L., van Donkelaar, A., Tripathi, S. N., Gupta, T., and Mohan, M. (2012). Variability of outdoor fine particulate (PM2. 5) concentration in the Indian Subcontinent: a remote sensing approach. Remote Sens. Environ. 127, 153–161. doi: 10.1016/j.rse.2012.08.021
Donahue, N. M., Robinson, A. L., Stanier, C. O., and Pandis, S. N. (2006). The coupled partitioning, dilution, and chemical aging of semivolatile organics. Environ. Sci. Technol. 40, 2635–2643. doi: 10.1021/es052297c
Du, Z., He, K., Cheng, Y., Duan, F., Ma, Y., Liu, J., et al. (2014). A yearlong study of water-soluble organic carbon in Beijing II: light absorption properties. Atmosph. Environ. 89, 235–241. doi: 10.1016/j.atmosenv.2014.02.022
Dutkiewicz, V. A., Alvi, S., Ghauri, B. M., Choudhary, M. I., and Husain, L. (2009). Black carbon aerosols in urban air in South Asia. Atmos. Environ. 43, 1737–1744. doi: 10.1016/j.atmosenv.2008.12.043
Frank, G., Martinsson, B. G., Cederfelt, S. I., Berg, O. H., Swietlicki, E., Wendisch, M., et al. (1998). Droplet formation and growth in polluted fogs. Contribut. Atmos. Phys. 71, 65–85.
Ganbavale, G., Zuend, A., Marcolli, C., and Peter, T. (2015). Improved AIOMFAC model parameterisation of the temperature dependence of activity coefficients for aqueous organic mixtures. Atmos. Chem. Phys. 15, 447–493. doi: 10.5194/acp-15-447-2015
Gao, Y., Lee, S. C., Huang, Y., Chow, J. C., and Watson, J. G. (2016). Chemical characterization and source apportionment of size-resolved particles in Hong Kong sub-urban area. Atmosph. Res. 170, 112–122. doi: 10.1016/j.atmosres.2015.11.015
Goel, A., Saxena, P., Sonwani, S., Rathi, S., Srivastava, A., Bharti, A. K., et al. (2021). Health benefits due to reduction in respirable particulates during COVID-19 lockdown in India. Aerosol Air Qual Res. 21, 200460. doi: 10.4209/aaqr.200460
Government of Delhi (2014). Transport Department, Statistics About Number of Vehicle as on 30 April 2014. Available online at: http://www.delhi.gov.in (accessed July 25, 2019).
Griffin, R. J., Dabdub, D., Kleeman, M. J., Fraser, M. P., Cass, G. R., and Seinfeld, J. H. (2002). Secondary organic aerosol, 3. Urban/regional scale model of size- and composition-resolved aerosols. J. Geophys. Res. 107, 4334. doi: 10.1029/2001JD000544
Gundel, L. A., Benner, W. H., and Hansen, A. D. A. (1994). Chemical composition of fog water and interstitial aerosol in Berkeley, California. Atmos. Environ. 28, 2715–2725. doi: 10.1016/1352-231090443-X
Han, Y. M., Chen, L. W., Huang, R. J., Chow, J. C., Watson, J. G., Ni, H. Y., et al. (2016). Carbonaceous aerosols in megacity Xi'an, China: implications of thermal/optical protocols comparison. Atmos. Environ. 132, 58–68. doi: 10.1016/j.atmosenv.2016.02.023
Helmig, D., Ortega, J., Duhl, T., Tanner, D., Guenther, A., Harley, P., et al. (2007). Sesquiterpene emissions from pine trees– identifications, emission rates and flux estimates for the contiguous United States. Environ. Sci. Technol. 41, 1545–1553. doi: 10.1021/es0618907
Ho, K. F., Lee, S. C., Cao, J. J., Li, Y. S., Chow, J. C., Watson, J. G., et al. (2006). Variability of organic and elemental carbon, water soluble organic carbon, and isotopes in Hong Kong. Atmos. Chem. Phys. 6, 4569–4576. doi: 10.5194/acp-6-4569-2006
Huang, X. H., Bian, Q. J., Louie, P. K., and Yu, J. Z. (2014). Contributions of vehicular carbonaceous aerosols to PM 2.5 in a roadside environment in Hong Kong. Atmos. Chem. Phys. 14, 9279–9293. doi: 10.5194/acp-14-9279-2014
Husain, L., Dutkiewicz, V. A., Khan, A. J., and Ghauri, B. M. (2007). Characterization of carbonaceous aerosols in urban air. Atmos. Environ. 41, 6872–6883. doi: 10.1016/j.atmosenv.2007.04.037
Jain, M., Dimri, A. P., and Niyogi, D. (2017). Land-air interactions over urban-rural transects using satellite observations: analysis over Delhi, India from 1991–2016. Remote Sens. 9, 1283. doi: 10.3390/rs9121283
Jain, M., Saxena, P., Sharma, S., and Sonwani, S. (2021). Investigation of forest fire activity changes over the central India domain using satellite observations during 2001–2020. GeoHealth 5, e2021GH000528. doi: 10.1029/2021GH000528
Jain, S., Sharma, S. K., Vijayan, N., and Mandal, T. K. (2020). Seasonal characteristics of aerosols (PM2. 5 and PM10) and their source apportionment using PMF: a four year study over Delhi, India. Environ. Pollut. 262, 114337. doi: 10.1016/j.envpol.2020.114337
Johnson, D., Utembe, S. R., and Jenkin, M. E. (2006). Simulating the detailed chemical composition of secondary organic aerosol formed on a regional scale during the TORCH 2003 campaign in the southern UK. Atmos. Chem. Phys. 6, 419–431. doi: 10.5194/acp-6-419-2006
Kaul, D. S., Gupta, T., Tripathi, S. N., Tare, V., and Collett, J. L. Jr. (2011). Secondary organic aerosol: a comparison between foggy and nonfoggy days. Environ. Sci. Technol. 45, 7307–7313. doi: 10.1021/es201081d
Kleeman, M. J., Ying, Q., Lu, J., Mysliwiec, M. J., Griffin, R. J., Chen, J., et al. (2007). Source apportionment of secondary organic aerosol during a severe photochemical smog episode. Atmos. Environ. 41, 576–591. doi: 10.1016/j.atmosenv.2006.08.042
Kroll, J. H., Ng, N. L., Murphy, S. M., Flagan, R. C., and Seinfeld, J. H. (2006). Secondary organic aerosol formation from isoprene photooxidation. Environ. Sci. Technol. 40, 1869–1877. doi: 10.1021/es0524301
Lee, S., Wang, Y, and Rusell, A. G. (2010). Assessment of secondary organic carbon in the southeastern united states: a review. J. Air Waste Manage. Assoc. 60, 1282–1292. doi: 10.3155/1047-3289.60.11.1282
Li, J., Wang, G., Zhang, Q., Li, J., Wu, C., Jiang, W., et al. (2019). Molecular characteristics and diurnal variations of organic aerosols at a rural site in the North China Plain with implications for the influence of regional biomass burning. Atmos. Chem. Phys. 19, 10481–10496. doi: 10.5194/acp-19-10481-2019
Li, Y. C., Shu, M., Ho, S. S. H., Wang, C., Cao, J. J., Wang, G. H., et al. (2015). Characteristics of PM2. 5 emitted from different cooking activities in China. Atmos. Res. 166, 83–91. doi: 10.1016/j.atmosres.2015.06.010
Murphy, B. N., Woody, M. C., Jimenez, J. L., Carlton, A. M. G., Hayes, P. L., Liu, S., et al. (2017). Semivolatile POA and parameterized total combustion SOA in CMAQv5. 2: impacts on source strength and partitioning. Atmos. Chem. Phys. 17, 11107–11133. doi: 10.5194/acp-17-11107-2017
Odum, J. R., Jungkamp, T. P. W., Griffin, R. J., Forstner, H. J. L., Flagan, R. C., and Seinfeld, J. H. (1997). Aromatics, reformulated gasoline, and atmospheric organic aerosol formation. Environ. Sci. Technol. 31, 1890–1897. doi: 10.1021/es960535l
Pal, R., Chowdhury, S., Dey, S., and Sharma, A. R. (2018). 18-year ambient PM2. 5 exposure and night light trends in Indian cities: vulnerability assessment. Aerosol Air Qual. Res. 18, 2332–2342. doi: 10.4209/aaqr.2017.10.0425
Pant, P., Shi, Z., Pope, F. D., and Harrison, R. M. (2017). Characterization of traffic-related particulate matter emissions in a road tunnel in Birmingham, UK: trace metals and organic molecular markers. Aerosol Air Qual. Res. 17, 117–130. doi: 10.4209/aaqr.2016.01.0040
Pio, C., Cerqueira, M., Harrison, R. M., Nunes, T., Mirante, F., Alves, C., et al. (2011). OC/EC ratio observations in Europe: re-thinking the approach for apportionment between primary and secondary organic carbon. Atmos. Environ. 45, 6121–6132. doi: 10.1016/j.atmosenv.2011.08.045
Pöhlker, C., Wiedemann, K. T., Sinha, B., Shiraiwa, M., Gunthe, S. S., Smith, M., et al. (2012). Biogenic potassium salt particles as seeds for secondary organic aerosol in the Amazon. Science 337, 1075–1078. doi: 10.1126/science.1223264
Pöschl, U. (2005). Atmospheric aerosols: composition, transformation, climate and health effects. Angew. Chem. Int. Ed. 44, 7520–7540. doi: 10.1002/anie.200501122
Poschl, U., and Shiraiwa, M. (2015). Multiphase chemistry at the atmosphere-biosphere interface influencing climate and public health in the anthropocene. Chem. Rev. 115, 4440–4475. doi: 10.1021/cr500487s
Pun, B. K., Wu, S., Seigneur, C., Seinfeld, J. H., Griffin, R. J., and Pandis, S. N. (2003). Uncertainties in modeling secondary organic aerosols: three-dimensional modeling studies in Nashville/Western Tennessee. Environ. Sci. Technol. 37, 3647–3661. doi: 10.1021/es0341541
Rajput, P., Sarin, M., Sharma, D., and Singh, D. (2016). “Characteristics and emission budget of carbonaceous species from post-harvest agricultural-waste burning in source region of the Indo-Gangetic Plain,” in Air Quality. Apple Academic Press, 271–292.
Ram, K., and Sarin, M. M. (2010). Spatio-temporal variability in atmospheric abundances of EC, OC and WSOC over Northern India. J. Aerosol Sci. 41, 88–98. doi: 10.1016/j.jaerosci.2009.11.004
Ravindra, K., Singh, T., Mandal, T. K., Sharma, S. K., and Mor, S. (2022). Seasonal variations in carbonaceous species of PM2. 5 aerosols at an urban location situated in Indo-Gangetic Plain and its relationship with transport pathways, including the potential sources. J. Environ. Manage. 303, 114049. doi: 10.1016/j.jenvman.2021.114049
Renbaum-Wolff, L., Grayson, J. W., Bateman, A. P., Kuwata, M., Sellier, M., Murray, B. J., et al. (2013). Viscosity of α-pinene secondary organic material and implications for particle growth and reactivity. Proc. Natl. Acad. Sci. U.S.A. 110, 8014–8019. doi: 10.1073/pnas.1219548110
Rengarajan, R., Sarin, M. M., and Sudheer, A. K. (2007). Carbonaceous and inorganic species in atmospheric aerosols during wintertime over urban and high-altitude sites in North India. J. Geophys. Res. Atmos. 112, e2006JD008150. doi: 10.1029/2006JD008150
Saarikoski, S., Timonen, H., Saarnio, K., Aurela, M., Järvi, L., Keronen, P., et al. (2008). Sources of organic carbon in fine particulate matter in northern European urban air. Atmos. Chem. Phys. 8, 6281–6295. doi: 10.5194/acp-8-6281-2008
Sarkar, S., Khillare, P. S., Jyethi, D. S., Hasan, A., and Parween, M. (2010). Chemical speciation of respirable suspended particulate matter during a major firework festival in India. J. Hazard. Mater. 184, 321–330. doi: 10.1016/j.jhazmat.2010.08.039
Satish, R., Shamjad, P., Thamban, N., Tripathi, S., and Rastogi, N. (2017). Temporal characteristics of brown carbon over the central Indo-Gangetic Plain. Environ. Sci. Technol. 51, 6765–6772. doi: 10.1021/acs.est.7b00734
Saxena, P., Sonwani, S., Sharma, S. K., Kumar, P., and Chandra, N. (2020b). Carbonaceous aerosol variations in foggy days: a critical analysis during the fireworks festival. Fresenius Environ. Bull. 29, 6639–6656.
Saxena, P., Sonwani, S., Srivastava, A., Jain, M., Srivastava, A., Bharti, A., et al. (2021). Impact of crop residue burning in Haryana on the air quality of Delhi, India. Heliyon 7, e06973. doi: 10.1016/j.heliyon.2021.e06973
Saxena, P., Srivastava, A., Verma, S., Singh, L., and Sonwani, S. (2020a). “Analysis of atmospheric pollutants during fireworks festival ‘Diwali'at a residential site Delhi in India,” in Measurement, Analysis and Remediation of Environmental Pollutants, eds Tarun, G., Swatantra, P.S., Prashant, R., Avinash, K.A. (Singapore: Springer), 91–105. doi: 10.1007/978-981-15-0540-9_4
Schauer, J. J., Kleeman, M. J., Cass, G. R., and Simoneit, B. R. (2001). Measurement of emissions from air pollution sources. 3. C1–C29 organic compounds from fireplace combustion of wood. Environ. Sci. Technol. 35, 1716–1728. doi: 10.1021/es001331e
Schauer, J. J., Kleeman, M. J., Cass, G. R., and Simoneit, B. R. T. (2002). Measurement of emissions from air pollution sources. 4. C1-C27 organic compounds from cooking with seed oils. Environ. Sci. Technol. 36, 567–575. doi: 10.1021/es002053m
Schell, B., Ackermann, I. J., Hass, H., Binkowski, F. S., and Ebel, A. (2001). Modeling the formation of secondary organic aerosol within a comprehensive air quality model system. J. Geophys. Res. 106, 28275–28294. doi: 10.1029/2001JD000384
See, S. W., and Balasubramanian, R. (2008). Chemical characteristics of fine particles emitted from different gas cooking methods. Atmos. Environ. 42, 8852–8862. doi: 10.1016/j.atmosenv.2008.09.011
Seinfeld, J. H., and Pandis, S.N. (2006). Atmospheric Chemistry and Physics: From Air Pollution to Climate Change. New York, NY: John Willey & Sons. Inc.
Seinfeld, J. H., and Pandis, S. N. (1998). From air pollution to climate change. Atmos. Chem. Phys. 1326.
Seinfeld, J. H., and Pankow, J. F. (2003). Organic atmospheric particulate material. Annu. Rev. Phys. Chem. 54, 121–140. doi: 10.1146/annurev.physchem.54.011002.103756
Sharma, S. K., Sharma, A., Saxena, M., Choudhary, N., Masiwal, R., Mandal, T. K., et al. (2016). Chemical characterization and source apportionment of aerosol at an urban area of Central Delhi, India. Atmos. Pollut. Res. 7, 110–121. doi: 10.1016/j.apr.2015.08.002
Shiraiwa, M., Zuend, A., Bertram, A. K., and Seinfeld, J. H. (2013). Gas–particle partitioning of atmospheric aerosols: interplay of physical state, non-ideal mixing and morphology. Phys. Chem. Chem. Phys. 15, 11441–11453. doi: 10.1039/c3cp51595h
Shivani Gadi, R., Sharma, S. K., and Mandal, T. K. (2019). Seasonal variation, source apportionment and source attributed health risk of fine carbonaceous aerosols over National Capital Region, India. Chemosphere 237, 124500. doi: 10.1016/j.chemosphere.2019.124500
Singh, V., Singh, S., and Biswal, A. (2021). Exceedances and trends of particulate matter (PM2. 5) in five Indian megacities. Sci. Total Environ. 750, 141461. doi: 10.1016/j.scitotenv.2020.141461
Song, M., Liu, P. F., Hanna, S. J., Li, Y. J., Martin, S. T., and Bertram, A. K. (2015). Relative humidity-dependent viscosities of isoprene-derived secondary organic material and atmospheric implications for isoprene-dominant forests. Atmos. Chem. Phys. 15, 5145–5159. doi: 10.5194/acp-15-5145-2015
Song, Y., Xie, S., Zhang, Y., Zeng, L., Salmon, L. G., and Zheng, M. (2006). Source apportionment of PM2. 5 in Beijing using principal component analysis/absolute principal component scores and UNMIX. Sci. Tot. Environ. 372, 278–286. doi: 10.1016/j.scitotenv.2006.08.041
Sonwani, S., and Kulshrestha, U. (2018). Morphology, elemental composition and source identification of airborne particles in Delhi, India. J. Indian Geophys. Union 22, 607–620.
Sonwani, S., and Kulshrestha, U. C. (2019). PM10 carbonaceous aerosols and their real-time wet scavenging during monsoon and non-monsoon seasons at Delhi, India. J. Atmos. Chem. 76, 171–200. doi: 10.1007/s10874-019-09396-z
Sonwani, S., and Saxena, P. (2021). Water-insoluble carbonaceous components in rainwater over an urban background location in Northern India during pre-monsoon and monsoon seasons. Environ. Sci. Pollut. Res. 28, 53058–53073. doi: 10.1007/s11356-021-14132-w
Sonwani, S., Saxena, P., and Khillare, P. S. (2022). Profile of atmospheric particulate PAHs near busy roadway in tropical megacity, India. Inhalat. Toxicol. 34, 39–50. doi: 10.1080/08958378.2022.2030442
Sonwani, S., Saxena, P., and Kulshrestha, U. (2016). “Role of global warming and plant signaling in BVOC emissions,” in Plant Responses to Air Pollution, eds Ulshrestha, U., and Saxena, P. (Singapore: Springer), 45–57. doi: 10.1007/978-981-10-1201-3_5
Sonwani, S., Saxena, P., and Shukla, A. (2021). Carbonaceous aerosol characterization and their relationship with meteorological parameters during summer monsoon and winter monsoon at an industrial region in Delhi, India. Earth Space Sci. 8, e2020EA001303. doi: 10.1029/2020EA001303
Srinivas, B., and Sarin, M. M. (2014). PM2. 5, EC and OC in atmospheric outflow from the Indo-Gangetic Plain: temporal variability and aerosol organic carbon-to-organic mass conversion factor. Sci. Total Environ. 487, 196–205. doi: 10.1016/j.scitotenv.2014.04.002
Subramanian, R., Donahue, N. M., Bernardo-Bricker, A., Rogge, W. F., and Robinson, A. L. (2007). Insights into the primary–secondary and regional–local contributions to organic aerosol and PM2. 5 mass in Pittsburgh, Pennsylvania. Atmos. Environ. 41, 7414–7433. doi: 10.1016/j.atmosenv.2007.05.058
Turpin, B. J., and Huntzicker, J. J. (1995). Identification of secondary organic aerosol episodes and quantitation of primary and secondary organic aerosol concentrations during SCAQS. Atmos. Environ. 29, 3527–3544. doi: 10.1016/1352-231000276-Q
Virtanen, A., Joutsensaari, J., Koop, T., Kannosto, J., Yli-Pirila, P., Leskinen, J., et al. (2010). An amorphous solid state of biogenic secondary organic aerosol particles. Nature 467, 824–827. doi: 10.1038/nature09455
Wang, D., Zhou, B., Fu, Q., Zhao, Q., Zhang, Q., Chen, J., et al. (2016). Intense secondary aerosol formation due to strong atmospheric photochemical reactions in summer: observations at a rural site in eastern Yangtze River Delta of China. Sci. Total Environ. 571, 1454–1466. doi: 10.1016/j.scitotenv.2016.06.212
Wang, J., Liu, D., Ge, X., Wu, Y., Shen, F., Chen, M., et al. (2019). Characterization of black carbon-containing fine particles in Beijing during wintertime. Atmos. Chem. Phys. 19, 447–458. doi: 10.5194/acp-19-447-2019
Weber, R.J., Sullivan, A.P., Peltier, R.E., Russell, A., Yan, B., Zheng, M., et al. (2007). A study of secondary organic aerosol formation in the anthropogenic-influenced southeastern United States. J. Geophys. Res. Atmos. 112, e2007JD008408. doi: 10.1029/2007JD008408
World Health Organization (2021). WHO Global Air Quality Guidelines: Particulate Matter (PM2. 5 and PM10), Ozone, Nitrogen Dioxide, Sulfur Dioxide and Carbon Monoxide. World Health Organization, xxi, 273. Available online at: https://apps.who.int/iris/handle/10665/345329
Xie, Y., Zhao, B., Zhang, L., and Luo, R. (2015). Spatiotemporal variations of PM2. 5 and PM10 concentrations between 31 Chinese cities and their relationships with SO2, NO2, CO and O3. Particuology 20, 141–149. doi: 10.1016/j.partic.2015.01.003
Xing, L., Wu, J., Elser, M., Tong, S., Liu, S., Li, X., et al. (2019). Wintertime secondary organic aerosol formation in Beijing-Tianjin-Hebei (BTH): contributions of HONO sources and heterogeneous reactions. Atmos. Chem. Phys. 19, 2343–2359. doi: 10.5194/acp-19-2343-2019
Zhang, F., Zhao, J., Chen, J., Xu, Y., and Xu, L. (2011). Pollution characteristics of organic and elemental carbon in PM 2.5 in Xiamen, China. J. Environ. Sci. 23, 1342–1349. doi: 10.1016/S1001-0742,60559-1
Zhang, J., Liu, L., Xu, L., Lin, Q., Zhao, H., Wang, Z., et al. (2020). Exploring wintertime regional haze in northeast China: role of coal and biomass burning. Atmos. Chem. Phys. 20, 5355–5372. doi: 10.5194/acp-20-5355-2020
Zhang, J., Yuan, Q., Liu, L., Wang, Y., Zhang, Y., Xu, L., et al. (2021). Trans-Regional transport of haze particles from the North China Plain to Yangtze River Delta during winter. J. Geophys. Res. Atmos. 126, e2020JD033778. doi: 10.1029/2020JD033778
Keywords: organic carbon, elemental carbon, air quality, secondary organic aerosol, Delhi
Citation: Sonwani S, Saxena P and Srivastava A (2022) Carbonaceous aerosol variability and SOA formation during foggy days in Delhi, India. Front. Sustain. Cities 4:951340. doi: 10.3389/frsc.2022.951340
Received: 23 May 2022; Accepted: 25 July 2022;
Published: 31 August 2022.
Edited by:
Weijun Li, Zhejiang University, ChinaReviewed by:
Jian Zhang, Yantai University, ChinaSudhir Kumar Sharma, National Physical Laboratory (CSIR), India
Copyright © 2022 Sonwani, Saxena and Srivastava. This is an open-access article distributed under the terms of the Creative Commons Attribution License (CC BY). The use, distribution or reproduction in other forums is permitted, provided the original author(s) and the copyright owner(s) are credited and that the original publication in this journal is cited, in accordance with accepted academic practice. No use, distribution or reproduction is permitted which does not comply with these terms.
*Correspondence: Pallavi Saxena, cGFsbGF2aWVudmlyb25tZW50JiN4MDAwNDA7Z21haWwuY29t