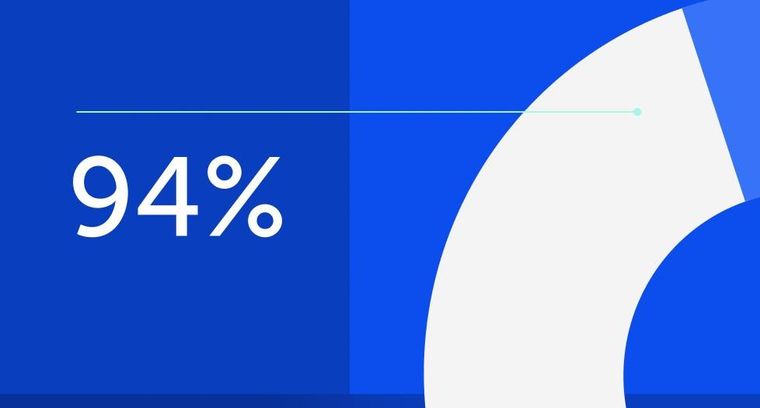
94% of researchers rate our articles as excellent or good
Learn more about the work of our research integrity team to safeguard the quality of each article we publish.
Find out more
REVIEW article
Front. Membr. Sci. Technol., 29 February 2024
Sec. Membrane Modules and Processes
Volume 3 - 2024 | https://doi.org/10.3389/frmst.2024.1361433
This article is part of the Research TopicReviews in Membrane Modules and ProcessesView all 5 articles
Membrane bioreactor (MBR) is an advanced wastewater treatment technology, which has been established for more than 3 decades. In MBRs, membrane separation allows not only rejecting microorganisms/greater-sized molecules but decoupling hydraulic retention time (HRT) and solid retention time (SRT). Low-pressure driven, porous membranes have been widely used in MBRs, but their performances are mainly limited for wastewater reuse applications. Recently, many attempts have been made to combine desalination technologies to advance hybrid MBR processes for wastewater reclamation. Nanofiltration (NF) and reverse osmosis (RO) have been applied with the MBRs to improve effluent quality, and their advantages and challenges have been well reported in terms of rejection efficiency, operational energy, fouling control and recovery of retentate stream. Alternatively, the direct introduction of non-pressurized desalination technologies such as forward osmosis (FO) and membrane distillation (MD) into MBR processes for wastewater reclamation or probably for microbial activity have been considered substantially due to their low energy consumption and excellent rejection efficiency of solid materials. However, several technical limitations still need to be resolved to commercialize hybrid FO- or MD-MBR processes. This paper reviews recent advances of MBR technology integrated with desalination technologies for wastewater reclamation and suggests perspectives to optimize membrane-based hybrid MBR process.
Membrane bioreactor (MBR) is a well-developed membrane-based wastewater process by combining biodegradation and separation to remove organic/inorganic contaminants from wastewater (Xue et al., 2010). The MBR has great advantages in terms of separating hydraulic retention time (HRT) and solid retention time (SRT) while producing excellent effluent (permeate) quality for discharge (Skouteris et al., 2012; Smith et al., 2012). Needs to incorporate reuse of wastewater effluents by consequences of population growth, rapid urbanization and water shortage are growing rapidly for sustainable wastewater management planning (Van de Walle et al., 2023). However, due to strict wastewater reuse standards, the MBR permeate may not meet such requirements, thus requiring additional treatments (Tibi et al., 2019; Kwon et al., 2021).
Value of reclaimed water can be enhanced further by developing membrane based hybrid MBR processes (Liu et al., 2010; Krzeminski et al., 2012). Desalination technologies such as reverse osmosis (RO), nanofiltration (NF), forward osmosis (FO) and membrane distillation (MD) have been established mostly in seawater desalination (Aliyu et al., 2018; Ray et al., 2018; Qasim et al., 2019; Wafi et al., 2019). Here, membrane-based hybrid MBR is classified into conventional MBR integrated with desalination membrane such as NF or RO as post-treatment and a novel hybrid MBR where NF, FO or MD membrane is introduced directly into bioreactor. However, there are many attempts how to combine and tailor desalination technologies with MBRs to provide synergistic impacts for wastewater reuse purposes. Membrane-based hybrid MBR processes offer great benefits because they can produce superior water quality criteria at small footprint and excellent rejection efficiency (Rodríguez-Hernández et al., 2014; Tang et al., 2022). Additionally, combining the MBRs with desalination membranes can intensify the MBR centered process for wastewater reclamation (Krzeminski et al., 2012; Wang et al., 2015b; Burman and Sinha, 2020; Zhu et al., 2022). Nevertheless, the effluent produced by MBR still contains variety of pollutants mostly caused by microbial activities, inorganic species and non-biodegradable fractions (Stoquart et al., 2012; Yan et al., 2018). Therefore, additional treatments for fit-for-purpose should be required to reuse secondary effluent for various reuse purposes. For indirect potable reuse, the existence of refractory (or non-biodegradable) natural organic matter (NOM) which would not be rejected effectively by MF or ultrafiltration (UF) in the MBR needs post-treatment or direct usage of the membranes having much high rejection capability in bioreactor. Organics of potential concern, particularly for indirect potable reuse applications, include pesticides, pharmaceutically-active chemicals and endocrine-disrupting chemicals. For anaerobic MBR (AnMBR), anaerobic effluent (permeate) contains high load of nutrients such as nitrogen and dissolved methane. Depending upon qualities in MBR permeate, desalination technologies are reliable options and thus needs to be tailored to improve not only effluent qualities but also microbial activities (Pearce, 2008; Falizi et al., 2018; Liu et al., 2023).
The MBR requires additional treatment although there is currently no agreement on the best among the current options; NF, RO, FO and MD technology. Mostly, the MBR uses MF or UF membranes for removal of particles, macromolecules and larger microbes. However, there are existences of non-biodegradable organics, effluent organic matter or colloidal fraction consisting of microbial by-products. Macromolecules associated with microbial product such as extracellular polymeric substance (EPS), soluble microbial product (SMP) or NOM, low-molecular weight organic chemicals and inorganic chemicals present in the MBR should also be multiple barriers to indirect potable reuse.
Generally, MBRs displayed excellent removal efficiency for organics and nutrients via biodegradation and membrane rejection, but showed limited rejection of smaller-sized, dissolved substances (such as humic-like substances, low molecule weight organics, heavy metals, micropollutants, etc.) that readily pass through the porous membranes (Judd, 2010). Towards achieving wastewater reclamation, further membrane separation processes, such as NF or RO, have been employed to further purify the MBR permeate. Many research studies listed in Table 1 have proven that the MBR + NF/RO hybrid processes could achieve high efficiency and stable performance, which allows them as the most promising technologies for wastewater reclamation.
Compared to RO processes, the NF processes are capable to provide a higher permeate flux or higher recovery ratio with lower operating pressure and cost (Sert et al., 2016). The NF membrane may be suitable to remove low salinity wastewater where high ion selectivity is not required, inducing monovalent-divalent ion selectivity (Nativ et al., 2021). The NF membranes can provide very high removal of macromolecules that include those associated with EPS, SMP and NOM. However, the RO membrane can remove all inorganic chemicals and low-molecular weight organic chemicals that would pass through the NF membrane. Thus, the MF/UF-MBR + NF process is considered as an alternative approach for wastewater reclamation that can compromise between permeability and selectivity. As indicated in Table 1, the MF/UF-MBR + NF process has been successfully applied to treat municipal wastewater (Jacob et al., 2010; Chon et al., 2011; Chon et al., 2013; Kappel et al., 2014; Chon et al., 2015; Woo et al., 2016; Arola et al., 2017; Hacıfazlıoğlu et al., 2019; Arola et al., 2021; Yacouba et al., 2021) and various types of industrial wastewater (Wintgens et al., 2002; Dialynas and Diamadopoulos, 2009; Andrade et al., 2014; Wang et al., 2015a; Hosseini et al., 2016; Li et al., 2016; Reis et al., 2017; Sert et al., 2017; Lan et al., 2018; Moser et al., 2018; Cinperi et al., 2019; Li et al., 2020a; Reis et al., 2020). In most of the studies, the treated water quality could meet the standards of reclaimed water quality for different uses, such as for urban uses, for industrial uses, for groundwater recharge, and for farmland irrigation (WHO, 2006).
Towards further improving the treated water quality (e.g., producing ultrapure water as fresh water source), a combination of MBR and RO was adopted, which could exhibit superior organic removals compared to MBR + NF systems. The total nitrogen (TN) in the permeates of MBR + RO and MBR + NF were different, possibly due to different rejection efficiencies of nitrogen species, for example, 71.7% for NF90% and 86.2% for BW30 membrane by both NF and RO membranes (Cinperi et al., 2019; Hacıfazlıoğlu et al., 2019). It was also reported that the MBR + RO rejected TN about 10% higher than MBR + NF (Cinperi et al., 2019). Also, there was only 3% higher in TN removal efficiency by MBR + RO than MBR + NF process operated at same transmembrane pressure (TMP) (Hacıfazlıoğlu et al., 2019). Thus, the conventional biological processes such as anaerobic and/or anoxic reactors are generally implemented with an aerobic MBR for elimination of both organics and nutrients (nitrogen and phosphorus) before the MBR permeate is fed into the RO system (Li et al., 2020b). Alternatively, a biofilm MBR is adopted for simultaneous removals of organic and nitrogen and its permeate is further purified by a RO membrane, which shows several advantages such as low energy consumption, small footprint, and limited sludge production (Wang et al., 2019). Whether the RO membranes would experience more fouling potential than the NF membranes in the MBR + NF/RO systems are still under debate. Some research work highlighted that the RO membrane displayed excellent rejection of both cations and anions, which could be responsible for more inorganic fouling of RO membranes (Hacıfazlıoğlu et al., 2019). While other studies pointed out the low molecular weight organic molecules in the MBR permeate could potentially contribute to internal pore blocking of NF membranes, which led to more serious NF membrane fouling, especially at lower water recovery ratios and for more tight NF membranes (Jacob et al., 2010).
Effluent quality from the MBR is critical in biofouling on NF/RO membranes (Al-Amoudi and Lovitt, 2007; Jiang et al., 2017; Matin et al., 2021). Nevertheless, studies to make direct comparison of fouling behaviors between NF and RO membrane are still very limited. Effect of surface roughness on biofouling was more pronounced than operational conditions for both NF and RO membrane (Alturki et al., 2010). Given its relatively high rejection efficiency, biofouling would be formed more preferentially by RO than NF membrane from MBR permeate (Kimura et al., 2009). Moreover, when RO and NF membranes were exposed to the same biofouling condition with Pseudomonas aeruginosa, final cell concentration on RO membrane was lower than NF membrane in the presence of pharmaceutically active compounds (Yang et al., 2018).
Nevertheless, improving the quality of MBR permeate (i.e., RO feed water) is crucial in alleviating RO membrane fouling. A feasible approach via integrating a bioreactor with an external porous NF membrane (i.e., NF-MBR) has been attempted as pretreatment of wastewater for the RO process (Tay et al., 2018; Tay et al., 2020). Apparently, compared to MF/UF-MBR, the NF-MBR produced the permeate with lower organic/inorganic substances, accordingly, the subsequent RO membrane receiving the NF-MBR permeate displayed better performance. Importantly, under the comparable energy consumption scenario, the NF-MBR-RO system could achieve a water recovery ratio up to 90%, higher than that of the MF/UF-MBR-RO system (recovery ratio of 75%).
In this decade, AnMBRs have received great attention due to their high-quality effluent, limited sludge production, and energy production (i.e., methane) (Wu and Kim, 2020). A combination of anaerobic MBR with RO process have been explored to produce high-grade reclaimed water for both non-potable and potable use (Gu et al., 2019; Li et al., 2020b). As stand-alone AnMBRs are ineffective for removing nutrients (e.g., ammonium and phosphate) and RO membranes have limited ammonium rejection efficiency, integration of an additional polishing process with AnMBR + RO for ammonium removal is suggested. For example, an ion exchange process was supplemented to purify AnMBR + RO permeate (Gu et al., 2019); a zeolite adsorption process was employed to treat an AnMBR permeate water before its feeding to a RO process (Li et al., 2020b).
Although NF/RO membranes show great rejection of organics/inorganics derived from the MBR permeate due to their dense membrane natures, the MBR + NF/RO permeate quality could be impacted by the NF/RO membrane property (such as pore size of NF membrane, surface charge, hydrophobicity, etc.). It has been well illustrated that size-exclusion performed a crucial role in rejection of organic substances by NF membranes, i.e., with decreasing NF membrane pore size, more organic substances (such as humic-like substances) could be retained by NF membranes, improving MBR + NF permeate quality (Chon et al., 2013; Chon et al., 2015). While, in terms of micropollutants, size exclusion, hydrophobic/hydrophilic interactions, and electrostatic interactions performed major roles in rejection of micropollutants in NF/RO processes, and the dominance of these mechanisms is associated with characteristics of NF/RO membranes and micropollutants. For example, the molecular weight cut-off of NF membranes governed the removal efficiencies of N-nitrosamines, heavy metals, and metalloids compared to adsorption and formation of membrane fouling on the NF membranes (Chon et al., 2013; Chon et al., 2015). In a study on MBR + NF for leachate wastewater treatment (Wintgens et al., 2002), it was found that the hydrophobicity of the NF membrane could influence the retention of nonylphenol (NP), but did not determine the retention of bisphenol A. With solution-diffusion transport of NP through NF membrane, the transport of NP is constant through the membrane while the water flux increases in more hydrophilic conditions, so that the NP retention increases (Wintgens et al., 2004). In contrast, the MBR + RO displayed excellent mitigation of organics (Jacob et al., 2010; Hacıfazlıoğlu et al., 2019), micropollutants, such as pesticides (de Almeida Lopes et al., 2020), heavy metals (Dialynas and Diamadopoulos, 2009). It should be realized that in certain conditions, the micropollutant removal ratios in the MBR + RO still slightly lower than that in the MBR + activated carbon system (e.g., >95.4% of 2,4-D, atrazine, carbendazim, and diuron removal in MBR + RO vs >98.6% in MBR + activated carbon), implying the importance of RO membrane property in micropollutant mitigation (de Almeida Lopes et al., 2020).
Furthermore, the NF/RO membrane characteristics also influence NF/RO fouling potential. Compared to the NF membrane with relatively larger pore size, the NF membrane with fine pore size had more significant flux decline when they received the same MBR permeate (Jacob et al., 2010; Chon et al., 2013). Similarly, the permeability decline of the RO membrane decreased more significantly than the NF membrane, especially at a higher recovery ratio (Jacob et al., 2010; Hacıfazlıoğlu et al., 2019). However, at a lower recovery ratio, the flux decline of the RO membrane was slower than that of the NF membrane because the low molecular weight molecules in the MBR permeate could cause serious pore blocking of the NF membrane (Jacob et al., 2010).
In the MBR + NF/RO processes, water recovery ratio of NF/RO process is an important parameter in determining productivity of reclaimed water. Increasing recovery ratio in the NF/RO process benefits improving water production, but more hydraulic driving force is needed due to increased osmotic pressure and fouling (cause by gel layer or adsorption) (Andrade et al., 2014; Arola et al., 2021). As a result, increased driving force could facilitate the retained molecules passing through the NF/RO membranes. In particular, such effects could be accelerated when the recovery ratio is above certain threshold level which is about 45% (Andrade et al., 2014). Towards superior NF/RO permeate quality, the NF recovery ratio at 50%-85% and RO recovery ratio at 50%-75% were generally adopted in the reported studies (Table 1). Thus, high volume of NF/RO concentrate with diluted nature is produced. Further post-treatment of NF/RO brines that contain great amounts of nutrients, refractory organic compounds, and inorganic salts is necessary as an efficient membrane concentrate management strategy. To increase water recovery ratio in the MBR + NF system, one solution was to employ the second-stage rotational NF process with high turbulent force by rotating blade on membrane surface to concentrate the first-stage NF brine, which allowed simultaneously improving water production, allowing 300 times NF brine volume reduction, and recovering phosphorus to 86% via spontaneous crystallization of calcium phosphate (Arola et al., 2021).
In the real operation of MBR + NF, the NF concentrate is generally recycled back to the preceding MBR in order to enhance wastewater recycling efficiency and reduced the discharge of NF brine wastewater. While, the recirculation of RO concentrate to the MBR is not practical due to their relatively higher salinity, which generally requires post-treatment before discharge. It is noted that recirculating NF brine back to the bioreactor caused the accumulation of organics, nitrogen, phosphorus and divalent ions in the MBRs, which could potentially affect the system performance and pollutant mitigation (Kappel et al., 2014; Wang et al., 2015a).
First, increasing NF brine recirculation ratio could lead to a decreased of biomass amount, which was attributed to the negative effects of accumulated toxic and refractory organics/inorganics (especially causing increased salinity) on the sludge production in the MBR. Nevertheless, no significant change of microbial community in the MBR was noticed with the recycling of the NF concentrate. Meanwhile, once the microbial community adopted to such operation conditions, the sludge was able to maintain at a relatively constant level (Kappel et al., 2014; Wang et al., 2015a; Li et al., 2020a).
Second, recirculating NF brine back to the MBR may influence the permeate quality of both MBR and MBR + NF. In several documented studies, it was observed that the presence of the recirculation of NF retentate could cause decreasing removal ratios of organics and ammonia in the MBR. Generally, the predominant organics in the MBR permeate are proteins, polysaccharides, and humic-like substances, which can be almost completely rejected by NF membranes (Li et al., 2016). Accordingly, the organic contents in the MBR + NF permeate was negligibly impacted by the NF brine recirculation ratio, showing steady performance of NF membranes. However, it was noticed that recirculating NF brine back to the MBR significantly aggregated the accumulation of nitrate in both the MBR and NF units, leading to more nitrate present in the MBR + NF permeate (Wang et al., 2015a; Li et al., 2016; Li et al., 2020a). Furthermore, recirculating NF retentate increases concentration-polarization layer on membrane due to the concentration of organics in MBR effluent, and this can reduce rejection efficiency by NF membrane. In terms of inorganics, especially monovalent ions that are not readily completely rejected by the NF membranes, their levels in the MBR + NF permeate could significantly increase with elevating the recirculation ratio of the NF retentate back to the MBR (Kappel et al., 2014; Li et al., 2016).
Third, the presence of NF brine recirculation back to the MBR aggregated both MBR and NF membrane fouling. Several studies have highlighted that divalent ions and SMPs brought by NF concentrate are the dominant factors causing the severe membrane fouling in MBRs. In addition, the organic fouling and inorganic scaling on the NF membrane appeared to increase due to relatively high levels of organics/inorganics in the MBR permeate (Kappel et al., 2014; Li et al., 2016; Li et al., 2020a). However, the dominant NF membrane fouling mechanisms in the reported MBR + NF systems were dissimilar, possibly relating to the different operation conditions and wastewater properties. For example, in the MBR + NF system for municipal wastewater reclamation, the predominance of NF membrane fouling was inorganic scaling, which could be alleviated by regulating the pH level of NF feed water (Kappel et al., 2014). While, in another study focusing on MBR + NF for antibiotic processing wastewater treatment, the soluble organic substances (especially fulvic acid-like and humic acid-like compounds) accumulated on the NF membrane determined the NF fouling potential (Li et al., 2016). Under these scenarios, more frequent membrane cleaning was therefore adopted in order to maintain constant permeate productivity, which led to increased operation cost and capital cost (due to shortening NF membrane lifespan). However, the high recovery of reclaimed water brought considerable potential economic benefits, thus, the applicability and feasibility of various MBR + NF operation configurations should be optimized with regards to both operation performance and overall economic benefits (Li et al., 2020a).
It has been well illustrated that (1) variable feed wastewater characteristics and operation conditions in the MBRs could influence on the membrane performance and permeate quality of MBRs (such as organics, nutrients, inorganics) (Wu and Fane, 2012; Meng et al., 2017; Wu and Kim, 2020); (2) The feed water quality of MBRs is a critical factor in determining organic fouling, scaling and biofouling of NF/RO membranes (Al-Amoudi and Lovitt, 2007; Jiang et al., 2017; Matin et al., 2021). Not surprisingly, the MBR operation conditions could have potential impact on the overall MBR + NF/RO performance.
As expected, several studies have revealed that a higher level of organic substances in the MBR permeate reduced NF/RO performance. For example, Reis et al. (2017) pointed out that a yeast-based MBR treating landfill leachate wastewater could benefit producing the permeate with less organics compared to a conventional MBR. As a result, the permeate of the yeast-based MBR + NF was superior than the conventional MBR + NF. Woo et al. (2016) found that the presence of activated carbon (1 g/L) in the MBR could produce the permeate with less organics, allowing the subsequent NF membrane operated at a higher flux compared to that without powdered activated carbon (PAC). Meanwhile, better permeate quality was achieved in the PAC-MBR + NF.
Despite of this fact, the MBRs generally show excellent organic removals from wastewater and produced superior permeate quality. However, the detailed organic compositions (such as hydrophobicity, aromaticity, biopolymer fraction, or carboxylate/acid/base nature of the organics) in the MBR permeate could be strongly dependent upon the feed wastewater conditions and operation conditions of MBRs, which potentially influence the subsequent NF/RO performance (Wu et al., 2013; Farias et al., 2014).
For example, Farias et al. (2014) found that increasing the SRT from 2 to 20 days facilitated alleviating membrane fouling and enhanced organic removal in the MF-MBRs, but increased fouling potential of the subsequent RO membranes. While, Wu et al. (2013) noticed that the MF-MBR operated at a high F/M ratio (0.50 g/g day−1, i.e., SRT at 7 days) had a more serious MF membrane fouling and produced the MBR permeate with greater amounts of organic substances, accordingly, causing a higher RO fouling rate compared to the low F/M ratio (0.17 g/g day−1, i.e., SRT at 45 days). In a recent study on NF-MBR + RO process (Tay et al., 2020), the NF-MBR at a longer SRT (60 days) had greater accumulation of divalent salts in the bioreactors compared to that at a shorter SRT (30 days), leading to more severe inorganic fouling on the NF membrane. As both NF-MBRs displayed similar microbial viability and biodegradation efficiency in terms of organic carbon and ammonia, comparable permeate qualities were achieved in both NF-MBRs. However, a higher RO fouling rate was observed when the RO membrane was fed with the permeate produced by NF-MBR at a higher SRT, implying the effect of organic composites (such as assimilable organic carbon) in the NF-MBR permeate on the RO performance. Notably, the conclusions relating to the influence of SRT on the consequent NF/RO performance were not always in a consistent pattern in these reported studies, possibly due to dissimilar wastewater and membrane nature, reactor configuration, and operating philosophy. Nevertheless, operational parameters of HRT and SRT should influence membrane fouling because they should be involved critically for substrate utilization and cell growth.
Additionally, the dissolved oxygen (DO) level in the MBRs has also been reported to impact the subsequent NF/RO membrane performance. It was found that decreasing the DO level in a moving bed biofilm MBR from 4.0, to 2.5, and to 1.0 mg/L, the fouling rate of the RO membrane fed with the MBR permeate increased from 0.015, to 0.023, and to 0.055 bar/d (Wang et al., 2019). The biopolymers in the MBR permeate was further identified to be correspondent with the RO fouling potential, i.e., the MBR at a higher DO level produced the permeate with less amounts of biopolymers. Similarly, although the aerobic MBR and AnMBR-zeolite column produced the permeate with comparable organic and nitrogen concentrations, the performance of the RO membrane fed with the aerobic MBR permeate was better than that with the AnMBR-zeolite column effluent. It appears that the divalent ions released from the zeolite column could interact with phosphate to accelerate inorganic colloidal fouling on the RO membranes (Li et al., 2020b).
Such observations highlighted that (1) besides organic amounts, the organic/inorganic compositions in the MBR permeate could have a significant influence on the performance of following NF/RO processes, which should be given more attention in future research; (2) during optimization of MBR + NF/RO for wastewater reclamation, the combined effects of the operation conditions of MBRs on both MBR and NF/RO performance need be carefully evaluated in terms of membrane fouling control and economic benefits.
As discussed above, the MBR permeate quality could influence NF/RO membrane performance. Thus, an additional physical/chemical process is suggested to be employed for post-treatment of the MBR permeate before it is fed to NF/RO systems, aiming to remove the substances that could be potential foulants of NF/RO membranes. For example, in the MBR + RO system, installation of cartridge filters (∼5 μm) in the RO feed line and recycled RO concentrate line significantly mitigated RO fouling from 1.65 to 0.30 bar/day, which was majorly attributed to reduced accumulation of organic substances on the RO membranes by reducing its dry weight to 128.6 from 282 mg/m2. day (Wu et al., 2013). In addition, a NF process was suggested to treat MBR permeate before it was fed into the RO process in a study on industrial wastewater treatment. The results confirmed that with NF pretreatment of MBR permeate (significantly rejecting organics and divalent ions), the RO membrane could be operated at a relatively higher flux, 97 and 86 L/m2. hr (LMH) for NF and MBR, respectively. Meanwhile, MBR + NF + RO could produce the superior permeate quality than the MBR + RO compared to single MBR in terms of total dissolved (17.1 vs 29.6 mg/L as total dissolved solids (TDS)) and TN (2.8 vs 3.8 mg/L) (Parlar et al., 2019).
Furthermore, in a hybrid MBR + NF process in treating municipal wastewater, additional ozonation of MBR permeate before its feeding into the NF process could alleviate 40% of NF membrane fouling. This was attributed to the oxidation of the protein-like, fulvic and humic-like substances in the MBR permeate via decomposing their carbon-carbon double bonds and aromatic rings, which formed more hydrophilic nature compounds with less propensity to induce irreversible fouling (Yacouba et al., 2021). Alternatively, employing UV/H2O2 to treat MBR permeate benefited for mineralization of organics in the MBR permeate. Accordingly, when the MBR-UV/H2O2 effluent was fed into the NF process, less NF membrane fouling and superior NF permeate quality were observed compared to that fed with the MBR permeate. Importantly, the integration of H2O2/UV with the MBR-NF process saved 20% of capital and operation cost respectively due to reduced NF membrane cleaning frequency and prolonging NF membrane lifespan (Moser et al., 2018).
Nevertheless, long-term addition of oxidant in MBR permeate as pretreatment may reduce membrane performances depending upon ozone dose and membrane materials. In the MBR-ozonation hybrid process, the target ozone dose needed to be maintained below 5.0 mg/L to prevent membrane damage (Sun et al., 2018). When the polyethersulfone (PES)-based membrane such as NP10 and polyamide (PA)-based membrane such as NF90and NF270 membrane were exposed to 10 mg/L of dissolved ozone at pH 7 for 1 h, there was no significant degradation observed with NP10 membrane while PA-based NF membranes were damaged severely (Ouali et al., 2021).
In case of the MBR coupled with UV/H2O2 process, the limitation of the H2O2 concentration recirculated to the MBR step was reported as 3–7 mg/L to prevent not only microbial activity but also membrane damage (Laera et al., 2012). As PA-TFC composite NF membrane was exposed to 4 mg/L of H2O2 during 24 h, the rejection of micropollutants were reduced slightly due to membrane damage (Lin et al., 2021).
Generally, porous membranes such as MF or UF, are employed in MBRs to retain active biomass effectively. To obtain high permeate quality for wastewater reclamation, feasibility of NF membrane is also explored by directly combining NF membrane with bioreactor. Since physicochemical separation employed by the NF membrane is dominated by both size exclusion and solution-diffusion mechanisms, various contaminants such as colloidal and organic compounds can be rejected effectively (Choi et al., 2002). However, monovalent ions or nanoscale- organics present in wastewater are difficult to be retained by NF membrane, accordingly, they may not be accumulated easily in bioreactors. This enables the NF-MBR to be operated under relatively lower suction pressures (than RO membranes), while preventing the inhibition against microbial growth and activity (Choi et al., 2002). Additionally, the NF membrane in the MBR process did not provide any adverse impact on biological conversion of NH4-N (Cao et al., 2022).
However, NF-MBRs suffer low permeability nature, e.g., ∼0.01 to 0.04 LMH of permeate flux was reported in the submerged NF-MBR with commercial NF membrane (Cao et al., 2022). To enhance membrane permeability, novel NF membranes with tailored properties have been adopted in the NF-MBR. For example, the loosely structured hollow-fiber membrane was applied in the NF-MBR, allowing to be operated at ∼2 LMH with more than 95% COD removal efficiency (Cao et al., 2022). In a moving bed biofilm reactor with a side-streamed NF membrane module (using layer-by-layer polyethersulfone NF membrane) under ∼22 h of HRT, about 10 LMH of set-point flux was achieved (Tay et al., 2018). The new NF membrane with high permeability and selectivity should be developed for MBR. With the side-stream membrane configuration, generation of the concentrate stream from the NF membrane that returns into bioreactor is not avoidable, requiring additional treatment unit such as chemical oxidation or electrodialysis to mitigate the concentrate load and protect microbial activity (Kappel et al., 2014). Research works need to be directed into the NF-MBR to maintain desired cell concentration in MBR without wash-out and potential inhibitory effect caused by transportation of monovalent ions or nano-scaled organic compounds on microbial activity.
Despite the advances in NF or RO that promise to increase MBR permeate quality, it consumes high operational energy. In recent years, combining a FO membrane process with a bioreactor, termed as FO-MBR or osmotic MBR has been emerging as a result of recognition of FO to provide excellent permeate (effluent) qualities and reduction in operational cost over traditional high-pressure driven membrane processes (Holloway et al., 2015; Wang et al., 2016; Pathak et al., 2021; Moktadir et al., 2023). The FO filtration uses a semipermeable membrane and is driven by natural osmotic pressure generated by draw solution to produce permeate from feed solutions (Ndiaye et al., 2021). Since the FO filtration relies on the osmotic pressure caused by concentration gradient through membrane materials, an energy demand required for TMP to operate the system is very low. As filtration progresses with time, however, the draw solution becomes diluted, so that both feed and bulk solution in the MBR should be concentrated subsequently. The waste product concentrated by FO membrane is easy to be disposed or reused downstream as useful energy and resource source. Since the wastewater is transported from a feed side toward draw solution, it can be recovered for the production of high quality of permeate given that draw solution regeneration step is integrated, for example, a double-barrier membrane (Holloway et al., 2014; Lutchmiah et al., 2014; Im et al., 2020). Nevertheless, the purity of the permeate depends on the rejection features of FO membranes and the separation effectiveness of permeate water from draw solution strongly. Depending upon the characteristics of the effluent stream and the effectiveness of the entire treatment train, the extracted permeate can also be reused for various purposes such as cooling, rinsing or even irrigation and drinking water. In particular, this unique characteristics in FO membrane process is also beneficial for Zero Liquid Discharge (ZLD) waste disposal system because it leaves much less water to be removed by evaporators, so that energy demand can be further reduced significantly. Here, the product water extracted by a FO membrane can be reused for other purposes such as process water for industrial manufactures (Im et al., 2018). The FO-MBR shows acceptable permeate flux and superior removal efficiency of organic contaminants as well as other emerging contaminants without applying external pressure (Zhu and Li, 2013; Jang et al., 2018; Sivodia and Sinha, 2023). Therefore, the FO-MBR should have a great potential to replace the conventional MBR processes for wastewater reclamation conditioning that sources suitable for draw solution, i.e., seawater can be obtained easily nearby the MBR plants (Arnaldos et al., 2023).
For the FO-MBR process, there are two functions designated by FO membranes such as wastewater concentration and water recovery or extraction (Wu et al., 2021). The wastewater concentrated by the FO process should be particularly helpful in anaerobic MBR systems because it can retain anaerobes completely in anaerobic bioreactor (Chen et al., 2014; Anjum et al., 2021). Additionally, the FO membrane process has been employed for the treatment of AnMBR effluents containing high nutrient loadings such as ammonia nitrogen as they are applied as post-treatment (Kwon et al., 2020). Although biodegradation and/or biotransformation is the main mechanism for trace organic contaminants removal by both aerobic MBR (AeMBR) and AnMBR, the removal of some specific materials can be different since the microbial community differs between the two (Liu et al., 2020). While there is no significant difference in permeate qualities produced by desalination technologies with AnMBR, some portion of methane produced can be dissolved in permeate particularly AnMBR treats domestic sewage. In terms of water extraction, the FO membranes can be applied to separate the water from MBR effluents or directly from bioreactor. Nevertheless, the regeneration step for the recovery of draw solution should be required, and this will be the bottleneck to be overcome for FO-MBR processes (Qiu et al., 2016; Song et al., 2020).
The selection of draw solution acts as an important role in FO-MBR processes because it can determine not only permeate flux but also water quality as well as microbial activities. Especially, the reverse salt transport may increase the amount of salt in bulk suspension of MBRs, which would provide adverse effects on microbial community and bacterial growth (Nawaz et al., 2013; Nawaz et al., 2016). Subsequently, the salt concentration in the MBR increased by the FO membrane varies depending upon the growth rate of biomass, particle size distribution and sludge filterability (Nguyen et al., 2015; Adnan et al., 2019). Therefore, more studies are needed to understand effect of draw solution concentrated by FO membrane in bioreactor performance, such as microbial activities. Also, proper selection of draw solution should be suggested to confirm operational energy.
Like other membrane processes, the membrane fouling observed with FO membrane is a necessary phenomenon because biofouling can be formed particularly on the surface of FO membrane (Qin et al., 2010; Bao et al., 2019). In fact, the FO membrane can be fouled more easily than other types of high pressure-driven membrane due to relatively lower crossflow velocity on membrane surface. Furthermore, the fouling rate on FO membrane can be severe as it is combined with bioreactor because both organic/inorganic loadings increase gradually by FO filtration (Parida and Ng, 2013; Aftab et al., 2017). High organic loading can increase microbial by-products such as EPS while reducing sludge dewaterability (Aftab et al., 2015). A recent study (Olives et al., 2023) on granular sludge based AnMBR proved that fouling occurred less frequently than MF. It was also confirmed that the change in salinity within the MBR caused the draw solution’s reverse salt flux. The use of FO resulted in complete and very high purity in effluent, but the presence of MF was necessary for the removal of salts. A method combining thermal osmotic backwashing and air scouring and osmotic backwashing were evaluated as non-chemical methods to remove biological contamination of FO-MBR (Satterfield et al., 2021). Considering energy efficiency, optimization of backwashing period and frequency was found to be the most economically efficient way to remove FO-MBR fouling.
MD is the thermally driven hybrid membrane process. The MD process could concentrate the non-volatile impurities in the feed solution and transport volatile matters to the permeate solution though the hydrophobic pores of membranes. The schematic diagram of the MD process for wastewater treatment is presented in Figure 1. Due to the relatively lower fouling potential than that of pressurized-driven membrane process, the MD process is combined with a bioreactor has been applied as main or side streams for various wastewater treatment and water reuse (Julian et al., 2022; Kharraz et al., 2022), treatment of industrial wastewaters (petrochemical (Santos et al., 2020), flue gas (Li et al., 2021), desulfurization (Zheng et al., 2022), coking (Guo et al., 2022), textile (Elcik et al., 2021), seawater brine (Kim et al., 2019) and radioactive (Jia et al., 2021)) as well as resource recovery (Julian et al., 2022; Kharraz et al., 2022). In the MD-MBR, the MD process could be used as final barrier in the water treatment process because it allows the transports for the volatile matters such as water. However, feed solution containing volatile impurities such as nitrogen (ammonia) sometimes required a pH control to regulate ammonia transport through membrane (Tun et al., 2016; Lee et al., 2021b).
As mentioned, the main purpose of MBR process is to separate biomass and improve permeate quality by controlling HRT and SRT independently. When the MD process is combined with MBR in municipal wastewater treatment, the deionized water could be produced by municipal wastewater (Tun et al., 2016; Song et al., 2018; Lee et al., 2021a; Simoni et al., 2021; Dow et al., 2022). Like pressure-driven membrane process, the MD membrane experiences severe fouling caused by particulates (Choudhury et al., 2019), organic compounds (Guo et al., 2019; Elcik et al., 2021; Jeong et al., 2021), inorganic compounds (Kim et al., 2019; Kim et al., 2022), and microorganisms (Zheng et al., 2022). Additionally, the fouling rate can be accelerated by the membrane wetting (Choudhury et al., 2019; Kim et al., 2019; Madalosso et al., 2022) during long-term operation of MD membrane. To improve the membrane permeability of MD membrane or antifouling capability, the modified MD membrane materials have been adopted (Günay et al., 2023). The performance of MD membrane could be enhanced by fabricating the membranes with proper membrane pore size and thickness to prevent membrane wetting or heat loss across the membrane (Tibi et al., 2020; Julian et al., 2022; Kharraz et al., 2022). It is reported that higher hydrophobicity and porosity of the MD membrane results in higher rejection with organic and inorganic species (Tibi et al., 2020; Julian et al., 2022; Kharraz et al., 2022). The PVDF, polypropylene (PP), and polytetrafluoroethylene (PTFE) polymers have been used to fabricate a flat-sheet or hollow fiber type MD membranes, which have been used in various MD module configurations such as direct contact membrane distillation (DCMD), air-gap membrane distillation (AGMD), solar driven membrane distillation (SGMD), and vacuum membrane distillation (VMD) (Tibi et al., 2020; Julian et al., 2022; Kharraz et al., 2022). Specifically, the aramid layer was suggested to be coated on the PVDF membrane to enhance chemical resistances (e.g., harsh pH conditions) (Ji et al., 2021). Moreover, the MD membrane can be modified for anti-wetting (Madalosso et al., 2022), self-cleaning efficiency (Yan et al., 2022), and photothermal property (Lee et al., 2022) to achieve its high sustainability for hybrid MD-MBR processes. Nevertheless, the techno-economic analysis should be conducted for hybrid MD-MBRs to accelerate commercialization. Operational energy required for MD processes may be still high due to maintaining required temperature driving force, but if the renewable energy or waste heat is available from anaerobic bioreactor, for example, the energy demand to operate the MD-MBRs can decrease significantly. As reported in a recent study of pilot-scale MD-MBR (treating 10 m3/day wastewater) using single-solar power panel without and with the heat pump, the costs were expected to be 56.5 and 87.3 $/m3 produced water respectively, considering the gain output ratio (GOR) of 0.5–0.8 (Choi et al., 2022). It has been well documented, for the multi-staged MD system, the water production cost was from 6.1 to 7.4 $/m3 with reduction of GOR from 4.6 to 4.4 as the number of stages increased from 8 to 16 (Dudchenko et al., 2021). Both evaporation efficiencies (48%-69%) and the GOR (2.0-3.4) of the multi-stage MD system could be increased with increasing wind speed (8-11 m/s). Moreover, waste heat can be produced by the wind turbine, which is available for energy source to operate MD system (Memon et al., 2022). As a result, it is worthwhile to develop MD-MBR processes to simultaneously capture renewable energy and perform wastewater reclamation.
This review describes the state-of-art of the MBR integrated with desalination technologies to improve effluent quality and membrane performance as well as optimize it for wastewater reuse applications. Applications of RO, NF, FO and MD to improve MBR based process for wastewater reclamation were reviewed critically. Although NF/RO can provide high permeate quality, this should be impacted by membrane materials strongly. Increasing the ratio of water recovery in NF/RO process enhances productivity of reclaimed water. However, membrane fouling is necessary phenomena and thus it needs to be controlled. Recirculating NF/RO residual to bioreactor may provide adverse effect on both MBR permeability and NF or RO permeability, so that recovery ratio needs to be optimized. Both HRT and SRT are important operational parameters to determine both MBR performance and desalination performance. Nevertheless, the effectiveness to desalination membrane varies strongly depending upon wastewater characteristics, membrane materials and reactor configurations. The FO membrane has a great potential because it can reduce energy demand in hybrid MBR process significantly. However, increasing salt content in bulk solution of MBR due to dilution of draw solution may reduce microbial activity. The use of MD membrane has great potential to enhance the value of reclaimed water in hybrid MBR because it can use any potential waste heat source available. Nevertheless, the long-term performance of MD membrane in bioreactor needs to be evaluated.
JK: Conceptualization, Funding acquisition, Writing–original draft, Writing–review and editing. BW: Writing–original draft, Writing–review and editing. SaJ: Writing–original draft. SeJ: Writing–original draft. MK: Resources, Writing–review and editing.
The author(s) declare financial support was received for the research, authorship, and/or publication of this article. This study was supported by National Research Foundation of Korea (NRF) grant funded by the Korean Government (Ministry of Science and ICT) (2022R1A4A3029607, 2022R1A2C2010993).
The authors declare that the research was conducted in the absence of any commercial or financial relationships that could be construed as a potential conflict of interest.
The author(s) declared that they were an editorial board member of Frontiers, at the time of submission. This had no impact on the peer review process and the final decision.
All claims expressed in this article are solely those of the authors and do not necessarily represent those of their affiliated organizations, or those of the publisher, the editors and the reviewers. Any product that may be evaluated in this article, or claim that may be made by its manufacturer, is not guaranteed or endorsed by the publisher.
Adnan, M., Khan, S. J., Manzoor, K., and Hankins, N. P. (2019). Performance evaluation of fertilizer draw solutions for forward osmosis membrane bioreactor treating domestic wastewater. Process Saf. Environ. Prot. 127, 133–140. doi:10.1016/j.psep.2019.05.006
Aftab, B., Khan, S. J., Maqbool, T., and Hankins, N. P. (2015). High strength domestic wastewater treatment with submerged forward osmosis membrane bioreactor. Water Sci. Technol. 72 (1), 141–149. doi:10.2166/wst.2015.195
Aftab, B., Khan, S. J., Maqbool, T., and Hankins, N. P. (2017). Heavy metals removal by osmotic membrane bioreactor (OMBR) and their effect on sludge properties. Desalination 403, 117–127. doi:10.1016/j.desal.2016.07.003
Al-Amoudi, A., and Lovitt, R. W. (2007). Fouling strategies and the cleaning system of NF membranes and factors affecting cleaning efficiency. J. Membr. Sci. 303 (1-2), 4–28. doi:10.1016/j.memsci.2007.06.002
Aliyu, U. M., Rathilal, S., and Isa, Y. M. (2018). Membrane desalination technologies in water treatment: a review. Water Pract. Technol. 13 (4), 738–752. doi:10.2166/wpt.2018.084
Alturki, A. A., Tadkaew, N., McDonald, J. A., Khan, S. J., Price, W. E., and Nghiem, L. D. (2010). Combining MBR and NF/RO membrane filtration for the removal of trace organics in indirect potable water reuse applications. J. Membr. Sci. 365 (1-2), 206–215. doi:10.1016/j.memsci.2010.09.008
Andrade, L., Mendes, F., Espindola, J., and Amaral, M. (2014). Nanofiltration as tertiary treatment for the reuse of dairy wastewater treated by membrane bioreactor. Sep. Purif. Technol. 126, 21–29. doi:10.1016/j.seppur.2014.01.056
Anjum, F., Khan, I. M., Kim, J., Aslam, M., Blandin, G., Heran, M., et al. (2021). Trends and progress in AnMBR for domestic wastewater treatment and their impacts on process efficiency and membrane fouling. Environ. Technol. Innovation 21, 101204. doi:10.1016/j.eti.2020.101204
Arnaldos, M., Torre, T., Rodriguez, C., and Malfeito, J. (2023). “Feasibility evaluation of the FO-MBR process for wastewater reclamation,” in IDA world congress on desalination and water reuse (San Diego CA: Society of Petroleum Engineers).
Arola, K., Hatakka, H., Mänttäri, M., and Kallioinen, M. (2017). Novel process concept alternatives for improved removal of micropollutants in wastewater treatment. Sep. Purif. Technol. 186, 333–341. doi:10.1016/j.seppur.2017.06.019
Arola, K., Mänttäri, M., and Kallioinen, M. (2021). Two-stage nanofiltration for purification of membrane bioreactor treated municipal wastewater–minimization of concentrate volume and simultaneous recovery of phosphorus. Sep. Purif. Technol. 256, 117255. doi:10.1016/j.seppur.2020.117255
Bao, X., Wu, Q., Tian, J., Shi, W., Wang, W., Zhang, Z., et al. (2019). Fouling mechanism of forward osmosis membrane in domestic wastewater concentration: role of substrate structures. Chem. Eng. J. 370, 262–273. doi:10.1016/j.cej.2019.03.174
Burman, I., and Sinha, A. (2020). Anaerobic hybrid membrane bioreactor for treatment of synthetic leachate: impact of organic loading rate and sludge fractions on membrane fouling. Waste Manag. 108, 41–50. doi:10.1016/j.wasman.2020.04.031
Cao, L., Zhang, Y., Ni, L., and Feng, X. (2022). A novel loosely structured nanofiltration membrane bioreactor for wastewater treatment: process performance and membrane fouling. J. Membr. Sci. 644, 120128. doi:10.1016/j.memsci.2021.120128
Chen, L., Gu, Y., Cao, C., Zhang, J., Ng, J.-W., and Tang, C. (2014). Performance of a submerged anaerobic membrane bioreactor with forward osmosis membrane for low-strength wastewater treatment. Water Res. 50, 114–123. doi:10.1016/j.watres.2013.12.009
Choi, J., Cho, J., Shin, J., Cha, H., Jung, J., and Song, K. G. (2022). Performance and economic analysis of a solar membrane distillation pilot plant under various operating conditions. Energy Convers. Manag. 268, 115991. doi:10.1016/j.enconman.2022.115991
Choi, J.-H., Dockko, S., Fukushi, K., and Yamamoto, K. (2002). A novel application of a submerged nanofiltration membrane bioreactor (NF MBR) for wastewater treatment. Desalination 146 (1-3), 413–420. doi:10.1016/s0011-9164(02)00524-6
Chon, K., Cho, J., and Shon, H. K. (2013). Fouling characteristics of a membrane bioreactor and nanofiltration hybrid system for municipal wastewater reclamation. Bioresour. Technol. 130, 239–247. doi:10.1016/j.biortech.2012.12.007
Chon, K., Kim, S. H., and Cho, J. (2015). Removal of N-nitrosamines in a membrane bioreactor and nanofiltration hybrid system for municipal wastewater reclamation: process efficiency and mechanisms. Bioresour. Technol. 190, 499–507. doi:10.1016/j.biortech.2015.02.080
Chon, K., Sarp, S., Lee, S., Lee, J.-H., Lopez-Ramirez, J., and Cho, J. (2011). Evaluation of a membrane bioreactor and nanofiltration for municipal wastewater reclamation: trace contaminant control and fouling mitigation. Desalination 272 (1-3), 128–134. doi:10.1016/j.desal.2011.01.002
Choudhury, M. R., Anwar, N., Jassby, D., and Rahaman, M. S. (2019). Fouling and wetting in the membrane distillation driven wastewater reclamation process–A review. Adv. colloid interface Sci. 269, 370–399. doi:10.1016/j.cis.2019.04.008
Cinperi, N. C., Ozturk, E., Yigit, N. O., and Kitis, M. (2019). Treatment of woolen textile wastewater using membrane bioreactor, nanofiltration and reverse osmosis for reuse in production processes. J. Clean. Prod. 223, 837–848. doi:10.1016/j.jclepro.2019.03.166
de Almeida Lopes, T. S., Hessler, R., Bohner, C., Junior, G. B. A., and de Sena, R. F. (2020). Pesticides removal from industrial wastewater by a membrane bioreactor and post-treatment with either activated carbon, reverse osmosis or ozonation. J. Environ. Chem. Eng. 8 (6), 104538. doi:10.1016/j.jece.2020.104538
Dialynas, E., and Diamadopoulos, E. (2009). Integration of a membrane bioreactor coupled with reverse osmosis for advanced treatment of municipal wastewater. Desalination 238 (1-3), 302–311. doi:10.1016/j.desal.2008.01.046
Dow, N., Saldin, T. F., Duke, M., and Yang, X. (2022). Pilot demonstration of nitrogen removal from municipal wastewater by vacuum membrane distillation. J. Water Process Eng. 47, 102726. doi:10.1016/j.jwpe.2022.102726
Dudchenko, A. V., Bartholomew, T. V., and Mauter, M. S. (2021). High-impact innovations for high-salinity membrane desalination. Proc. Natl. Acad. Sci. 118 (37), e2022196118. doi:10.1073/pnas.2022196118
Elcik, H., Fortunato, L., Vrouwenvelder, J. S., and Ghaffour, N. (2021). Real-time membrane fouling analysis for the assessment of reclamation potential of textile wastewater processed by membrane distillation. J. Water Process Eng. 43, 102296. doi:10.1016/j.jwpe.2021.102296
Falizi, N. J., Hacıfazlıoğlu, M. C., Parlar, İ., Kabay, N., Pek, T. Ö., and Yüksel, M. (2018). Evaluation of MBR treated industrial wastewater quality before and after desalination by NF and RO processes for agricultural reuse. J. water process Eng. 22, 103–108. doi:10.1016/j.jwpe.2018.01.015
Farias, E. L., Howe, K. J., and Thomson, B. M. (2014). Effect of membrane bioreactor solids retention time on reverse osmosis membrane fouling for wastewater reuse. Water Res. 49, 53–61. doi:10.1016/j.watres.2013.11.006
Gu, J., Liu, H., Wang, S., Zhang, M., and Liu, Y. (2019). An innovative anaerobic MBR-reverse osmosis-ion exchange process for energy-efficient reclamation of municipal wastewater to NEWater-like product water. J. Clean. Prod. 230, 1287–1293. doi:10.1016/j.jclepro.2019.05.198
Günay, M. G., Kemerli, U., Karaman, C., Karaman, O., Güngör, A., and Karimi-Maleh, H. (2023). Review of functionalized nano porous membranes for desalination and water purification: MD simulations perspective. Environ. Res. 217, 114785. doi:10.1016/j.envres.2022.114785
Guo, J., Deka, B. J., Kim, K.-J., and An, A. K. (2019). Regeneration of superhydrophobic TiO2 electrospun membranes in seawater desalination by water flushing in membrane distillation. Desalination 468, 114054. doi:10.1016/j.desal.2019.06.020
Guo, S., Li, J., Ren, J., Zhao, H., and Cheng, F. (2022). Membrane fouling of raw coking wastewater in membrane distillation: identification of fouling potential of hydrophilic and hydrophobic components. Desalination 539, 115936. doi:10.1016/j.desal.2022.115936
Hacıfazlıoğlu, M. C., Parlar, İ., Pek, T. Ö., and Kabay, N. (2019). Evaluation of chemical cleaning to control fouling on nanofiltration and reverse osmosis membranes after desalination of MBR effluent. Desalination 466, 44–51. doi:10.1016/j.desal.2019.05.003
Holloway, R. W., Achilli, A., and Cath, T. Y. (2015). The osmotic membrane bioreactor: a critical review. Environ. Sci. Water Res. Technol. 1 (5), 581–605. doi:10.1039/c5ew00103j
Holloway, R. W., Regnery, J., Nghiem, L. D., and Cath, T. Y. (2014). Removal of trace organic chemicals and performance of a novel hybrid ultrafiltration-osmotic membrane bioreactor. Environ. Sci. Technol. 48 (18), 10859–10868. doi:10.1021/es501051b
Hosseini, S. S., Bringas, E., Tan, N. R., Ortiz, I., Ghahramani, M., and Shahmirzadi, M. A. A. (2016). Recent progress in development of high performance polymeric membranes and materials for metal plating wastewater treatment: a review. J. Water Process Eng. 9, 78–110. doi:10.1016/j.jwpe.2015.11.005
Im, S.-J., Jeong, G., Jeong, S., Cho, J., and Jang, A. (2020). Fouling and transport of organic matter in cellulose triacetate forward-osmosis membrane for wastewater reuse and seawater desalination. Chem. Eng. J. 384, 123341. doi:10.1016/j.cej.2019.123341
Im, S.-J., Rho, H., Jeong, S., and Jang, A. (2018). Organic fouling characterization of a CTA-based spiral-wound forward osmosis (SWFO) membrane used in wastewater reuse and seawater desalination. Chem. Eng. J. 336, 141–151. doi:10.1016/j.cej.2017.11.008
Jacob, M., Guigui, C., Cabassud, C., Darras, H., Lavison, G., and Moulin, L. (2010). Performances of RO and NF processes for wastewater reuse: tertiary treatment after a conventional activated sludge or a membrane bioreactor. Desalination 250 (2), 833–839. doi:10.1016/j.desal.2008.11.052
Jang, D., Jeong, S., Jang, A., and Kang, S. (2018). Relating solute properties of contaminants of emerging concern and their rejection by forward osmosis membrane. Sci. Total Environ. 639, 673–678. doi:10.1016/j.scitotenv.2018.05.078
Jeong, S., Song, K. G., Kim, J., Shin, J., Maeng, S. K., and Park, J. (2021). Feasibility of membrane distillation process for potable water reuse: a barrier for dissolved organic matters and pharmaceuticals. J. Hazard. Mater. 409, 124499. doi:10.1016/j.jhazmat.2020.124499
Ji, H., Zhang, G., Teng, L., Xing, J., Jia, X., Luo, H., et al. (2021). Fabrication of aramid-coated asymmetric PVDF membranes towards acidic and alkaline solutions concentration via direct contact membrane distillation. Appl. Surf. Sci. 562, 150185. doi:10.1016/j.apsusc.2021.150185
Jia, X., Lan, L., Zhang, X., Wang, T., Wang, Y., Ye, C., et al. (2021). Pilot-scale vacuum membrane distillation for decontamination of simulated radioactive wastewater: system design and performance evaluation. Sep. Purif. Technol. 275, 119129. doi:10.1016/j.seppur.2021.119129
Jiang, S., Li, Y., and Ladewig, B. P. (2017). A review of reverse osmosis membrane fouling and control strategies. Sci. Total Environ. 595, 567–583. doi:10.1016/j.scitotenv.2017.03.235
Judd, S. (2010). The MBR book: principles and applications of membrane bioreactors for water and wastewater treatment. Germany: Elsevier.
Julian, H., Nurgirisia, N., Qiu, G., Ting, Y.-P., and Wenten, I. G. (2022). Membrane distillation for wastewater treatment: current trends, challenges and prospects of dense membrane distillation. J. Water Process Eng. 46, 102615. doi:10.1016/j.jwpe.2022.102615
Kappel, C., Kemperman, A. J. B., Temmink, H., Zwijnenburg, A., Rijnaarts, H. H. M., and Nijmeijer, K. (2014). Impacts of NF concentrate recirculation on membrane performance in an integrated MBR and NF membrane process for wastewater treatment. J. Membr. Sci. 453, 359–368. doi:10.1016/j.memsci.2013.11.023
Kharraz, J. A., Khanzada, N. K., Farid, M. U., Kim, J., Jeong, S., and An, A. K. (2022). Membrane distillation bioreactor (MDBR) for wastewater treatment, water reuse, and resource recovery: a review. J. Water Process Eng. 47, 102687. doi:10.1016/j.jwpe.2022.102687
Kim, H.-W., Yun, T., Kang, P. K., Hong, S., Jeong, S., and Lee, S. (2019). Evaluation of a real-time visualization system for scaling detection during DCMD, and its correlation with wetting. Desalination 454, 59–70. doi:10.1016/j.desal.2018.12.014
Kim, J., Kim, H.-W., Tijing, L. D., Shon, H. K., and Hong, S. (2022). Elucidation of physicochemical scaling mechanisms in membrane distillation (MD): implication to the control of inorganic fouling. Desalination 527, 115573. doi:10.1016/j.desal.2022.115573
Kimura, K., Iwase, T., Kita, S., and Watanabe, Y. (2009). Influence of residual organic macromolecules produced in biological wastewater treatment processes on removal of pharmaceuticals by NF/RO membranes. Water Res. 43 (15), 3751–3758. doi:10.1016/j.watres.2009.05.042
Krzeminski, P., Langhorst, W., Schyns, P., De Vente, D., Van den Broeck, R., Smets, I., et al. (2012). The optimal MBR configuration: hybrid versus stand-alone—comparison between three full-scale MBRs treating municipal wastewater. Desalination 284, 341–348. doi:10.1016/j.desal.2011.10.038
Kwon, D., Bae, W., and Kim, J. (2021). Hybrid forward osmosis/membrane distillation integrated with anaerobic fluidized bed bioreactor for advanced wastewater treatment. J. Hazard. Mater. 404, 124160. doi:10.1016/j.jhazmat.2020.124160
Kwon, D., Kwon, S. J., Kim, J., and Lee, J.-H. (2020). Feasibility of the highly-permselective forward osmosis membrane process for the post-treatment of the anaerobic fluidized bed bioreactor effluent. Desalination 485, 114451. doi:10.1016/j.desal.2020.114451
Laera, G., Cassano, D., Lopez, A., Pinto, A., Pollice, A., Ricco, G., et al. (2012). Removal of organics and degradation products from industrial wastewater by a membrane bioreactor integrated with ozone or UV/H₂O₂ treatment. Environ. Sci. Technol. 46 (2), 1010–1018. doi:10.1021/es202707w
Lan, Y., Groenen-Serrano, K., Coetsier, C., and Causserand, C. (2017). Fouling control using critical, threshold and limiting fluxes concepts for cross-flow NF of a complex matrix: membrane BioReactor effluent. J. Membr. Sci. 524, 288–298. doi:10.1016/j.memsci.2016.11.001
Lan, Y., Groenen-Serrano, K., Coetsier, C., and Causserand, C. (2018). Nanofiltration performances after membrane bioreactor for hospital wastewater treatment: fouling mechanisms and the quantitative link between stable fluxes and the water matrix. Water Res. 146, 77–87. doi:10.1016/j.watres.2018.09.004
Lee, G., Kim, H.-W., Boo, C., Beak, Y., Kwak, R., Kim, C., et al. (2021a). Bibliometric analysis of twenty-year research trend in desalination technologies during 2000-2020. J. Korean Soc. Water Wastewater 35 (1), 39–52. doi:10.11001/jksww.2021.35.1.039
Lee, S., Bayarkhuu, B., Han, Y., Kim, H.-W., Jeong, S., Boo, C., et al. (2022). Multifunctional photo-Fenton-active membrane for solar-driven water purification. J. Membr. Sci. 660, 120832. doi:10.1016/j.memsci.2022.120832
Lee, W., An, S., and Choi, Y. (2021b). Ammonia harvesting via membrane gas extraction at moderately alkaline pH: a step toward net-profitable nitrogen recovery from domestic wastewater. Chem. Eng. J. 405, 126662. doi:10.1016/j.cej.2020.126662
Li, B., Yun, Y., Liu, G., Li, C., Li, X., Hilal, M., et al. (2021). Direct contact membrane distillation with softening Pre-treatment for effective reclaiming flue gas desulfurization wastewater. Sep. Purif. Technol. 277, 119637. doi:10.1016/j.seppur.2021.119637
Li, K., Cheng, Y., Wang, J., Zhang, J., Liu, J., Yu, D., et al. (2016). Effects of returning NF concentrate on the MBR-NF process treating antibiotic production wastewater. Environ. Sci. Pollut. Res. 23, 13114–13127. doi:10.1007/s11356-016-6467-x
Li, K., Liu, Q., Fang, F., Wu, X., Xin, J., Sun, S., et al. (2020a). Influence of nanofiltration concentrate recirculation on performance and economic feasibility of a pilot-scale membrane bioreactor-nanofiltration hybrid process for textile wastewater treatment with high water recovery. J. Clean. Prod. 261, 121067. doi:10.1016/j.jclepro.2020.121067
Li, Y., Sim, L. N., Ho, J. S., Chong, T. H., Wu, B., and Liu, Y. (2020b). Integration of an anaerobic fluidized-bed membrane bioreactor (MBR) with zeolite adsorption and reverse osmosis (RO) for municipal wastewater reclamation: comparison with an anoxic-aerobic MBR coupled with RO. Chemosphere 245, 125569. doi:10.1016/j.chemosphere.2019.125569
Lin, Y.-L., Zheng, N.-Y., and Chen, Y.-S. (2021). Enhancing H2O2 tolerance and separation performance through the modification of the polyamide layer of a thin-film composite nanofiltration membrane by using graphene oxide. Membranes 11 (8), 592. doi:10.3390/membranes11080592
Liu, Q., Wang, X. C., Liu, Y., Yuan, H., and Du, Y. (2010). Performance of a hybrid membrane bioreactor in municipal wastewater treatment. Desalination 258 (1-3), 143–147. doi:10.1016/j.desal.2010.03.024
Liu, W., Song, X., Huda, N., Xie, M., Li, G., and Luo, W. (2020). Comparison between aerobic and anaerobic membrane bioreactors for trace organic contaminant removal in wastewater treatment. Environ. Technol. Innovation 17, 100564. doi:10.1016/j.eti.2019.100564
Liu, X., Li, Y., Chen, Z., Yang, H., Wang, S., Tang, Z., et al. (2023). Recent progress of COFs membranes: design, synthesis and application in water treatment. USA: Eco-Environment and Health.
Lutchmiah, K., Verliefde, A. R. D., Roest, K., Rietveld, L. C., and Cornelissen, E. R. (2014). Forward osmosis for application in wastewater treatment: a review. Water Res. 58, 179–197. doi:10.1016/j.watres.2014.03.045
Madalosso, H. B., Silva, R. d.S., Machado, R. A. F., and Marangoni, C. (2022). Superhydrophobic PA membrane for robust anti-wetting membrane distillation to water reclamation from textile wastewater. J. Water Process Eng. 49, 103162. doi:10.1016/j.jwpe.2022.103162
Malamis, S., Katsou, E., Takopoulos, K., Demetriou, P., and Loizidou, M. (2012). Assessment of metal removal, biomass activity and RO concentrate treatment in an MBR–RO system. J. Hazard. Mater. 209-210, 1–8. doi:10.1016/j.jhazmat.2011.10.085
Matin, A., Laoui, T., Falath, W., and Farooque, M. (2021). Fouling control in reverse osmosis for water desalination and reuse: current practices and emerging environment-friendly technologies. Sci. Total Environ. 765, 142721. doi:10.1016/j.scitotenv.2020.142721
Memon, S., Lee, H.-S., Kim, W.-S., and Kim, Y.-D. (2022). Parametric investigation of modular configuration of multi-stage direct contact membrane distillation powered by waste heat of wind turbine. Desalination 533, 115770. doi:10.1016/j.desal.2022.115770
Meng, F., Zhang, S., Oh, Y., Zhou, Z., Shin, H.-S., and Chae, S.-R. (2017). Fouling in membrane bioreactors: an updated review. Water Res. 114, 151–180. doi:10.1016/j.watres.2017.02.006
Moktadir, M. A., Maliha, M., Munmun, S. A., Alam, S., Islam, M. A., Rahman, M. M., et al. (2023). Treatment of tannery wastewater by different membrane bioreactors: a critical review. Environ. Adv. 15, 100478. doi:10.1016/j.envadv.2023.100478
Moser, P. B., Ricci, B. C., Reis, B. G., Neta, L. S. F., Cerqueira, A. C., and Amaral, M. C. S. (2018). Effect of MBR-H2O2/UV Hybrid pre-treatment on nanofiltration performance for the treatment of petroleum refinery wastewater. Sep. Purif. Technol. 192, 176–184. doi:10.1016/j.seppur.2017.09.070
Nativ, P., Leifman, O., Lahav, O., and Epsztein, R. (2021). Desalinated brackish water with improved mineral composition using monovalent-selective nanofiltration followed by reverse osmosis. Desalination 520, 115364. doi:10.1016/j.desal.2021.115364
Nawaz, M. S., Gadelha, G., Khan, S. J., and Hankins, N. (2013). Microbial toxicity effects of reverse transported draw solute in the forward osmosis membrane bioreactor (FO-MBR). J. Membr. Sci. 429, 323–329. doi:10.1016/j.memsci.2012.11.057
Nawaz, M. S., Parveen, F., Gadelha, G., Khan, S. J., Wang, R., and Hankins, N. P. (2016). Reverse solute transport, microbial toxicity, membrane cleaning and flux of regenerated draw in the FO-MBR using a micellar draw solution. Desalination 391, 105–111. doi:10.1016/j.desal.2016.02.023
Ndiaye, I., Vaudreuil, S., and Bounahmidi, T. (2021). Forward osmosis process: state-of-the-art of membranes. Sep. Purif. Rev. 50 (1), 53–73. doi:10.1080/15422119.2019.1622133
Nguyen, N. C., Chen, S.-S., Nguyen, H. T., Ngo, H. H., Guo, W., Hao, C. W., et al. (2015). Applicability of a novel osmotic membrane bioreactor using a specific draw solution in wastewater treatment. Sci. Total Environ. 518-519, 586–594. doi:10.1016/j.scitotenv.2015.03.011
Olives, P., Sanchez, L., Lesage, G., Héran, M., Rodriguez-Roda, I., and Blandin, G. (2023). Impact of integration of FO membranes into a granular biomass AnMBR for water reuse. Membranes 13 (3), 265. doi:10.3390/membranes13030265
Ouali, S., Loulergue, P., Biard, P.-F., Nasrallah, N., and Szymczyk, A. (2021). Ozone compatibility with polymer nanofiltration membranes. J. Membr. Sci. 618, 118656. doi:10.1016/j.memsci.2020.118656
Parida, V., and Ng, H. Y. (2013). Forward osmosis organic fouling: effects of organic loading, calcium and membrane orientation. Desalination 312, 88–98. doi:10.1016/j.desal.2012.04.029
Parlar, I., Hacıfazlıoğlu, M., Kabay, N., Pek, T. Ö., and Yüksel, M. (2019). Performance comparison of reverse osmosis (RO) with integrated nanofiltration (NF) and reverse osmosis process for desalination of MBR effluent. J. Water Process Eng. 29, 100640. doi:10.1016/j.jwpe.2018.06.002
Pathak, N., Shon, H., and Vigneswaran, S. (2021). Advanced membrane bioreactor hybrid systems. Sustain. Technol. Water Wastewater Treat., 317–342. doi:10.1201/9781003052234-22
Pearce, G. K. (2008). UF/MF pre-treatment to RO in seawater and wastewater reuse applications: a comparison of energy costs. Desalination 222 (1-3), 66–73. doi:10.1016/j.desal.2007.05.029
Qasim, M., Badrelzaman, M., Darwish, N. N., Darwish, N. A., and Hilal, N. (2019). Reverse osmosis desalination: a state-of-the-art review. Desalination 459, 59–104. doi:10.1016/j.desal.2019.02.008
Qin, J.-J., Kekre, K. A., Oo, M. H., Tao, G., Lay, C. L., Lew, C. H., et al. (2010). Preliminary study of osmotic membrane bioreactor: effects of draw solution on water flux and air scouring on fouling. Water Sci. Technol. 62 (6), 1353–1360. doi:10.2166/wst.2010.426
Qiu, G., Zhang, S., Srinivasa Raghavan, D. S., Das, S., and Ting, Y.-P. (2016). The potential of hybrid forward osmosis membrane bioreactor (FOMBR) processes in achieving high throughput treatment of municipal wastewater with enhanced phosphorus recovery. Water Res. 105, 370–382. doi:10.1016/j.watres.2016.09.017
Ray, S. S., Chen, S.-S., Sangeetha, D., Chang, H.-M., Thanh, C. N. D., Le, Q. H., et al. (2018). Developments in forward osmosis and membrane distillation for desalination of waters. Environ. Chem. Lett. 16, 1247–1265. doi:10.1007/s10311-018-0750-7
Reis, B. G., Silveira, A. L., Lebron, Y. A. R., Moreira, V. R., Teixeira, L. P. T., Okuma, A. A., et al. (2020). Comprehensive investigation of landfill leachate treatment by integrated Fenton/microfiltration and aerobic membrane bioreactor with nanofiltration. Process Saf. Environ. Prot. 143, 121–128. doi:10.1016/j.psep.2020.06.037
Reis, B. G., Silveira, A. L., Tostes Teixeira, L. P., Okuma, A. A., Lange, L. C., and Amaral, M. C. S. (2017). Organic compounds removal and toxicity reduction of landfill leachate by commercial bakers’ yeast and conventional bacteria based membrane bioreactor integrated with nanofiltration. Waste Manag. 70, 170–180. doi:10.1016/j.wasman.2017.09.030
Rodríguez-Hernández, L., Esteban-García, A., and Tejero, I. (2014). Comparison between a fixed bed hybrid membrane bioreactor and a conventional membrane bioreactor for municipal wastewater treatment: a pilot-scale study. Bioresour. Technol. 152, 212–219. doi:10.1016/j.biortech.2013.10.081
Santos, P. G., Scherer, C. M., Fisch, A. G., and Rodrigues, M. A. S. (2020). Petrochemical wastewater treatment: water recovery using membrane distillation. J. Clean. Prod. 267, 121985. doi:10.1016/j.jclepro.2020.121985
Satterfield, D. J., Griffin, J. C., George, T., and Hiibel, S. R. (2021). Biological fouling mitigation in a forward-osmosis membrane bioreactor. J. Environ. Eng. 147 (8), 04021025. doi:10.1061/(asce)ee.1943-7870.0001894
Sert, G., Bunani, S., Kabay, N., Egemen, Ö., Arda, M., Pek, T. Ö., et al. (2016). Investigation of mini pilot scale MBR-NF and MBR-RO integrated systems performance—preliminary field tests. J. Water Process Eng. 12, 72–77. doi:10.1016/j.jwpe.2016.06.008
Sert, G., Bunani, S., Yörükoğlu, E., Kabay, N., Egemen, Ö., Arda, M., et al. (2017). Performances of some NF and RO membranes for desalination of MBR treated wastewater. J. Water Process Eng. 16, 193–198. doi:10.1016/j.jwpe.2016.11.009
Simoni, G., Kirkebæk, B. S., Quist-Jensen, C. A., Christensen, M. L., and Ali, A. (2021). A comparison of vacuum and direct contact membrane distillation for phosphorus and ammonia recovery from wastewater. J. Water Process Eng. 44, 102350. doi:10.1016/j.jwpe.2021.102350
Sivodia, C., and Sinha, A. (2023). “Advanced treatment methods for the emerging contaminants: an insight into the removal of anticancer drugs,” in Persistent pollutants in water and advanced treatment technology (Germany: Springer), 197–211.
Skouteris, G., Hermosilla, D., López, P., Negro, C., and Blanco, Á. (2012). Anaerobic membrane bioreactors for wastewater treatment: a review. Chem. Eng. J. 198, 138–148. doi:10.1016/j.cej.2012.05.070
Smith, A. L., Stadler, L. B., Love, N. G., Skerlos, S. J., and Raskin, L. (2012). Perspectives on anaerobic membrane bioreactor treatment of domestic wastewater: a critical review. Bioresour. Technol. 122, 149–159. doi:10.1016/j.biortech.2012.04.055
Song, W., Xie, B., Huang, S., Zhao, F., and Shi, X. (2020). “6 - aerobic membrane bioreactors for industrial wastewater treatment,” in Current developments in biotechnology and bioengineering. Editors H. Y. Ng, T. C. A. Ng, H. H. Ngo, G. Mannina, and A. Pandey (Germany: Elsevier), 129–145.
Song, X., Luo, W., McDonald, J., Khan, S. J., Hai, F. I., Price, W. E., et al. (2018). An anaerobic membrane bioreactor – membrane distillation hybrid system for energy recovery and water reuse: removal performance of organic carbon, nutrients, and trace organic contaminants. Sci. Total Environ. 628, 358–365. doi:10.1016/j.scitotenv.2018.02.057
Stoquart, C., Servais, P., Bérubé, P. R., and Barbeau, B. (2012). Hybrid membrane processes using activated carbon treatment for drinking water: a review. J. Membr. Sci. 411, 1–12. doi:10.1016/j.memsci.2012.04.012
Sun, H., Liu, H., Han, J., Zhang, X., Cheng, F., and Liu, Y. (2018). Chemical cleaning-associated generation of dissolved organic matter and halogenated byproducts in ceramic MBR: ozone versus hypochlorite. Water Res. 140, 243–250. doi:10.1016/j.watres.2018.04.050
Sun, H., Liu, H., Zhang, M., and Liu, Y. (2021). A novel single-stage ceramic membrane moving bed biofilm reactor coupled with reverse osmosis for reclamation of municipal wastewater to NEWater-like product water. Chemosphere 268, 128836. doi:10.1016/j.chemosphere.2020.128836
Tang, K., Xie, J., Pan, Y., Zou, X., Sun, F., Yu, Y., et al. (2022). The optimization and regulation of energy consumption for MBR process: a critical review. J. Environ. Chem. Eng. 10, 108406. doi:10.1016/j.jece.2022.108406
Tay, M. F., Lee, S., Xu, H., Jeong, K., Liu, C., Cornelissen, E. R., et al. (2020). Impact of salt accumulation in the bioreactor on the performance of nanofiltration membrane bioreactor (NF-MBR)+Reverse osmosis (RO) process for water reclamation. Water Res. 170, 115352. doi:10.1016/j.watres.2019.115352
Tay, M. F., Liu, C., Cornelissen, E. R., Wu, B., and Chong, T. H. (2018). The feasibility of nanofiltration membrane bioreactor (NF-MBR)+reverse osmosis (RO) process for water reclamation: comparison with ultrafiltration membrane bioreactor (UF-MBR)+RO process. Water Res. 129, 180–189. doi:10.1016/j.watres.2017.11.013
Tibi, F., Charfi, A., Cho, J., and Kim, J. (2020). Fabrication of polymeric membranes for membrane distillation process and application for wastewater treatment: critical review. Process Saf. Environ. Prot. 141, 190–201. doi:10.1016/j.psep.2020.05.026
Tibi, F., Guo, J., Ahmad, R., Lim, M., Kim, M., and Kim, J. (2019). Membrane distillation as post-treatment for anaerobic fluidized bed membrane bioreactor for organic and nitrogen removal. Chemosphere 234, 756–762. doi:10.1016/j.chemosphere.2019.06.043
Tun, L. L., Jeong, D., Jeong, S., Cho, K., Lee, S., and Bae, H. (2016). Dewatering of source-separated human urine for nitrogen recovery by membrane distillation. J. Membr. Sci. 512, 13–20. doi:10.1016/j.memsci.2016.04.004
Van de Walle, A., Kim, M., Alam, M. K., Wang, X., Wu, D., Dash, S. R., et al. (2023). Greywater reuse as a key enabler for improving urban wastewater management. Environ. Sci. Ecotechnology 16, 100277. doi:10.1016/j.ese.2023.100277
Wafi, M. K., Hussain, N., El-Sharief Abdalla, O., Al-Far, M. D., Al-Hajaj, N. A., and Alzonnikah, K. F. (2019). Nanofiltration as a cost-saving desalination process. SN Appl. Sci. 1, 1–9. doi:10.1007/s42452-019-0775-y
Wang, J., Li, K., Wei, Y., Cheng, Y., Wei, D., and Li, M. (2015a). Performance and fate of organics in a pilot MBR–NF for treating antibiotic production wastewater with recycling NF concentrate. Chemosphere 121, 92–100. doi:10.1016/j.chemosphere.2014.11.034
Wang, S., Liu, H., Gu, J., Sun, H., Zhang, M., and Liu, Y. (2019). Technology feasibility and economic viability of an innovative integrated ceramic membrane bioreactor and reverse osmosis process for producing ultrapure water from municipal wastewater. Chem. Eng. J. 375, 122078. doi:10.1016/j.cej.2019.122078
Wang, X., Chang, V. W. C., and Tang, C. Y. (2016). Osmotic membrane bioreactor (OMBR) technology for wastewater treatment and reclamation: advances, challenges, and prospects for the future. J. Membr. Sci. 504, 113–132. doi:10.1016/j.memsci.2016.01.010
Wang, Y.-K., Pan, X.-R., Sheng, G.-P., Li, W.-W., Shi, B.-J., and Yu, H.-Q. (2015b). Development of an energy-saving anaerobic hybrid membrane bioreactors for 2-chlorophenol-contained wastewater treatment. Chemosphere 140, 79–84. doi:10.1016/j.chemosphere.2014.04.101
WHO (2006). Guidelines for the safe use of wastewater, excreta and grey water use in agriculture. Switzerland: Geneva.
Wintgens, T., Gallenkemper, M., and Melin, T. (2002). Endocrine disrupter removal from wastewater using membrane bioreactor and nanofiltration technology. Desalination 146 (1), 387–391. doi:10.1016/S0011-9164(02)00519-2
Wintgens, T., Gallenkemper, M., and Melin, T. (2004). Removal of endocrine disrupting compounds with membrane processes in wastewater treatment and reuse. Water Sci. Technol. 50 (5), 1–8. doi:10.2166/wst.2004.0301
Woo, Y. C., Lee, J. J., Shim, W.-G., Shon, H. K., Tijing, L. D., Yao, M., et al. (2016). Effect of powdered activated carbon on integrated submerged membrane bioreactor–nanofiltration process for wastewater reclamation. Bioresour. Technol. 210, 18–25. doi:10.1016/j.biortech.2016.02.023
Wu, B., and Fane, A. G. (2012). Microbial relevant fouling in membrane bioreactors: influencing factors, characterization, and fouling control. Membranes 2 (3), 565–584. doi:10.3390/membranes2030565
Wu, B., and Kim, J. (2020). Anaerobic membrane bioreactors for nonpotable water reuse and energy recovery. J. Environ. Eng. 146 (2), 03119002. doi:10.1061/(asce)ee.1943-7870.0001637
Wu, B., Kitade, T., Chong, T. H., Uemura, T., and Fane, A. G. (2013). Impact of membrane bioreactor operating conditions on fouling behavior of reverse osmosis membranes in MBR–RO processes. Desalination 311, 37–45. doi:10.1016/j.desal.2012.11.020
Wu, X., Lau, C. H., Pramanik, B. K., Zhang, J., and Xie, Z. (2021). State-of-the-art and opportunities for forward osmosis in sewage concentration and wastewater treatment. Membranes 11 (5), 305. doi:10.3390/membranes11050305
Xue, W., Wu, C., Xiao, K., Huang, X., Zhou, H., Tsuno, H., et al. (2010). Elimination and fate of selected micro-organic pollutants in a full-scale anaerobic/anoxic/aerobic process combined with membrane bioreactor for municipal wastewater reclamation. Water Res. 44 (20), 5999–6010. doi:10.1016/j.watres.2010.07.052
Yacouba, Z. A., Mendret, J., Lesage, G., Zaviska, F., and Brosillon, S. (2021). Removal of organic micropollutants from domestic wastewater: the effect of ozone-based advanced oxidation process on nanofiltration. J. Water Process Eng. 39, 101869. doi:10.1016/j.jwpe.2020.101869
Yan, T., Ye, Y., Ma, H., Zhang, Y., Guo, W., Du, B., et al. (2018). A critical review on membrane hybrid system for nutrient recovery from wastewater. Chem. Eng. J. 348, 143–156. doi:10.1016/j.cej.2018.04.166
Yan, Z., Chen, X., Bao, S., Chang, H., Liu, H., Fan, G., et al. (2022). Integration of in situ Fenton-like self-cleaning and photothermal membrane distillation for wastewater treatment via Co-MoS2/CNT catalytic membrane. Sep. Purif. Technol. 303, 122207. doi:10.1016/j.seppur.2022.122207
Yang, Y., Li, C., and Hou, L.-a. (2018). Impact of dead cells on biofouling and pharmaceutically active compounds retention by NF/RO membranes. Chem. Eng. J. 337, 51–59. doi:10.1016/j.cej.2017.12.081
Zheng, L., Zhang, C., Kang, S., Li, C., Hou, D., Wu, S., et al. (2022). Insight into the microbial distribution and succession and biofouling mechanism in membrane distillation for desulfurization wastewater treatment. Chem. Eng. J. 428, 131097. doi:10.1016/j.cej.2021.131097
Zhu, H., and Li, W. (2013). Bisphenol A removal from synthetic municipal wastewater by a bioreactor coupled with either a forward osmotic membrane or a microfiltration membrane unit. Front. Environ. Sci. Eng. 7, 294–300. doi:10.1007/s11783-013-0486-3
Keywords: membrane bioreactor, desalination, wastewater reclamation, fouling, wastewater reuse
Citation: Kim J, Wu B, Jeong S, Jeong S and Kim M (2024) Recent advances of membrane-based hybrid membrane bioreactors for wastewater reclamation. Front. Membr. Sci. Technol. 3:1361433. doi: 10.3389/frmst.2024.1361433
Received: 26 December 2023; Accepted: 15 February 2024;
Published: 29 February 2024.
Edited by:
Mohammad Mahdi A. Shirazi, Aalborg University, DenmarkReviewed by:
Hang Liu, Chinese Academy of Sciences (CAS), ChinaCopyright © 2024 Kim, Wu, Jeong, Jeong and Kim. This is an open-access article distributed under the terms of the Creative Commons Attribution License (CC BY). The use, distribution or reproduction in other forums is permitted, provided the original author(s) and the copyright owner(s) are credited and that the original publication in this journal is cited, in accordance with accepted academic practice. No use, distribution or reproduction is permitted which does not comply with these terms.
*Correspondence: Jeonghwan Kim, amVvbmdod2Fua2ltQGluaGEuYWMua3I=
Disclaimer: All claims expressed in this article are solely those of the authors and do not necessarily represent those of their affiliated organizations, or those of the publisher, the editors and the reviewers. Any product that may be evaluated in this article or claim that may be made by its manufacturer is not guaranteed or endorsed by the publisher.
Research integrity at Frontiers
Learn more about the work of our research integrity team to safeguard the quality of each article we publish.