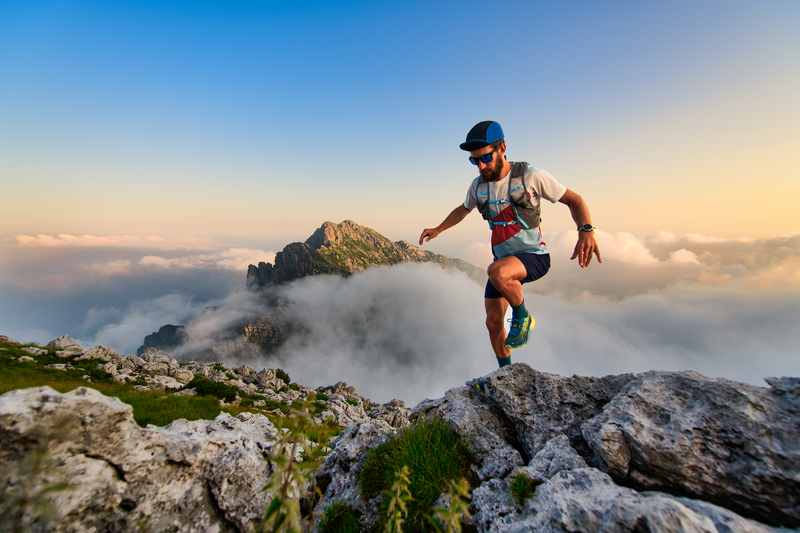
95% of researchers rate our articles as excellent or good
Learn more about the work of our research integrity team to safeguard the quality of each article we publish.
Find out more
REVIEW article
Front. Sustain. Cities , 18 August 2021
Sec. Climate Change and Cities
Volume 3 - 2021 | https://doi.org/10.3389/frsc.2021.690444
This article is part of the Research Topic The Role of Climate and Air Pollution in Human Health and Urban Chemistry in Asian Cities View all 11 articles
Nanoparticles (NPs) are receiving an increasing attention from many scientific communities due to their strong influence on human health. NPs are an important marker of air pollution caused by a variety of natural and anthropogenic sources. Due to their ultrafine size, they can be suspended in the atmosphere for a long time and can thus travel larger distances and cause several health issues after exposure. A variety of NPs that are found in indoor and outdoor settings cause respiratory and cardiovascular diseases. Exposure to NPs through active and passive smoking and household and occupational subjection is reported with thick septum, shortness of breath, and a high level of interleukin protein and tumour necrosis factor (TNF-α) that cause tumour generation in the exposed population. This comprehensive review summarises NPs' source, exposure, and impact on different organ systems. Respiratory models (experimental and computational) used to determine the particle's deposition, airflow transport, and health impact are also discussed. Further, muco-ciliary escalation and macrophage activity, the body's clearance mechanisms after exposure to NPs, have been mentioned. An in-depth analysis of exposure to NPs through inhalation and their health impact has been provided with detailed insights about oxidative stress, inflammation, genotoxicity, and tumourigenicity. Overall, this review offers scientific evidence and background for researchers working in the field of epidemiology, biochemistry, and toxicological studies with reference to atmospheric nanoparticles.
Among particles of various sizes present in the atmosphere, NPs are particles with sizes of 1–100 nm in diameter (Kumar et al., 2010; Nemmar et al., 2013). The residence time of particles in the diameter range of 0.1–10 μm is ~1 week. Coarser particulate matter is removed by settling, while smaller particles can only be removed via diffusion and coagulation (Scholl et al., 2012; Slezakova et al., 2013; Bakshi et al., 2015). Moreover, the fine size and higher atmospheric retention time of NPs are the basic reasons behind the difficultly in their removal from the atmosphere, consequently causing human health hazard. Nanoparticles originate from vehicular exhaust, combustion reactions, forest fires, and industrial emission; they are also produced in the bodies of insects, plants, and humans (Jeevanandam et al., 2018). The atmospheric concentration of nanoparticles is high in Asian countries due to the high rate of urbanisation, industrialisation, vehicular emission, extreme events (dust storms, volcanic eruption, forest fire, etc.), and episodic events (such as firework activity and crop residue burning) (Nakajima et al., 2007; Saxena et al., 2020; Sonwani et al., 2021). Most of the anthropogenic nanoparticles are made up of carbon, silicon, metal, or metal oxides (Querol et al., 2004). India's coal-fired plants emit more than 110,000 tonnes of particulate matter, 4.3 million tonnes of SO2, and 1.2 million tonnes of NOx per year (Oliveira et al., 2014).
According to the 2007 report of the Intergovernmental Panel on Climate Change (IPCC), nanoparticles act as precursors to coarser particles through their aggregation during the atmospheric ageing process thereby influencing the global climate and urban visibility. Due to their distinct chemical composition and reactivity, they also alter the atmospheric chemistry and global climate (Slezakova et al., 2013). Moreover, these particles are responsible for global warming (by absorbing solar radiation) and cooling effects (by scattering solar radiation), further affecting the global radiation budget (Buseck and Adachi, 2008). Another striking phenomenon is the production of atmospheric brown clouds (ABCs). A study found out that 70% of the ABCs over South Asia are made up of soot emitted from biomass burning, responsible for the melting of glaciers, climate change, and reducing sunlight, which further impacts agricultural production (Engling and Gelencsér, 2010).
NPs also play a significant role in plants by entering via two pathways, viz. apoplastic and symplastic, and thus interact with the cells and environment mostly using Van Der Waals, electrostatic, and stearic forces (Vera-Reyes et al., 2018). NPs-bound metal oxides are found to be one of the harmful agents for plants (Giorgetti, 2019). NPs are also one of the phytotoxic agents for plant species, besides tropospheric ozone, that affect the global food security system. NPs are believed to have adverse effects on human health as they enter via multiple exposure pathways such as dermal, inhalation, and ingestion (Sajid et al., 2015). These exposure pathways are known to have a direct connexion with the incipience of neurodegenerative disorders and defects in apoptosis (Chen et al., 2016). With inhalation exposure being the major pathway, NPs enter the body via the nasal passage where it gets deposited on the olfactory mucosa of the nasal chamber more than in any other part of the respiratory system (Seaton et al., 2010). They cause inflammatory responses and oxidative stress in the lungs, impairing the epithelial cells of the system (Rothen-Rutishauser et al., 2008; Nemmar et al., 2013). Bronchial epithelial cells are known to release interleukin 6, a pro-inflammatory cytokine, on exposure to nano-sized diesel exhaust particles (DEP) (Donaldson et al., 2007). The two most prevailing respiratory diseases, asthma and COPD, currently affecting 300 and 210 million people, respectively, across the world are also influenced by exposure to fine particles (Yhee et al., 2016). Moreover, NPs cause acute and chronic problems ranging from asthma and metal fume fever to fibrosis, carcinogenesis, and other chronic lung maladies (Bakand et al., 2012). Herr et al. (2003) also confirms that respiratory tract infections such as bronchitis, wheezing, and general health issues (tiredness, shivering, and nausea) are caused due to the presence of bioaerosols like pollen and fungi moulds in the air. In addition, some studies prove that exposure to NPs cause intestinal fibrosis, ulcers in the gastrointestinal tract, and oxidative DNA damage in caco-2 cells of the colon (Gerloff et al., 2009; Sajid et al., 2015). In low- and middle-income countries, around 4–8% of recorded mortality is solely caused by indoor smoke from solid fuel, urban air pollution, and occupational airborne particulates, after tobacco, leading to chronic respiratory diseases (Moitra et al., 2015). According to a 2009 WHO report, in India, 488,200 people die prematurely every year as a result of indoor air pollution, whereas 119,900 die prematurely every year because of outdoor air pollution. The number of deaths are increasing and alarmingly high due to rapid industrialisation, urbanisation, and change in lifestyle activities. The rapid population expansion in developing nations like India also impose a huge burden on the physical and mental health of people, especially those residing in megacities (Sonwani and Kulshreshtha, 2016; Saxena and Sonwani, 2019; Goel et al., 2021). The incidence of respiratory diseases in Delhi is 12 times the national average, and 30% of the population was found suffering from 166 different respiratory disorders (Gurjar et al., 2016). Many in vitro and in vivo models of the airway walls of lungs are used to evaluate the extent and outcome of exposure (Nakajima et al., 2007; Slezakova et al., 2013). Several countries are trying to devise measures that can reduce the adverse impacts of atmospheric pollutants (Slezakova et al., 2013; Saxena and Sonwani, 2020); however, there is a lack of studies on the effects of NPs on human health, especially in Asian countries.
Thus, the present review focuses on NPs' source, exposure, and impact on different human body systems, particularly the respiratory system. It also provides a detailed overview of the appearance of toxicological, genotoxic, and tumourigenic effects in the human body due to exposure to NPs. In particular, this is the first review that offers deep insights about the origin of atmospheric NPs, their transportation, deposition, and toxicity, which leads to hazardous diseases in humans.
The sources of the nanoparticles in the atmosphere can be natural and anthropogenic. Details on the sources are described in Figure 1.
Figure 1. Emission sources (Natural and anthropogenic) of nanoparticles in the environment (adopted from Buzea and Pacheco, 2017).
About 10% of the total aerosols in the atmosphere are generated by human activity, whereas the remaining 90% of atmospheric aerosols are naturally generated (Pipal et al., 2014; Jeevanandam et al., 2018; Sonwani and Saxena, 2021). Nanoparticles are abundant in nature and are released/produced from a variety of sources, mainly from biogenic emission, sea spray, forest fire, volcanic eruption, landslide, and dust storm. Organic compounds form a large proportion of atmospheric nanoparticles because of their ability to react with clouds and air particles (Riipinen et al., 2012). Both natural and anthropogenic sources make ambient air rich in VOCs, nitrogen oxides, and primary organic aerosols, which ultimately react to form secondary organic aerosols (SOA) (Tiwari and Saxena, 2021). About 90% of the SOA formation is because of biogenic volatile organic compounds (bVOCs) that constitute about 10–40% of the global organic aerosol mass (Volkamer et al., 2006; Saxena and Kulshrestha, 2016; Sonwani et al., 2016).
The chemical composition of NPs differs significantly from place to place as it depends on the types of local sources and their relative contributions. Very few studies have investigated the contribution of NPs in different ambient atmosphere. A study in Pittsburgh mentioned that NPs are mostly composed of organic carbon (OC) and salts of ammonium and sulphate constituting 45–55% and 35–40% respectively (Kuhn et al., 2005); Another study at two sites in Los Angeles found that the composition of OC ranged from 32 to 69%, EC from 1 to 34%, sulphate from 0 to 24%, and nitrate from 0 to 4% (Sardar et al. (2005). Moreover, a study based in two sites in Helsinki trace elements (Ca, Na, Fe, K, and Zn) in higher fractions as compared with heavy metals (Ni, V, Cu, and Pb) that contribute not more than 1% of the total weight of NPs (Pakkanen et al., 2001). Furthermore, incomplete combustion and geological sources are the other significant sources of nanoparticles production in the atmosphere. Forest fires and volcanic sources are the major combustion-generated sources, whereas volcanic eruption along with earthquakes, glaciation, and dust storms are the significant geological sources (Strambeanu et al., 2015). Volcanic ash plays a crucial role in the global transport of toxic chemical species released with NPs (Ermolin et al., 2018). Ash released during volcanic eruptions has a very complex structure and comprises both solid and liquid particulate matter. With time, its composition changes due to cooling and chemical reactions (Strambeanu et al., 2015). The concentrations of toxic elements (Ni, Zn, Cd, Ag, Sn, Se, Te, Hg, Tl, Pb, and Bi) in volcanic ash NPs are seemingly higher than the total content of these elements in any other samples (Ermolin et al., 2018). The eruption of Krakatoa volcano on 27 August 1883 was a remarkable historic event where the smoke column reached 80 km in height and particles were thrown into the ionosphere. This caused strange optical effects and a decline in the global temperature by 1.5°C for the next 2 years in North America and Europe (Strambeanu et al., 2015). Likewise, the Pinatubo eruption of 1991 (Luzon, Philippines) injected vast quantities of aerosol particles, SO2, and other sulphate particles into the atmosphere, causing a net effect of global cooling of ~0.5°C and significant ozone depletion (Buseck and Adachi, 2008).
Urban areas are identified as the centre of anthropogenic sources for NPs as compared with natural sources (Slezakova et al., 2013; Jeevanandam et al., 2018). Anthropogenic sources are classified as either intentional or unintentional. Unintentional sources include incomplete combustion (automobiles and industries), biomass burning, and the incineration of non-biodegradable wastes. Intentional sources consist of the use of pesticides and fertilisers producing a lot of NPs. Based on origin, NPs are also classified into primary and secondary sources. Primary sources include ore extraction, industrial emissions, energy production, and transport activities. Primary sources can be both stationary and mobile. Stationary sources comprise emissions from thermal power plants, chemical and metallurgical industries, and mining activities. Thermal power plants are one of the largest sources of NPs, especially in metropolises where the number of power plants are higher than in any other region like semi-urban/rural areas (Sonwani et al., 2021). The mobile sources are mostly motor vehicles, ships, submarines, aeroplanes, engines, and rockets launched into the extra-atmospheric space (Strambeanu et al., 2015).
Vehicular exhaust is a major source of NPs, released after the incomplete combustion of fuel. It is amongst the leading causes for air pollution due to the rapid rise in vehicular fleet (Walsh, 2011; Banerjee and Christian, 2018). The global vehicle population exceeded 1 billion in 2002 and has continued to climb steadily since then (Walsh, 2011). The major constituents of exhaust include carbon monoxide (CO), hydrocarbons (HCs), nitrogen oxides (NOx), particulate matter (PM), sulphur oxides (SOx), volatile organic compounds (VOCs), and their secondary by-products (Walsh, 2011; Banerjee and Christian, 2018). Most particles in vehicle exhaust are in the size range of 20–130 nm for diesel engines and 20–60 nm for petrol engines (Buzea et al., 2007). The sulphur content in fuel is an important parameter as it triggers the nucleation mode in PM formation (Chen et al., 2017). These emissions and ultrafine particles pose a serious risk to environmental health as they are known to produce carcinogenic effects (Banerjee and Christian, 2018).
The second major producer of anthropogenic NPs in the atmosphere are the construction and mining sectors. Surface mining and excavation through mine shafts can produce nanoparticles directly, while NPs' indirect production can occur through decantation, sedimentation, and floatation (Strambeanu et al., 2015). Meteorology and demolition methods also affect the concentration of NPs in the atmosphere. Respirable asbestos fibres, lead, glass, wood, and other toxic particles besides dust are found at the sites of demolition, and these particles can rise high up in the air, sometimes even forming dust clouds that can travel several kilometres, affecting nearby regions (Kumar et al., 2013). The inhalation of metal fumes (copper and zinc) and dust (nickel, chromium, and cobalt) causes pulmonary fibrosis and, in severe cases, cancer, which attributes to 15% of occupational hazards (Buzea et al., 2007).
The transboundary movement of air pollutants also play an important role in increasing the pollution load in a particular area, raising the NPs' level in the atmosphere. Air mass movement from desert and ocean area significantly affects the air quality in remote locations as it carries a variety of minerals and salts along with transported fine particles (Sonwani and Saxena, 2021). For example, African mineral dust is transported to the Caribbean basin, resulting in seasonal disturbance (Buzea and Pacheco, 2017). Likewise, volcanic eruptions in Iceland in 2011 and the forest fires in Indonesia's Sumatra in 1997 caused environmental havoc in Asia, especially in Singapore and Malaysia. The transportation of fine particle emissions from anthropogenic sources have also been reported by several authors across the world, particularly in the South Asian region (Abas et al., 2019; Cheong et al., 2019; Saxena et al., 2020). Crop residue burning (CRB) is also one of the main factors responsible for the air quality degradation in neighbouring states through transboundary movement, where a large fraction of emission from CRB affects the air quality in the Indo-Gangetic Plains (IGP) (Badarinath et al., 2009a; Saxena et al., 2021a). One of the most affected regions in the IGP is Delhi, which is often affected by CRB activities in the neighbouring states of Punjab and Haryana during the kharif season (Sarkar et al., 2018b; Saxena et al., 2021a). Interestingly, Delhi's air quality has also been affected in the Rabi season due to CRB in Haryana, reported in a recent study by Saxena et al. (2021b). At present, three-fourths of the crop residue, amounting to ~70–80 million tones, is disposed of by burning; therefore, it becomes a major source of NPs in the air and is well-known to aggravate respiratory disorders. The daily newspapers of northern India during October–November published reports on the incidences of thick clouds of smog formed due to crop residue burning by farmers, reducing the visibility that makes the Air Quality Index (AQI) severe (Badarinath et al., 2009b). In Asia, dust storms are more pronounced around the pre-monsoon period (March to early June) due to high temperatures and wind speed as compared with the other seasons. Such episodic events are largely responsible for contributing to NP pollution in downwind locations (Sarkar et al., 2018a). While in winters, low temperature, lower boundary layers, relatively stable atmosphere, and the transportation of air masses from the adjoining areas enhance the concentration of NPs in that particular area. Badarinath et al. (2009a), using multi-satellite data, reported that crop residue burning in the IGP affects the air quality over the south coast and the Arabian Sea coast of India every year with smoke columns as high as 2.5–3.5 kilometres (Pipal et al., 2014; Singh and Kaskaoutis, 2014; Ravindra et al., 2019).
Human exposure to NPs through inhalation produces various respiratory ailments. Ezzati et al. (2004) argued that indoor air pollution due to domestic fuel combustion acts as the eighth-largest risk factor for the global burden of disease. The indoor nanoparticle pollution in households is mainly due to the smoke from inefficient burning stoves and biomass fuels used most commonly in rural areas. Traditional practises, illiteracy, and unawareness are some of the key problems that inhibit the people of rural areas from shifting to modern chulas and ecofriendly fuels. The most popular fuel used for domestic cooking in rural regions is the unprocessed biomass solid fuel, known to cause 50 times more pollution than gas stoves (Ravindra et al., 2019). According to a study in rural Senegal, West Africa, out of 1,103 Senegalese women, only 1% used propane gas cylinders (combustion pollution-free) as their primary cooking equipment (Hooper et al., 2015). A 10-year long research carried out in 11 villages of Bangladesh reported 946 cardiopulmonary deaths, out of which 884 people used solid fuels and only 62 deaths were reported in gas-supplied households (Alam et al., 2012). A survey done in 15 homes in Xuanwei and Fuyuan, China, demonstrated experimentally how combustion-derived nanoparticles were generated using all kinds of stoves, while they were significantly reduced with the use of chimneys (Hosgood et al., 2012). An experimental study conducted in Uttar Pradesh, India, reported the average concentration of black carbon (an important fraction of fine particles) emissions was 6–20 μg/m3 and 3–1,070 μg/m3 for an urban and rural kitchen, respectively (Ravindra et al., 2019). Project Surya, carried out in India, advocated for the use of improved cookstoves widely proposed as a black carbon mitigation measure for Indian households and hence suggested a need for extensive testing and improved machinery for traditional cookstoves (Kar et al., 2012). Such eco-friendly sustainable techniques can reduce the concentration of NPs in the atmosphere.
Cigarette smoking is yet another common reason for exposure to NPs. One cigarette is known to emit almost 8.8 × 109 nanoparticles. Pertaining to the high particle concentrations and the rapid dilution in air, studies on identifying different types of particles present in cigarette smoke are limited. A study in Netherlands experimentally proved how a longer duration of puff and higher concentrations of tar values in one cigarette contribute directly to a large number of NPs (Williams et al., 2013). Adam et al. (2009) demonstrated the increase in NPs in low-ventilation conditions. They also studied how NP concentration was directly proportional to the number of puffs from a cigarette. Studies suggest an increased incidence of cancers, increased body mass index (BMI), and lower lung function in passive smokers than non-smokers (Gordon et al., 2002; Mohammad et al., 2013). Benzene, 1,3-butadiene, and particulate-bound 2,5-dimethylfuran (DMF) are human carcinogens that showed increased levels in non-smokers due to cigarette smoke exposure, thereby proving the adverse effects of passive smoking (Gordon et al., 2002). Occupational health hazards due to exposure to NPs are a common problem rising in developing and under-developed nations. Elevated cases of respiratory and cardiovascular disorders have been recorded in traffic officers due to their excessive exposure to vehicular exhaust. A collaborative study done by India and the United Kingdom in 2017, wherein various medical tests of 532 traffic officers (exposed to atmospheric NPs) were compared with 150 office workers (working in indoor environments), reported 50% increased cases of thick sputum, pain in joints, and shortness of breath in traffic officers (Bajaj et al., 2017). A similar study conducted in Kathmandu, Nepal, observed increased levels of inflammatory interleukins and tumour necrosis factor (TNF-α) in traffic officers (Shakya et al., 2019). NPs released from welding, flour dust, and diesel exhaust have long-term inflammatory and carcinogenic effects. Welding fumes contain large quantities of partially oxidised, ionised, and reactive metallic nanoparticles (Nasterlack et al., 2008). Another study suggested that the exposure to NPs can cause the production of reactive oxygen species (ROS), cytotoxicity, genotoxicity, and stimulation of ToxTracker reporter cell lines (Bajaj et al., 2017). Asbestos fibre (in all its commercial forms) is classified as one of the potential carcinogens by the International Agency of Research on Cancer (IARC) (Donaldson et al., 2011). Tungsten carbide-cobalt dust fumes (WC-Co particles) released during mining and drilling showed deposition in keratinocytes (epidermal cells; skin cell), DNA damage, inhibition of DNA repair, and alterations in gene expression and cell function after short-term exposure (Armstead and Li, 2016). Donaldson et al. (2011) determined the bio-persistence of different size fractions of occupational fibres in several human body systems. Longer slender fibres are usually more persistent, thereby causing various health hazards in our body. Figure 2 illustrates the clearance and deposition mechanisms of these fibres in our body and their potential impacts on humans. The detailed impact of nanoparticles from inhalation to disease site is described in Figure 3.
Figure 2. Demonstration of clearance and deposition mechanisms of NP fibres and with their potential impacts on human body (adopted and modified from Donaldson et al., 2011).
Authors provide detailed insights about the NP's inhalation, deposition, clearance, and impacts on the respiratory system and related human body systems. A summary of the effects of NPs on various organs and organ systems of the human body are mentioned in Table 1.
Human lungs have an internal surface area between 75 and 140 m2 and about 3,00,106 alveoli (Buzea et al., 2007). Lungs have a direct contact with the outside environment and hence acts as the main gateway for the entry of nanoparticles into the body. They are also considered important and the primary target site to study the effects of nanoparticles (Bakand et al., 2012; Pacurari et al., 2016).
Air is inhaled via nasal and oral routes that pass through the pharynx, progresses into the tracheobronchial tree, and finally reaches the alveoli. The transport of particles dependent on the structure of the respiratory airway, which can be described using the Weibel bifurcating tubes' model, classified into the conducting zone and respiratory zone, as already described in detail. Owing to their small size and high retention time, NPs can diffuse and accumulate in the alveolar region and some may pass through the alveolar epithelium and capillary endothelial cells to enter the cardiovascular system and other internal organs (Buzea et al., 2007; Qiao et al., 2015). Furthermore, electron microscopy proves that the penetration of nanoparticles can occur in both the outer and inner cellular compartments, deep inside the cytoplasm and karyoplasm of pulmonary epithelial and mesothelial cells of the lungs (Bakand et al., 2012).
The site of NP deposition in the respiratory tract depends on the particle's aerodynamic diameter (Ferreira et al., 2013). Many fibres with larger diameters are mostly deposited at the “saddle points” in the branching respiratory airways. Thus, they are unable to penetrate deep into the respiratory tract (Buzea et al., 2007). For smaller particles, deposition is mostly governed by Brownian movements. Energy filtering transmission electron microscopy (EFTEM) provides the evidence that aerosol NPs of 20 nm size, within 24 h of inhalation, bypass most of the clearance mechanisms and get deposited in the alveolar region of the lungs (Geiser, 2010).
According to the classical model developed by the International Commission on Radiological Protection (ICRP), NPs (≤20 nm diameter) have a high probability to reach the alveolar region (Kreyling et al., 2006; Ferreira et al., 2013). Thus, particles larger than 20 nm in diameter are restricted in the previous sections of the respiratory tract through mucociliary escalation and macrophage activity. Such activities help with the clearance of particles in the tract before they enter the pulmonary region.
The deposition is chiefly determined by particle size, the ventilatory parameters, and airway characteristics, whereas the clearance of particulate compounds, once deposited, is dependent on the physicochemical characteristics of the compound. During inhalation, larger particles are deposited at the extra-thoracic region (nose and larynx) and intrathoracic bifurcations due to impaction. These upper respiratory airways are covered with mucus (a complex fluid containing mucins [hydrogel-forming glycoproteins] that protect the body from environmental influences) (Moller et al., 2004; Kirch et al., 2012). This mucus layer traps the large particles, which are transported out by ciliary beating, removing the inhaled NPs, making it the dominant pathway of clearance from the regions of trachea, bronchus, and upper bronchioles (Moller et al., 2004; Geiser, 2010; Kirch et al., 2012). The outer viscoelastic mucus blanket has an underlying periciliary layer that provides conditions for an efficient ciliary beating cycle. The tips of the cilia reach the mucus blanket and accelerate this layer by a coordinated beating (Kirch et al., 2012). Upon entering the pharyngeal region, the mucus along with the NPs are finally swallowed and undergo further processing in the gastrointestinal tract (Henning et al., 2010). Smaller particles penetrate deep inside the lungs where air velocity decreases substantially and are deposited in the bronchioles and alveoli. This is the site where NPs undergo mucus transport and macrophages participate in the phagocytosis of biogenic NPs like viruses, protozoans, and bacteria (Moller et al., 2004). Macrophage-associated clearance is a much slower process as compared with mucociliary clearance (Henning et al., 2010). Most of these NPs translocate to the connective tissues and involve in the blood circulation, bringing the NPs in contact with the macrophage populations in the non-alveolar region. NPs are mostly cleared by interstitial and intravascular macrophages, as surface macrophages are relatively less effective in NP clearance (Geiser, 2010). After the inhalation and deposition of NPs on the surfactant layer with the underlying epithelial lining fluid, they come in contact with several proteins and biomolecules (including opsonins that enhance phagocytosis by marking an antigen), which makes NPs more susceptible to macrophages. It was observed that there was less phagocytosis in the absence of opsonins; moreover, rapid phagocytosis by alveolar macrophages was reported in the case of opsonin-directed migration towards the NPs (Kreyling et al., 2006).
A significant difference is observed in the behaviour and clearance of soluble and insoluble NPs in the lungs. Soluble NPs dissolve in the aqueous fluid and further goes into the cardiovascular systems. In contrast, the insoluble NPs (black carbon) are removed through mucociliary escalator or macrophage phagocytosis (Buzea et al., 2007). It has been reported that the insoluble NPs cause more tissue damage, inflammation, and lung tumours (Ferreira et al., 2013). The insoluble NPs accumulate more rapidly, exceeding the macrophage clearing capacity, and hence the defence mechanisms of the lungs fail to operate, resulting in lung injury (Buzea et al., 2007). It was also observed that acute lung inflammation is induced by the production of IL-1β and TGF-β1 for a shorter period in the bronchoalveolar lavage fluid. However, a longer exposure duration leads to collagen production, causing lung burden and potential pulmonary fibrosis (Lin et al., 2014).
Human health hazards due to lung burden is directly associated with the increasing cases of premature mortality, especially in developing nations. The determination of lung burden is dependent on the rate of particle deposition, rate of clearance, and residence time of the NPs (Buzea et al., 2007). Morphological observations and retrospective evidence indicate a slower particle clearance of insoluble NPs in large mammalian species (humans and primates) and a greater tendency for retained lung burden, which can translocate from the original alveolar deposition sites to the interstitial compartment of the respiratory system (Warheit, 2004). A long-term exposure to NPs causes high lung burden that leads to carcinogenesis in the lung tissues (Buzea et al., 2007). However, this level of exposure rarely happens in humans, suggesting lower chances of acquiring the malady (Donaldson and Poland, 2012).
Figure 4 explains the impact of toxicity generated through NP inhalation on human body. Human lungs constitute pseudostratified epithelium in the lung–blood stream barrier. The airways are composed of thin columnar epithelium, together with the bronchial epithelium (3–5 mm) and bronchiolar epithelium (0.5–1 mm), that all protected with mucous layer (Praphawatvet et al., 2020). The lung tissue consists of more than 40 different cells, so to study the aggregated effect of NPs on lungs, different cell models were made and tested. The exposure to NPs primarily affects the epithelial lining of the respiratory system, where A549 cell lines (derived from the human adenocarcinoma of the lung) are most often used in toxicity testing, and Calu-3, 16HBE14o-, and BEAS-2B cell lines are used as models for the bronchial barrier system (Fröhlich and Salar-Behzadi, 2014).
Figure 4. Impacts of nanoparticle generated toxicity to the human body (adopted from Li et al., 2010; Chakraborty et al., 2018).
The size of NPs is inversely proportional to the damage they cause in the respiratory tract, as smaller particles easily reach and get deposited in the distal parts of the respiratory tract (Donaldson et al., 2005).
Four main types of toxicological effects of nanoparticles are discussed as follows:
• Oxidative Stress
• Inflammation
• Genotoxicity
• Tumourigenicity
Oxidative stress is the prime consequence of nano-toxicity that occurs due to the imbalance between free radicals and antioxidants in the body in such a way that the extra free radicals with uneven number of electrons react inadvertently with other molecules to produce an imbalance in the respiratory system (Sharma et al., 2012).
Reactive Oxygen Species (ROS) Generation. The production of ROS is one of the important oxidative steps for creating toxicity in human lungs. The overproduction of ROS is caused by NPs' activity in the mitochondrial electron transport chain of the cell (Donaldson et al., 2010). Metallic NPs trigger Fenton-type reactions causing free-radical-mediated toxicity, and carbon NPs are known to affect mitochondrial reactions (Martin and Sarkar, 2017). The increased potential of hydrogen peroxide (H2O2) (due to special oxygen transfer properties of some metals) causes the generation of highly reactive hydroxyl radicals (OH·), and this process is called as Fenton's reaction (Li et al., 2008).
The extent of ROS production (produced by a particular NP) depends on the catalytic activity of surface groups. In a particle of 30 nm in size, about 10% of its molecules are expressed, whereas in the case particles of 10 and 3 nm in size, only around 20 and 50% molecules are expressed respectively (Hao and Chen, 2012).
The deposition of nanoparticles leads to the formation of molecular oxygen-dependent superoxide anion radicals (), H2O2, and hydroxyl radicals (OH·). These chemical species are proven to have cytotoxic responses in BEAS-2B bronchial epithelial cells. It was also reported that inflammatory phagocytes (macrophages and neutrophils) are also released from the respiratory system in response to NPs. Such inflammatory phagocytes induce oxidative outburst as a defence mechanism and ultimately raises the number of ROS in that particular region (Martin and Sarkar, 2017).
The Creation of Oxidative Stress. Oxidative stress is caused in the body either due to the overproduction of ROS or by the weakening of the processes of antioxidant defence. Another factor that determines the presence of oxidative stress in a region is the GSH/GSSG ratio, i.e., the cellular glutathione/glutathione disulphide ratio. Low values of this ratio indicate stress and toxicity in the tested region, where stress is created when lower quantities of glutathione are present and glutathione disulphide accumulates (Nel et al., 2006; Hao and Chen, 2012).
An in vitro study by scientists in Korea on BEAS-2B cell lines clearly indicated a rise in oxidative stress due to silica nanoparticle exposure as well as the induction of HO-1 (heme oxygenase-1—an antioxidant enzyme) via the Nrf-2–ERK MAP kinase signalling pathway, which suggests how oxidative stress disrupts the ROS balance of the cells (Huang et al., 2010). Meanwhile, in another study done in the United States, oxidative stress caused by ZnO NPs was directly linked to the increased calcium levels in cells, causing cellular dysfunction, impaired signal transduction, and other diseased states (Nho, 2020). Scientists have raised concerns over the inadequate results pertaining to NP exposure gained by in vitro models and have therefore adapted a more realistic approach by switching to in vivo models to study these xenobiotics (Ursini et al., 2014).
A study conducted in China in 2012 on the different organs of carp (Cyprinus carpio), wherein three different concentrations of 0.5, 5, and 50 mg/L of zinc oxide (ZnO) nanoparticles were tested for their effects on the concentrations of antioxidant enzymes (e.g., superoxide dismutase [SOD], catalase [CAT], etc.) before and after administration, indicated an increased mucus production and apoptosis of cells at higher concentrations and exposure time of ZnO NPs (Cho et al., 2012). TiO2 nanoparticles on a 90-day exposure increased the concentration of ROS as well as the level of lipid peroxidation and therefore decreased the antioxidant capacity of the lungs, leading to the creation of oxidative stress, which is the direct cause of inflammation and genotoxicity (Lu et al., 2014). Oxidative response is directly linked to inflammatory response as it is proven to cause chronic inflammation. The macrophages of the immune system are known to produce free radicals (the cause of oxidative stress) and pro-inflammatory hormones. A mitogen-activated protein kinase (MAPK) pathway activates and produces transcription factors that ultimately produce proteins responsible for inflammation, cell stress, cancer in the cell, etc.
Our immune system is divided into innate and adaptive immune systems. The innate immune system is the first line of defence against any foreign particle encountering on entering the body. If the innate immune system is not able to deactivate the foreign particle (antigen) on its own, it then induces the adaptive immune system, which is much more complex and effective. This activation is carried out by the dendritic cells in the body. Nanoparticles, being foreign particles, also activate dendritic cells, which in turn generate ROS, cytokines, and chemokines and activate naïve T-cells and various inflammasomes.
Determinants of Inflammation. NPs elicit an inflammatory response in the respiratory system as well as the other systems of the body (Medina et al., 2007; Clift et al., 2011; Padmanabhan and Kyriakides, 2015). A well-known determinant of an NP's ability to cause inflammation is the zeta potential (ξP) of the particle. ξP is the electric potential created when charged groups (present at the surface of a particle) interact with the suspension medium. Since the medium in human body is acidic, if more positively charged groups are present on the surface, more will be the solubility of that particle in the medium and hence there will be more interaction with macrophages, leading to inflammation (Schins, 2013). Magnesium and zinc oxide nanoparticles show high solubility in these solutions. A study reported that non-biodegradable NPs and cationic polymers induce even more inflammation than biodegradable and anionic makeshifts as titanium- and silicon-based NPs induce more inflammation than zinc (Nishi et al., 2020).
The presence of white blood cells (WBCs) in blood is another measure used to determine the presence of inflammation in the body. Inflammation is noticed based on the increase in WBC count above the normal range, which signals a decreasing immunity. When NPs enter via inhalation, their deposition in the lungs leads to the release of pro-inflammatory hormones, and they encounter alveolar macrophages (as they constitute the first line of immunity). Thus, the inactive macrophages become activated to stimulate the movement of various types of pro-inflammatory cytokines (IL-1 family, IL-6, IL-8, and i-CAM pro-inflammatory protein expression) to the affected site (Clift et al., 2011; Foldbjerg et al., 2011). An experiment was performed in the United Kingdom using eight different metal oxides in an in vivo rat model to investigate the effect of exposure on bronchoalveolar lavage fluid (BALF). This study reported that the cerium and nickel metal oxides are associated with an immediate neutrophilic cytotoxicity pattern, wherein the increased levels of IL-1β, MIP-2, and LDH indicate neutrophilic inflammation, and the same has been observed for copper and zinc by the end of 4 weeks of exposure (Magdolenova et al., 2014). Another study demonstrates that particle size is directly linked to inflammation, and this has been proven by an experiment performed on rats, wherein they were exposed to equal masses of titanium dioxide in two particle size ranges, showing more retention of ultrafine particles in the lung interstitium and the development of an increased inflammatory response (Peters et al., 2007). It was also reported that A549 cells (human alveolar carcinoma cells) were used to detect the change in cytokine secretion after treating the cell lines with TiO2 NPs and an increased release of the pro-inflammatory hormone interleukin-6 (Medina et al., 2007). Moreover, an in vivo experiment with nickel oxide NPs on rats' lungs found that chronic lung inflammations persist for long durations after exposure (Brown et al., 2001). A similar study done by Padmanabhan and Kyriakides (2015) showed that rats exposed to C13 ultrafine particles were deposited more in olfactory bulb on Day 7 than on Day 1, which clearly indicates how these particles get circulated in the body after inhalation.
The Adverse Effects of Inflammation. Inflammation results in the destruction of cilia in the respiratory tract and hence impairs the movement of mucus (mucus basically traps infectious agents and dirt) through cilia. Inflammation also leads to epithelial injury by breaking the epithelial barrier between the lung surface and blood stream, leading to clotting, decreased lung function, the impaired circulation of blood and oxygen in the body. Pneumonitis and the inflammation of lung tissue are the symptoms of lung cancer, pulmonary fibrosis, chronic obstructive pulmonary disease (COPD), asthma, and cystic fibrosis. Various types of pulmonary fibrosis are the main causes of death in patients diagnosed with chronic lung diseases (Chen et al., 2006). This has been proved in a study done on rats, wherein they were exposed to a long multi-walled carbon nanotube for 30 days, which showed the presence of irreversible granuloma, considered as chronic inflammation in lungs (Lu et al., 2014). Inflammation also results in blood clotting, which activates thrombosis cascade at different locations, ultimately leading to problems in the cardiovascular system, upsetting the cardiac rhythm and increasing the chances of cardiac arrest in the body (Donaldson et al., 2005).
Genotoxicity can be induced either by the direct interaction of NPs with DNA or indirectly because of oxidative stress or inflammation. Based on different studies and consequent effects, genotoxicity can be divided into primary and secondary genotoxicity. Primary genotoxicity occurs when ultrafine particles enter the nucleus and cause modifications in DNA. Another primary indirect mechanism is related to the DNA repair cascade. Secondary genotoxicity is caused by oxidative stress and inflammation induced by NPs (Zhu et al., 2013; Magdolenova et al., 2014). Figure 5 shows silica NPs' deposition in the lungs (via p53 signalling) and the related genotoxicity involved in the tumour formation in rats.
Figure 5. Diagrammatic depiction of a study where Silica nanoparticles were deposited in lungs via p53 signalling and then tested in-vitro on lung cell models as well in-vivo on live rats induced aberrant p53 signalling and malignant tumours, respectively (adopted from Stueckle et al., 2017).
Direct Primary Genotoxicity
The oxidation of DNA results in mutagenic modifications such as the hydroxylation of adenine and guanine, thereby leading to the formation of DNA adducts (Donaldson et al., 2005; Berube et al., 2007). DNA adducts are a result of the covalent modifications in DNA in the presence of certain carcinogens. Particulate carcinogens such as asbestos, crystalline silica, etc., have the ability to directly enter the cellular membranes and nucleus. They then interact/disrupt the process and functionality of the different components of the mitotic spindle and ergo produces dysfunctionalities (Magdolenova et al., 2014). These foreign NPs can disrupt all cellular processes including mitosis, the replication of DNA, and the transcription of DNA into mRNA. A study reported that aluminium NPs induced structural damage and DNA instability, besides other mechanisms that explained the DNA damage (including single-strand breaks and double-strand breaks), DNA deletions, and genomic instability in the form of an increase in 8-hydroxy-20 -deoxyguanosine levels (Donaldson et al., 2013; Chakraborty et al., 2018). Cobalt-chromium nanoparticles significantly increased single- and double-strand breaks and the chromosomal aberrations in mice on exposure, consequently affecting the brain cells in the resulting offspring (Clift et al., 2011).
Indirect Primary Genotoxicity
In the indirect method, NPs attach to the nuclear proteins responsible for DNA repair mechanisms, disrupting their functioning and hence indirectly favouring slip-ups in the nuclear processes. Topoisomerases are the enzymes responsible for carrying out this repair cascade. An experiment showed that Carbon-60 fullerenes can bind to human topoisomerase-II alpha in the ATP-binding domain and therefore disturb its enzymatic activity (Donaldson et al., 2013; Magdolenova et al., 2014).
Secondary genotoxicity refers to the errors created in the structure and function of nuclear components due to the increased ROS production and inflammation, ultimately leading to disrupted DNA sequences. Nanoparticles trigger ROS production in phagocytes (macrophages, neutrophils, etc.), which induces DNA damage and mutagenesis in the neighbouring cells (Donaldson et al., 2013; Magdolenova et al., 2014). It has been reported that in any mechanism, silica triggers the production of ROS leading to the activation of NF-kβ (necrosis factor) and AP-1 (primarily in gene expression), causing an increase in the quantities of growth factors and oncogenes that causes mutations and ultimately cancer (Hong et al., 2017). Exposure to diesel exhaust particles produces inflammation, leading to epigenetic changes that include impairment in gene expression, the formation of adducts, cell proliferation, and interruptions in DNA repair (Shi et al., 1998). In another experiment, researchers demonstrated how the A549 cell line (present in alveoli) is more susceptible to DNA damage than the BEAS-2B cell line (present in bronchi) using FPG comet assay (Medina et al., 2007). A study undertaken in Denmark on the A549 cell lines clearly demonstrates that silver NPs induce oxidative stress correlated to cytotoxicity and genotoxicity (Berube et al., 2007).
The MAPK/ERK pathway (also known as the Ras-Raf-MEK-ERK pathway) is a chain of proteins in the cell that communicates a signal from a receptor on the cell's surface to the DNA in the cell nucleus. The signal starts when a signalling molecule binds to the receptor on the cell surface called epidermal growth factor receptor (EGFR). In normal state, extracellular ligands such as epidermal growth factors bind to this receptor and phosphorylates activates it, signalling a cascade of docking proteins that ultimately result in the making of mRNA, which gets encoded to form various proteins.
In this case, ROS and sometimes direct NPs interact with these receptors, and abnormal proteins could either be formed or not due to the changes in the DNA code. When one of the proteins in the pathway mutates, it can become stuck in the “on” or “off” position, which is a fundamental step in the development of many cancers. The entry of NPs plays a major role in increasing the levels of 8-hydroxy-2′-deoxyguanosine (8-OHdG) in the body. 8-OHdG can give rise to G-to-T transversion mutations in the key genes known to be involved in the development of cancer, hence giving rise to tumours (Guo et al., 2017). Thus, an excessive level of 8-OHdG is directly proportional to the occurrence of carcinogenicity that weakens the immunity. A study observed the levels of 8-OHdG in the urine and white blood cells of 130 workers handling indium tin oxide who were easily exposed to metal oxide NPs (Falcone et al., 2018). Other pathways include p53 inactivation and caveolin-1 overexpression. p53 inactivation occurs when NPs decrease the phosphate levels (required to activate proteins) in cells and therefore increase the chances of tumour formation (Stueckle et al., 2017). Likewise, there are many proteins that are found in high concentrations in the body in the presence of a tumour, and such high levels of proteins are often used to test the presence of neoplasm (the abnormal growth of cells). Caveolin-1 protein's overexpression is associated with carcinogenesis and metastasis. A study reported the inhalation exposure to Titanium oxide NPs on mice, wherein an increased level of squamous cell carcinoma antigen (SCC-Ag) (tumour marker) was observed in high concentrations due to the formation of tumours (Shi et al., 1998).
Tumour suppressor genes in the body are responsible for expressing proteins that prevent the uncontrolled growth of cells forming tumours and hence the decrease chances of genomic mutability. Mutated tumour suppressor genes due to the genotoxicity caused by NPs aren't able to carry out their function, eventually leading to the loss of growth regulation (Luanpitpong et al., 2010). Liou et al. (2017) identified the appearance of cancer stem-like cells on chronic exposure to carbon NPs. Ceria NPs have an ability to generate ROS and cause DNA damage, leading to alveolar papillary neoplasm and carcinoma. Carcinoma also depends on particle size, bio-persistence, and the co-morbidity in an individual. Other recent in vivo studies confirm that the iron oxide NPs as an important cause of hyperplasia and fibrosis of the lungs (Manke et al., 2013). Thus, an excessive increase in fibrosis leads to the death of an individual. Chromium, nickel, and iron oxides released with NPs from welding fumes act as major carcinogens for the human body that significantly increase the chances of tumour formation (Chen et al., 2006).
As discussed previously, the respiratory system is one of the most important pathways for the entry of airborne pollutants into the body and the distribution of NPs to the other biological systems of the body. The retained NPs in the lungs come in contact with the alveoli–blood barrier (present between the alveoli of the lungs and the transporting vessels of the circulatory system), which helps in the exchange of gases stored by alveoli to the entire body and vice versa by the process of diffusion of air particles. The retained NPs seep into these vessels through endocytosis in the alveolar epithelial cells and get transported to the other organs via blood. Endocytosis is a process by which a cell transports particles in and out of the cell by the formation of vacuoles. The second mechanism involves in the diffusion of these ultrafine particles into the olfactory bulbs of neurons via the nasal epithelial barrier for reaching the central nervous system of the body. This system is spread throughout the body and therefore the NPs travel and get deposited in distant places of the different systems of the body (Iversen et al., 2011; Elsaesser and Howard, 2012). Figure 6 shows the impact of exposure to NPs on the different systems of the human body.
The digestive system also called as the gastrointestinal tract consists mainly of the oesophagus, stomach, and intestines. NP exposure to the gut mostly occurs either through water or food intake. The presence of silver NPs increases the interleukin-8 levels, directly linked to inflammation and increased mucus production (Georgantzopoulou et al., 2015). Depending on their size, some NPs escape the junctions between the intestinal epithelial cells to enter blood vessels, perturbing the mucus and epithelial cell layers. Furthermore, they finally accumulate in the lamina propria of the intestines where they disrupt the function of goblet cells (Zhang et al., 2014). If there are already underlying conditions such as Crohn's disease or ulcerative colitis in the individual, then the rate of NP accumulation is even higher, leading to inflammation in the intestinal areas (Lomer et al., 2004; Jones et al., 2015). Consequently, the toxicity levels get increased and will lead to colon cancer and other carcinomas.
NPs can enter our body via inhalation (intranasal or intra-tracheal) or by oral exposure to the gastro-intestinal tract, which subsequently reaches the circulatory system. These can cause significant alterations in the normal functioning of the system. The increase in blood pressure, decrease in heart rate, and altered vascular tone and dysfunction are some of the initial effects of NPs on the cardiovascular system (Yu et al., 2016). Depending upon the physical properties, concentration, and retention time of NPs, they can have angiogenic/antiangiogenic, vasodilation/vasoconstriction, pro-oxidant/antioxidant, cytotoxic, apoptotic, and phagocytic effects on our body (Gonzalez et al., 2016). Individuals with pre-existing cardiovascular diseases are more susceptible to these changes. Such persons are more prone to sudden cardiac arrests, heart attacks, and blood clotting due to the assimilation of NPs in the blood stream. Epidemiological studies have revealed evidence of both the acute and chronic effects of NPs (Donaldson et al., 2013). An increased ROS generation because of nanoparticles has been observed as the main cause for these maladies due to the increased oxidative stress (Miller et al., 2012).
Silver NPs that are currently used in pacemakers, drugs, and coupled antibodies have a dual and opposite effect on blood composition, angiogenesis (vessel formation), and the permeability of membranes (Gonzalez et al., 2016). Long-term exposure to TiO2 NPs can lead to their accumulation in the heart, causing sparse cardiac muscle fibres, inflammation, cellular necrosis, and cardiac biochemical dysfunction. A study on SiO2 NPs in old rats suggested increased Fbg concentrations and blood viscosity along with myocardial ischemic damage and atrioventricular blockage (Yu et al., 2016). Experiments performed on rodents by Mills and Miller (2011) support the aforementioned results and also provide evidence to endothelial cell dysfunction, the inhibition of NO pathways, and the enhanced responsiveness to vasoconstrictors.
The kidney contains a network of blood capillaries in each nephron that philtres the toxins from blood; therefore, it is quite susceptible to xenobiotics and the bioaccumulation of toxins (Pujalté et al., 2011). NPs, after glomerular filtration, tend to concentrate in the proximal convoluted tubules (PCT), which can lead to internalisation via tubular cells through endocytosis. A study on TH1 cells exposed to inorganic NPs showed not only the acceleration of NP-induced nephrotoxicity but also the DNA damage in these cells (Sramkova et al., 2019). Similar effects were observed by another study when tubular cells in mice were exposed to copper NPs (Pujalté et al., 2011). This will further lead to genetic disorders and chromosomal aberrations in the genetic material.
Wang et al. (2009) observed that the smaller the particle, the greater its toxicity. This study on HEK293 cells (cultured human embryonic kidney cells) demonstrated that kidney cells underwent shrinkage and nuclear condensation, which are signs of apoptosis on exposure to SiO2 nanoparticles at dosage levels of 20–100 μg/ml. ROS production in HEK293 cells and oxidative stress induction also indicate the nephrotoxic potential by nanoparticles. Impaired nephrotoxic potential causes dysfunction in the elasticity of nephrons and may cause cerebral epilepsy. The cytotoxicity of NPs was also evaluated in the IP15 (glomerular mesangial) and HK-2 (epithelial proximal) cell lines (Pujalté et al., 2011). The study observed that ZnO and CdS nanoparticles exerted cytotoxic effects on glomerular and tubular human renal cells. These effects were correlated with metal composition, particle size, and metal solubility (Pujalté et al., 2011).
Neurons together with neuroglial cells constitute the nervous tissue making up the nervous system. NPs can reach the central nervous system (CNS) via different routes, most commonly by inhalation that can reach the brain through the upper respiratory tract to the olfactory bulb to the trigeminal nerve to the trigeminal nucleus and thalamus to the blood-brain barrier and ultimately to different parts of the brain (Haase et al., 2012; Feng et al., 2015). NPs produce toxicity effects on neural cells by accumulating in different regions of the brain and affecting the expression of genes that helps in the development and function of CNS (Yang et al., 2010). The malfunctioning of CNS can show symptoms like depression, lack of concentration, and even mental retardation. ZnO NPs induce significant cytotoxicity, including a decrease in viability, apoptosis, cell cycle alterations, and different kinds of genetic damage such as oxidative DNA damage (Valdiglesias et al., 2013). Studies show induced inflammation in rats' brain due to manganese oxide inhalation. Silver NPs cause oxidative stress and the upregulation of oxidative stress-related genes in the cortex and hippocampus of mice (Haase et al., 2012; Feng et al., 2015).
In the past few decades, there has been a substantial rise in disorders of the immune system. One of the reasons associated with it is the exposure of workers and the general population to contaminants that exert adverse effects on endocrine-disrupting chemicals (EDCs) (e.g., CdTe quantum dots), which play a prime role in altering the hormonal and homeostatic systems (Iavicoli et al., 2013; Lu et al., 2013). A study shows how 150 μg/kg of iron oxide NPs administered in male rats increased the T3 thyroid hormone and decreased the thyroid-stimulating hormone, thus creating a net imbalance (Jiang et al., 2019). Commencing with overstimulation, palladium NPs have shown to gradually act on hormone receptors, blocking the signal cascades (Leso et al., 2018). Some studies have observed that diabetic animals are more prone to hormonal disbalance that non-diabetic ones due to NP exposure (Li et al., 2009; Lu et al., 2013).
One of the main reasons for the recent spike in infertility cases has been attributed to the accumulation of NPs. An experimental study on high-fat diet rats shows that administering silica NPs (which are common at workplaces) decreased sperm concentration and motility rates and increased the abnormality rates of sperm (Zhang et al., 2020). TiO2 NPs exhibit genotoxic effects as they cross the blood–testis barrier and causes inflammation and cytotoxicity, thus a significant loss of sperm DNA integrity was observed (Santonastaso et al., 2019). NPs affect the reproductive system by inducing cytotoxicity in ovarian cells, thereby disrupting the process of oogenesis and eventually causing the overproduction of ROS, apoptosis, and sex-hormone imbalance in the body (Iavicoli et al., 2013). In a study, an animal administered with MoO3 showed a significant decrease in the right ovary's weight and uterine weight, which ultimately led to an adverse effect on the reproduction process (Asadi et al., 2019). Hence, NPs are significantly responsible for decreasing birth rates and causing abnormalities in offsprings. In urban areas, such cases are commonly found in females who are exposed to air pollutants, particularly particulate matter (especially NPs), due to their daily lifestyle.
Specific models have been devised to understand the structure and effects of NPs on individual organs. The Weibel bifurcating tubes' model with 23 bifurcation units describes the branching pattern of lungs, i.e., the 23 levels of bifurcations—G0–G23. The first 16 bifurcations (G0–G16) comprise the conducting zone, while the rest (G17–G23) comprise the respiratory zone involved in the exchange of gases. This model helps in understanding the structure of the airway path (Qiao et al., 2015), and models similar to the organ have been prepared to study the effects of NPs on other body systems. To comprehend and quantify the rates and mechanism of particle deposition, clearing, and impact, the models were divided into experimental and computational methods (Qiao et al., 2015). The experimental methods include in vivo and in vitro experiments on a model organism exposed to different doses of NPs to determine deposition, clearing, and health impacts (Ji and Yu, 2012; Qiao et al., 2015).
A computational method like computational fluid dynamics (CFD) is primarily used an effective tool in predicting the airflow, particle transport, deposition, and the particle number concentration in fine particles. It is also used in the field of NP instrumentation, evolution of NP dynamics in different environment (human respiratory tract, workplaces, aerosol transport/delivery system, energy systems, etc.), and NP synthesis (Qiao et al., 2015).
Respiratory tract dosimetry is a biologically based, mechanistic method that is a useful experimental technique to estimate the exposure concentrations of an inhaled substance in a model organism (Kuempel et al., 2006) that can be extrapolated to human equivalent exposure levels by adjusting the lung mass and lung surface area (Kuempel et al., 2006; Ji and Yu, 2012). The multiple-path particle dosimetry model (MPPD) developed by the Chemical Industry Institute of Toxicology (CIIT, currently known as the Hamner Institutes for Health Sciences) and the Dutch National Institute for Public Health and the Environment (RIVM) is used in the assessment of deposition and clearance of course to ultrafine particles (Ji and Yu, 2012). MPPD provides a quick, inexpensive, and efficient means to assess the internal estimates of tissue dose. This model is an efficient tool to determine the percentage change in the particulate load of respirable deposits in the human respiratory system. MPPD estimates particle deposition in the tracheobronchial region where most of the respirable particulate deposition occurs as compared with the pulmonary region (Goel et al., 2021). As the respiratory tract is divided into various regions based on anatomical function, sub-models of ventilation and aerosol transport are constructed (Asgharian et al., 2014). Settings like flow characteristics, orientation, inspiratory fraction, and parameters including multiple respiratory volumes can be altered to meet the needs of the experiment (Manigrasso et al., 2019). Another popular in vitro model is the air–blood barrier (ABB) models that elucidates the biological mechanisms related to the potential effects of inhaled nanoparticles. In this model, the effect of NPs on the cell lines of respiratory epithelium, endothelium, and monocyte cells are studied (Bengalli et al., 2017).
The CFD method is mostly used to highlight the impact of several factors including upstream flow, inlet profiles, and turbulent enhanced dispersion (Longest and Vinchurkar, 2007). Like MPPD, the airway is divided into subdivisions called tiny meshes that can help quantify the deposition of particles (Longest and Vinchurkar, 2007; Garcia and Kimbell, 2009; Qiao et al., 2015). Two approaches have been used to investigate the deposition study. First is the Eulerian approach that uses a constant laminar flow rate with non-stop fluid process, dilute particle phase (treated as interpenetrating fields), Brownian motion (depending on the concentration gradient), and a diffusion coefficient (depending on particle size). This model is used to calculate the deposition efficiency of various particle sizes. Deposition efficiency (DE) can be calculated using the following equation:
where ,
Lpipe is the pipe length, Uinlet is the inlet velocity, and R is the pipe radius. This approach neglects particle inertia and is effective for studying a large number of particles (Inthavong et al., 2011). The assumption of laminar flow is supported by the low Reynolds number (Re) (Longest and Vinchurkar, 2007; Garcia and Kimbell, 2009). The second approach is called the Lagrangian approach that considers all the above variables along with particle inertia. This is effective in studying individual particle motion. The trajectories of each particle are traced by integrating a force balance equation on the individual particles:
Here, Fg is the gravity term; FD is the drag force per unit particle mass; FB is the amplitude of the Brownian force component; FL is the lift due to shear; and FT is the thermophoretic force. In comparison, the Eulerian model is less computationally demanding due to the single convection–diffusion equation that controls the fluid–particle interrelationship (Inthavong et al., 2011).
Apart from the aforementioned models, the World Health Organisation (WHO) recently introduced new models for the assessment of air quality. Air quality models like the AirQ model and the AirQ 2.2 software are currently in use (Conti et al., 2017). Air quality models use mathematical and numerical methods to simulate the physical and chemical processes caused by air pollutants that trigger their dispersion and chemical transformation in the atmosphere. The purpose of these models is to relate the effects of emission sources with the pollutant concentrations and to cheque whether they are crossing the standard limits or not.
The health effects of nanoparticles are well-known, and their exposure can cause serious respiratory and cardiovascular diseases. Epidemiological and toxicological studies have mentioned that nanoparticles are much toxic than coarser particles due to their ultrafine size, chemical reactivity, and longer residence time in the atmosphere. It has also be observed that as particles decrease in size, their ability to penetrate deep inside the lungs increases. The source of nanoparticles varies from anthropogenic activities (vehicular emission, industrial emission, welding fume, cigarette smoke, etc.) to natural activities (forest fire, dust storm, volcanic eruption, etc.). The transport of NP in the lungs is explained with the Weibel bifurcating tubes' model, classified into the conducting zone and respiratory zone. Owing to the ultrafine size and high retention time, nanoparticles diffuse and accumulate in the alveolar region, and some pass through the alveolar epithelium and capillary endothelial cells to enter the cardiovascular system and other internal organs. Different types of clearance mechanisms are exhibited by the human body to counter the effect of NP exposure and protect the body before the onset of the disease. Mucociliary escalation clears the upper respiratory tract, while macrophages with certain proteins help ingest the deep deposited material. Regardless, insoluble NPs get bioaccumulated in the respiratory zone over time, causing lung burden. Such NPs are attacked by phagocytes as a part of the immune response. The exposure to NPs cause the generation of ROS, resulting in cytotoxicity that leads to genotoxicity and tumourigenesis. The overproduction of ROS and the weakening of antioxidant defence system cause oxidative stress, known to trigger the release of more pro-inflammatory hormones that lead to inflammation as well as acute and chronic lung diseases. Thus, NPs cause damage in single-stranded and double-stranded DNA or deletions of its parts and induce mutations in the genetic material, generating neoplasms and carcinomas in the lungs. Besides the harm caused in the lungs, NPs also cause damage in the other body systems including the cardiovascular and gastrointestinal system and lead to cardiac arrest and ulcer, respectively. Comorbid individuals are always at a higher risk of several health complications due to NP accumulation.
This is the first comprehensive review about NPs' source, exposure, and impact on different human body systems, focusing on the respiratory system. The review provides detailed insights about the complex mechanism of NP transportation, clearance, and deposition in lungs that cause lung burden after long-term NP exposure. It offers a detailed overview of the influence of NP exposure in causing toxicological, genotoxic, and tumourigenic effects on the human body. It also mentions the experimental and computational respiratory models, which can be important tools to understand NP's transportation, clearance mechanism, and deposition patterns in human body.
SSo wrote the full text of manuscript. SSo and PS involved in conceptualization and design of the work. SM and JA contributed in acquisition, analysis and interpretation of data for the work, and helped in manuscript writing. SSu involved in acquisition and interpretation of data and critically reviewed the manuscript. DR, NM, and TV performed the whole formatting and editing of manuscript. PS coordinated and supervised the entire team. All authors contributed to the article and approved the submitted version.
The authors declare that the research was conducted in the absence of any commercial or financial relationships that could be construed as a potential conflict of interest.
All claims expressed in this article are solely those of the authors and do not necessarily represent those of their affiliated organizations, or those of the publisher, the editors and the reviewers. Any product that may be evaluated in this article, or claim that may be made by its manufacturer, is not guaranteed or endorsed by the publisher.
Authors express their sincere thanks to the reviewers for providing constructive comments and suggestions.
Abas, N., Saleem, M. S., Kalair, E., and Khan, N. (2019). Cooperative control of regional transboundary air pollutants. Environ. Syst. Res. 8, 1–14. doi: 10.1186/s40068-019-0138-0
Adam, T., McAughey, J., McGrath, C., Mocker, C., and Zimmermann, R. (2009). Simultaneous on-line size and chemical analysis of gas phase and particulate phase of cigarette mainstream smoke. Anal. Bioanal. Chem. 394, 1193–1203. doi: 10.1007/s00216-009-2784-y
Alam, D. S., Chowdhury, M. A. H., Siddiquee, A. T., Ahmed, S., Hossain, M. D., Pervin, S., et al. (2012). Adult cardiopulmonary mortality and indoor air pollution: a 10-year retrospective cohort study in a low-income rural setting. Glob. Heart. 7, 215–221. doi: 10.1016/j.gheart.2012.06.008
Armstead, A. L., and Li, B. (2016). Nanotoxicity: emerging concerns regarding nanomaterial safety and occupational hard metal (WC-Co) nanoparticle exposure. Int. J. Nanomed. 11:6421. doi: 10.2147/IJN.S121238
Asadi, F., Sadeghzadeh, M., Jalilvand, A., Nedaei, K., Asadi, Y., and Heidari, A. (2019). Effect of molybdenum trioxide nanoparticles on ovary function in female rats. J. Adv. Med. Biomed. Res. 27, 48–53. doi: 10.30699/jambs.27.121.48
Asgharian, B., Price, O. T., Oldham, M., Chen, L. C., Saunders, E. L., Gordon, T., et al. (2014). Computational modeling of nanoscale and microscale particle deposition, retention and dosimetry in the mouse respiratory tract. Inhal. Toxicol. 26, 829–842. doi: 10.3109/08958378.2014.935535
Badarinath, K. V. S., Kharol, S. K., and Sharma, A. R. (2009a). Long-range transport of aerosols from agriculture crop residue burning in Indo-Gangetic Plains—a study using LIDAR, ground measurements and satellite data. J. Atmos. Solar Terrestr. Phys. 71, 112–120. doi: 10.1016/j.jastp.2008.09.035
Badarinath, K. V. S., Kharol, S. K., Sharma, A. R., and Prasad, V. K. (2009b). Analysis of aerosol and carbon monoxide characteristics over Arabian Sea during crop residue burning period in the Indo-Gangetic Plains using multi-satellite remote sensing datasets. J. Atmos. Solar Terrestr. Phys. 71, 1267–1276. doi: 10.1016/j.jastp.2009.04.004
Bajaj, N., Sharma, T., Suneja, D., Jain, S., and Kumar, P. (2017). Determinants of respiratory and cardiovascular health effects in traffic policemen: A perception-based comparative analysis. J. Transport Health 4, 30–39. doi: 10.1016/j.jth.2016.12.003
Bakand, S., Hayes, A., and Dechsakulthorn, F. (2012). Nanoparticles: a review of particle toxicology following inhalation exposure. Inhal. Toxicol. 24, 125–135. doi: 10.3109/08958378.2010.642021
Bakshi, S., He, Z. L., and Harris, W. G. (2015). Natural nanoparticles: implications for environment and human health. Crit. Rev. Environ. Sci. Technol. 45, 861–904. doi: 10.1080/10643389.2014.921975
Banerjee, T., and Christian, R. A. (2018). A review on nanoparticle dispersion from vehicular exhaust: assessment of Indian urban environment. Atmos. Pollut. Res. 9, 342–357. doi: 10.1016/j.apr.2017.10.009
Bengalli, R., Gualtieri, M., Capasso, L., Urani, C., and Camatini, M. (2017). Impact of zinc oxide nanoparticles on an in vitro model of the human air-blood barrier. Toxicol. Lett. 279, 22–32. doi: 10.1016/j.toxlet.2017.07.877
Berube, K. A., Balharry, D., Sexton, K. J., Koshy, L., and Jones, T. P. (2007). Combustion-derived nanoparticles: mechanisms of pulmonary toxicity. Clin. Exp. Pharmacol. Physiol. 34, 1044–1050. doi: 10.1111/j.1440-1681.2007.04733.x
Brown, D. M., Wilson, M. R., MacNee, W., Stone, V., and Donaldson, K. (2001). Size-dependent proinflammatory effects of ultrafine polystyrene particles: a role for surface area and oxidative stress in the enhanced activity of ultrafines. Toxicol. Appl. Pharmacol. 175, 191–199. doi: 10.1006/taap.2001.9240
Buseck, P. R., and Adachi, K. (2008). Nanoparticles in the atmosphere. Elements 4, 389–394. doi: 10.2113/gselements.4.6.389
Buzea, C., and Pacheco, I. (2017). “Nanomaterial and nanoparticle: origin and activity,” in Nanoscience and Plant-Soil Systems. Soil Biology, Vol. 48, eds M. Ghorbanpour, K. Manika, and A. Varma (Cham: Springer). doi: 10.1007/978-3-319-46835-8_3
Buzea, C., Pacheco, I. I., and Robbie, K. (2007). Nanomaterials and nanoparticles: sources and toxicity. Biointerphases 2:MR17-MR71. doi: 10.1116/1.2815690
Chakraborty, A., Ghosh, S., Chakraborty, R., Chatterjee, S. M., and Hopper, W. (2018). Molecular mechanism of nanotoxicity-a critical review. Int. J. Curr. Biotechnol. 6, 1–12.
Chen, H. W., Su, S. F., Chien, C. T., Lin, W. H., Yu, S. L., Chou, C. C., et al. (2006). Titanium dioxide nanoparticles induce emphysema-like lung injury in mice. FASEB J. 20, 2393–2395. doi: 10.1096/fj.06-6485fje
Chen, L., Liang, Z., Liu, H., Ding, S., and Li, Y. (2017). Sensitivity analysis of fuel types and operational parameters on the particulate matter emissions from an aviation piston engine burning heavy fuels. Fuel 202, 520–528. doi: 10.1016/j.fuel.2017.04.052
Chen, R., Hu, B., Liu, Y., Xu, J., Yang, G., Xu, D., et al. (2016). Beyond PM2. 5: the role of ultrafine particles on adverse health effects of air pollution. Biochim. Biophys. Acta Gen. Subj. 1860, 2844–2855. doi: 10.1016/j.bbagen.2016.03.019
Cheong, K. H., Ngiam, N. J., Morgan, G. G., Pek, P. P., Tan, B. Y. Q., Lai, J. W., et al. (2019). Acute health impacts of the Southeast Asian transboundary haze problem—a review. Int. J. Environ. Res. Public Health 16:3286. doi: 10.3390/ijerph16183286
Cho, W. S., Duffin, R., Thielbeer, F., Bradley, M., Megson, I. L., MacNee, W., et al. (2012). Zeta potential and solubility to toxic ions as mechanisms of lung inflammation caused by metal/metal oxide nanoparticles. Toxicol. Sci. 126, 469–477. doi: 10.1093/toxsci/kfs006
Clift, M. J., Gehr, P., and Rothen-Rutishauser, B. (2011). Nanotoxicology: a perspective and discussion of whether or not in vitro testing is a valid alternative. Arch. Toxicol. 85, 723–731. doi: 10.1007/s00204-010-0560-6
Conti, G. O., Heibati, B., Kloog, I., Fiore, M., and Ferrante, M. (2017). A review of AirQ Models and their applications for forecasting the air pollution health outcomes. Environ. Sci. Pollut. Res. 24, 6426–6445. doi: 10.1007/s11356-016-8180-1
Donaldson, K., Duffin, R., Langrish, J. P., Miller, M. R., Mills, N. L., Poland, C. A., et al. (2013). Nanoparticles and the cardiovascular system: a critical review. Nanomedicine 8, 403–423. doi: 10.2217/nnm.13.16
Donaldson, K., Murphy, F., Schinwald, A., Duffin, R., and Poland, C. A. (2011). Identifying the pulmonary hazard of high aspect ratio nanoparticles to enable their safety-by-design. Nanomedicine 6, 143–156. doi: 10.2217/nnm.10.139
Donaldson, K., Newby, D. E., MacNee, W., Duffin, R., Lucking, A. J., and Mills, N. L. (2007). “Pulmonary and cardiovascular effects of nanoparticles. characterization, dosing and health effects,” in Nanotoxicology: Characterization, Dosing and Health Effects, eds N. A. Monteiro-Riviere and C. L. Tran (CRC Press), 267–295. doi: 10.3109/9781420045154-18
Donaldson, K., and Poland, C. A. (2012). Inhaled nanoparticles and lung cancer-what we can learn from conventional particle toxicology. Swiss Med. Wkly. 142:w13547. doi: 10.4414/smw.2012.13547
Donaldson, K., Poland, C. A., and Schins, R. P. (2010). Possible genotoxic mechanisms of nanoparticles: criteria for improved test strategies. Nanotoxicology 4, 414–420. doi: 10.3109/17435390.2010.482751
Donaldson, K., Tran, L., Jimenez, L. A., Duffin, R., Newby, D. E., Mills, N., et al. (2005). Combustion-derived nanoparticles: a review of their toxicology following inhalation exposure. Part. Fibre Toxicol. 2, 1–14. doi: 10.1186/1743-8977-2-10
Elsaesser, A., and Howard, C. V. (2012). Toxicology of nanoparticles. Adv. Drug Deliv. Rev. 64, 129–137. doi: 10.1016/j.addr.2011.09.001
Engling, G., and Gelencsér, A. (2010). Atmospheric brown clouds: from local air pollution to climate change. Elements 6, 223–228. doi: 10.2113/gselements.6.4.223
Ermolin, M. S., Fedotov, P. S., Malik, N. A., and Karandashev, V. K. (2018). Nanoparticles of volcanic ash as a carrier for toxic elements on the global scale. Chemosphere 200, 16–22. doi: 10.1016/j.chemosphere.2018.02.089
Ezzati, M., Lopez, A. D., Rodgers, A., and Murray, C. J. (2004). Comparative Quantification of Health Risks. Global and Regional Burden of Disease Attributable to Selected Major Risk Factors. Geneva: World Health Organization, 1987–1997.
Falcone, L. M., Erdely, A., Salmen, R., Keane, M., Battelli, L., Kodali, V., et al. (2018). Pulmonary toxicity and lung tumorigenic potential of surrogate metal oxides in gas metal arc welding–stainless steel fume: Iron as a primary mediator versus chromium and nickel. PLoS ONE 13:e0209413. doi: 10.1371/journal.pone.0209413
Feng, X., Chen, A., Zhang, Y., Wang, J., Shao, L., and Wei, L. (2015). Central nervous system toxicity of metallic nanoparticles. Int. J. Nanomed. 10:4321. doi: 10.2147/IJN.S78308
Ferreira, A. J., Cemlyn-Jones, J., and Cordeiro, C. R. (2013). Nanoparticles, nanotechnology and pulmonary nanotoxicology. Rev. Portuguesa Pneumol. 19, 28–37. doi: 10.1016/j.rppnen.2013.01.004
Foldbjerg, R., Dang, D. A., and Autrup, H. (2011). Cytotoxicity and genotoxicity of silver nanoparticles in the human lung cancer cell line, A549. Arch. Toxicol. 85, 743–750. doi: 10.1007/s00204-010-0545-5
Fröhlich, E., and Salar-Behzadi, S. (2014). Toxicological assessment of inhaled nanoparticles: role of in vivo, ex vivo, in vitro, and in silico studies. Int. J. Mol. Sci. 15, 4795–4822. doi: 10.3390/ijms15034795
Garcia, G. J., and Kimbell, J. S. (2009). Deposition of inhaled nanoparticles in the rat nasal passages: dose to the olfactory region. Inhal. Toxicol. 21, 1165–1175. doi: 10.3109/08958370902882713
Geiser, M. (2010). Update on macrophage clearance of inhaled micro-and nanoparticles. J. Aerosol Med. Pulm. Drug Deliv. 23, 207–217. doi: 10.1089/jamp.2009.0797
Georgantzopoulou, A., Serchi, T., Cambier, S., Leclercq, C. C., Renaut, J., Shao, J., et al. (2015). Effects of silver nanoparticles and ions on a co-culture model for the gastrointestinal epithelium. Part. Fibre Toxicol. 13, 1–17. doi: 10.1186/s12989-016-0117-9
Gerloff, K., Albrecht, C., Boots, A. W., Förster, I., and Schins, R. P. (2009). Cytotoxicity and oxidative DNA damage by nanoparticles in human intestinal Caco-2 cells. Nanotoxicology 3, 355–364. doi: 10.3109/17435390903276933
Giorgetti, L. (2019). Effects of nanoparticles in plants: phytotoxicity and genotoxicity assessment. Nanomater. Plants Algae Microorg. 1, 65–87. doi: 10.1016/B978-0-12-811488-9.00004-4
Goel, A., Saxena, P., Sonwani, S., Rathi, S., Srivastava, A., Bharti, A. K., et al. (2021). Health benefits due to reduction in respirable particulates during COVID-19 lockdown in India. Aerosol Air Qual. Res. 21:200460. doi: 10.4209/aaqr.200460
Gonzalez, C., Rosas-Hernandez, H., Ramirez-Lee, M. A., Salazar-García, S., and Ali, S. F. (2016). Role of silver nanoparticles (AgNPs) on the cardiovascular system. Arch. Toxicol. 90, 493–511. doi: 10.1007/s00204-014-1447-8
Gordon, S. M., Wallace, L. A., Brinkman, M. C., Callahan, P. J., and Kenny, D. V. (2002). Volatile organic compounds as breath biomarkers for active and passive smoking. Environ. Health Perspect. 110, 689–698. doi: 10.1289/ehp.02110689
Guo, C., Wang, J., Yang, M., Li, Y., Cui, S., Zhou, X., et al. (2017). Amorphous silica nanoparticles induce malignant transformation and tumorigenesis of human lung epithelial cells via P53 signaling. Nanotoxicology 11, 1176–1194. doi: 10.1080/17435390.2017.1403658
Gurjar, B. R., Ravindra, K., and Nagpure, A. S. (2016). Air pollution trends over Indian megacities and their local-to-global implications. Atmos. Environ. 142, 475–495. doi: 10.1016/j.atmosenv.2016.06.030
Haase, A., Rott, S., Mantion, A., Graf, P., Plendl, J., Thünemann, A. F., et al. (2012). Effects of silver nanoparticles on primary mixed neural cell cultures: uptake, oxidative stress and acute calcium responses. Toxicol. Sci. 126, 457–468. doi: 10.1093/toxsci/kfs003
Hao, L., and Chen, L. (2012). Oxidative stress responses in different organs of carp (Cyprinus carpio) with exposure to ZnO nanoparticles. Ecotoxicol. Environ. Saf. 80, 103–110. doi: 10.1016/j.ecoenv.2012.02.017
Henning, A., Schneider, M., Nafee, N., Muijs, L., Rytting, E., Wang, X., et al. (2010). Influence of particle size and material properties on mucociliary clearance from the airways. J. Aerosol Med. Pulm. Drug Deliv. 23, 233–241. doi: 10.1089/jamp.2009.0806
Herr, C. E. W., Zur Nieden, A., Jankofsky, M., Stilianakis, N. I., Boedeker, R. H., and Eikmann, T. F. (2003). Effects of bioaerosol polluted outdoor air on airways of residents: a cross sectional study. Occup. Environ. Med. 60, 336–342. doi: 10.1136/oem.60.5.336
Hong, F., Ji, L., Zhou, Y., and Wang, L. (2017). Retracted: chronic nasal exposure to nanoparticulate TiO2 causes pulmonary tumorigenesis in male mice. Environ. Toxicol. 32, 1651–1657. doi: 10.1002/tox.22393
Hooper, L. G., Dieye, Y., Ndiaye, A., Fan, V. S., Diallo, A., and Ortiz, J. R. (2015). B46 health effects of air pollution and nanoparticles: women's exposure to household air pollution in rural senegal. Am. J. Respir. Crit. Care Med. 191:1.
Hosgood, H. D., Lan, Q., Vermeulen, R., Wei, H., Reiss, B., Coble, J., et al. (2012). Combustion-derived nanoparticle exposure and household solid fuel use in Xuanwei and Fuyuan, China. Int. J. Environ. Health Res. 22, 571–581. doi: 10.1080/09603123.2012.684147
Huang, C. C., Aronstam, R. S., Chen, D. R., and Huang, Y. W. (2010). Oxidative stress, calcium homeostasis, and altered gene expression in human lung epithelial cells exposed to ZnO nanoparticles. Toxicol. In Vitro 24, 45–55. doi: 10.1016/j.tiv.2009.09.007
Iavicoli, I., Fontana, L., Leso, V., and Bergamaschi, A. (2013). The effects of nanomaterials as endocrine disruptors. Int. J. Mol. Sci. 14, 16732–16801. doi: 10.3390/ijms140816732
Inthavong, K., Zhang, K., and Tu, J. (2011). Numerical modelling of nanoparticle deposition in the nasal cavity and the tracheobronchial airway. Comput. Methods Biomech. Biomed. Engin. 14, 633–643. doi: 10.1080/10255842.2010.493510
Iversen, T. G., Skotland, T., and Sandvig, K. (2011). Endocytosis and intracellular transport of nanoparticles: present knowledge and need for future studies. Nano Today 6, 176–185. doi: 10.1016/j.nantod.2011.02.003
Jeevanandam, J., Barhoum, A., Chan, Y. S., Dufresne, A., and Danquah, M. K. (2018). Review on nanoparticles and nanostructured materials: history, sources, toxicity and regulations. Beilstein J. Nanotechnol. 9, 1050–1074. doi: 10.3762/bjnano.9.98
Ji, J. H., and Yu, I. J. (2012). Estimation of human equivalent exposure from rat inhalation toxicity study of silver nanoparticles using multi-path particle dosimetry model. Toxicol. Res. 1, 206–210. doi: 10.1039/c2tx20029e
Jiang, Z., Shan, K., Song, J., Liu, J., Rajendran, S., Pugazhendhi, A., et al. (2019). Toxic effects of magnetic nanoparticles on normal cells and organs. Life Sci. 220, 156–161. doi: 10.1016/j.lfs.2019.01.056
Jones, K., Morton, J., Smith, I., Jurkschat, K., Harding, A. H., and Evans, G. (2015). Human in vivo and in vitro studies on gastrointestinal absorption of titanium dioxide nanoparticles. Toxicol. Lett. 233, 95–101. doi: 10.1016/j.toxlet.2014.12.005
Kar, A., Rehman, I. H., Burney, J., Puppala, S. P., Suresh, R., Singh, L., et al. (2012). Real-time assessment of black carbon pollution in Indian households due to traditional and improved biomass cookstoves. Environ. Sci. Technol. 46, 2993–3000. doi: 10.1021/es203388g
Kirch, J., Guenther, M., Doshi, N., Schaefer, U. F., Schneider, M., Mitragotri, S., et al. (2012). Mucociliary clearance of micro-and nanoparticles is independent of size, shape and charge—an ex vivo and in silico approach. J. Controlled Release 159, 128–134. doi: 10.1016/j.jconrel.2011.12.015
Kreyling, W. G., Semmler-Behnke, M., and Möller, W. (2006). Ultrafine particle–lung interactions: does size matter?. Jo. Aerosol Med. 19, 74–83. doi: 10.1089/jam.2006.19.74
Kuempel, E. D., Tran, C. L., Castranova, V., and Bailer, A. J. (2006). Lung dosimetry and risk assessment of nanoparticles: evaluating and extending current models in rats and humans. Inhal. Toxicol. 18, 717–724. doi: 10.1080/08958370600747887
Kuhn, T., Biswas, S., and Sioutas, C. (2005). Diurnal and seasonal characteristics of particle volatility and chemical composition in the vicinity of a light-duty vehicle freeway. Atmos. Environ. 39, 7154–7166. doi: 10.1016/j.atmosenv.2005.08.025
Kumar, P., Pirjola, L., Ketzel, M., and Harrison, R. M. (2013). Nanoparticle emissions from 11 non-vehicle exhaust sources–a review. Atmos. Environ. 67, 252–277. doi: 10.1016/j.atmosenv.2012.11.011
Kumar, P., Robins, A., Vardoulakis, S., and Britter, R. (2010). A review of the characteristics of nanoparticles in the urban atmosphere and the prospects for developing regulatory controls. Atmos. Environ. 44, 5035–5052. doi: 10.1016/j.atmosenv.2010.08.016
Leso, V., Fontana, L., Marinaccio, A., Leopold, K., Fanali, C., Lucchetti, D., et al. (2018). Palladium nanoparticle effects on endocrine reproductive system of female rats. Hum. Exp. Toxicol. 37, 1069–1079. doi: 10.1177/0960327118756722
Li, C., Taneda, S., Taya, K., Watanabe, G., Li, X., Fujitani, Y., et al. (2009). Effects of inhaled nanoparticle-rich diesel exhaust on regulation of testicular function in adult male rats. Inhal. Toxicol. 21, 803–811. doi: 10.1080/08958370802524381
Li, J. J. E., Muralikrishnan, S., Ng, C. T., Yung, L. Y. L., and Bay, B. H. (2010). Nanoparticle-induced pulmonary toxicity. Exp. Biol. Med. 235, 1025–1033. doi: 10.1258/ebm.2010.010021
Li, N., Xia, T., and Nel, A. E. (2008). The role of oxidative stress in ambient particulate matter-induced lung diseases and its implications in the toxicity of engineered nanoparticles. Free Radic. Biol. Med. 44, 1689–1699. doi: 10.1016/j.freeradbiomed.2008.01.028
Lin, S., Wang, X., Ji, Z., Chang, C. H., Dong, Y., Meng, H., et al. (2014). Aspect ratio plays a role in the hazard potential of CeO2 nanoparticles in mouse lung and zebrafish gastrointestinal tract. ACS Nano 8, 4450–4464. doi: 10.1021/nn5012754
Liou, S. H., Wu, W. T., Liao, H. Y., Chen, C. Y., Tsai, C. Y., Jung, W. T., et al. (2017). Global DNA methylation and oxidative stress biomarkers in workers exposed to metal oxide nanoparticles. J. Hazard. Mater. 331, 329–335. doi: 10.1016/j.jhazmat.2017.02.042
Lomer, M. C., Hutchinson, C., Volkert, S., Greenfield, S. M., Catterall, A., Thompson, R. P., et al. (2004). Dietary sources of inorganic microparticles and their intake in healthy subjects and patients with Crohn's disease. Br. J. Nutr. 92, 947–955. doi: 10.1079/BJN20041276
Longest, P. W., and Vinchurkar, S. (2007). Validating CFD predictions of respiratory aerosol deposition: effects of upstream transition and turbulence. J. Biomech. 40, 305–316. doi: 10.1016/j.jbiomech.2006.01.006
Lu, X., Liu, Y., Kong, X., Lobie, P. E., Chen, C., and Zhu, T. (2013). Nanotoxicity: a growing need for study in the endocrine system. Small 9, 1654–1671. doi: 10.1002/smll.201201517
Lu, X., Zhu, T., Chen, C., and Liu, Y. (2014). Right or left: the role of nanoparticles in pulmonary diseases. Int. J. Mol. Sci. 15, 17577–17600. doi: 10.3390/ijms151017577
Luanpitpong, S., Talbott, S. J., Rojanasakul, Y., Nimmannit, U., Pongrakhananon, V., Wang, L., et al. (2010). Regulation of lung cancer cell migration and invasion by reactive oxygen species and caveolin-1. J. Biol. Chem. 285, 38832–38840. doi: 10.1074/jbc.M110.124958
Magdolenova, Z., Collins, A., Kumar, A., Dhawan, A., Stone, V., and Dusinska, M. (2014). Mechanisms of genotoxicity. A review of in vitro and in vivo studies with engineered nanoparticles. Nanotoxicology 8, 233–278. doi: 10.3109/17435390.2013.773464
Manigrasso, M., Protano, C., Astolfi, M. L., Massimi, L., Avino, P., Vitali, M., et al. (2019). Evidences of copper nanoparticle exposure in indoor environments: Long-term assessment, high-resolution field emission scanning electron microscopy evaluation, in silico respiratory dosimetry study and possible health implications. Sci. Total Environ. 653, 1192–1203. doi: 10.1016/j.scitotenv.2018.11.044
Manke, A., Wang, L., and Rojanasakul, Y. (2013). Mechanisms of nanoparticle-induced oxidative stress and toxicity. Biomed Res. Int. 2013:942916. doi: 10.1155/2013/942916
Martin, A., and Sarkar, A. (2017). Overview on biological implications of metal oxide nanoparticle exposure to human alveolar A549 cell line. Nanotoxicology 11, 713–724. doi: 10.1080/17435390.2017.1366574
Medina, C., Santos-Martinez, M. J., Radomski, A., Corrigan, O. I., and Radomski, M. W. (2007). Nanoparticles: pharmacological and toxicological significance. Br. J. Pharmacol. 150, 552–558. doi: 10.1038/sj.bjp.0707130
Miller, M. R., Shaw, C. A., and Langrish, J. P. (2012). From particles to patients: oxidative stress and the cardiovascular effects of air pollution. Fut. Cardiol. 8, 577–602. doi: 10.2217/fca.12.43
Mills, N. L., and Miller, M. R. (2011). Particles and the vascular endothelium. Cardiovasc. Effects Inhaled Ultrafine Nanosized Particles 1, 379–402. doi: 10.1002/9780470910917.ch19
Mohammad, Y., Shaaban, R., Abou Al-Zahab, B., Khaltaev, N., Bousquet, J., and Dubaybo, B. (2013). Impact of active and passive smoking as risk factors for asthma and COPD in women presenting to primary care in Syria: first report by the WHO-GARD survey group. Int. J. Chron. Obstruct. Pulmon. Dis. 8:473. doi: 10.2147/COPD.S50551
Moitra, S., Puri, R., Paul, D., and Huang, Y. C. T. (2015). Global perspectives of emerging occupational and environmental lung diseases. Curr. Opin. Pulm. Med. 21, 114–120. doi: 10.1097/MCP.0000000000000136
Moller, W., Haussinger, K., Winkler-Heil, R., Stahlhofen, W., Meyer, T., Hofmann, W., et al. (2004). Mucociliary and long-term particle clearance in the airways of healthy nonsmoker subjects. J. Appl. Physiol. 97, 2200–2206. doi: 10.1152/japplphysiol.00970.2003
Nakajima, T., Yoon, S. C., Ramanathan, V., Shi, G. Y., Takemura, T., Higurashi, A., et al. (2007). Overview of the atmospheric brown cloud East Asian regional experiment 2005 and a study of the aerosol direct radiative forcing in east Asia. J. Geophys. Res. Atmos. 112:1–23. doi: 10.1029/2007JD009009
Nasterlack, M., Zober, A., and Oberlinner, C. (2008). Considerations on occupational medical surveillance in employees handling nanoparticles. Int. Arch. Occup. Environ. Health. 81, 721–726. doi: 10.1007/s00420-007-0245-5
Nel, A., Xia, T., Mädler, L., and Li, N. (2006). Toxic potential of materials at the nanolevel. Science 311, 622–627. doi: 10.1126/science.1114397
Nemmar, A., Holme, J. A., Rosas, I., Schwarze, P. E., and Alfaro-Moreno, E. (2013). Recent advances in particulate matter and nanoparticle toxicology: a review of the in vivo and in vitro studies. Biomed Res. Int. 2013:279371. doi: 10.1155/2013/279371
Nho, R. (2020). Pathological effects of nano-sized particles on the respiratory system. Nanomed. Nanotechnol. Biol. Med. 29:102242. doi: 10.1016/j.nano.2020.102242
Nishi, K. I., Kadoya, C., Ogami, A., Oyabu, T., Morimoto, Y., Ueno, S., et al. (2020). Changes over time in pulmonary inflammatory response in rat lungs after intratracheal instillation of nickel oxide nanoparticles. J. Occup. Health. 62:e12162. doi: 10.1002/1348-9585.12162
Oliveira, M. L., Marostega, F., Taffarel, S. R., Saikia, B. K., Waanders, F. B., DaBoit, K., et al. (2014). Nano-mineralogical investigation of coal and fly ashes from coal-based captive power plant (India): an introduction of occupational health hazards. Sci. Total Environ. 468, 1128–1137. doi: 10.1016/j.scitotenv.2013.09.040
Pacurari, M., Lowe, K., Tchounwou, P. B., and Kafoury, R. (2016). A review on the respiratory system toxicity of carbon nanoparticles. Int. J. Environ. Res. Public Health 13:325. doi: 10.3390/ijerph13030325
Padmanabhan, J., and Kyriakides, T. R. (2015). Nanomaterials, inflammation, and tissue engineering. Wiley Interdiscip. Rev. Nanomed. Nanobiotechnol. 7, 355–370. doi: 10.1002/wnan.1320
Pakkanen, T. A., Kerminen, V. M., Korhonen, C. H., Hillamo, R. E., Aarnio, P., Koskentalo, T., et al. (2001). Urban and rural ultrafine (PM0. 1) particles in the Helsinki area. Atmos. Environ. 35, 4593–4607. doi: 10.1016/S1352-2310(01)00167-4
Peters, K., Unger, R. E., Gatti, A. M., Sabbioni, E., Tsaryk, R., and Kirkpatrick, C. J. (2007). Metallic nanoparticles exhibit paradoxical effects on oxidative stress and pro-inflammatory response in endothelial cells in vitro. Int. J. Immunopathol. Pharmacol. 20, 685–695. doi: 10.1177/039463200702000404
Pipal, A. S., Taneja, A., and Jaiswar, G. (2014). “Risk assessment and toxic effects of exposure to nanoparticles associated with natural and anthropogenic sources,” in Chemistry: The Key to our Sustainable Future, eds M. Gupta Bhowon, S. Jhaumeer-Laulloo, H. Li Kam Wah, and P. Ramasami (Dordrecht: Springer). doi: 10.1007/978-94-007-7389-9_6
Praphawatvet, T., Peters, J. I., and Williams, R. O. III. (2020). Inhaled nanoparticles—an updated review. Int. J. Pharm. 587:119671. doi: 10.1016/j.ijpharm.2020.119671
Pujalté, I., Passagne, I., Brouillaud, B., Tréguer, M., Durand, E., Ohayon-Courtès, C., et al. (2011). Cytotoxicity and oxidative stress induced by different metallic nanoparticles on human kidney cells. Part. Fibre Toxicol. 8, 1–16. doi: 10.1186/1743-8977-8-10
Qiao, H., Liu, W., Gu, H., Wang, D., and Wang, Y. (2015). The transport and deposition of nanoparticles in respiratory system by inhalation. J. Nanomater. 2015:394507. doi: 10.1155/2015/394507
Querol, X., Alastuey, A., Pey, J., Viana, M., Moreno, T., Minguillón, M. C., et al. (2004). Nanoparticles in the atmosphere. Seminario Sem. 8, 24–39.
Ravindra, K., Singh, T., Mor, S., Singh, V., Mandal, T. K., Bhatti, M. S., et al. (2019). Real-time monitoring of air pollutants in seven cities of North India during crop residue burning and their relationship with meteorology and transboundary movement of air. Sci. Total Environ. 690, 717–729. doi: 10.1016/j.scitotenv.2019.06.216
Riipinen, I., Yli-Juuti, T., Pierce, J. R., Petäjä, T., Worsnop, D. R., Kulmala, M., et al. (2012). The contribution of organics to atmospheric nanoparticle growth. Nat. Geosci. 5, 453–458. doi: 10.1038/ngeo1499
Rothen-Rutishauser, B., Müller, L., Blank, F., Brandenberger, C., Mühlfeld, C., and Gehr, P. (2008). A newly developed in vitro model of the human epithelial airway barrier to study the toxic potential of nanoparticles. ALTEX-Altern. Anim. Experiment. 25, 191–196. doi: 10.14573/altex.2008.3.191
Sajid, M., Ilyas, M., Basheer, C., Tariq, M., Daud, M., Baig, N., et al. (2015). Impact of nanoparticles on human and environment: review of toxicity factors, exposures, control strategies, and future prospects. Environ. Sci. Pollut. Res. 22, 4122–4143. doi: 10.1007/s11356-014-3994-1
Santonastaso, M., Mottola, F., Colacurci, N., Iovine, C., Pacifico, S., Cammarota, M., et al. (2019). In vitro genotoxic effects of titanium dioxide nanoparticles (n-TiO2) in human sperm cells. Mol. Reprod. Dev. 86, 1369–1377. doi: 10.1002/mrd.23134
Sardar, S. B., Fine, P. M., Mayo, P. R., and Sioutas, C. (2005). Size-fractionated measurements of ambient ultrafine particle chemical composition in Los Angeles using the NanoMOUDI. Environ. Sci. Technol. 39, 932–944. doi: 10.1021/es049478j
Sarkar, S., Singh, R. P., and Chauhan, A. (2018a). Crop residue burning in northern India: increasing threat to Greater India. J. Geophys. Res. Atmos. 123, 6920–6934. doi: 10.1029/2018JD028428
Sarkar, S., Singh, R. P., and Chauhan, A. (2018b). Increasing health threat to greater parts of India due to crop residue burning. Lancet Planetary Health 2, e327–e328. doi: 10.1016/S2542-5196(18)30166-9
Saxena, P., and Kulshrestha, U. C. (2016). Contribution of carbonaceous species in SOA formation during fog and non-fog period. J. Chem. Biol. Phys. Sci. 7, 31–48.
Saxena, P., and Sonwani, S. (2019). Criteria Air Pollutants and Their Impact on Environmental Health. Singapore: Springer.
Saxena, P., and Sonwani, S. (2020). Remediation of ozone pollution by ornamental plants in indoor environment. Global J. Environ. Sci. Manag. 6, 497–508. doi: 10.22034/GJESM.2020.04.06
Saxena, P., Sonwani, S., Srivastava, A., Jain, M., Srivastava, A., Bharti, A., et al. (2021b). Impact of crop residue burning in Haryana on the air quality of Delhi, India. Heliyon 7:e06973. doi: 10.1016/j.heliyon.2021.e06973
Saxena, P., Srivastava, A., Shweta, Rangra, D., Nancy, Bharti, A., Bhardwaj S., et al. (2021a). “Chapter 15 - Investigating the Problem of Crop Residue Burning in an Indo-Gangetic Plain (IGP)-An Emerging Concern to Air Quality,” in Management of Contaminants of Emerging Concern (CEC) in Environment, eds P. Singh, C. M. Hussain, and S. Rajkhowa (Elsevier), 395–414. doi: 10.1016/B978-0-12-822263-8.00015-4
Saxena, P., Srivastava, A., Verma, S., Shweta, Singh L., and Sonwani, S. (2020). “Analysis of atmospheric pollutants during fireworks festival ‘diwali’ at a residential site Delhi in India,” in Measurement, Analysis and Remediation of Environmental Pollutants. Energy, Environment, and Sustainability, eds T. Gupta, S. Singh, P. Rajput, and A. Agarwal (Singapore: Springer). doi: 10.1007/978-981-15-0540-9_4
Schins, R. (2013). Genotoxicity of nanoparticles. Nanomaterials 1, 60–64. doi: 10.1002/9783527673919.ch8
Scholl, J. A., Koh, A. L., and Dionne, J. A. (2012). Quantum plasmon resonances of individual metallic nanoparticles. Nature 483, 421–427. doi: 10.1038/nature10904
Seaton, A., Tran, L., Aitken, R., and Donaldson, K. (2010). Nanoparticles, human health hazard and regulation. J. R. Soc. Interface 7, S119–S129. doi: 10.1098/rsif.2009.0252.focus
Shakya, K. M., Peltier, R. E., Zhang, Y., and Pandey, B. D. (2019). Roadside exposure and inflammation biomarkers among a cohort of traffic police in Kathmandu, Nepal. Int. J. Environ. Res. Public Health 16:377. doi: 10.3390/ijerph16030377
Sharma, V., Singh, P., Pandey, A. K., and Dhawan, A. (2012). Induction of oxidative stress, DNA damage and apoptosis in mouse liver after sub-acute oral exposure to zinc oxide nanoparticles. Mutat. Res. Genet. Toxicol. Environ. Mutagenesis 745, 84–91. doi: 10.1016/j.mrgentox.2011.12.009
Shi, X., Castranova, V., Halliwell, B., and Vallyathan, V. (1998). Reactive oxygen species and silica-induced carcinogenesis. J. Toxicol. Environ. Health Part B Crit. Rev. 1, 181–197. doi: 10.1080/10937409809524551
Singh, R. P., and Kaskaoutis, D. G. (2014). Crop residue burning: a threat to South Asian air quality. Eos Transac. Am. Geophys. Union 95, 333–334. doi: 10.1002/2014EO370001
Slezakova, K., Morais, S., and do Carmo Pereira, M. (2013). “Atmospheric nanoparticles and their impacts on public health,” in Current Topics in Public Health, eds A. J. Rodriguez-Morales (London: IntechOpen), 503–529. doi: 10.5772/54775
Sonwani, S., and Kulshreshtha, U. (2016). “Particulate matter levels and it's associated health risks in East Delhi,” in Proceedings of Indian Aerosol Science and Technology Association Conference on Aerosol and Climate Change: Insight and Challenges. IASTA Bull, Vol. 22 (Ahmedabad), 269–272.
Sonwani, S., and Saxena, P. (2021). Water-insoluble carbonaceous components in rainwater over an urban background location in Northern India during pre-monsoon and monsoon seasons. Environ. Sci. Pollut. Res. 1–16. doi: 10.1007/s11356-021-14132-w
Sonwani, S., Saxena, P., and Kulshrestha, U. (2016). “Role of global warming and plant signaling in BVOC emissions,” in Plant Responses to Air Pollution, eds U. Kulshrestha and P. Saxena (Singapore: Springer). doi: 10.1007/978-981-10-1201-3_5
Sonwani, S., Saxena, P., and Shukla, A. (2021). Carbonaceous aerosol characterization and their relationship with meteorological parameters during summer monsoon and winter monsoon at an industrial region in Delhi, India. Earth Space Sci. 8, 1–16. doi: 10.1029/2020EA001303
Sramkova, M., Kozics, K., Masanova, V., Uhnakova, I., Razga, F., Nemethova, V., et al. (2019). Kidney nanotoxicity studied in human renal proximal tubule epithelial cell line TH1. Mutat. Res. Genet. Toxicol. Environ. Mutagenesis 845:403017. doi: 10.1016/j.mrgentox.2019.01.012
Strambeanu, N., Demetrovici, L., and Dragos, D. (2015). “Anthropogenic sources of nanoparticles,” in Nanoparticles' Promises and Risks (Cham: Springer), 21–54.
Stueckle, T. A., Davidson, D. C., Derk, R., Kornberg, T. G., Schwegler-Berry, D., Pirela, S. V., et al. (2017). Evaluation of tumorigenic potential of CeO2 and Fe2O3 engineered nanoparticles by a human cell in vitro screening model. Nanoimpact 6, 39–54. doi: 10.1016/j.impact.2016.11.001
Tiwari, S., and Saxena, P. (2021). Air Pollution and Its Complications From the Regional to the Global Scale. Cham: Springer.
Ursini, C. L., Cavallo, D., Fresegna, A. M., Ciervo, A., Maiello, R., Tassone, P., et al. (2014). Evaluation of cytotoxic, genotoxic and inflammatory response in human alveolar and bronchial epithelial cells exposed to titanium dioxide nanoparticles. J. Appl. Toxicol. 34, 1209–1219. doi: 10.1002/jat.3038
Valdiglesias, V., Costa, C., Kiliç, G., Costa, S., Pásaro, E., Laffon, B., et al. (2013). Neuronal cytotoxicity and genotoxicity induced by zinc oxide nanoparticles. Environ. Int. 55, 92–100. doi: 10.1016/j.envint.2013.02.013
Vera-Reyes, I., Vázquez-Núñez, E., Lira-Saldivar, R. H., and Méndez-Argüello, B. (2018). “Effects of nanoparticles on germination, growth, and plant crop development,” in Agricultural Nanobiotechnology (Cham: Springer), 77–110.
Volkamer, R., Jimenez, J. L., San Martini, F., Dzepina, K., Zhang, Q., Salcedo, D., et al. (2006). Secondary organic aerosol formation from anthropogenic air pollution: Rapid and higher than expected. Geophys. Res. Lett. 33:L17811. doi: 10.1029/2006GL026899
Walsh, M. (2011). Global trends in motor vehicle pollution control: a 2011 update. Part 1. Silniki Spalinowe 50, 106–117. doi: 10.19206/CE-117109
Wang, F., Gao, F., Lan, M., Yuan, H., Huang, Y., and Liu, J. (2009). Oxidative stress contributes to silica nanoparticle-induced cytotoxicity in human embryonic kidney cells. Toxicol. In Vitro 23, 808–815. doi: 10.1016/j.tiv.2009.04.009
Warheit, D. B. (2004). Nanoparticles: health impacts?. Mater. Today 7, 32–35. doi: 10.1016/S1369-7021(04)00081-1
Williams, M., Villarreal, A., Bozhilov, K., Lin, S., and Talbot, P. (2013). Metal and silicate particles including nanoparticles are present in electronic cigarette cartomizer fluid and aerosol. PLoS ONE 8:e57987. doi: 10.1371/journal.pone.0057987
Yang, Z., Liu, Z. W., Allaker, R. P., Reip, P., Oxford, J., Ahmad, Z., et al. (2010). A review of nanoparticle functionality and toxicity on the central nervous system. J. Roy. Soc. Interface 7, S411–S422. doi: 10.1098/rsif.2010.0158.focus
Yhee, J. Y., Im, J., and Nho, R. S. (2016). Advanced therapeutic strategies for chronic lung disease using nanoparticle-based drug delivery. J. Clin. Med. 5:82. doi: 10.3390/jcm5090082
Yu, X., Hong, F., and Zhang, Y. Q. (2016). Bio-effect of nanoparticles in the cardiovascular system. J. Biomed. Mater. Res. Part A 104, 2881–2897. doi: 10.1002/jbm.a.35804
Zhang, L., Wei, J., Duan, J., Guo, C., Zhang, J., Ren, L., et al. (2020). Silica nanoparticles exacerbates reproductive toxicity development in high-fat diet-treated Wistar rats. J. Hazard. Mater. 384:121361. doi: 10.1016/j.jhazmat.2019.121361
Zhang, Y., Bai, Y., Jia, J., Gao, N., Li, Y., Zhang, R., et al. (2014). Perturbation of physiological systems by nanoparticles. Chem. Soc. Rev. 43, 3762–3809. doi: 10.1039/C3CS60338E
Keywords: nanoparticles, atmosphere, respiratory system, air quality, human health
Citation: Sonwani S, Madaan S, Arora J, Suryanarayan S, Rangra D, Mongia N, Vats T and Saxena P (2021) Inhalation Exposure to Atmospheric Nanoparticles and Its Associated Impacts on Human Health: A Review. Front. Sustain. Cities 3:690444. doi: 10.3389/frsc.2021.690444
Received: 02 April 2021; Accepted: 26 July 2021;
Published: 18 August 2021.
Edited by:
Prashant Rajput, Banaras Hindu University, IndiaReviewed by:
Hamid Omidvarborna, University of Surrey, United KingdomCopyright © 2021 Sonwani, Madaan, Arora, Suryanarayan, Rangra, Mongia, Vats and Saxena. This is an open-access article distributed under the terms of the Creative Commons Attribution License (CC BY). The use, distribution or reproduction in other forums is permitted, provided the original author(s) and the copyright owner(s) are credited and that the original publication in this journal is cited, in accordance with accepted academic practice. No use, distribution or reproduction is permitted which does not comply with these terms.
*Correspondence: Pallavi Saxena, cGFsbGF2aWVudmlyb25tZW50QGdtYWlsLmNvbQ==; cGFsbGF2aXNheGVuYUBoaW5kdWNvbGxlZ2UuYWMuaW4=
Disclaimer: All claims expressed in this article are solely those of the authors and do not necessarily represent those of their affiliated organizations, or those of the publisher, the editors and the reviewers. Any product that may be evaluated in this article or claim that may be made by its manufacturer is not guaranteed or endorsed by the publisher.
Research integrity at Frontiers
Learn more about the work of our research integrity team to safeguard the quality of each article we publish.