- School of Dentistry, Philips Institute for Oral Health Research, Massey Cancer Center, Virginia Commonwealth University, Richmond, VA, United States
Head and neck cancer is the sixth leading cancer worldwide; head and neck squamous cell carcinoma (HNSCC) accounts for more than 90% of incident cases. In the US, cases of HNSCC associated with human papillomavirus (HPV) have been growing in proportion amongst a younger demographic with superior outcomes to the same treatments, relative to cases associated with tobacco. Yet failures to improve the long-term prognosis of advanced HNSCC over the last three decades persist in part due to intrinsic and acquired mechanisms of resistance. Deregulation of the pathways to respond to stress, such as apoptosis and autophagy, often contributes to drug resistance and tumor progression. Here we review the stress-response pathways in drug response and resistance in HNSCC to explore strategies to overcome these resistance mechanisms. We focus on the mechanisms of resistance to current standard cares, such as chemotherapy (i.e., cisplatin), radiation, and cetuximab. Then, we discuss the strategies to overcome these resistances, including novel combinations and immunotherapy.
Introduction
Epidemiology
Head and neck cancer is the sixth most common category of malignancy worldwide, with head and neck squamous cell carcinomas (HNSCCs) accounting for >90% of cases. In much of the world, HNSCC correlates to tobacco usage and is diagnosed in advanced stages. These patients suffer >50% mortality as well as severe disabilities that result from highly toxic multimodality therapy [1–3]. In the developed world, there is rapidly increasing incidence of a human papillomavirus-related (HPV+) subtype of HNSCC, which arises in a younger patient demographic that includes many never-smokers. Worldwide, both HPV(+) and HPV(–) cases typically develop only locoregional disease, with just 7–9% of patients suffering distant metastasis at any point in the disease course. Although they receive the same toxic therapies, the HPV(+) cases have markedly superior survival outcomes [4]; this has created a large population of cured patients with lifelong treatment-related disabilities from a relatively young age and a proportional demand for deintensification strategies.
Current Standard of Care
Cisplatin remains the favored systemic agent for definitive therapy of HPV(+) and HPV(–) HNSCCs and is combined with external beam radiation during either primary non-surgical therapy or postoperative adjuvant therapy. In both treatment paradigms, cisplatin improves control of locoregional disease but may not prevent the rare instances of distant metastatic relapse, whose incidence was not reduced by the drug in two large phase III adjuvant trials [5, 6]. Cisplatin can cross-link with DNA, most often between guanine-guanine groups, causing DNA damage. This leads to the inhibition of replication and induces the cell death response. Unfortunately, cisplatin has acute dose-limiting toxicities that are potentially lethal and frequently contribute to serious long-term disability [2, 7, 8]. The worst effects of systemic administration include life-threatening bone marrow suppression, irreversible renal injury, and permanent hearing loss. As a result, many patients with poor performance status, reduced renal function, or hearing impairment at baseline suffer worse oncologic outcomes because of contraindication to receiving cisplatin. In addition, cisplatin is well-known to exacerbate the mucositis that is caused by radiation and creates a spectrum of permanent swallowing difficulties, including permanent feeding tube dependence [9, 10]. Thus, there is a major unmet clinical need to avoid the permanently disabling and potentially lethal toxicities of cisplatin for HNSCC patients while maintaining its proven benefit to control locoregional disease. Furthermore, some extent of inherent and acquired resistance to chemotherapy contributes to treatment failure. Therefore, it is necessary to elucidate the mechanisms underlying the development of these resistances.
Radiotherapy (RT) for tumor elimination is based on tumor-targeted ionizing radiation (IR): electromagnetic energy of sufficient magnitude to directly ionize atoms or molecules (e.g., γ-rays) that can induce cell death both directly via mitogenic stress and indirectly via oxidative stress [11]. Direct ionization of DNA induces double-strand breaks (DSBs) and single-strand breaks (SSBs) that trigger growth arrest and DNA repair. Direct ionization of RNA, lipids and proteins damage cell membranes, cytoskeletal networks and enzyme activity to deregulate cell functions and mitochondrial activity. Direct ionization of water generates reactive oxygen species (ROS)—including superoxide anion, hydrogen peroxide, and hydroxyl radical—that amplifies this damage through electron transfer to DNA, proteins and lipids (i.e., indirect ionization) and dysregulation of endogenous oxidative signaling [12]. Indeed, oxidative stress from RT can produce changes in local tissue electrical parameters (e.g., resistance, impedance module) that are predictive of oral mucositis in HNSCC patients [13]. Nevertheless, after cancer cells are exposed to IR or another source of ROS, numerous cell processes are activated to adapt to the stress and avoid cell death [14].
Targeting Programmed Cell Death
TP53
The tumor suppressor TP53 is a transcription factor that regulates the genes to preserve genome integrity in response to diverse endogenous (e.g., DNA damage, ROS, oncogenes) and exogenous stresses (e.g., smoking, cisplatin). At homeostasis, healthy cells maintain a low level of TP53 with continuous production, ubiquitination and degradation; but stresses which damage cell machinery and downregulate the proteasome can suppress the degradation of TP53 and lead to its accumulation. If the degree of stress is relatively low or transient, this accumulation of TP53 activates cell cycle arrest and DNA repair activity for survival. In contrast, relatively severe or sustained stresses lead to unrepairable damage and enhanced accumulation of TP53 that activates its killer functions, including cellular senescence and apoptosis [15, 16].
TP53 is the most commonly mutated gene (65–85%) in HNSCC, and TP53 mutations are largely associated with poor survival and resistance to chemotherapy and radiation in HNSCC patients [17, 18]. Thus, the TP53 status could be a prognostic and predictive biomarker of clinical response in these patients [19]. The exposure to DNA damaging agents (e.g., cisplatin) or radiation induces DNA SSBs or DSBs that activate TP53. In HPV(–) HNSCC cells with loss-of-function mutations in TP53, cell-cycle arrest and apoptosis are mitigated, resulting in cell survival and treatment failure with chemotherapy and radiation [20]. In contrast, gain-of-function (GOF) TP53 (e.g., R248W, R273H, R175H) not only lose the wild-type (WT) TP53 function, but also confer additional oncogenic properties to tumor cells [21–23]. Since most of the GOF TP53 mutations are located at the DNA binding domain, a subset of the target genes of GOF TP53 are different from those of WT TP53. Furthermore, the GOF TP53 protein becomes much more stable than WT TP53. As a result, GOF TP53 leads to resistance to DNA damage-induced cell death via downregulation and upregulation of pro-apoptotic and pro-survival genes, respectively. GOF TP53 can also impair recruitment of the Mre11-Rad50-NBS1 (MRN) complex to the site of DNA damage to inactivate ATM and promote proliferation, migration, and invasion, all of which may contribute to resistance [24]. Although HPV(+) oropharyngeal tumors are relatively treatment-sensitive, the mechanism is still obscure [25, 26]. Speculations include an association with TP53 status. The HPV genome contains the oncogenes, E6 and E7, which inactivate TP53 and Rb, respectively. E6 induces TP53 degradation by binding to E6-AP (UBE3A), a ubiquitin-protein ligase, thus, most HPV(+) tumors rarely express TP53 mutations. It has been suggested that E6 levels may be elevated in the early stage of tumorigenesis, so that inactivation of TP53 may be an early transient event [27]. Thus, the remaining WT TP53 may activate the killer functions.
Besides the mutations, the level and function of TP53 is tightly regulated by Mouse Double Minute 2 (MDM2) that is a target gene of TP53 and has an E3 ligase activity. MDM2 is transcriptionally induced by TP53, binds to TP53 and ubiquitinates following the proteasomal degradation. Therefore, TP53 and MDM2 are balanced by a negative feedback mechanism. In HNSCC, overexpression of MDM2 has been reported in ~40% of cases [28]. Mechanistically, it has been demonstrated that nucleotide polymorphisms (e.g., SNP309, rs2279744) contributes to the MDM2 expression, which may determine the sensitivity of chemoradiation [29].
To combat therapy-resistance conferred by mutant TP53 proteins, there are mainly two strategies to target them either directly or indirectly (Figure 1). WT TP53 is a potent inducer of apoptosis and senescence when expressed in tumor cells, thus reactivation of wild-type function in mutant TP53 is an attractive therapeutic approach to directly target mutant TP53. Since mutant TP53 proteins are generally expressed at high levels, several compounds that can restore WT TP53 function have been developed. PRIMA-1, a mutant TP53-reactivating compound and its derivative, PRIMA-1Met (APR-246), specifically bind to mutant TP53 proteins and interact with the DNA-binding domain, thereby promoting proper folding of mutant proteins and restoration of some WT TP53 functions [30, 31]. While APR-246 is being investigated in the Phase III clinical trial with TP53 mutant myelodysplastic syndromes (NCT03745716), one proposed mechanism of action is that both compounds are converted to methylene quinuclidinone (MQ) to covalently bind and modify thiols in the TP53 core domain, thus causes TP53 to restore its function to induce tumor cell death [32].
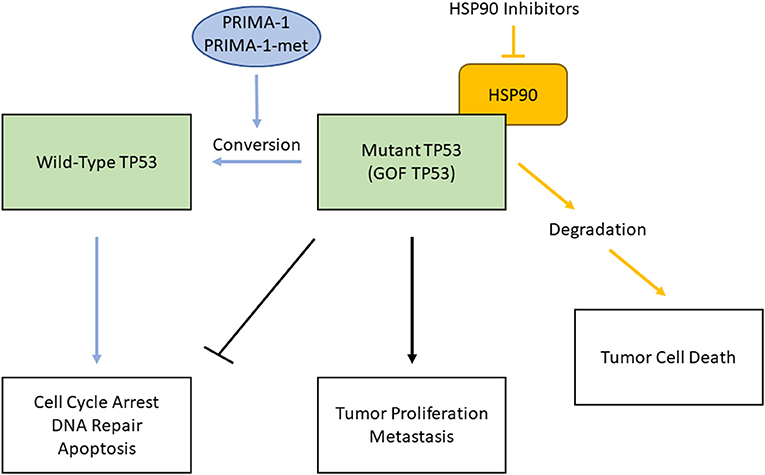
Figure 1. Two strategies to combat therapy-resistance caused by mutant TP53 proteins in HPV(-) HNSCC. Mutant, gain-of-function (GOF) TP53 loses the function of WT TP53 (e.g., cell cycle arrest, DNA repair, apoptosis) and acquires tumor proliferation and metastasis functions (black lines). PRIMA-1 and PRIMA-1-met bind to mutant TP53, interact with the DNA-binding domain, and restore WT TP53 functions (blue lines). Inhibition of HSP90 chaperone machinery destabilizes GOF TP53, which leads to cell death (orange lines).
Since GOF TP53 is often highly stabilized by the chaperone machinery, targeting this machinery is another strategy that induce mutant TP53 inactivation through protein degradation. For example, a major determinant of mutant p53 stabilization is mediated by HSP90 machinery. Inhibition of HSP90 alone or in combination with its regulator, HDAC6, a cytosolic non-histone histone deacetylase (HDAC), has marked anti-tumoral effects in vivo [33–35]. Inhibition of HDAC6 leads to inactivation of HSP90 and allows for reactivation of ubiquitin ligases MDM2 and CHIP E3 to mediate mutant TP53 degradation, but not degradation of WT TP53 [33]. These anticancer effects are concomitant with mutant TP53 degradation followed by cancer cell death, indicating tumor addiction to highly stable mutant TP53.
The BCL-2 Family
The BCL-2 family primarily regulates mitochondrial-dependent apoptosis induced by a variety of external stimuli including chemotherapy and radiation. The BCL-2 family is subdivided into three groups based on the structure and function; [1] multi-domain pro-apoptotic (e.g., BAX, BAK), [2] pro-survival (e.g., BCL-2, BCL-XL, MCL-1), [3] BH3-only pro-apoptotic (e.g., NOXA, BIM, BAD, BID). When BH3-only proteins are activated by a variety of external stimuli, BAX and/or BAK is subsequently conformationally changed and oligomerized at the mitochondria, resulting in cytochrome c release to the cytosol, while the pro-survival proteins prevent the activation of BH3-only proteins and/or BAX/BAK [36–38]. The balance between pro- and anti-apoptotic proteins governs the cells either to survival or death. Tumor cells often overexpress anti-apoptotic proteins, such as BCL-2, BCL-XL and MCL-1, which results in the intrinsic as well as the acquired resistance to chemotherapy and radiotherapy. BCL-XL and MCL-1 are overexpressed in a majority of primary HNSCC specimens, whereas overexpression of BCL-2 is observed somewhat less frequently [39]. Upregulation of BCL-2 and BCL-XL is correlated with chemotherapy-resistance in HNSCC cells, and downregulation of these proteins by antisense or siRNA can sensitize to chemotherapy in HNSCC cells. It has been reported that BCL-XL is involved in the resistance of oropharyngeal cancer to ionizing radiation, and radioresistant laryngeal cancer is associated with BCL-2 expression [40]. Another report suggests that MCL-1 expression (but not BCL-2 or BCL-XL) is upregulated in both chemo-resistant HNSCC lines and chemo-resistant tumors compared with chemo-sensitive counterparts [41]. These data suggest that these anti-apoptotic proteins confer chemotherapy and radiotherapy resistance.
In order to inhibit the function of anti-apoptotic BCL-2 family members to overcome resistance, a number of small molecules have been developed. These compounds, so called BH3-mimetics, bind to the hydrophobic pockets of anti-apoptotic BCL-2 family proteins, which results in the release of pro-apoptotic BCL-2 family proteins to activate downstream caspase cascades [42, 43] (Figure 2). ABT-737 is a prototype of this class of compound that binds to BCL-XL and BCL-2, resulting in the release of pro-apoptotic BH3-only proteins and BAX/BAK. This compound can strongly synergize with the chemotherapy drugs or radiation to promote apoptosis of HNSCC cells [44, 45]. More recent reports have shown that inhibition of BCL-XL by an orally available ABT-737-derivative, ABT-263 (navitoclax), and MCL-1 by A-1210477 enhances apoptosis in HNSCC cells [46]. We have recently demonstrated that simultaneous inhibition of all anti-apoptotic BCL-2 family proteins by ectopic expression of NOXA, a BH3-only protein inhibiting MCL-1, and ABT-263 enhances apoptosis regardless of the HPV or p53 statuses [47]. Furthermore, an inducer of endoplasmic reticulum stress, fenretinide, increases the NOXA expression, and a combination of fenretinide and ABT-263 strongly enhances apoptosis in HNSCC cell lines. Future clinical trials using the above drugs in combination are awaiting to judge efficacy and non-specific toxicities.
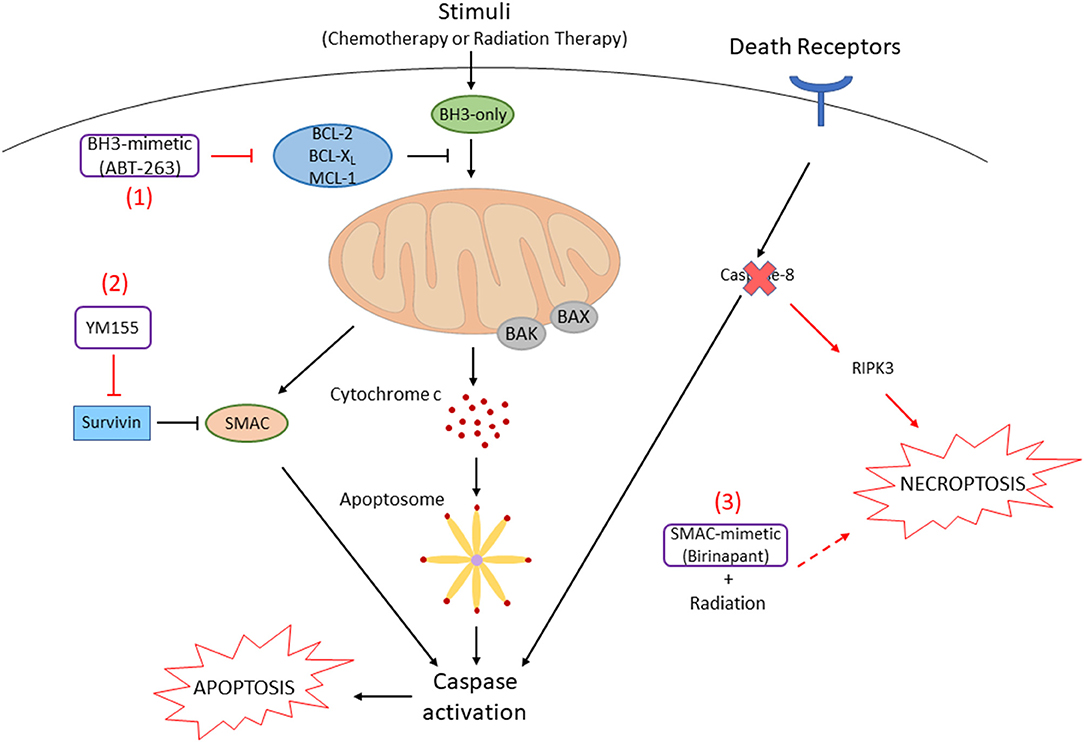
Figure 2. Small-molecule inhibitors promote cell death in HNSCC cells. (1) In HNSCC cells, anti-apoptotic BCL-2 family proteins are often overexpressed. The BH3-mimetic compound, ABT-263, inhibits the function of these proteins by binding to their hydrophobic pockets. Pro-apoptotic BCL-2 family proteins (BAX, BAK) release to initiate the release of cytochrome c, following downstream caspase cascades that result in apoptosis. (2) Survivin, an Inhibitor of Apoptosis Protein (IAP), inhibits Smac that is released from the mitochondria followed by cell death stimuli. Survivin is also often overexpressed in HNSCC, which can be inhibited by the survivin inhibitor, YM155. (3) Caspase-8, which is activated by extrinsic death receptors (e.g., FAS, TNFR) to induce apoptosis, is mutated and inactivated in ~10% of HNSCC. When caspase-8 is inactivated, RIPK3-mediated necroptosis can be promoted. Necroptosis by radiation is enhanced when combined with a SMAC mimetic, birinapant.
Survivin
Survivin was originally identified as an Inhibitor of Apoptosis Protein (IAP) family member that acts as a cell death suppressor [48]. Subsequently, survivin has been recognized as a mediator between the cell cycle and apoptosis [49]. Survivin can block apoptosis and promote cell proliferation and survival by the interaction with the proteins that regulate intrinsic and extrinsic apoptotic pathways. Cytosolic survivin prevents caspase-9 activation by binding to Smac (second mitochondria-derived activator of caspase) and prevents subsequent caspase-3 activation [50]. Survivin also inhibits caspase-independent cell death by interacting with the apoptosis-inducing factor (AIF) [51]. In addition, survivin contributes to the cellular stress response by interfering with autophagy [52]. For example, Beclin-1 is able to bind to survivin, resulting in the initiation of autophagy. In addition, survivin interacts with LC3 (microtubule-associated protein 1 light chain 3) and interferes with the formation of autophagosome by preventing the conversion from LC3-I to LC3-II [53].
Survivin is often overexpressed in about 80% of HNSCCs and 50% of premalignant lesions, suggesting that survivin may be involved in the early stages of tumorigenesis [54, 55]. Survivin is proposed as an ideal biomarker for HNSCC due to its expression in selective tumors and near absence in normal tissues [56]. Interestingly, the localization of survivin in cytosol is associated with poor overall survival and disease outcome [57, 58]. Furthermore, it has been demonstrated that cytosolic survivin confers resistance to chemotherapy- and radiation-induced apoptosis in HNSCC cell lines [59, 60].
A selective small-molecule inhibitor for survivin, YM155, has been developed, and it can reverse cisplatin-resistance by decreasing the levels of cytoplasmic survivin [61]. YM155 can induce apoptosis through mitochondrial- and death receptor-dependent pathways (Figure 2). YM155 can also induce autophagic cell death in HNSCC cells by inhibiting the pro-survival AKT/mTOR pathway and inducing Beclin-1 expression [62]. YM155 is able to promote autophagic cell death in breast cancer cells by increasing the conversion from LC3-I to LC3-II [63]. Phase I/II clinical trials have investigated the effect of YM155 in patients with advanced solid tumors including HNSCC [64–66]. The trials revealed that YM155 as monotherapy is safe with slight side effects, but positive effects are not observed in patients. It may still have a clinical benefit in combination with a less toxic dose of chemotherapy, radiation, and other targeting drugs.
Caspase-8
Caspase-8 (CASP8) is an initiator of the extrinsic apoptosis pathway and inhibits necroptosis, indicating a molecular switch for these cell death pathways [67]. In HPV (-) HNSCC, caspase-8 is one of the most frequently mutated genes, with approximately 10% of cases [68]. The distribution of CASP8 mutations in patient tumors and cell lines suggests that the function of mutants is inactivated. The loss of caspase-8 function is known to shift signaling by death receptors from apoptosis signaling to necroptosis signaling. SMAC mimetics are small-molecule inhibitors that generally promote caspase activation and apoptosis through neutralization of IAPs [69]. Several preclinical studies have demonstrated that SMAC mimetics enhance radiosensitivity in HNSCC xenograft models. It has been recently demonstrated that inhibition of CASP8 function enhances necroptosis by radiation when combined with a SMAC mimetic, birinapant in vitro and in vivo (Figure 2) [70]. Furthermore, the level of RIPK3 expression determines necroptosis sensitivity in HNSCC. Thus, the status of CASP8 and RIPK3 would be biomarkers to justify the necroptosis pathway as a therapeutic target in HNSCC patients.
Targeting Cell Machinery that Self-Heals Stress: DNA Repair and Autophagy
DNA Repair
Several forms of DNA damage have been shown to activate TP53, including those generated by chemotherapy and radiation. Since chemotherapy- and radiation-induced DSBs are primarily repaired via non-homologous end joining (NHEJ), targeting NHEJ has the potential to sensitize tumor cells to chemotherapy and radiation [71, 72]. NHEJ repair consists of termini recognition, bridging, processing, and ligation of DNA. For example, Ku80 expression is associated with locoregional failure and patient death post radiotherapy in HNSCC [73]. It has been shown that depletion of Ku70 or Ku80 sensitizes pancreatic cancer cells to radiation [74]. Although there are currently no Ku inhibitors, inhibition of Ku proteins with concurrent radiation offers an attractive treatment option, and Ku could serve as a DNA repair-related biomarker of radioresistance in HNSCCs [73]. DNA-dependent protein kinase (DNA-PK) is responsible for phosphorylation of key proteins required for the NHEJ pathway, and when it is inhibited, repair is compromised. Thus, several DNA-PK inhibitors have been developed [75, 76]. In addition, compounds for targeting DNA end-processing have been made to disrupt NHEJ repair following radiation-induced DSBs. Inhibitors of DNA ligase IV have been developed to inhibit the ligation step of NHEJ repair [77, 78].
In response to DNA damage, TP53 activation leads to cell cycle arrest and initiation of DNA repair. Thus, inhibitors of the DNA damage response (DDR) and cell cycle progression have potential to sensitize to chemotherapy and radiation in HNSCC cells with loss of function TP53. This therapeutic vulnerability has been exploited by targeting the cell cycle using small-molecule inhibitors for ATM, ATR, the checkpoint kinase-1/2 (CHK1/2), and the WEE1 kinase [79–83]. The ATR-ATM-CHK1-WEE1 signaling pathway is crucial for the surveillance mechanism of the replication phase and is activated at low thresholds even during the unperturbed S-phase [84–86]. Therefore, cells with replication stress can undergo senescence or apoptosis through inhibition of this pathway. Several inhibitors of this pathway in combination with chemotherapy or radiation have been going into clinical trials with a variety of solid tumors including HNSCC [18].
Autophagy
Autophagy is a cellular recycling and quality control mechanism required for eliminating unnecessary or non-functional cellular organelles and proteins in living cells. Although the basal level of autophagy is thought to contribute to cell survival in cancer cells, prolonged activation of autophagy can cause cell death by self-degradation of cellular components [87]. Thus, autophagy is considered as a double-edged sword in tumorigenesis and resistance to treatments. In radioresistant cells, anti-apoptotic BCL-2 protein is often overexpressed, leading to inhibition of apoptosis [88]. Since Beclin-1 has a BH3-domain and is capable of binding to BCL-2, Beclin-1-dependent cytotoxic autophagy can be inhibited, which may maintain a basal level of autophagy for cell survival, rather than cell death [89]. Moreover, suppression of autophagic cell death, but not apoptosis, might be a reason behind radio-resistance, and thus, prolonged enhancement of autophagy may significantly sensitize to radiotherapy in such tumor cells [90].
On the contrary, cell survival activity of autophagy results in chemoresistance to DNA damaging agents such as cisplatin in several cancers [91, 92]. Moreover, it has been shown that hypoxia suppresses the cytotoxic autophagy activation toward a pro-survival mechanism by inhibiting autophagy-mediated cell death, as observed in HNSCC cells treated with etoposide [87]. In such cases, inhibition of autophagy either by using pharmacological inhibitors or by siRNA-mediated inhibition has shown favorable treatment outcome.
Cancer-associated fibroblasts (CAFs) play important roles in tumorigenesis of tongue squamous cell carcinoma (TSCC). It has been demonstrated that CAFs confer cisplatin resistance of TSCC cells through autophagy activation [93]. Inhibition of autophagy by chloroquine or Beclin-1 siRNA in TSCC cells can increase cisplatin-induced apoptosis and inhibit viability of TSCC cells co-cultured with CAFs, suggesting that autophagy inhibition could be a strategy to overcome chemoresistance of TSCC. In contrast, New et al. [94] demonstrated that CAF-facilitated HNSCC progression can be reduced after blocking CAF autophagy. The levels of secreted IL-6, IL-8, and other cytokines in cell growth-conditioned media were modulated by blockade of CAF autophagy with chloroquine or Beclin-1 siRNA. When HNSCC cells are cocultured with normal fibroblasts, autophagy is upregulated through IL-6, IL-8, and bFGF (basic fibroblast growth factor). In a mouse xenograft model of HNSCC, the inhibition of autophagy with a Vps34 inhibitor, SAR405, enhanced the antitumor efficacy of cisplatin. These results strongly suggest that inhibition of CAF autophagy could overcome cisplatin-resistance of HNSCC.
Targeting Cell Transformation that Escapes Stress: Cancer Stem Cells and Epithelial-Mesenchymal Transition
Ample evidence demonstrates that a small fraction of cells serve as CSCs or cancer initiating cells that are critical for tumor initiation and growth and might be associated with metastasis and tumor recurrence [95, 96]. It has been hypothesized that CSCs are responsible for tumor recurrence or resistance after chemotherapy. Epithelial to mesenchymal transition (EMT) is a process that allows epithelial cells to acquire mesenchymal properties to become migratory and invasive. This is linked to aggressive disease progression in multiple cancers and allows tumor cells to escape apoptosis. The role of EMT in HNSCC has largely not been elucidated, but several studies suggests EMT as a prognostic marker [97]. In HNSCC, TWIST1, a basic helix-loop-helix transcription factor, and BMI1, a polycomb-group protein which regulates gene transcription, act cooperatively to induce EMT and stemness, thereby indicating a role for BMI1 in HNSCC metastasis. TWIST1 has been implicated in resistance to both cisplatin and cetuximab [98]. BMI1+ CSCs drive invasive growth and cervical lymph node metastasis in squamous cell carcinoma. BMI1+ CSCs have increased AP-1 activity and are cisplatin-resistant, and combination therapy that targets BMI1+ CSCs and the tumor bulk yields better outcomes and effectively prevents metastasis [99]. Because high fatality rates in HNSCC are mainly caused by metastases, the use of therapeutics to target EMT has potential to provide better prognosis. A study explored CSC-3436, a flavonoid derivative, to inhibit TWIST-induced EMT, metastases, and tumor-initiated ability through the TWIST/BMI1-Akt/β-catenin pathway [100]. HNSCC patients without TWIST1 or BMI1 expression have a better prognosis compared to patients that express both proteins. Therefore, it is warranted to develop a drug that can target TWIST1 and BMI1 for HNSCC treatment.
Targeting Immunomodulation that Clears Damaged Cells and Adapts to Chronic Stress
Chemotherapy and radiation augment antigen-specific antitumor immune responses. There are various mechanisms involved in this process including; [1] activation and proliferation of tumor-infiltrating lymphocytes, [2] altering chemokines that preferentially recruit cytotoxic T lymphocytes and lead to the upregulation of major histocompatibility complex (MHC) class I expression, [3] release of tumor neoantigens through inflammatory cell death, [4] activation and migration of dendritic cells. Of note, an abscopal effect with higher doses of irradiation in one area results in tumor regression outside the field of radiation [101, 102]. Therefore, numerous clinical trials are ongoing in chemotherapy or radiation in combination with immunotherapy for HNSCC.
Radiation can alter the expression of cell adhesion molecules and increase the density and infiltration of tumor-infiltrating lymphocytes (TILs) involved in tumor cell lysis. For instance, the expression of cell adhesion molecules (e.g., ICAM-1) on the cell surface of endothelium are enhanced by radiation [103]. TILs are emerging as biomarkers in HNSCC as certain TILs such as CD3, CD4, and CD8 T cells are associated with improved prognosis and therapy response. A study observed that HNSCC patients with high expression of CD3 and CD8 T cells in their tumors benefited from longer overall survival, distant-metastasis-free survival, and progression-free survival compared to the patients with low expression of these TILs [104]. Another study reported that HNSCC patients with CD4 and CD8 T cells were associated with improved overall survival [105]. It is also important to note that patients with HPV(+) HNSCC are reported to have higher levels of TILs thus are characterized with longer survival.
Radiation can induce immunogenic cell death (ICD) through damage-associated molecular patterns (DAMPs) in cancer cells. For example, radiation-induced high mobility group protein B1 (HMGB1), a ligand for TLR4, activates the innate immune response and changes the cytokine profile toward an immune stimulatory phenotype [106]. Radiation can also activate antigen-specific anti-tumor immune responses through the upregulation of MHC class I expression [107]. Chemotherapy can increase antigen presentation, leading to increased T cell activation [108, 109]. Furthermore, chemotherapy increases the cytotoxic effects of CTLs and induce ICD [110, 111]. It has also been demonstrated that cisplatin, taxanes, and 5-fluorouracil (5-FU), which are frequently used for HNSCC treatment, decrease myeloid derived suppressor cells (MDSCs) in animal models, which can ultimately enhance anti-tumor immunity [112–114]. Moreover, platinum compounds can increase T-cell activation by dendritic cells (DC) through downregulation by the STAT6 pathway [115].
Based on the diverse immunomodulatory effects of chemotherapy and radiation, the combination of chemo/radiotherapy and immunotherapy is under intensive investigation [102]. Programmed death-1 (PD-1) and its ligand, PD-L1, are immune checkpoints that are exploited in HNSCC to for tumor immune invasion. The anti-PD-1 antibodies, pembrolizumab and nivolumab, were FDA-approved for HNSCC in 2016. These drugs are designed to enhance antitumor immune activity by blocking the PD-1/PD-L1 pathway. Currently, numerous clinical trials are testing the potential synergistic combination of anti-PD-1/PD-L1 with radiotherapy and platinum or cetuximab, or anti-PD-1/PD-L1 used in a neoadjuvant setting [116–118].
HNSCC can develop as a response to chronic inflammation. Inflammatory chemokines, cytokines, and growth factors can contribute tumor proliferation. Chronic inflammation can induce MDSCs, regulatory T cells (Tregs), and tumor-associated macrophages (TAMs), which can impair T cell response, resulting in tumorigenesis while chronic inflammation can suppress T cells and NK cells. The inflammasome, NLRP3 (nod-like receptor protein 3), can initiate inflammatory cell death and promotes tumorigenesis in HNSCC. Studies have demonstrated that NLRP3 is overexpressed in human tissues and that NLRP3 initiates the release of inflammatory cytokines such as IL-1β and IL-18, which are also associated with HNSCC tumorigenesis. When NLRP3 was inhibited in mice, MDSCs, Tregs, and TAMs were decreased, and 5-FU-induced apoptosis was enhanced [119, 120]. Thus, NLRP3 would be a potential target to overcome chemo-resistance.
Dendritic cells (DCs) are the most potent antigen presenting cells and are essential to initiate a primary immune response and migrate to lymphoid organs to present processed antigens to lymphocytes and stimulate immune response. DCs are known to infiltrate solid tumor tissues including HNSCC. EGFR-targeted cetuximab or nimotuzumab antibody dependent cellular cytotoxicity (ADCC) and activated NK cells were both shown to induce natural killer (NK)-DC crosstalk, which resulted in DC maturation and EGFR-specific CD8(+) T-cell priming and enhanced anti-tumor effects [121, 122]. Cetuximab-activated NK cells were also shown to secrete the cytokines IFN-γ, MCP-1, MIP-1β, which inhibited tumor cell proliferation, enhanced antigen presentation, and chemokines that aid in the chemotaxis of T cells. Therefore, a potential therapeutic strategy involves combining cetuximab with an immune cell targeting mAb. CD137, a member of the tumor necrosis factor receptor family, is activated in CD8(+) T cells, NK cells, and DCs. Urelumab, CD137-specific human IgG4 mAb, enhances the efficacy of cetuximab in head and neck cancer patients by enhancing NK-DC crosstalk and inducing DC maturation [123, 124].
Targeting the Oncogenic Pathways that Drive Constitutive Stress for Survival
Epidermal Growth Factor Receptor
Overexpression of epidermal growth factor receptor (EGFR) is commonly associated with tumorigenesis and treatment resistance in HNSCC. EGFR is overexpressed in 90% of HNSCCs and as a consequence, two primary downstream pathways, PI3K/Akt/mTOR and RAS/RAF/MEK/ERK, are activated and contribute to cell proliferation and survival [125]. Cetuximab, a monoclonal antibody targeting EGFR, is one of the few drugs approved by FDA for use in HNSCC, but its response rate is only 13% when used as monotherapy [126] (Figure 3). It is now well-acknowledged that EGFR amplification or overexpression cannot be used for the prediction of cetuximab response in HNSCC.
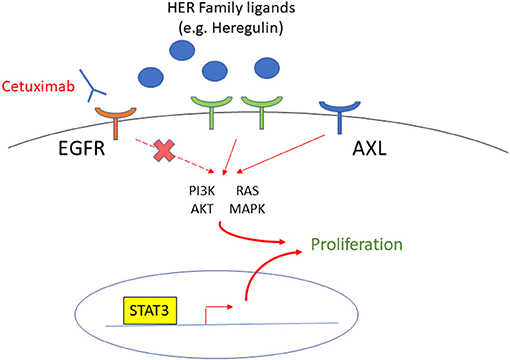
Figure 3. The targets to overcome cetuximab resistance in HNSCC patients. EGFR is overexpressed in most HNSCC, and cetuximab is an FDA approved monoclonal antibody to target EGFR. The HER Family, AXL, and STAT3 are upregulated in cetuximab-resistant HNSCC, and therefore, are targets to overcome cetuximab resistance.
A recent proteogenomic study of 108 HPV(–) HNSCC patient tumors suggests that the EGFR ligands, instead of the receptor itself, are the rate-limiting factors for EGFR pathway activity [127]. For example, heregulin, a ligand of the epidermal growth factor receptor 3 (HER3) of the same family as EGFR, is upregulated in HNSCC cells with acquired cetuximab resistance and confers survival through the AKT signaling. However, cell growth is decreased by Pan-HER inhibition by the small molecule tyrosine kinase inhibitor (TKI), afatinib, which inhibits EGFR, HER2, HER4, and signal transduction of HER3 [128], suggesting that targeting the HER family effectively overcomes resistance to cetuximab. In addition, the receptor tyrosine kinase AXL has been implicated in cancer cell resistance to anti-EGFR TKI [129]. Since AXL is upregulated in both acquired and intrinsically cetuximab-resistant HNSCC cells, this is one of the targets that show the greatest promise for overcoming resistance to cetuximab. Another target of interest is signal transducer and activator of transcription 3 (STAT3). STAT3 has been shown to be upregulated and more active in cetuximab-resistant HNSCC cell lines, and its inhibition decreased cell growth in cell lines resistant to anti-EGFR therapy [130, 131].
The Rb Pathway
Cyclin-dependent kinases (CDKs) are critical regulators of cell cycle progression. The key regulatory pathway of the G1-S transition is cyclin D–CDK4/6–INK4–Rb. When activated by mitogenic signals, both CDK4 and CDK6 kinases can associate with three cyclin Ds (D1, D2 and D3). These active complexes of cyclin D–CDK4/6 phosphorylate and inactivate the retinoblastoma protein (Rb), which promotes the dissociation of the Rb–E2F complex repressive in terms of E2F-dependent transcription. The released E2F then activates the genes necessary for entry into the S phase and DNA replication. The CDK4/6 activity is negatively regulated by p16 INK4A. INK4A inhibits the cyclin D–CDK4/6 activity by directly binding to the CDKs. While loss of Rb is responsible for the G1–S transition in certain types of cancer, the vast majority of cancers have wild-type Rb. These Rb-positive cancers may develop by overexpression or activation of cyclin D–CDK4/6, by loss of negative regulators of cyclin D-CDK4/6 (e.g., INK4A), or by oncogenic signaling pathways that activate cyclin D–CDK4/6 for cell proliferation [132–134].
In HPV(-) HNSCC, the cyclin D–CDK4/6–INK4–Rb pathway is impaired by amplification of CCND1 (20-30% of cases) and inactivation of p16INK4A (approximately 90% of cases) [1, 68]. RB1 is also found to be impaired in ~5% of patients. In HPV(+) HNSCC, E7 protein directly binds to Rb, resulting in proteasomal degradation of Rb to release E2F. Since the CDK4/6 activity is important for the regulation of cell proliferation, the selective inhibition of CDK4/6 appears as a promising therapeutic choice (Figure 4). Several clinical trials to inhibit CDK4/6 activity have been/are being performed in recurrent/metastatic HNSCC. A recent phase II trial with cisplatin-resistant or cetuximab-resistant HPV(-) HNSCC patients treated with a CDK4/6 inhibitor, palbociclib, and cetuximab results in promising activity outcomes [135]. A phase I trial with recurrent/metastatic p16-negative HNSCC patients treated with a CDK4/6/inhibitor, ribociclib, and cetuximab showed safety and efficacy [136]. With a CDK4/6 inhibitor, abemaciclib, phase II trials are underway evaluating the effects of abemaciclib monotherapy or abemaciclib plus nivolumab in HPV(–) HNSCC (NCT03655444, NCT04169074). These studies will elucidate whether CDK4/6 inhibitors play a therapeutic role in the prognostically unfavorable p16/HPV(–) subgroup of patients.
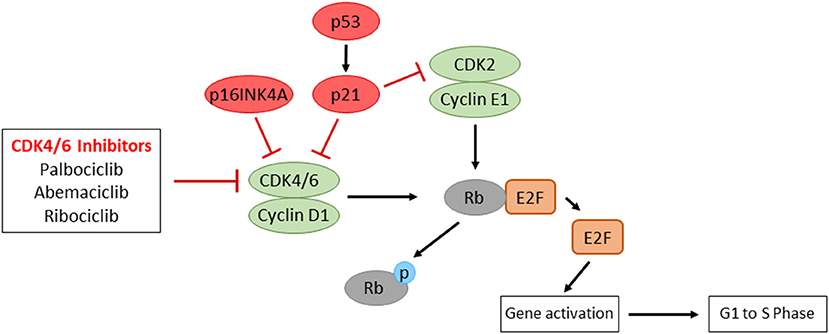
Figure 4. The representative regulatory pathways of cell cycle progression and the mode of action of CDK4/6 inhibitors. Overexpression of Cyclin D1, inactivation of p16INK4A, or inactivated mutations of Rb, which are often observed in HNSCC patients, contribute to uncontrolled cell proliferation. CDK4/6 inhibitors result in the inhibition of E2F transcriptional activity, which show promising prognostic outcomes in HNSCC patients.
Conclusion
Treatment for HNSCC is still generally multimodal, consisting of surgery followed by chemoradiation for oral cavity cancers and primary chemoradiation for pharynx and larynx cancers. It is obvious that HNSCC are able to use a multitude of mechanisms to develop treatment resistance. Thus, one single strategy might not be sufficient, and increased insights to the resistance mechanisms as well as identification of biomarkers will help to design novel and optimized therapeutic strategies, which either direct against tumor cells and/or modulate tumor microenvironment. These issues are also discussed in several recent excellent reviews [3, 137–139].
Author Contributions
TB and HH wrote the initial draft of the manuscript. JAR made substantial revision. All authors contributed to manuscript revision and approved the final version.
Funding
Work from the laboratory of HH is supported by NIH R03 CA235097, R01 CA239706, Massey Cancer Center Pilot Project grant, and Massey Cancer Center Support Grant P30 CA016059.
Conflict of Interest
JAR is a part of Light Switch Bio, LLC., a startup company that is developing light-based therapeutics and diagnostics for commercial applications, including the treatment of cancers.
The remaining authors declare that the research was conducted in the absence of any commercial or financial relationships that could be construed as a potential conflict of interest.
Acknowledgments
Authors acknowledge Drs. Joyce Lloyd and Rita Shiang for support by the VCU Postbaccalaureate Education Research Program and thank Drs. Matthew Hartman and Devraj Basu for their valuable comments.
References
1. Leemans CR, Braakhuis BJ, and Brakenhoff RH. The molecular biology of head and neck cancer. Nat Rev Cancer. (2011) 11:9–22. doi: 10.1038/nrc2982
2. Alsahafi E, Begg K, Amelio I, Raulf N, Lucarelli P, Sauter T, et al. Clinical update on head and neck cancer: molecular biology and ongoing challenges. Cell Death Dis. (2019) 10:540. doi: 10.1038/s41419-019-1769-9
3. Johnson DE, Burtness B, Leemans CR, Lui VWY, Bauman JE, and Grandis JR. Head and neck squamous cell carcinoma. Nat Rev Dis Primers. (2020) 6:92. doi: 10.1038/s41572-020-00224-3
4. Rothenberg SM, and Ellisen LW. The molecular pathogenesis of head and neck squamous cell carcinoma. J Clin Investig. (2012) 122:1951–7. doi: 10.1172/JCI59889
5. Bernier J, Domenge C, Ozsahin M, Matuszewska K, Lefebvre JL, Greiner RH, et al. Postoperative irradiation with or without concomitant chemotherapy for locally advanced head and neck cancer. N Engl J Med. (2004) 350:1945–52. doi: 10.1056/NEJMoa032641
6. Cooper JS, Pajak TF, Forastiere AA, Jacobs J, Campbell BH, Saxman SB, et al. Postoperative concurrent radiotherapy and chemotherapy for high-risk squamous-cell carcinoma of the head and neck. N Engl J Med. (2004) 350:1937–44. doi: 10.1056/NEJMoa032646
7. Vermorken JB, Remenar E, van Herpen C, Gorlia T, Mesia R, Degardin M, et al. Cisplatin, fluorouracil, and docetaxel in unresectable head and neck cancer. N Engl J Med. (2007) 357:1695–704. doi: 10.1056/NEJMoa071028
8. Schultz JD, Bran G, Anders C, Sadick H, Faber A, Hormann K, et al. Induction chemotherapy with TPF (Docetaxel, Carboplatin and Fluorouracil) in the treatment of locally advanced squamous cell carcinoma of the head and neck. Oncol Rep. (2010) 24:1213–6. doi: 10.3892/or_00000974
9. Decatris MP, Sundar S, and O'Byrne KJ. Platinum-based chemotherapy in metastatic breast cancer: current status. Cancer Treat Rev. (2004) 30:53–81. doi: 10.1016/S0305-7372(03)00139-7
10. Wang D, and Lippard SJ. Cellular processing of platinum anticancer drugs. Nat Rev Drug Discov. (2005) 4:307–20. doi: 10.1038/nrd1691
11. Bernier J, Hall EJ, and Giaccia A. Radiation oncology: a century of achievements. Nat Rev Cancer. (2004) 4:737–47. doi: 10.1038/nrc1451
12. Baskar R, Lee KA, Yeo R, and Yeoh KW. Cancer and radiation therapy: current advances and future directions. Int J Med Sci. (2012) 9:193–9. doi: 10.7150/ijms.3635
13. Sanches GLG, da Silva Menezes AS, Santos LI, Duraes CP, Fonseca LL, Baldo MP, et al. Local tissue electrical parameters predict oral mucositis in HNSCC patients: a diagnostic accuracy double-blind, randomized controlled trial. Sci Rep. (2020) 10:9530. doi: 10.1038/s41598-020-66351-9
14. Zuo L, Prather ER, Stetskiv M, Garrison DE, Meade JR, Peace TI, et al. Inflammaging and oxidative stress in human diseases: from molecular mechanisms to novel treatments. Int J Mol Sci. (2019) 20:4472. doi: 10.3390/ijms20184472
15. Vogelstein B, Lane D, and Levine AJ. Surfing the p53 network. Nature. (2000) 408:307–10. doi: 10.1038/35042675
16. Sengupta S, and Harris CC. p53: traffic cop at the crossroads of DNA repair and recombination. Nat Rev Mol Cell Biol. (2005) 6:44–55. doi: 10.1038/nrm1546
17. Stransky N, Egloff AM, Tward AD, Kostic AD, Cibulskis K, Sivachenko A, et al. The mutational landscape of head and neck squamous cell carcinoma. Science. (2011) 333:1157–60. doi: 10.1126/science.1208130
18. Lindemann A, Takahashi H, Patel AA, Osman AA, and Myers JN. Targeting the DNA damage response in OSCC with TP53 mutations. J Dent Res. (2018) 97:635–44. doi: 10.1177/0022034518759068
19. Neskey DM, Osman AA, Ow TJ, Katsonis P, McDonald T, Hicks SC, et al. Evolutionary action score of TP53 identifies high-risk mutations associated with decreased survival and increased distant metastases in head and neck cancer. Cancer Res. (2015) 75:1527–36. doi: 10.1158/0008-5472.CAN-14-2735
20. Valente LJ, Gray DH, Michalak EM, Pinon-Hofbauer J, Egle A, Scott CL, et al. p53 efficiently suppresses tumor development in the complete absence of its cell-cycle inhibitory and proapoptotic effectors p21, Puma, and Noxa. Cell Rep. (2013) 3:1339–45. doi: 10.1016/j.celrep.2013.04.012
21. Brosh R, and Rotter V. When mutants gain new powers: news from the mutant p53 field. Nat Rev Cancer. (2009) 9:701–13. doi: 10.1038/nrc2693
22. Muller PA, and Vousden KH. p53 mutations in cancer. Nat Cell Biol. (2013) 15:2–8. doi: 10.1038/ncb2641
23. Zhou G, Liu Z, and Myers JN. TP53 mutations in head and neck squamous cell carcinoma and their impact on disease progression and treatment response. J Cell Biochem. (2016) 117:2682–92. doi: 10.1002/jcb.25592
24. Song H, Hollstein M, and Xu Y. p53 gain-of-function cancer mutants induce genetic instability by inactivating ATM. Nat Cell Biol. (2007) 9:573–80. doi: 10.1038/ncb1571
25. Marur S, D'Souza G, Westra WH, and Forastiere AA. HPV-associated head and neck cancer: a virus-related cancer epidemic. Lancet Oncol. (2010) 11:781–9. doi: 10.1016/S1470-2045(10)70017-6
26. Riaz N, Morris LG, Lee W, and Chan TA. Unraveling the molecular genetics of head and neck cancer through genome-wide approaches. Genes Dis. (2014) 1:75–86. doi: 10.1016/j.gendis.2014.07.002
27. Butz K, Shahabeddin L, Geisen C, Spitkovsky D, Ullmann A, and Hoppe-Seyler F. Functional p53 protein in human papillomavirus-positive cancer cells. Oncogene. (1995) 10:927–36.
28. Denaro N, Lo Nigro C, Natoli G, Russi EG, Adamo V, and Merlano MC. The role of p53 and MDM2 in head and neck cancer. ISRN Otolaryngol. (2011) 2011:931813. doi: 10.5402/2011/931813
29. Vivenza D, Monteverde M, Lattanzio L, Tonissi F, Astesana V, Denaro N, et al. Correlation of TP53 and MDM2 genotypes and clinical outcome in platinum-treated head and neck cancer patients with more than 10 years' follow-up. Int J Biol Mark. (2016) 31:e183–92. doi: 10.5301/jbm.5000192
30. Bykov VJ, Zache N, Stridh H, Westman J, Bergman J, Selivanova G, et al. PRIMA-1(MET) synergizes with cisplatin to induce tumor cell apoptosis. Oncogene. (2005) 24:3484–91. doi: 10.1038/sj.onc.1208419
31. Bykov VJ, Zhang Q, Zhang M, Ceder S, Abrahmsen L, and Wiman KG. Targeting of mutant p53 and the cellular redox balance by APR-246 as a strategy for efficient cancer therapy. Front Oncol. (2016) 6:21. doi: 10.3389/fonc.2016.00021
32. Omar SI, and Tuszynski J. The molecular mechanism of action of methylene quinuclidinone and its effects on the structure of p53 mutants. Oncotarget. (2018) 9:37137–56. doi: 10.18632/oncotarget.26440
33. Li D, Marchenko ND, and Moll UM. SAHA shows preferential cytotoxicity in mutant p53 cancer cells by destabilizing mutant p53 through inhibition of the HDAC6-Hsp90 chaperone axis. Cell Death Differ. (2011) 18:1904–13. doi: 10.1038/cdd.2011.71
34. Routray S, Sunkavalli A, Swain N, and Shankar AA. Emphasizing on heat shock protein 90's utility in head and neck squamous cell carcinoma treatment. hboxJ Cancer Res Ther. (2013) 9:583–6. doi: 10.4103/0973-1482.126451
35. McLaughlin M, Barker HE, Khan AA, Pedersen M, Dillon M, Mansfield DC, et al. HSP90 inhibition sensitizes head and neck cancer to platin-based chemoradiotherapy by modulation of the DNA damage response resulting in chromosomal fragmentation. BMC Cancer. (2017) 17:86. doi: 10.1186/s12885-017-3084-0
36. Adams JM, and Cory S. The Bcl-2 apoptotic switch in cancer development and therapy. Oncogene. (2007) 26:1324–37. doi: 10.1038/sj.onc.1210220
37. Youle RJ, and Strasser A. The BCL-2 protein family: opposing activities that mediate cell death. Nat Rev Mol Cell Biol. (2008) 9:47–59. doi: 10.1038/nrm2308
38. Chipuk JE, Moldoveanu T, Llambi F, Parsons MJ, and Green DR. The BCL-2 family reunion. Mol Cell. (2010) 37:299–310. doi: 10.1016/j.molcel.2010.01.025
39. Carter RJ, Milani M, Butterworth M, Alotibi A, Harper N, Yedida G, et al. Exploring the potential of BH3 mimetic therapy in squamous cell carcinoma of the head and neck. Cell Death Dis. (2019) 10:912. doi: 10.1038/s41419-019-2150-8
40. Nix P, Cawkwell L, Patmore H, Greenman J, and Stafford N. Bcl-2 expression predicts radiotherapy failure in laryngeal cancer. Br J Cancer. (2005) 92:2185–9. doi: 10.1038/sj.bjc.6602647
41. Maji S, Shriwas O, Samal SK, Priyadarshini M, Rath R, Panda S, et al. STAT3- and GSK3beta-mediated Mcl-1 regulation modulates TPF resistance in oral squamous cell carcinoma. Carcinogenesis. (2019) 40:173–83. doi: 10.1093/carcin/bgy135
42. Montero J, and Letai A. Why do BCL-2 inhibitors work and where should we use them in the clinic? Cell Death Differ. (2018) 25:56–64. doi: 10.1038/cdd.2017.183
43. Strasser A, and Vaux DL. Cell death in the origin and treatment of cancer. Mol Cell. (2020) 78:1045–54. doi: 10.1016/j.molcel.2020.05.014
44. Li R, Zang Y, Li C, Patel NS, Grandis JR, and Johnson DE. ABT-737 synergizes with chemotherapy to kill head and neck squamous cell carcinoma cells via a Noxa-mediated pathway. Mol Pharmacol. (2009) 75:1231–9. doi: 10.1124/mol.108.052969
45. Gilormini M, Malesys C, Armandy E, Manas P, Guy JB, Magne N, et al. Preferential targeting of cancer stem cells in the radiosensitizing effect of ABT-737 on HNSCC. Oncotarget. (2016) 7:16731–44. doi: 10.18632/oncotarget.7744
46. Ow TJ, Fulcher CD, Thomas C, Broin PO, Lopez A, Reyna DE, et al. Optimal targeting of BCL-family proteins in head and neck squamous cell carcinoma requires inhibition of both BCL-xL and MCL-1. Oncotarget. (2019) 10:494–510. doi: 10.18632/oncotarget.26563
47. Britt EL, Raman S, Leek K, Sheehy CH, Kim SW, and Harada H. Combination of fenretinide and ABT-263 induces apoptosis through NOXA for head and neck squamous cell carcinoma treatment. PLoS ONE. (2019) 14:e0219398. doi: 10.1371/journal.pone.0219398
48. Altieri DC. Survivin, versatile modulation of cell division and apoptosis in cancer. Oncogene. (2003) 22:8581–9. doi: 10.1038/sj.onc.1207113
49. Li F, Ackermann EJ, Bennett CF, Rothermel AL, Plescia J, Tognin S, et al. Pleiotropic cell-division defects and apoptosis induced by interference with survivin function. Nat Cell Biol. (1999) 1:461–6. doi: 10.1038/70242
50. Zangemeister-Wittke U, and Simon HU. An IAP in action: the multiple roles of survivin in differentiation, immunity and malignancy. Cell Cycle. (2004) 3:1121–3. doi: 10.4161/cc.3.9.1093
51. Croci DO, Cogno IS, Vittar NB, Salvatierra E, Trajtenberg F, and Podhajcer OL. Silencing survivin gene expression promotes apoptosis of human breast cancer cells through a caspase-independent pathway. J Cell Biochem. (2008) 105:381–90. doi: 10.1002/jcb.21836
52. Roca H, Varsos ZS, Mizutani K, and Pienta KJ. CCL2, survivin and autophagy: new links with implications in human cancer. Autophagy. (2008) 4:969–71. doi: 10.4161/auto.6822
53. Humphry NJ, and Wheatley SP. Survivin inhibits excessive autophagy in cancer cells but does so independently of its interaction with LC3. Biol Open. (2018) 7:bio037374. doi: 10.1242/bio.037374
54. Marioni G, D'Alessandro E, Bertolin A, and Staffieri A. Survivin multifaceted activity in head and neck carcinoma: current evidence and future therapeutic challenges. Acta Otolaryngol. (2010) 130:4–9. doi: 10.3109/00016480902856588
55. Khan Z, Khan AA, Yadav H, Prasad G, and Bisen PS. Survivin, a molecular target for therapeutic interventions in squamous cell carcinoma. Cell Mol Biol Lett. (2017) 22:8. doi: 10.1186/s11658-017-0038-0
56. Khan SA, Burke M, Zhu F, Yang DH, Dubyk C, Mehra R, et al. Survivin expression and impact on head and neck cancer outcomes. Oral Oncol. (2021) 112:105049. doi: 10.1016/j.oraloncology.2020.105049
57. Lippert BM, Knauer SK, Fetz V, Mann W, and Stauber RH. Dynamic survivin in head and neck cancer: molecular mechanism and therapeutic potential. Int J Cancer. (2007) 121:1169–74. doi: 10.1002/ijc.22941
58. Troiano G, Guida A, Aquino G, Botti G, Losito NS, Papagerakis S, et al. Integrative histologic and bioinformatics analysis of birc5/survivin expression in oral squamous cell carcinoma. Int J Mol Sci. (2018) 19:2664. doi: 10.3390/ijms19092664
59. Liu S, Shi L, Yang X, Ye D, Wang T, Dong C, et al. Nuclear survivin promoted by acetylation is associated with the aggressive phenotype of oral squamous cell carcinoma. Cell Cycle. (2017) 16:894–902. doi: 10.1080/15384101.2017.1310352
60. Frassanito MA, Saltarella I, Vinella A, Muzio LL, Pannone G, Fumarulo R, et al. Survivin overexpression in head and neck squamous cell carcinomas as a new therapeutic target (Review). Oncol Rep. (2019) 41:2615–24. doi: 10.3892/or.2019.7082
61. Kumar B, Yadav A, Lang JC, Cipolla MJ, Schmitt AC, Arradaza N, et al. YM155 reverses cisplatin resistance in head and neck cancer by decreasing cytoplasmic survivin levels. Mol Cancer Ther. (2012) 11:1988–98. doi: 10.1158/1535-7163.MCT-12-0167
62. Zhang L, Zhang W, Wang YF, Liu B, Zhang WF, Zhao YF, et al. Dual induction of apoptotic and autophagic cell death by targeting survivin in head neck squamous cell carcinoma. Cell Death Dis. (2015) 6:e1771. doi: 10.1038/cddis.2015.139
63. Cheng SM, Chang YC, Liu CY, Lee JY, Chan HH, Kuo CW, et al. YM155 down-regulates survivin and XIAP, modulates autophagy and induces autophagy-dependent DNA damage in breast cancer cells. Br J Pharmacol. (2015) 172:214–34. doi: 10.1111/bph.12935
64. Satoh T, Okamoto I, Miyazaki M, Morinaga R, Tsuya A, Hasegawa Y, et al. Phase I study of YM155, a novel survivin suppressant, in patients with advanced solid tumors. Clin Cancer Res. (2009) 15:3872–80. doi: 10.1158/1078-0432.CCR-08-1946
65. Giaccone G, Zatloukal P, Roubec J, Floor K, Musil J, Kuta M, et al. Multicenter phase II trial of YM155, a small-molecule suppressor of survivin, in patients with advanced, refractory, non-small-cell lung cancer. J Clin Oncol. (2009) 27:4481–6. doi: 10.1200/JCO.2008.21.1862
66. Kelly RJ, Thomas A, Rajan A, Chun G, Lopez-Chavez A, Szabo E, et al. A phase I/II study of sepantronium bromide (YM155, survivin suppressor) with paclitaxel and carboplatin in patients with advanced non-small-cell lung cancer. Ann Oncol. (2013) 24:2601–6. doi: 10.1093/annonc/mdt249
67. Tummers B, and Green DR. Caspase-8: regulating life and death. Immunol Rev. (2017) 277:76–89. doi: 10.1111/imr.12541
68. Pickering CR, Zhang J, Yoo SY, Bengtsson L, Moorthy S, Neskey DM, et al. Integrative genomic characterization of oral squamous cell carcinoma identifies frequent somatic drivers. Cancer Discov. (2013) 3:770–81. doi: 10.1158/2159-8290.CD-12-0537
69. Fulda S. Promises and Challenges of Smac Mimetics as Cancer Therapeutics. Clin Cancer Res. (2015) 21:5030–6. doi: 10.1158/1078-0432.CCR-15-0365
70. Uzunparmak B, Gao M, Lindemann A, Erikson K, Wang L, Lin E, et al. Caspase-8 loss radiosensitizes head and neck squamous cell carcinoma to SMAC mimetic-induced necroptosis. JCI Insight. (2020) 5:e139837. doi: 10.1172/jci.insight.139837
71. Wang C, Liu XQ, Hou JS, Wang JN, and Huang HZ. Molecular mechanisms of chemoresistance in oral cancer. Chin J Dent Res. (2016) 19:25–33. doi: 10.3290/j.cjdr.a35694
72. Hutchinson MND, Mierzwa M, and D'Silva NJ. Radiation resistance in head and neck squamous cell carcinoma: dire need for an appropriate sensitizer. Oncogene. (2020) 39:3638–49. doi: 10.1038/s41388-020-1250-3
73. Moeller BJ, Yordy JS, Williams MD, Giri U, Raju U, Molkentine DP, et al. DNA repair biomarker profiling of head and neck cancer: Ku80 expression predicts locoregional failure and death following radiotherapy. Clin Cancer Res. (2011) 17:2035–43. doi: 10.1158/1078-0432.CCR-10-2641
74. Li YH, Wang X, Pan Y, Lee DH, Chowdhury D, and Kimmelman AC. Inhibition of non-homologous end joining repair impairs pancreatic cancer growth and enhances radiation response. PLoS ONE. (2012) 7:e39588. doi: 10.1371/journal.pone.0039588
75. Nutley BP, Smith NF, Hayes A, Kelland LR, Brunton L, Golding BT, et al. Preclinical pharmacokinetics and metabolism of a novel prototype DNA-PK inhibitor NU7026. Br J Cancer. (2005) 93:1011–8. doi: 10.1038/sj.bjc.6602823
76. Khan AJ, Misenko SM, Thandoni A, Schiff D, Jhawar SR, Bunting SF, et al. VX-984 is a selective inhibitor of non-homologous end joining, with possible preferential activity in transformed cells. Oncotarget. (2018) 9:25833–41. doi: 10.18632/oncotarget.25383
77. Chen X, Zhong S, Zhu X, Dziegielewska B, Ellenberger T, Wilson GM, et al. Rational design of human DNA ligase inhibitors that target cellular DNA replication and repair. Cancer Res. (2008) 68:3169–77. doi: 10.1158/0008-5472.CAN-07-6636
78. Srivastava M, Nambiar M, Sharma S, Karki SS, Goldsmith G, Hegde M, et al. An inhibitor of non-homologous end-joining abrogates double-strand break repair and impedes cancer progression. Cell. (2012) 151:1474–87. doi: 10.1016/j.cell.2012.11.054
79. Glorieux M, Dok R, and Nuyts S. Novel DNA targeted therapies for head and neck cancers: clinical potential and biomarkers. Oncotarget. (2017) 8:81662–78. doi: 10.18632/oncotarget.20953
80. Gadhikar MA, Sciuto MR, Alves MV, Pickering CR, Osman AA, Neskey DM, et al. Chk1/2 inhibition overcomes the cisplatin resistance of head and neck cancer cells secondary to the loss of functional p53. Mol Cancer Ther. (2013) 12:1860–73. doi: 10.1158/1535-7163.MCT-13-0157
81. Gadhikar MA, Zhang J, Shen L, Rao X, Wang J, Zhao M, et al. CDKN2A/p16 deletion in head and neck cancer cells is associated with CDK2 activation, replication stress, and vulnerability to CHK1 inhibition. Cancer Res. (2018) 78:781–97. doi: 10.1158/0008-5472.CAN-17-2802
82. Mendez E, Rodriguez CP, Kao MC, Raju S, Diab A, Harbison RA, et al. A phase I clinical trial of AZD1775 in combination with neoadjuvant weekly docetaxel and cisplatin before definitive therapy in head and neck squamous cell carcinoma. Clin Cancer Res. (2018) 24:2740–8. doi: 10.1158/1078-0432.CCR-17-3796
83. Kong A, Good J, Kirkham A, Savage J, Mant R, Llewellyn L, et al. Phase I trial of WEE1 inhibition with chemotherapy and radiotherapy as adjuvant treatment, and a window of opportunity trial with cisplatin in patients with head and neck cancer: the WISTERIA trial protocol. BMJ Open. (2020) 10:e033009. doi: 10.1136/bmjopen-2019-033009
84. Bartek J, and Lukas J. Chk1 and Chk2 kinases in checkpoint control and cancer. Cancer Cell. (2003) 3:421–9. doi: 10.1016/S1535-6108(03)00110-7
85. Shechter D, Costanzo V, and Gautier J. ATR and ATM regulate the timing of DNA replication origin firing. Nat Cell Biol. (2004) 6:648–55. doi: 10.1038/ncb1145
86. De Witt Hamer PC, Mir SE, Noske D, Van Noorden CJ, and Wurdinger T. WEE1 kinase targeting combined with DNA-damaging cancer therapy catalyzes mitotic catastrophe. Clin Cancer Res. (2011) 17:4200–7. doi: 10.1158/1078-0432.CCR-10-2537
87. Sannigrahi MK, Singh V, Sharma R, Panda NK, and Khullar M. Role of autophagy in head and neck cancer and therapeutic resistance. Oral Dis. (2015) 21:283–91. doi: 10.1111/odi.12254
88. Condon LT, Ashman JN, Ell SR, Stafford ND, Greenman J, and Cawkwell L. Overexpression of Bcl-2 in squamous cell carcinoma of the larynx: a marker of radioresistance. Int J Cancer. (2002) 100:472–5. doi: 10.1002/ijc.10503
89. Pattingre S, Tassa A, Qu X, Garuti R, Liang XH, Mizushima N, et al. Bcl-2 antiapoptotic proteins inhibit Beclin 1-dependent autophagy. Cell. (2005) 122:927–39. doi: 10.1016/j.cell.2005.07.002
90. Kuwahara Y, Oikawa T, Ochiai Y, Roudkenar MH, Fukumoto M, Shimura T, et al. Enhancement of autophagy is a potential modality for tumors refractory to radiotherapy. Cell Death Dis. (2011) 2:e177. doi: 10.1038/cddis.2011.56
91. Liu D, Yang Y, Liu Q, and Wang J. Inhibition of autophagy by 3-MA potentiates cisplatin-induced apoptosis in esophageal squamous cell carcinoma cells. Med Oncol. (2011) 28:105–11. doi: 10.1007/s12032-009-9397-3
92. Claerhout S, Verschooten L, Van Kelst S, De Vos R, Proby C, Agostinis P, et al. Concomitant inhibition of AKT and autophagy is required for efficient cisplatin-induced apoptosis of metastatic skin carcinoma. Int J Cancer. (2010) 127:2790–803. doi: 10.1002/ijc.25300
93. Liao JK, Zhou B, Zhuang XM, Zhuang PL, Zhang DM, and Chen WL. Cancer-associated fi broblasts confer cisplatin resistance of tongue cancer via autophagy activation. Biomed Pharmacother. (2018) 97:1341–8. doi: 10.1016/j.biopha.2017.11.024
94. New J, Arnold L, Ananth M, Alvi S, Thornton M, Werner L, et al. Secretory autophagy in cancer-associated fibroblasts promotes head and neck cancer progression and offers a novel therapeutic target. Cancer Res. (2017) 77:6679–91. doi: 10.1158/0008-5472.CAN-17-1077
95. Sayed SI, Dwivedi RC, Katna R, Garg A, Pathak KA, Nutting CM, et al. Implications of understanding cancer stem cell (CSC) biology in head and neck squamous cell cancer. Oral Oncol. (2011) 47:237–43. doi: 10.1016/j.oraloncology.2011.02.009
96. Bhaijee F, Pepper DJ, Pitman KT, and Bell D. Cancer stem cells in head and neck squamous cell carcinoma: a review of current knowledge and future applications. Head Neck. (2012) 34:894–9. doi: 10.1002/hed.21801
97. Scanlon CS, Van Tubergen EA, Inglehart RC, and D'Silva NJ. Biomarkers of epithelial-mesenchymal transition in squamous cell carcinoma. J Dent Res. (2013) 92:114–21. doi: 10.1177/0022034512467352
98. Peng F, Zhang H, Du Y, and Tan P. miR-23a promotes cisplatin chemoresistance and protects against cisplatin-induced apoptosis in tongue squamous cell carcinoma cells through Twist. Oncol Rep. (2015) 33:942–50. doi: 10.3892/or.2014.3664
99. Chen D, Wu M, Li Y, Chang I, Yuan Q, Ekimyan-Salvo M, et al. Targeting BMI1(+) cancer stem cells overcomes chemoresistance and inhibits metastases in squamous cell carcinoma. Cell Stem Cell. (2017) 20:621–34. e6. doi: 10.1016/j.stem.2017.02.003
100. Lai YJ, Yu WN, Kuo SC, Ho CT, Hung CM, Way TD, et al. CSC-3436 inhibits TWIST-induced epithelial-mesenchymal transition via the suppression of Twist/Bmi1/Akt pathway in head and neck squamous cell carcinoma. J Cell Physiol. (2019) 234:9118–29. doi: 10.1002/jcp.27589
101. Ngwa W, Irabor OC, Schoenfeld JD, Hesser J, Demaria S, and Formenti SC. Using immunotherapy to boost the abscopal effect. Nat Rev Cancer. (2018) 18:313–22. doi: 10.1038/nrc.2018.6
102. Miyauchi S, Kim SS, Pang J, Gold KA, Gutkind JS, Califano JA, et al. Immune modulation of head and neck squamous cell carcinoma and the tumor microenvironment by conventional therapeutics. Clin Cancer Res. (2019) 25:4211–23. doi: 10.1158/1078-0432.CCR-18-0871
103. Mondini M, Nizard M, Tran T, Mauge L, Loi M, Clemenson C, et al. Synergy of radiotherapy and a cancer vaccine for the treatment of HPV-associated head and neck cancer. Mol Cancer Ther. (2015) 14:1336–45. doi: 10.1158/1535-7163.MCT-14-1015
104. Balermpas P, Michel Y, Wagenblast J, Seitz O, Weiss C, Rodel F, et al. Tumour-infiltrating lymphocytes predict response to definitive chemoradiotherapy in head and neck cancer. Br J Cancer. (2014) 110:501–9. doi: 10.1038/bjc.2013.640
105. Nguyen N, Bellile E, Thomas D, McHugh J, Rozek L, Virani S, et al. Tumor infiltrating lymphocytes and survival in patients with head and neck squamous cell carcinoma. Head Neck. (2016) 38:1074–84. doi: 10.1002/hed.24406
106. Apetoh L, Ghiringhelli F, Tesniere A, Obeid M, Ortiz C, Criollo A, et al. Toll-like receptor 4-dependent contribution of the immune system to anticancer chemotherapy and radiotherapy. Nat Med. (2007) 13:1050–9. doi: 10.1038/nm1622
107. Reits EA, Hodge JW, Herberts CA, Groothuis TA, Chakraborty M, Wansley EK, et al. Radiation modulates the peptide repertoire, enhances MHC class I expression, and induces successful antitumor immunotherapy. J Exp Med. (2006) 203:1259–71. doi: 10.1084/jem.20052494
108. Shurin GV, Tourkova IL, Kaneno R, and Shurin MR. Chemotherapeutic agents in non-cytotoxic concentrations increase antigen presentation by dendritic cells via an IL-12-dependent mechanism. J Immunol. (2009) 183:137–44. doi: 10.4049/jimmunol.0900734
109. Lesterhuis WJ, Punt CJ, Hato SV, Eleveld-Trancikova D, Jansen BJ, Nierkens S, et al. Platinum-based drugs disrupt STAT6-mediated suppression of immune responses against cancer in humans and mice. J Clin Investig. (2011) 121:3100–8. doi: 10.1172/JCI43656
110. Ramakrishnan R, Assudani D, Nagaraj S, Hunter T, Cho HI, Antonia S, et al. Chemotherapy enhances tumor cell susceptibility to CTL-mediated killing during cancer immunotherapy in mice. J Clin Investig. (2010) 120:1111–24. doi: 10.1172/JCI40269
111. Zitvogel L, Kepp O, Senovilla L, Menger L, Chaput N, and Kroemer G. Immunogenic tumor cell death for optimal anticancer therapy: the calreticulin exposure pathway. Clin Cancer Res. (2010) 16:3100–4. doi: 10.1158/1078-0432.CCR-09-2891
112. Vincent J, Mignot G, Chalmin F, Ladoire S, Bruchard M, Chevriaux A, et al. 5-Fluorouracil selectively kills tumor-associated myeloid-derived suppressor cells resulting in enhanced T cell-dependent antitumor immunity. Cancer Res. (2010) 70:3052–61. doi: 10.1158/0008-5472.CAN-09-3690
113. Kodumudi KN, Woan K, Gilvary DL, Sahakian E, Wei S, and Djeu JY. A novel chemoimmunomodulating property of docetaxel: suppression of myeloid-derived suppressor cells in tumor bearers. Clin Cancer Res. (2010) 16:4583–94. doi: 10.1158/1078-0432.CCR-10-0733
114. Huang X, Cui S, and Shu Y. Cisplatin selectively downregulated the frequency and immunoinhibitory function of myeloid-derived suppressor cells in a murine B16 melanoma model. Immunol Res. (2016) 64:160–70. doi: 10.1007/s12026-015-8734-1
115. Hato SV, Khong A, de Vries IJ, and Lesterhuis WJ. Molecular pathways: the immunogenic effects of platinum-based chemotherapeutics. Clin Cancer Res. (2014) 20:2831–7. doi: 10.1158/1078-0432.CCR-13-3141
116. Cohen EEW, Bell RB, Bifulco CB, Burtness B, Gillison ML, Harrington KJ, et al. The Society for Immunotherapy of Cancer consensus statement on immunotherapy for the treatment of squamous cell carcinoma of the head and neck (HNSCC). J Immunother Cancer. (2019) 7:184. doi: 10.1186/s40425-019-0662-5
117. Seliger B, Massa C, Yang B, Bethmann D, Kappler M, Eckert AW, et al. Immune escape mechanisms and their clinical relevance in head and neck squamous cell carcinoma. Int J Mol Sci. (2020) 21:7032. doi: 10.20944/preprints202008.0490.v2
118. Borel C, Jung AC, and Burgy M. Immunotherapy breakthroughs in the treatment of recurrent or metastatic head and neck squamous cell carcinoma. Cancers. (2020) 12:2691. doi: 10.3390/cancers12092691
119. Chen L, Huang CF, Li YC, Deng WW, Mao L, Wu L, et al. Blockage of the NLRP3 inflammasome by MCC950 improves anti-tumor immune responses in head and neck squamous cell carcinoma. Cell Mol Life Sci. (2018) 75:2045–58. doi: 10.1007/s00018-017-2720-9
120. Feng X, Luo Q, Zhang H, Wang H, Chen W, Meng G, et al. The role of NLRP3 inflammasome in 5-fluorouracil resistance of oral squamous cell carcinoma. J Exp Clin Cancer Res. (2017) 36:81. doi: 10.1186/s13046-017-0553-x
121. Srivastava RM, Lee SC, Andrade Filho PA, Lord CA, Jie HB, Davidson HC, et al. Cetuximab-activated natural killer and dendritic cells collaborate to trigger tumor antigen-specific T-cell immunity in head and neck cancer patients. Clin Cancer Res. (2013) 19:1858–72. doi: 10.1158/1078-0432.CCR-12-2426
122. Mazorra Z, Lavastida A, Concha-Benavente F, Valdes A, Srivastava RM, Garcia-Bates TM, et al. Nimotuzumab induces NK cell activation, cytotoxicity, dendritic cell maturation and expansion of EGFR-specific T cells in head and neck cancer patients. Front Pharmacol. (2017) 8:382. doi: 10.3389/fphar.2017.00382
123. Chu DT, Bac ND, Nguyen KH, Tien NLB, Thanh VV, Nga VT, et al. An update on anti-CD137 antibodies in immunotherapies for cancer. Int J Mol Sci. (2019) 20:1822. doi: 10.3390/ijms20081822
124. Srivastava RM, Trivedi S, Concha-Benavente F, Gibson SP, Reeder C, Ferrone S, et al. CD137 Stimulation enhances cetuximab-induced natural killer: dendritic cell priming of antitumor T-cell immunity in patients with head and neck cancer. Clin Cancer Res. (2017) 23:707–16. doi: 10.1158/1078-0432.CCR-16-0879
125. Picon H, and Guddati AK. Mechanisms of resistance in head and neck cancer. Am J Cancer Res. (2020) 10:2742–51. doi: 10.1002/hed.21109
126. Vermorken JB, Trigo J, Hitt R, Koralewski P, Diaz-Rubio E, Rolland F, et al. Open-label, uncontrolled, multicenter phase II study to evaluate the efficacy and toxicity of cetuximab as a single agent in patients with recurrent and/or metastatic squamous cell carcinoma of the head and neck who failed to respond to platinum-based therapy. J Clin Oncol. (2007) 25:2171–7. doi: 10.1200/JCO.2006.06.7447
127. Huang C, Chen L, Savage SR, Eguez RV, Dou Y, Li Y, et al. Proteogenomic insights into the biology and treatment of HPV-negative head and neck squamous cell carcinoma. Cancer Cell. (2021) 39:361–79. doi: 10.1016/j.ccell.2020.12.007
128. Yonesaka K, Tanaka K, Kitano M, Kawakami H, Hayashi H, Takeda M, et al. Aberrant HER3 ligand heregulin-expressing head and neck squamous cell carcinoma is resistant to anti-EGFR antibody cetuximab, but not second-generation EGFR-TKI. Oncogenesis. (2019) 8:54. doi: 10.1038/s41389-019-0164-9
129. Brand TM, Iida M, Stein AP, Corrigan KL, Braverman CM, Luthar N, et al. AXL mediates resistance to cetuximab therapy. Cancer Res. (2014) 74:5152–64. doi: 10.1158/0008-5472.CAN-14-0294
130. Sen M, Joyce S, Panahandeh M, Li C, Thomas SM, Maxwell J, et al. Targeting Stat3 abrogates EGFR inhibitor resistance in cancer. Clin Cancer Res. (2012) 18:4986–96. doi: 10.1158/1078-0432.CCR-12-0792
131. Willey CD, Anderson JC, Trummell HQ, Naji F, de Wijn R, Yang ES, et al. Differential escape mechanisms in cetuximab-resistant head and neck cancer cells. Biochem Biophys Res Commun. (2019) 517:36–42. doi: 10.1016/j.bbrc.2019.06.159
132. Shapiro GI. Cyclin-dependent kinase pathways as targets for cancer treatment. J Clin Oncol. (2006) 24:1770–83. doi: 10.1200/JCO.2005.03.7689
133. Baker SJ, and Reddy EP. CDK4: a key player in the cell cycle, development, and cancer. Genes Cancer. (2012) 3:658–69. doi: 10.1177/1947601913478972
134. Billard-Sandu C, Tao YG, Sablin MP, Dumitrescu G, Billard D, and Deutsch E. CDK4/6 inhibitors in P16/HPV16-negative squamous cell carcinoma of the head and neck. Eur Arch Otorhinolaryngol. (2020) 277:1273–80. doi: 10.1007/s00405-020-05891-2
135. Adkins D, Ley J, Neupane P, Worden F, Sacco AG, Palka K, et al. Palbociclib and cetuximab in platinum-resistant and in cetuximab-resistant human papillomavirus-unrelated head and neck cancer: a multicentre, multigroup, phase 2 trial. Lancet Oncol. (2019) 20:1295–305. doi: 10.1016/S1470-2045(19)30405-X
136. Seront E, Schmitz S, Papier M, van Maanen A, Henry S, Lonchay C, et al. Phase 1 study evaluating the association of the cyclin-dependent kinase 4/6 inhibitor ribociclib and cetuximab in recurrent/metastatic p16-negative squamous cell carcinoma of the head and neck. Front Oncol. (2019) 9:155. doi: 10.3389/fonc.2019.00155
137. Raudenska M, Balvan J, and Masarik M. Cell death in head and neck cancer pathogenesis and treatment. Cell Death Dis. (2021) 12:192. doi: 10.1038/s41419-021-03474-5
138. Ortiz-Cuaran S, Bouaoud J, Karabajakian A, Fayette J, and Saintigny P. Precision medicine approaches to overcome resistance to therapy in head and neck cancers. Front Oncol. (2021) 11:614332. doi: 10.3389/fonc.2021.614332
Keywords: head and neck squamous cell carcinoma, radiotherapy, resistance, chemotherapy, targeted therapy, oxidative stress
Citation: Bos T, Ratti JA and Harada H (2021) Targeting Stress-Response Pathways and Therapeutic Resistance in Head and Neck Cancer. Front. Oral. Health 2:676643. doi: 10.3389/froh.2021.676643
Received: 05 March 2021; Accepted: 28 May 2021;
Published: 23 June 2021.
Edited by:
Andrew Yeudall, Augusta University, United StatesReviewed by:
Daitoku Sakamuro, Augusta University, United StatesAhmad Waseem, Queen Mary University of London, United Kingdom
Copyright © 2021 Bos, Ratti and Harada. This is an open-access article distributed under the terms of the Creative Commons Attribution License (CC BY). The use, distribution or reproduction in other forums is permitted, provided the original author(s) and the copyright owner(s) are credited and that the original publication in this journal is cited, in accordance with accepted academic practice. No use, distribution or reproduction is permitted which does not comply with these terms.
*Correspondence: Hisashi Harada, aGhhcmFkYUB2Y3UuZWR1
†These authors have contributed equally to this work