- 1Regional Water and Environmental Sanitation Centre, Kwame Nkrumah University of Science and Technology, University Post Office (UPO), Kumasi, Ghana
- 2Department of Chemical Engineering, Kwame Nkrumah University of Science and Technology, University Post Office (UPO), Kumasi, Ghana
- 3Department of Materials Engineering, Kwame Nkrumah University of Science and Technology, University Post Office (UPO), Kumasi, Ghana
- 4Institute for Nanotechnology and Water Sustainability, College of Science, Engineering and Technology, University of South Africa, Johannesburg, South Africa
The demand for freshwater keeps increasing on a global scale, and on the other hand, the availability of freshwater keeps diminishing. Groundwater has been identified as the largest source of freshwater that is readily accessible. Although the water is available for abstraction, it must be treated to meet application standards. Membrane processes are the options that industry and researchers are turning to for the purification of groundwater. This review provides an insight into the use of pressure-driven membrane processes for groundwater treatment, with focus on the spiral wound membrane module. A brief description of what a spiral wound module is and the plant set-up in which it is used is given. The various applications of the spiral wound module with regards to groundwater treatment have been reviewed. The shortcomings and challenges limiting the application of spiral wound modules and by extension, the treatment plant itself have been highlighted. To cap it all, the opportunities that can be exploited to overcome these challenges and position pressure-driven membrane processes for groundwater treatment as the go-to purification method have been discussed.
1 Introduction
Over the years, the design and performance of membranes for desalination has improved enormously, starting with the use of hollow fibers, cellulose acetate and eventually polyamide as the active layer in thin film composite (TFC) membranes. TFC membranes are now state-of-the-art for desalination and widely used in the spiral wound module configuration. This configuration has also seen significant developments in terms of improved production capacity, active surface area, permeability, selectivity, stability among others, making it the go-to choice for desalination (Johnson and Busch, 2010; Johnson, 2013; Hassan et al., 2020).
Groundwater is the source of over 96% of all liquid freshwater on earth as graphically shown in Figure 1 (UN Water, 2022). Groundwater flows, but not as fast as surface waters, hence aquifers can hold them for several years. As a result of this, groundwater tends to have the characteristics of the aquifers in which they are located. This implies that if for instance the aquifer has fluoride, iron or manganese bearing rocks, the water would most likely contain them as well. Apart from aquifer characteristics, there are other factors that contribute to the chemistry of groundwater. These include soil processes, anthropogenic activities, and the chemistry of recharging water (Burkart and Stoner, 2001). Water obtained from natural sources, such as groundwater, typically has elements that have been dissolved in it. Technically, these elements are known as total dissolved solids (TDS) and their concentration is used to classify the water into various groups. Shallow groundwater tends to have a TDS value of less than 1,000 mg/L and is classified as freshwater. Groundwater obtained from below shallow waters tends to have a TDS value between 4,000 mg/L and 10,000 mg/L and is classified as brackish. At deeper depths, groundwater can contain up to 35, 000 mg/L of TDS or more and these are classified as saline and brine respectively. The components of TDS make up the chemistry of groundwater and are broadly classified into three categories; these are major constituents, minor constituents, and trace elements. Anions such as chloride, bicarbonate, and sulphate, and then cations such as sodium, calcium, and magnesium make up the major constituents. Minor constituents include fluoride, silica, nitrate, potassium, iron, and manganese. Trace elements include lithium, boron, barium, aluminium, zinc, strontium, arsenic, among others (Poeter et al., 2020).
In some aquifers, the concentration of these constituents or elements is very high such that the water becomes contaminated and does not meet the drinking water quality guidelines, hence requiring treatment or desalination. The motivation for this article is to address the need to treat groundwater of low quality to meet application requirement standards. Groundwater accounts for about half of domestic water used around the globe. It also tends to be the major source of water for all applications for those who find themselves in small communities and towns where national water distribution systems are not available (UN Water, 2022).
Over the years, various technologies have been developed for the treatment of water to make it portable for drinking. The characteristics of these technologies are outlined in Table 1. Currently some advanced technologies have been developed for treatment of water to meet drinking water quality standards. Among these technologies is membrane filtration. It has advantages such as the ability to produce highly purified water, simple operation, and a small carbon footprint as compared to the conventional methods (Madhura et al., 2018).
There are various membrane filtration processes and the characteristics of the water to be treated determine which would be the most suitable. When considering seawater, brackish water, and groundwater as the source of water to be treated, their composition and salinity are the main determinants of which process to select. Seawater usually has a high salinity of about 35, 000 mg/L of TDS, characterized by a very high osmotic pressure, making reverse osmosis the preferred treatment process (Lim et al., 2021). In using the reverse osmosis process, higher pressures can be applied to overcome the osmotic pressure and obtain highly purified water (J. Kim et al., 2019). Brackish water tends to have high salinities as well but not as high as that of seawater. Hence reverse osmosis can be used to treat it as well. However, there are commercial membranes available specifically for treating brackish water (Madduri et al., 2023). In the case of groundwater, as stated earlier, its salinity and composition vary greatly depending on the aquifer. If the water is classified as fresh water, it has very low salinities and can be treated by nanofiltration which does not require the application of very high pressures as in the case of seawater and brackish water (Elmenshawy et al., 2023).
The objective of this paper is to review how spiral wound membrane modules (SWM) are being used to filtrate water, with specific focus on groundwater sources, to make it suitable for various applications. To this end, background information on spiral wound membrane modules and the plant set-up in which it is used will be presented. Furthermore, relevant literature on its application in treating groundwater will be provided. Lastly, the challenges and opportunities of the use of spiral wound membrane modules for treating groundwater will be highlighted.
2 Spiral wound membrane module
The configuration in which a membrane is packed for industrial application is known as the membrane module. Available membrane modules include tubular (Xue et al., 2021), hollow fiber (Ismail et al., 2019a), plate-and-frame (Yasukawa et al., 2018), and spiral wound. The major characteristics of each of these configurations as compared to one another are presented in Table 2.
There are different forms of the spiral wound modules (SWM), depending on the application. It can be used as a contactor in membrane bioreactors (Verma et al., 2022). They can also be used in electrodialysis (Wright and Winter, 2019), in forward osmosis (S. Lee, 2020) and in membrane distillation (Lee et al., 2020). The spiral wound module reviewed in this paper is the type used in pressure-driven applications such as reserve osmosis (RO) and nanofiltration (NF), with special focus on groundwater treatment.
The major parts of this kind of module are flat sheet membranes, feed and permeate spacers, a permeate tube and a housing for the previously mentioned parts, that can withstand high in-service pressures (Schwinge et al., 2004; Kostoglou and Karabelas, 2009). The membrane is typically a thin film composite (TFC) membrane that is folded along the breadth in the middle into two equal halves, with the active layer facing inward. The permeate spacer (permeate carrier material) is placed inside the folded membrane and the membrane is glued along the three open sides to form an envelope. Several of these envelopes are made and wound round a perforated tube, starting from the folded or unglued edge. Each of the envelopes are separated by feed spacers (feed water carrier). Feed spacers are used to create flow channels for water between the membrane envelopes (Karabelas et al., 2018a). The perforated tube collects all the water that has been filtered by the membranes at the middle of the module and sends it to the permeate collection pipe. The rejected water, known as concentrate, then flows to the concentrate pipe. The technical details involved in the fabrication of SWMs can be obtained from these references (Chen et al., 2020; Sutariya et al., 2022).
Depending on the dimension and/or number of envelopes that are wound round the perforated tube, there are different sizes of spiral wound modules. Typically, the length is 40″ (approximately 1 m) and available diameter sizes include 1.8″, 2.5″, 4″, 8″, 16″and 18". The 1.8″, 2.5″ and 4″ modules are normally used for pre-industrial scale activities such as laboratory studies. The 8″ module is the commonly used full scale operation size, while the 16″ and 18″ are rarely used. The active membrane area for the modules varies depending on the manufacturer. However, it is approximately 7, 41 and 158 m2 for the 4, 8 and 16″ modules respectively (Johnson, 2013).
The modules are housed in pressurized vessels for application. An anti-telescoping device is used to prevent the membrane envelopes from unraveling or extending when it is under pressure. Normally, about three to eight modules, also known as elements, are packed into the pressurized vessel and their perforated tubes are connected with coupling units for standard application (Bucs et al., 2018). A schematic diagram of a spiral wound module is shown in Figure 2. Usually, the individual modules are arranged in series in the pressure vessel. The vessels can then be arranged in parallel into a stage, also known as a bank. The stages can then also be arrange (Wang et al., 2023)d in series to form an array, also known as a cascade. Some array configurations, that is 1:1:1, 3:3:3, 3:2:1, are depicted in Figure 3 for a better understanding of the concept. SWMs are often used in multi stages in treatment plants. The total number of modules used per stage depends on the production requirements of the plant. The modules may be arranged for a single-pass process or to allow for recirculation or multi-pass processes. The arrangement of modules are often influenced by the feed quality and then the permeate and concentrate quality desired (Schwinge et al., 2004).
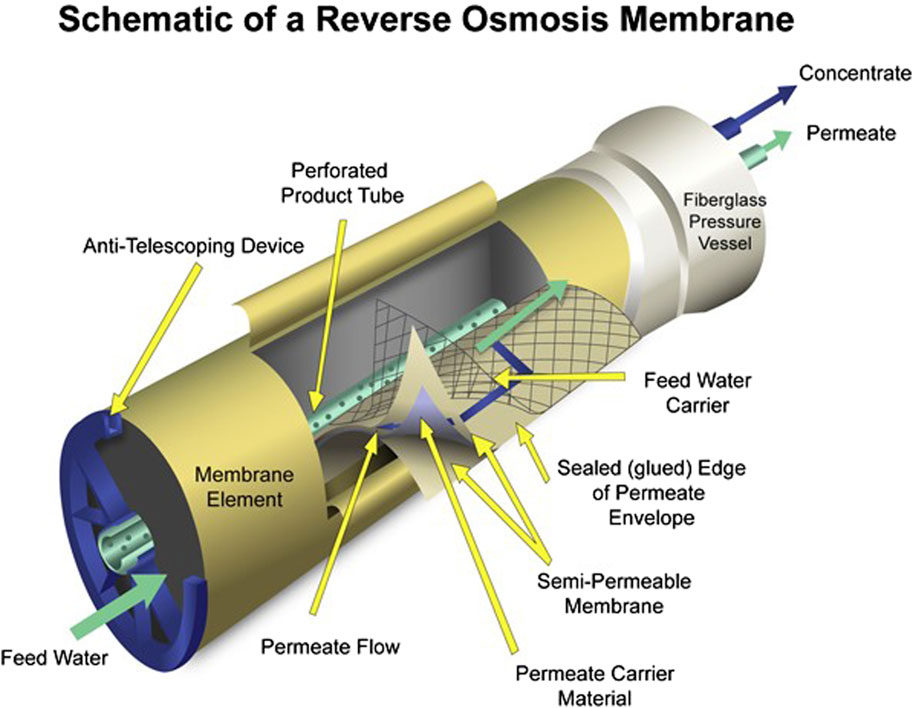
Figure 2. Schematic diagram of Spiral Wound Membrane Module (source (Ionics Freshwater Ltd, 2010).
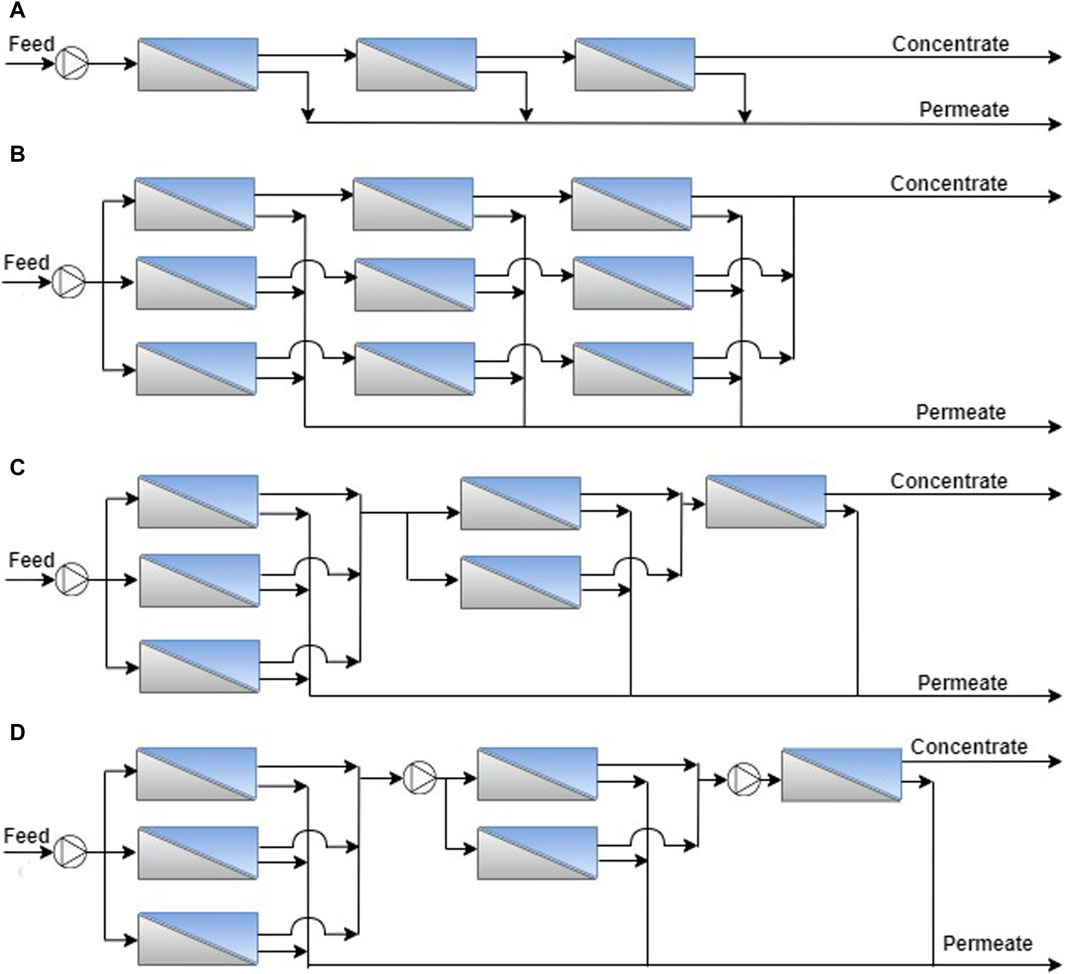
Figure 3. Spiral Wound Module arrays (A) 1:1:1 (B) 3:3:3 (C) 3:2:1 (D) 3:2:1 with inter-stage pumping (source redrawn from Schwinge et al, (2004)).
2.1 Thin film composite membrane
The part of the spiral wound membrane module that does the actual rejection of contaminants is the flat sheet membrane that has been wound round the perforated tube. It is usually a thin film composite (TFC) membrane. The preparation of TFC membranes which could be commercialized was pioneered by Cadotte et al. (1980) over 4 decades ago. However, the foundation for the use of membranes of this kind for water treatment was laid by 2 major discoveries which include preferential salt rejection in the 1950s using pressure driven processes by Reid (Reid and Breton, 1959) and the use of asymmetric membranes to achieve a high permeate flux in the 1960s by Loeb and Sourirajan (Loeb and Sourirajan, 1963; Nunes and Peinemann, 2006; Imbrogno and Belfort, 2016). A Typical TFC membrane consists of three layers, which are a support layer, an interlayer, and an active layer. The support layer is usually a porous ultrafiltration membrane made of either polyethersulfone or polysulfone and provides mechanical support for the TFC membrane (Mokarizadeh et al., 2021). In between the support layer and the active layer is the interlayer and it acts as a transitional zone. It can be used to improve adhesion between the support layer and the active layer, reduce the occurrence of defects in the TFC membrane and modify the surface of the support. Materials such as polyvinyl alcohol, polyethyleneimine, carbon nanotubes, graphene oxide and water channels can be used to form the interlayer (Li et al., 2024). The active layer is where the selective rejection of ions or selective transport of water molecules takes place. It is usually a thin film of polyamide obtained by an interfacial polymerization reaction between an aqueous phase which contains a monomer such as m-phenylenediamine or piperazine and an organic phase which also contains a monomer such as trimesoyl chloride (Nunes and Peinemann, 2006; Chew and Yong, 2021). Depending on the characteristics of the active layer, it could be used for nanofiltration or reverse osmosis. In nanofiltration applications, the active layer has a relatively higher porosity with a molecular weight cut-off of up to about 1,000 Da and is able to reject most large contaminants and divalent ions, but not monovalent ions (Greenlee et al., 2009). Transport across the membrane is controlled by both solution diffusion and pore flow (Geise et al., 2010). On the other hand, active layers used for reverse osmosis have a very fine and connected pore structure with a molecular weight cut-off less than 200 Da and can reject almost all ions, particles, and dissolved substances including monovalent ions (Greenlee et al., 2009). Here, transport across the membrane is mainly controlled by solution diffusion (Geise et al., 2010). Due to these intrinsic properties of the TFC, there is usually a trade-off between permeability and selectivity. If the active layer is more porous, it has a high permeability but a corresponding reduced selectivity and vice versa (Q. Wang et al., 2023).
2.2 Spacers
2.2.1 Feed spacers
Feed spacers are an essential part of the spiral wound module. They are used to create flow channels for the feed between the membrane envelopes. Commonly available commercial feed spacers are composed of two layers of seemingly cylindrical polymeric filaments arranged into a net-like sheet that meets at 90° but not woven (Karabelas et al., 2018b).
The characteristics of the feed spacer, together with other operating parameters such as pressure, temperature, membrane specification and feed flow rate influence the overall performance of the SWM. One characteristic is that it should be able to withstand the tension imposed on it during fabrication and also application pressures (Karabelas et al., 2018a; Haidari et al., 2018). Another important one is the geometry of the feed spacer. Several geometries are possible based on a combination of the angle at which the filaments meet (mesh angle), the angles which each of the layers of filaments make with the principal flow direction (attack angle), the mesh length, and filament diameter and as to if the filaments are woven or not and the extent of the weave (partially woven or fully woven). Gu et al. (2017) investigated 20 feed spacer geometries by varying 4 spacer kinds (fully woven, partially woven, not woven, and middle layer) and 5 combinations of mesh and attack angles. They observed that the fully woven spacers had the highest fluxes, highest pressure drop and lowest concentration polarization. Furthermore, the mesh angle mostly influences the concentration polarization and permeate flux, such that when it reduces, there is a corresponding reduction in permeate flux and an increase in concentration polarization.
However, the use of feed spacers can result in some challenges. A typical challenge is that they serve as a resistance to fluid flow, causing a pressure drop in the feed channel. This phenomenon can lead to significant energy losses in the presence of fouling (Lin et al., 2021). Also, the points where filaments of the spacer meet and where filaments get into contact with the membrane tend to slow down feed flow and cause stagnant zones. This increases the potential for particulate and biofouling and as well as concentration polarization (Haidari et al., 2018; Kerdi et al., 2018; Lin et al., 2021).
Hence, feed spacer characteristics include appropriate geometry and thickness that promote mixing in the feed stream for mass transfer, keep pressure drop at the barest minimum, reduce scaling potential, and allow for maximum membrane packing density to form the module. All these coming together implies that there would always be a tradeoff (Park et al., 2016; Gu et al., 2017). Hence, it would be more appropriate to design spacers for specific feed types and applications to accommodate the tradeoffs involved.
2.2.2 Permeate spacers
Permeate spacers are used to create flow channels for the permeate water within the membrane envelopes, thus facilitating the collection of water by the permeate tube. Unlike feed spacers, permeate spacers have to provide mechanical strength for the membrane sheets to be able to withstand the high pressure of the feed (Haidari et al., 2018). Due to this functionality of providing mechanical strength, permeate spacers are woven and much more less porous as compared to feed spacers (Koutsou et al., 2013). The key parameters of the permeate spacer that influence the performance of the SWM is their permeability and thickness (Koutsou et al., 2015). These two parameters should be such that the spacer is porous enough to provide less resistance to fluid flow to minimize pressure drop in the permeate stream, while at the same time rigid enough to provide mechanical strength the membrane sheets (Koutsou et al., 2015; Haidari et al., 2018).
2.3 Typical plant overview
Water treatment plants that use SWMs typically consist of an abstraction and storage stage of the groundwater, a pre-treatment process before the water is filtered through the modules, and then post treatment and storage stages (Mengesha and Sahu, 2022). Figure 4 shows the flow of these processes. Pre-treatment is mostly carried out to reduce the amount or concentration as well as take out larger sized contaminants present in the feed water. Flocculation, coagulation, sand filtration, cartridge filtration, and anti-scalant dosing are various forms of pre-treatment unit operations. Pre-treatment can be by conventional methods or by membrane-based technologies. Conventional methods generally include the use of cartridge filters and ion exchange resins. Whereas membrane-based technologies include forward osmosis (FO), microfiltration (MF) and ultrafiltration (UF). In applications where the feed water has a very high TDS content, membrane-based technologies are preferred for pre-treatment because they can produce water with a low silt density index (SDI). SDI is used as a measurement of the potential of feed water to foul NF and RO membranes. A value between 1 and 3 is considered good and the water has a low fouling potential (Alhadidi et al., 2012).
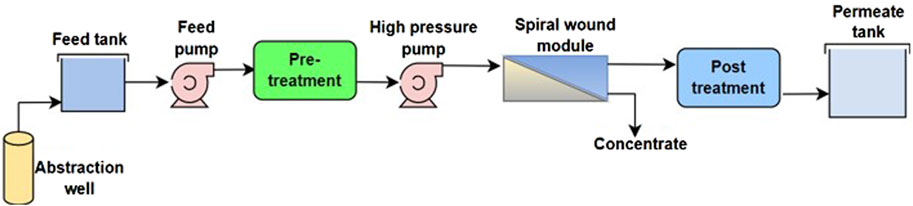
Figure 4. Schematic diagram of the flow process of a water treatment plant which uses spiral wound modules.
Post-treatment is mostly carried out to make the water suitable for specific applications. For instance, permeates intended for some industrial applications are further passed through ion exchange tanks or electro-dialysis to improve the purity of the water. Typical post-treatment unit operations include pH adjustment, degassing, disinfection and re-mineralization to add essential nutrients like potassium and phosphorus to the water (Semiat, 2010).
Some related technical terms include rejection, recovery, and specific energy consumption (SEC). Rejection is a term used to describe the performance of the plant with regards to the concentration of ions in the permeate water. A plant with a high rejection implies that the membranes can retain most of the ions (contaminants) and prevent them from permeating into the final product water.
Recovery is a term used to describe the performance of the plant with regards to water production, hence it is a ratio of how much permeate is obtained as against the amount of feed supplied within a given time frame (Srivastava et al., 2022). Rejection and recovery are usually calculated as a percentage.
The overall amount of energy required by the plant to operate is termed its SEC and is measured in kWh per m3 of permeate water produced. Each section of the plant contributes to the SEC. This includes the process of water abstraction, pre-treatment, membrane filtration, energy recovery devices if used, pumps, post-treatment, and concentrate disposal (Stillwell and Webber, 2016; Karabelas et al., 2018b).
3 Applications of spiral wound membrane module
3.1 Production of water for domestic use
Spiral wound modules can be used to remove various contaminants from groundwater to make it suitable for domestic applications. Bouhadjar et al. (2019) treated groundwater containing up to 57 mg/L of fluoride. About 98% of the fluoride was removed, resulting in a permeate concentration of less than 1 mg/L, which is below the world health organization’s (WHO) guideline value of 1.5 mg/L for fluoride in drinking water (WHO, 2017). This high rejection however meant that recovery had to be low, thereby generating a lot of concentrate. The concentrate was not discarded but recycled for sanitary use (toilet flushing, hand washing and laundry). However, the concentrate could have been handled more properly and more studies are needed in this regard. Especially, the effect of long-term direct contact of water containing high concentrations of fluoride on the skin, as previous studies have focused on its use as drinking water. Also, recycling it for sanitary use is fine but it also means that all the rejected fluoride goes back directly into the environment and eventually into raw groundwater. Du et al. (2020) studied the treatment of highly saline groundwater (up to 16 g/L) with NF and low-pressure RO SWMs. They observed that the modules were able to reduce the salinity of the water with rejection ranging from 46%–97% depending on the operating conditions. The flux and rejection of the NF modules are presented in Figures 5, 6. However, under the same operating conditions, the NF modules have a higher flux but reduced permeate quality as compared to the RO modules.
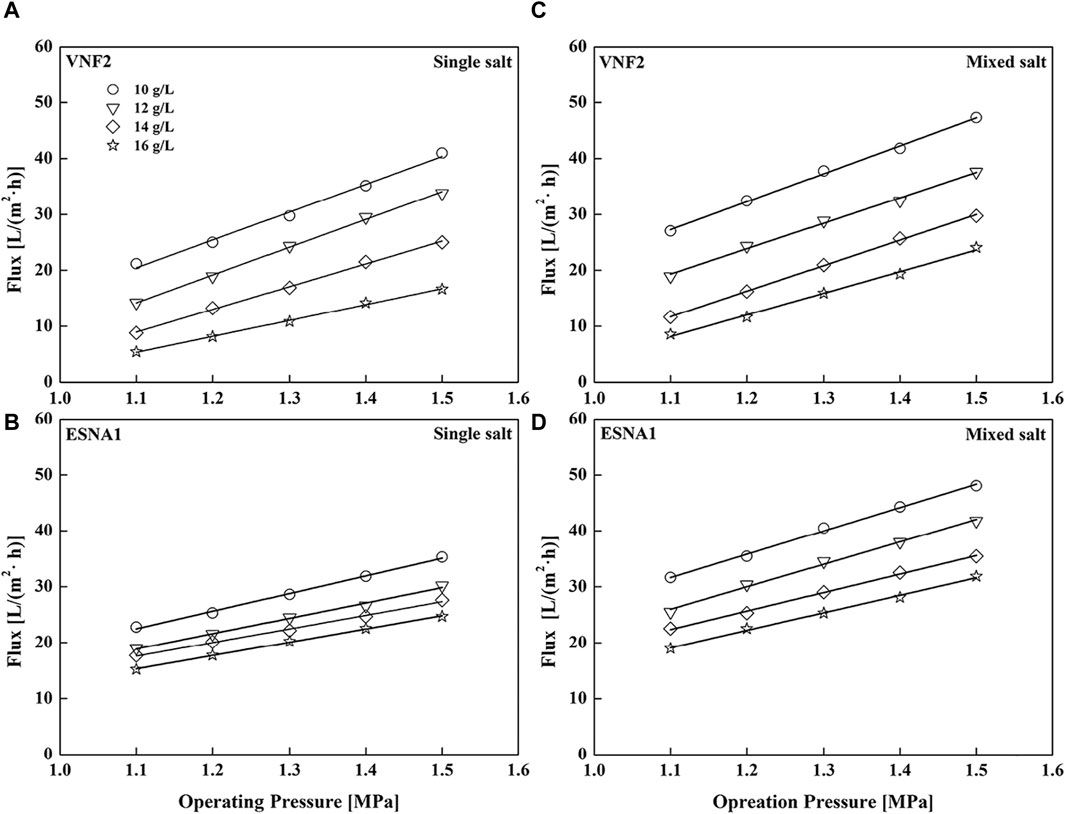
Figure 5. Effect of pressure on flux at 2 m3/h flow rate. NaCl feed solution (A, B) NaCl + MgSO4 feed solution (C, D) (source (Du et al., 2020).
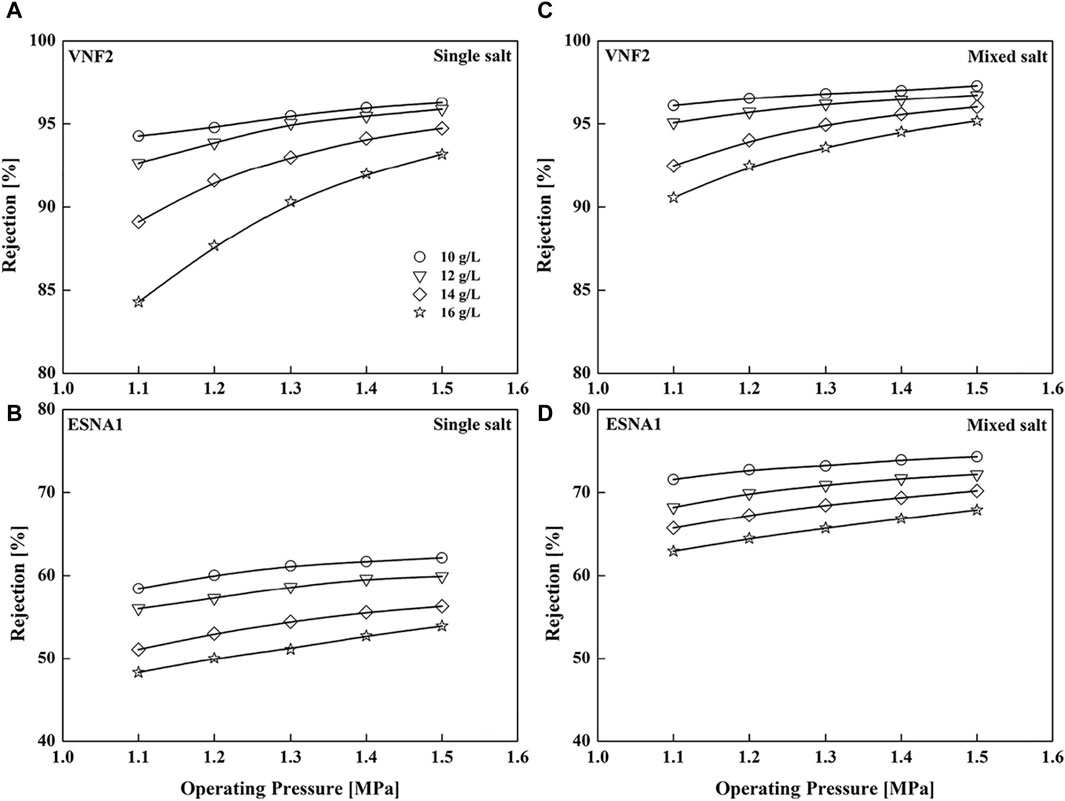
Figure 6. Effect of pressure on rejection at 2 m3/h flow rate. NaCl feed solution (A, B) NaCl + MgSO4 feed solution (C, B) (source (Du et al., 2020).
Karunakaran et al. (2022) studied the removal of chromium from groundwater. Optimum process variables of their study resulted in chromium removal of up to 98.38% and a permeate flux of 48.73 L/m3h. Chen et al. (2020) reported how they fabricated and optimized an NF TFC membrane which was subsequently packed into a SWM. The performance of the SWM is presented in Figures 7, 8. Iron, lead, manganese, zinc, and mercury were removed to only trace levels in the permeate. In the case of Cu and Zn, over 90% could be removed from the feed water. Over 95% of magnesium sulphate and about 40% of sodium chloride were rejected respectively by the SWM. In terms of pure water flux and magnesium sulphate rejection, the membranes performed better compared to some commercially available membranes.
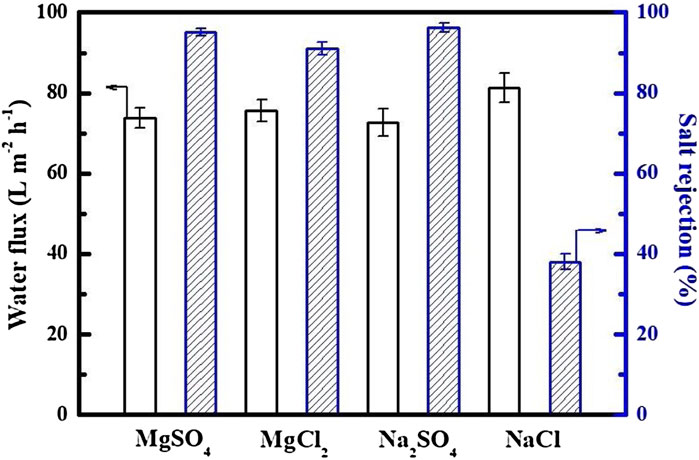
Figure 7. Water flux and rejection of 2 mg/L inorganic salts (source (Chen et al., 2020).
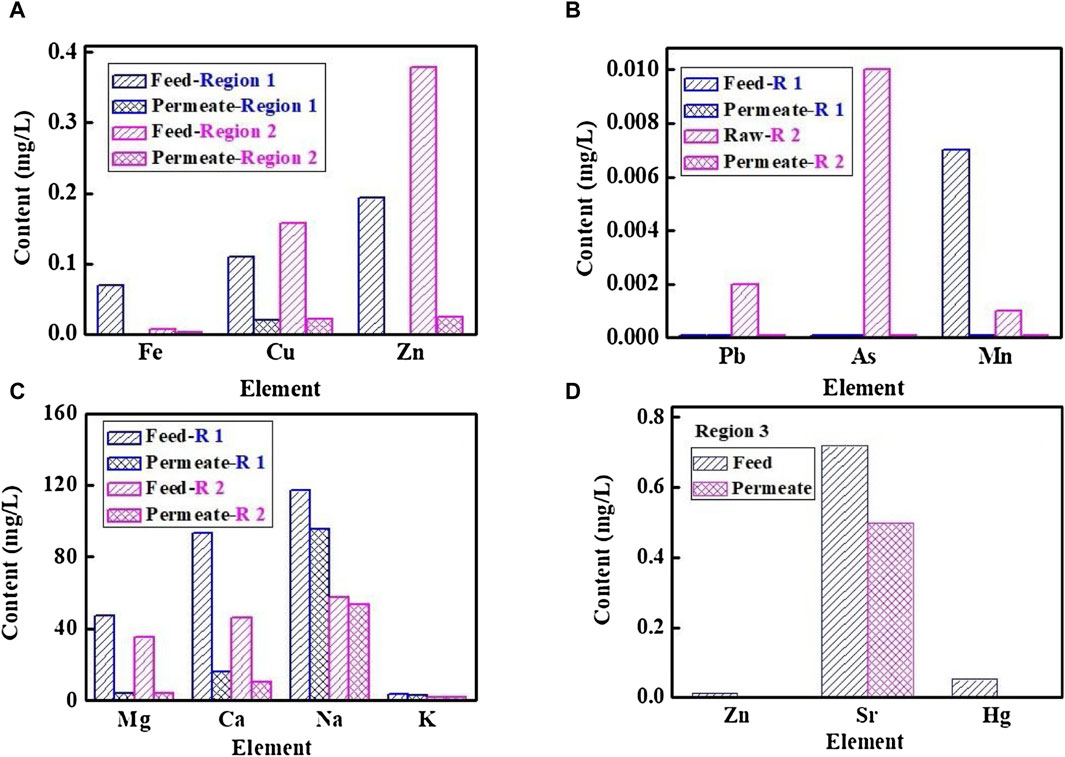
Figure 8. Rejection of Fe, Cu and Zn (A) Pb, As and Mn (B) Mg, Ca, Na and K (C), Zn, Sr, and Hg (D).
Liu et al. (2021) studied NF membranes for removal of per- and polyfluoroalkyl substances (PFASs) from groundwater and to subsequently degrade them with UV-sulfite. Over 95% of 12 PFASs identified in groundwater could be rejected by the NF SWM when operating at a permeate recovery of 90%. Upon the addition of RO SWMs, over 99% of the PFASs could be removed. The UV treatment could degrade over 90% of the PFASs in the NF concentrate after a contact time of 8 h. Degradation was however dependent on pH and the type and concentration of UV photosensitizer used. According to the Centers for Disease Control and Prevention (CDC) and Agency for Toxic Substances and Disease Registry, the immune system could be negatively impacted by exposure to high levels of PFASs. It is comforting to know that apart from the popularly known contaminants such as high salinity and heavy metals, NF SWMs can be used to remove PFASs from water sources.
Point-of-entry (POE) and point-of-use (POU) RO and NF modules are also available for treating groundwater for domestic use. POE systems are installed to treat water at the location from where it first enters a building. POU systems usually come in the form of a kit consisting of a pre-filter, the module itself, a post filter which can be installed on counter tops or under a sink. In some instances, the kit may include a storage tank and a faucet. A study by A. S. C. Chen et al. (2020) showed that these systems can be used to produce drinking water free of arsenic and nitrate.
3.2 Production of water for irrigation
Crop production requires water for good yield. In the absence of readily available surface water and rainfall, groundwater is relied upon for irrigation of crops (Fantaye et al., 2023). However, in some cases, the groundwater is saline and needs to be treated before it can be used for irrigation purposes. Saline water can increase the soil salinity and this makes the soil less suitable for crop production (Abbasnia et al., 2019; Zhang et al., 2021). Spiral wound modules can be used to treat groundwater for irrigation. A report by Ruiz-García and Nuez, (2020) stated that a full-scale brackish groundwater RO plant using BW30-400 Filmtec™ modules in a 2-stage arrangement had been used to treat groundwater for tomato cultivation. Although the water demand pattern resulted in the plant being operated under intermittent conditions, it had been successfully used for 14 years. During this 14-year period, the ideal SEC of the plant increased by about 20% and regular cleaning in place (CIP) could have potentially helped to maintain the initial performance of the system. In this study however, SEC calculations were done without factoring in other parameters such as anti-scalant dosing, performance losses and pumps. A comprehensive study encompassing all necessary parameters will go a long way to provide more comprehensive data on such plants.
4 Spiral wound module application modes
4.1 Continuous mode
Most often than not, treatment systems are designed to operate in continuous mode, with or without staging, as described in section 2. In the continuous mode of operation, there is a continuous supply of fresh feed water and the final permeate and concentrate streams are collected with no recirculation. Here, operating conditions are constant with time in all elements if minor fluctuations are neglected and major fluctuations are compensated for by adjusting the inlet feed pressure (Ahdab and Lienhard, 2021). All application processes discussed in section 3 are operated in continuous mode.
4.2 Batch application
In the batch mode application, a fixed amount of feed water is used to start the operation and only the permeate leaves the system. The concentrate is recirculated into the module(s) to recover as much permeate as possible. When the target amount of permeate is achieved, the brine is let out of the system by the introduction of a new batch of feed water. One of the advantages of this system is the reduced risk of fouling as a result of the flux being relatively uniform and regular flushing of the modules. In theory, this kind of operation should also result in high recovery with the use of less energy (Qiu and Davies, 2012a; Li, 2023). However, during the stage of letting out the brine, the new feed water for the next batch tends to mix with the brine, causing an increase in concentration of the stream being used in the subsequent batch (es). This phenomenon is known as longitudinal dispersion and makes the system use more energy than intended theoretically. Qiu and Davies, (2012b) sought to address this situation and developed a model relating longitudinal dispersion, recovery, and energy consumption, by analysis of these parameters at different flow rates. It was observed that operating in batch mode at fast flow rates can counter the negative impact of longitudinal dispersion. This model can be used to optimize a batch process to achieve somewhat high energy efficiency and significant high recovery. Recently, the group has reported the design and optimization of another model for a batch RO system for brackish groundwater treatment that uses a free piston instead of a forced piston (K. Park et al., 2020). In a forced piston system, a rankine cycle is used to drive the piston, and the piston is difficult to seal due to uneven pressure across it. The free piston was chosen because it does not have these shortfalls and an electric pump pressurizes water to drive the piston. The model was based on the use of a single 8-inch spiral wound module that either had a high rejection or high flux at a recovery of 0.8. They state that with this system, with regards to the high flux module, energy efficiency of brackish groundwater RO system increases from the current industry value of 15%–33.2%. In the case of the high rejection module, efficiency increases to 24.7%. The group has further experimented with simulated brackish groundwater that had a sodium chloride content of up to 5 g/L and at a pressure of up to 20 bars to validate the model (Hosseinipour et al., 2022). They observed that osmotic backflow contributes to a reduction in the system recovery, however, this can be countered by reducing the amount of brine let out of the module at the end of the treatment cycle. They also observed that with this system, an electrical SEC of less than 0.8 kWh/m3 at a sodium chloride content of 4 g/L is possible. This makes the free piston system an interesting choice for end user application, because with conventional RO operations, this SEC can only be obtained with a multistage arrangement, implying more cost in terms of modules.
4.3 Semi batch (closed circuit)
The batch mode has some drawbacks despite its benefits. These include the need for a large work exchange vessel, especially in the case of the free piston system. Also, when a recovery of more than 80% is needed, a large flexible bladder is required. To counter these drawbacks, a semi batch mode was proposed (K. Park and Davies, 2021). Additionally, the semi batch mode has flexible operation regimes which can be used to obtain various water recoveries by controlling the flushing and filtration time intervals (M. Li, 2023). The semi batch mode is also known as closed-circuit mode. Closed-Circuit Desalination (CCD) is the name of the semi-batch mode patent which has been obtained by DuPont (Efraty, 2009). In this operation mode, the concentrate is recycled by mixing it with fresh feed water and feeding both to the module(s). The flow rate at which the raw feed is added to the recycled concentrate matches that of the permeate flow rate (T. Lee et al., 2019). This system is designed to mimic the operation of a multi-stage RO process that uses booster pumps, by applying a pressure that varies temporally to filtrate the feed water (M. Li, 2023). A closed circuit RO (CCRO) system was used alongside a wastewater RO treatment plant in an attempt to increase the overall recovery of the treatment facility (H. Gu et al., 2021). The RO plant was a multi-stage system with 3 stages and operated at a maximum recovery of 85%. The CCRO was used in the form of a fourth stage to further treat the concentrate of the RO to obtain higher recoveries. After optimizing the operations of the CCRO system, a total treatment facility recovery of 92% was possible. Also, the interval for cleaning in place of the CCRO modules could be increased from 30 days to range between 63 and 73 days.
The operation of a CCRO, conventional single plug flow RO and 2-stage plug flow RO systems were investigated by (Futterlieb et al., 2021) in terms of the use of ion exchange as pretreatment, number of elements and pressure vessels required, and the use of anti-scalants. Their findings showed that with 13 pressure vessels (6 m), each containing 4 elements, CCRO coupled with ion exchange pretreatment, without anti-scalant dosing can lead to a recovery of about 94% as compared to both conventional plug flow RO systems that had the recovery being limited by hydraulics.
Li, (2023) sought to investigate the actual energy savings that the semi batch possess when compared to the multi-stage RO system using cyclic simulations. They conclude that when flux is used as basis for comparison between CCRO and multi-stage RO systems in the desalination of brackish water with low salinity, specific energy consumption is higher in CCRO. Also, potential scaling of the elements at the rear end of the process in CCRO systems is higher as compared to multi-stage RO systems. These imply that their findings do not validate the concept that semi batch systems have superior advantages over multi-stage RO systems in general. However, CCRO has its advantages and would be more likely advantageous for treating brackish water that does not have a high salinity as compared to sea water. This is because low to moderate salinity will eliminate the dangers of salt retention which tend to affect the semi batch system negatively in terms of SEC.
4.4 Hybrid processes
Some researchers propose the use of hybrid systems to maximize the potential of pressure driven membrane technologies. Based on this concept, there have been studies on RO and NF hybrid systems for treating groundwater. Lew et al. (2020) conducted a study to find out which water was best for irrigation, among raw groundwater, NF permeate, tap water and water from an NF/RO hybrid system. The hybrid system was composed of the NF permeate being pumped to the RO module as feed water. Subsequently, the concentrate from the NF module and permeate from the RO module are mixed in a ratio of 2:3 and used for irrigation. Theoretically, water from the NF + RO hybrid system should be of the best quality (rich in divalent ions and low in monovalent ions) for irrigation purposes. However, according to the study, when plant characteristics (height and number of leaves) are used as the basis to pick the best performing water source, there is no statistical difference among raw groundwater, NF permeate and NF (brine) + RO (permeate) hybrid system. But when fruit yield (number of fruits and weight) is used as the basis to pick the best performing water source, NF (brine) + RO (permeate) hybrid system was ranked first. This implies that the hybrid system is most suitable for crops which are grown for their fruits but not for their leaves.
A similar study (mixing of NF brine with RO permeate) was carried out by Nativ et al. (2021). The targeted application of their hybrid system is water for drinking purposes with reduced sodium chloride content. They conclude that divalent ions such as magnesium and calcium can be separated from raw groundwater using NF SWMs and stored for later use. These ions are contained in the concentrate of the NF process and a portion of it can be mixed with the permeate of the RO process to re-mineralize the water as a post-treatment step, to make the water suitable for drinking. Also, the hybrid system can achieve a total recovery ratio of 85.5% and has a competitive price of $ 0.242/m3, which is comparable to the price of existing groundwater desalination which is between $ 0.07 - $ 0.79 (Anis et al., 2019; Lesimple et al., 2020). Furthermore, the initial NF step can reduce the potential of scaling of the RO modules, thereby increasing their replacement intervals.
A hybrid system of a batch and semi-batch system has been proposed and studied by (K. Park and Davies, 2021). The study consisted of modelling a semi-batch, a batch and a hybrid of both systems to compare their performance in terms of energy consumption and recovery. The concept is that this system will maximize the advantages of the individual systems, as the individual pros and cons are opposite to each other. A cycle in this system consists of three steps; application of pressure by the semi-batch method (that is variable pressure), followed by the application of pressure by the batch method (fixed pressure) and then the introduction of a new batch of feed water to remove the brine from the system at predetermined times. They conclude that the hybrid process provides a compact system when very high recoveries are needed (up to 95%), since the size of the work exchange vessel can be reduced to as low as half of what is required in the conventional batch system, by starting the cycle with a semi-batch process. Then salt retention which leads to higher SEC in the semi-batch process is prevented by switching to a batch process. The system they propose, and the results obtained are interesting, but the study is based on a low salinity (3,000 mg/L) groundwater and a single spiral wound module. Further studies with multiple spiral wound modules and higher salinity brackish water are necessary to have a detailed overview of the performance of this hybrid system.
A model was developed and validated by (Srivastava et al., 2022) to compare RO, NF and hybrid RO-NF systems for groundwater treatment. It was observed that the RO system had a very high rejection (99.05%), a very low recovery (18. 8%) and a high SEC of 5.09 kWH/m3. The NF system had the lowest rejection (21.88%), a considerable recovery (30.89%) and the lowest SEC (2.46 kWH/m3). The hybrid system on the other hand had a rejection of 39.89%, the highest recovery which was 40.35% and a SEC of 3.8 kWH/m3. In essence, all three systems are suitable for treatment of groundwater, as rejection is above 20% for each of them. Hence the choice of which system to adopt should be influenced by the application of the treated water. High specific energy consumption can be reduced using energy recovery devices if feasible and staging can be used to increase the recovery of the system. The same group previously developed 2 different models, 1 based on response surface methodology (RSM) and another based on artificial neural network (ANN) to compare 3 types of hybrid systems (Srivastava et al., 2021). The hybrid systems were: 1) a parallel arrangement of NF and RO modules (NF-RO) where the permeate from both were collected together as one type of water; 2) a series arrangement where the concentrate of the RO module was fed to the NF module and permeate from both modules were collected together as one type of water (RO-C-NF); and 3) Another series arrangement were concentrate from the NF module is fed to the RO module and permeate from both modules are collected together as one type of water (NF-C-RO). They observed that the parallel NF-RO system had the highest recovery, which is 57.18% and a rejection of 44.89%. The series RO-C-NF system had a recovery of 49.55% and rejection of 38.64%. Whereas the series NF-C-RO system had the highest rejection of 49.66% and lowest recovery of 39.53%. Thin film composite (TFC) membranes which are typically used for spiral wound membranes are known to have a recovery-rejection trade-off (Di Vincenzo et al., 2021a). This is clearly validated by the findings of both studies (Srivastava et al., 2021; Srivastava et al., 2022) by the group. This indicates that each configuration will be suitable for a specific intended application, as stated earlier. Water channels have been introduced and proven to help overcome this recovery-rejection trade-off (Di Vincenzo et al., 2021b; Huang et al., 2021). It would be interesting to know the percentage recovery and rejection of the configurations when the membranes are incorporated with water channels.
5 Challenges in the use of spiral wound modules
Spiral wound membrane modules were invented in the 1960s (Wang et al., 2011) and since then, there have been various studies, targeting different aspects of their operation, to improve performance as well as make them competitive in the commercial space. Despite the improvements, challenges still prevail.
5.1 Damaged modules
SWMs can become damaged in service, thereby compromising their integrity. The damage could be mechanical or chemical. Mechanical damages can occur due to incidents such as failure of envelope glue lines, friction caused by solid particles that accidently get into the feed stream, indentation of membrane surface by housing materials and feed spacers and failure of connectors and sealing O-rings. Aging and long-term exposure of modules to cleaning chemicals and chlorine can cause chemical damage to SWMs (Tian et al., 2023). These damages tend to reduce the performance of the modules over time and shorten their life span, which eventually increase operational cost.
5.2 Fouling
Fouling is said to have occurred when the membrane surface or pores gets covered with or filled, respectively with particles and solutes from the water being treated (Ismail et al., 2019b). The performance of membranes is reduced when they get fouled. This happens to be one of the drawbacks limiting the full potential of membrane systems. More so with spiral wound modules due to their complex physical nature. A clear understanding of foulants and the mechanisms by which they foul membranes is key to improving the overall performance of the modules. Due to the variable nature of groundwater over a period, fouling mechanisms can be complex as compared to other water sources such as sea water (Ruiz-García et al., 2018). The most important benefit of the pre-treatment step is that it has the potential to promote the production of high quality permeate and to protect the SWMs from fouling and scaling. Consequently, the frequency at which they must be cleaned is also reduced, thereby increasing production time and replacement intervals (Khanzada et al., 2017). A study was carried out by Khanzada et al. (2017) to compare three types of pre-treatment options for treating brackish water. They observed that FO and the UF were the best options as these pre-treatments resulted in RO feed water with less potential to foul the membrane modules. However, the cost of using these methods was higher as compared to the cartridge filters. Groundwater mostly tends to have a varying characteristic over time, giving it a high fouling potential. Hence the high cost of using FO and UF is justified for the long haul.
Apart from pre-treatment, there are other operating parameters that must be considered for optimum results from SWM water treatment systems. These include pressure, temperature, salinity, pH and feed flow rate among others. Feed flow rate, temperature, salinity and pressure were investigated by Al-Obaidi et al. (2018) to ascertain their impact on the performance of a brackish groundwater treatment plant using RO membranes. They observed that when feed flow rate is increased up to 20%, there is a corresponding significant decrease in the total recovery of the plant, but rejection increases very insignificantly. However, at a much higher percentage increase in feed flow rate, a corresponding significant increase in rejection is observed (Figure 9A). A 20% variation in feed temperature causes a slight reduction in rejection because of an increased flux of ions through the membranes. Hence, recovery increases but salinity of the permeate also increases considerably (Figure 9B). In the case of salinity, a 20% increase resulted in a decrease in the rejection capacity of the plant (Figure 9C). In the case of the operating inlet feed pressure, a 20% increase does not cause a significant change, but just a slight increase in the rejection of the plant, salinity of the permeate decreases by 22% and plant recovery increases by 13.4% because of increased flux (Figure 9D). The impact of these parameters on system performance is evident and must be optimized for specific requirements.
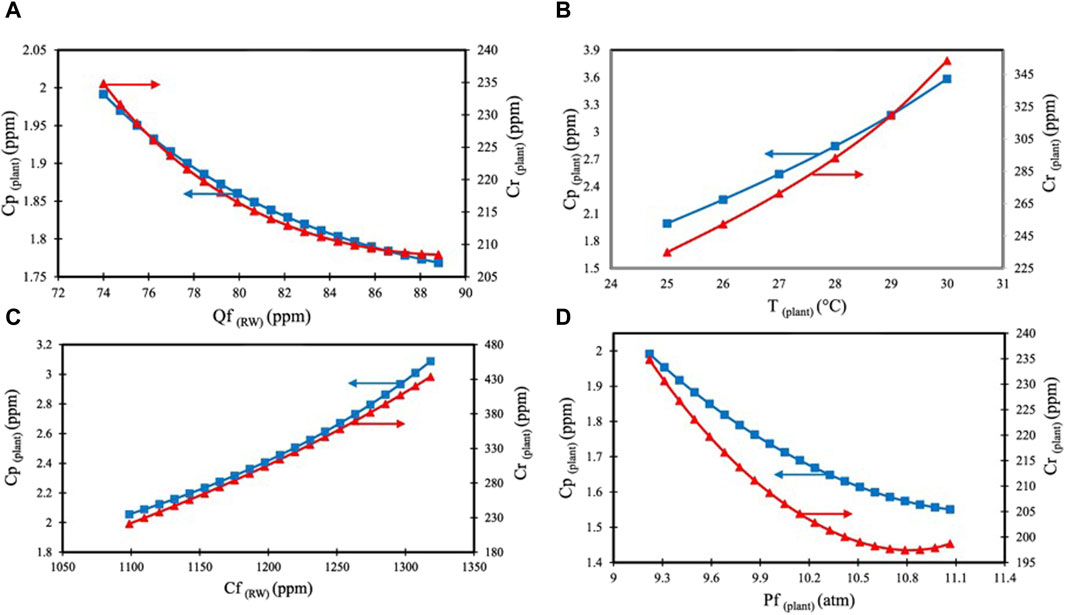
Figure 9. Impact of feed (A) flow rate (B) temperature (C) salinity (D) pressure on permeate (Cp) and retentate (Cr) concentrations (source (Al-Obaidi et al., 2018).
The kind of foulants and the manner in which they are transported and distributed in spiral wound modules that had been used for about 5 years to produce drinking water from raw groundwater was studied by Kim et al. (2015) using the membrane autopsy technique. Their observation was that dissolved organic components (DOC) were the cause of fouling in the membranes and the main foulant was fulvic acid. The minor foulants were complexes that had been formed between fulvic acid and ions of either iron or aluminium. With regards to the distribution of the foulants, there were more layers on the back side of the membrane sheets than there were on the front side. Water quality analysis showed that the feed water had 1.8 mg/L of DOC and the pre-treatment steps employed by the plant were ineffective in removing a significant amount of the foulants from the raw water before it gets to the membrane modules. Hence causing the membranes to get fouled and this could also potentially impact the aesthetics of the permeate water in storage. The implication is that it is necessary to design pre-treatment to suit the quality of the raw feed water, as this can help to minimize fouling rate.
This concept is clearly evident in a study conducted by Ruiz-García et al. (2020). Groundwater feed data collected over a 10-year period was used to predict by the solution-diffusion transport model the available operating windows, in terms of feed flow and feed pressure for a 2-stage brackish water RO (BWRO) plant. The configurations that were considered for the study were 3:2 and 2:1. They conclude that the characteristics of the feed water greatly affect the performance of the BWRO systems, however a wide operating window was observed in both configurations, although the 3:2 had the highest. Also, BWRO systems should be designed to be flexible in order to fit into several operating windows, influenced by fluctuating feed characteristics. This notwithstanding, real-time control strategies should be implemented to obtain optimum performance. Incorporation of flexibility into the system design of plants to be able to operate with varying feed water characteristics is very important, since factors such as seasonal changes tend to affect groundwater quality.
The membrane autopsy technique, in addition to performance data was used by Ruiz-García et al. (2018) to also characterize fouled SWMs. The study showed that diatoms, calcium carbonate and aluminosilicates were the main foulants. The diatoms had formed biofilms on each of the modules. In terms of the distribution of the foulants, the quantity of deposits decreased along the array and were of different compositions. The performance data showed that the fouling had increased the resistance of the membranes by over 2 folds over a11-year period, but normalized salt rejection was fairly constant. However, although salt rejection did not decrease much, the efficiency of the system dropped. It is inferred from this study that with the occurrence of this kind of foulants, the modules can be in service for a decade with the implementation of suitable operating procedures, to compensate for the cost of initial set-up.
Jiang et al. (2015) proposes a procedure known as high salinity direct osmosis backwash (HS-DOBW) which can be used to clean NF membranes that have been fouled from the filtration of groundwater. In this procedure, a solution with high salinity is pumped into the module as feed water, at the same time, permeate is pumped back into the module in the reverse direction in the form of a backwash. Since the permeate has a higher water chemical potential, it passes through the membrane towards the high salinity solution which has a lower chemical potential. In the process of passing through the membrane, it causes the foulants that have been deposited on the membrane to be lifted or detached from the membrane, and the feed water sweeps them off towards the brine valve to be taken out of the module. They report that fouling is almost reversible with HS-DOBW as about 99.78% of the initial permeate is recoverable after cleaning. However, they do also state that cleaning is much easier in the initial stages of using the membrane since the foulants are inorganic. When organic fouling sets in with time, cleaning becomes much more difficult. It is obvious that this procedure will result in a large quantity of concentrate to be handled and disposed of, and this currently an undesired situation. Also, the study was carried out with NF membrane sheets, further research using NF SWMs is required to know if this procedure can be used to clean membranes in real desalination applications as current module and case design do not allow for sweep introduction, unless they have been modified for this purpose.
Fouled membranes can increase the production cost of a plant. A key parameter that can give an indication of fouling or the extent of fouling, so that mitigation measures can be employed, in the SWM is pressure drop. Pressure drop is the difference in the pressure of the feed water entering the pressure vessel and the concentrate stream leaving the pressure vessel. A model that takes fouling into account for predicting pressure drop in full scale BWRO plants was developed by Ruiz-García and Nuez, (2021). It was realized that the model had to be time dependent, because pressure drop increased with increasing operating time, because of fouling of the membranes over time. Furthermore, pressure drop increases very fast in the beginning when the plant starts operating but stabilizes after the plant has been in operation for a long term. Pressure drop is one of the important parameters to monitor in spiral wound membranes, because it is a subtle indicator of the occurrence of fouling in the membranes and is directly proportional to the energy consumed by the plant. Pressure-drop values also give an indication of how much pressure is required to be able to pump the feed water through the membranes.
5.3 Scaling
Scaling is a type of fouling that is caused by inorganic constituents. These inorganic constituents are mostly present in water as TDS and may include magnesium carbonate, calcium carbonate, barium sulfate, calcium sulfate, silica, and metallic silicates. When these inorganic constituents exceed their solubility limit, they precipitate into crystals that grow over time and form scales on the membrane.
A significant reduction in performance of a 2-stage RO plant used to treat groundwater after 2 years in service led to an autopsy being performed on some of the modules by Karmal et al. (2020). Scaling was observed and found to be unevenly distributed on the membrane sheets. The scales had a brownish colorization and were more profound in membranes closest to the feed water and the intensity decreased along the membrane length towards the concentrate outlet, because of sedimentation of contaminants in the feed. However, scaling was more severe in portions of the membrane at the concentrate outlet, with the formation of a thicker scale layer that decreased towards to the feed side of the membrane due to higher concentrations in this region. The scales were mainly composed of inorganic constituents. Authors state that the raw groundwater was very saline, approximately 4,000 ppm. However, pre-treatment did not include any protection of the modules against scaling. This has the potential to reduce the in-service time of the modules. A filter bed of sand and anthracite, followed by microfiltration, constituted the pre-treatment process. The feed water can be dosed with anti-scalant before pumping to the modules, this will reduce the scaling potential and extend the service life of the membranes. Anti-scalant are mostly chemicals that act by being absorbed unto scale forming constituents and prevent them from precipitating or growing into large crystals (Singh, 2015; Karmal et al., 2020). It is by this mechanism that the scaling potential of the membranes is reduced.
Electromagnetic field (EMF) has also been proposed by Jiang et al. (2022) as a tool to control scaling of spiral wound modules used to treat groundwater and to remove previously formed scales in the whole treatment system. The impact of EMF on low salinity and high salinity feed groundwater was assessed using RO modules. Their study showed that EMF is an effective tool to control scaling of the membranes. The scale layer was not strongly adhered to the membranes and could be easily rinsed off with water. This was observed for both low and high saline groundwater. In the case of removing previously formed scales, scales in pipes and tanks could be removed but not from the membrane sheets. Also, EMF can be used in place of chemical and relatively costly anti-scalants, to protect the modules. However, the EMF is generated by the energy of the feed water flowing towards the membrane modules, hence optimal performance can only occur at high flow rates (113.5 L/min is recommended). This implies that scaling control with EMF is not suitable for low flow rate RO brackish groundwater systems like one which would be used for a small rural community.
5.4 Waste disposal
Several waste streams associated with SWMs are generated. Major ones include spent or damaged modules, cleaning in place chemicals and concentrate. SWMs have an average life span of between 5 and 10 years. It is estimated that by the year 2025, about 30, 000 tons of spent SWMs would have been generated globally, thereby raising concerns for their disposal (Grossi et al., 2024). Currently, available disposal options include landfilling, reuse, recycling, and incineration with some associated limitations such as transportation costs and not being environmentally friendly (Tian et al., 2023). Environmental and socioeconomic assessment by (Grossi et al., 2024) and a life cycle assessment by (Lawler et al., 2015) propose reuse and establishing of disassembly plants to recover parts for recycling as the most viable options.
Regular cleaning of SWMs is carried out to restore permeate flux and/or prevent severe fouling of the membranes. When chemicals are used, the issue of how to dispose of them safely arises together with other problems such as handling and transporting costs. The development and use of greener chemicals and methods are necessary to overcome this challenge. Some researchers propose the use of osmotic back washing (Alnumani et al., 2024).
Innovative means have been sought to safely handle concentrate streams. One of such is to inject the concentrate into deep confined aquifers with similar characteristics. A system based on this concept was designed and piloted by Haidari et al. (2017) and nicknamed Put-RO (PURO). The system consists of a Brackish water reverse osmosis unit which is installed in a well. Brackish water is abstracted and filtered by cartridge filters directly without chemical pre-treatment, then through the spiral wound modules and pumped out of the well to be added to water from a local pumping station for distribution. The concentrate is then injected in an aquifer of similar concentration. The advantages of the system are that no chemical pre-treatment is used. As a result of this, no xenobiotic compounds are discharged into the environment through the concentrate. Also, in comparison to convention BWRO systems of same capacity, this concept reduces energy consumption by about 39%. Although this concept is environmentally friendly, it is saddled with some downsides. A typical one is that the system must be operated at only 50% recovery to be able to meet the 39% decrease in energy consumption. In addition to that, a lot of technical expertise and initial investment cost would be required to install and operate such a system.
Another school of thought in handling concentrate is to have a system that generates little (minimal liquid discharge (MLD)) or perhaps no concentrate at all (zero liquid discharge (ZLD)). The concept of MLD was explored by Park and Davies, (2021) through the study of a batch/semi batch hybrid system. The system can recover as much as 95% of the feed water, producing just a minimal amount of concentrate for handling and disposal. Z. Wang et al. (2020) proposes the use of low-salt rejection RO membranes (LSRRO) for the realization of MLD or ZLD, by employing moderate hydraulic pressures that result in relatively lower SEC as compared to conventional RO systems that use only high salt rejection membranes. LSRRO is a multi-staged process that uses a high salt rejection RO membrane only in the first stage and low salt rejection (loose) membranes in the subsequent stages. Only permeate from the first stage is collected as freshwater while permeate from the subsequent stages are recycled as feed for the stage that precedes it. In this way, there is minimal to almost no concentrate collected in the final stage for disposal.
5.5 Energy requirement
Energy is a key component in the operation of SWM water treatment systems. The challenge when it comes to energy is the high cost involved in obtaining it from the source, and the potential environmental risk when the source is not sustainable or disposal of energy storage systems such as batteries. In recent times, attention is being shifted to sustainable energy sources such as wind and solar systems. Usually, solar powered systems are used for water production or treatment when the sun is shining and then shut down when the sun sets. This is known as intermittent operation and mostly carried out in an attempt to reduce overall production cost in terms of energy storage systems. However some researchers have reported that intermittent operation can increase the fouling rate of the membranes (Moudjeber et al., 2014; Cipollina et al., 2015; Freire-Gormaly and Bilton, 2018). Freire-Gormaly and Bilton, (2018) recommends some steps that can be taken to reduce the fouling rate of membranes used intermittently to treat groundwater. They propose the use of anti-scalants and rinsing of the SWMs before each shutdown in intermittent operation to control the fast rate of fouling which this operation method is associated with.
To overcome the problem of intermittent operation when the energy source is weather dependent, electrical energy storage systems such as batteries and hydrogen energy systems are used. However, high energy losses are incurred during charging and discharging of these storage systems and there is an environmental risk when it comes to their disposal. Sanna et al. (2021) proposes a pumped storage system. In their study, solar energy is stored in the form of hydraulic energy instead of electrical energy to overcome charging and discharging energy losses. They concluded that a connection to an electricity grid or an electrical energy storage system such as a battery is still required if a plant powered by solar and hydraulic energy sources is to provide drinking water on a continuous basis. Also, a minimum of a 34-kW nominal power solar plant is required to produce 100 m3 drinking water per day from brackish groundwater with a salinity of about 5 g/L and a borehole depth of 100 m. Finally, on average, only 35.7% of energy produced by the solar plant is used to directly produce drinking water, in terms of extraction of the groundwater, pre-treatment, RO filtration and post-treatment, on an annual basis. Over 60% of the remaining energy is used to charge the pumped storage system and battery. Although this study provides a vast amount of technical information, a solution for treating groundwater on a continuous basis without a battery was not obtained. Furthermore, pumped storage requires a specific geographic site (which are not readily available) for it to be feasible. In this study for instance, a mountain was used.
A study was conducted by Boussouga et al. (2021) to ascertain the influence of the feed water quality and type of membrane on a solar powered system that operates on fluctuating energy supplied by a Solar System without a means of energy storage. This implies that the system was connected directly to solar power and no battery was used for back-up power. The key focus was to identify how the system absorbs and adapts to the shock of the fluctuation and how quickly it moves to the initial or new state that corresponds to the energy being supplied because of the fluctuation. They observed that the system is more affected by the membrane type (Figure 10) as compared to the feed water type (Figure 11). They then conclude that, if the permeate quality is of primary importance, tight membranes are recommended as against loose membranes. Furthermore, they do recommend feeding water with a low electrical conductivity (441–907 μS/cm) for such a system, as only a small variation in quality was observed as compared to feed water with a high electrical conductivity (3,340–4,940 μS/cm). Further study is required with focus on other groundwater contaminants such as heavy metal ions and the use of sensors which are contaminant specific, to truly know how such systems can be operated for optimum performance.
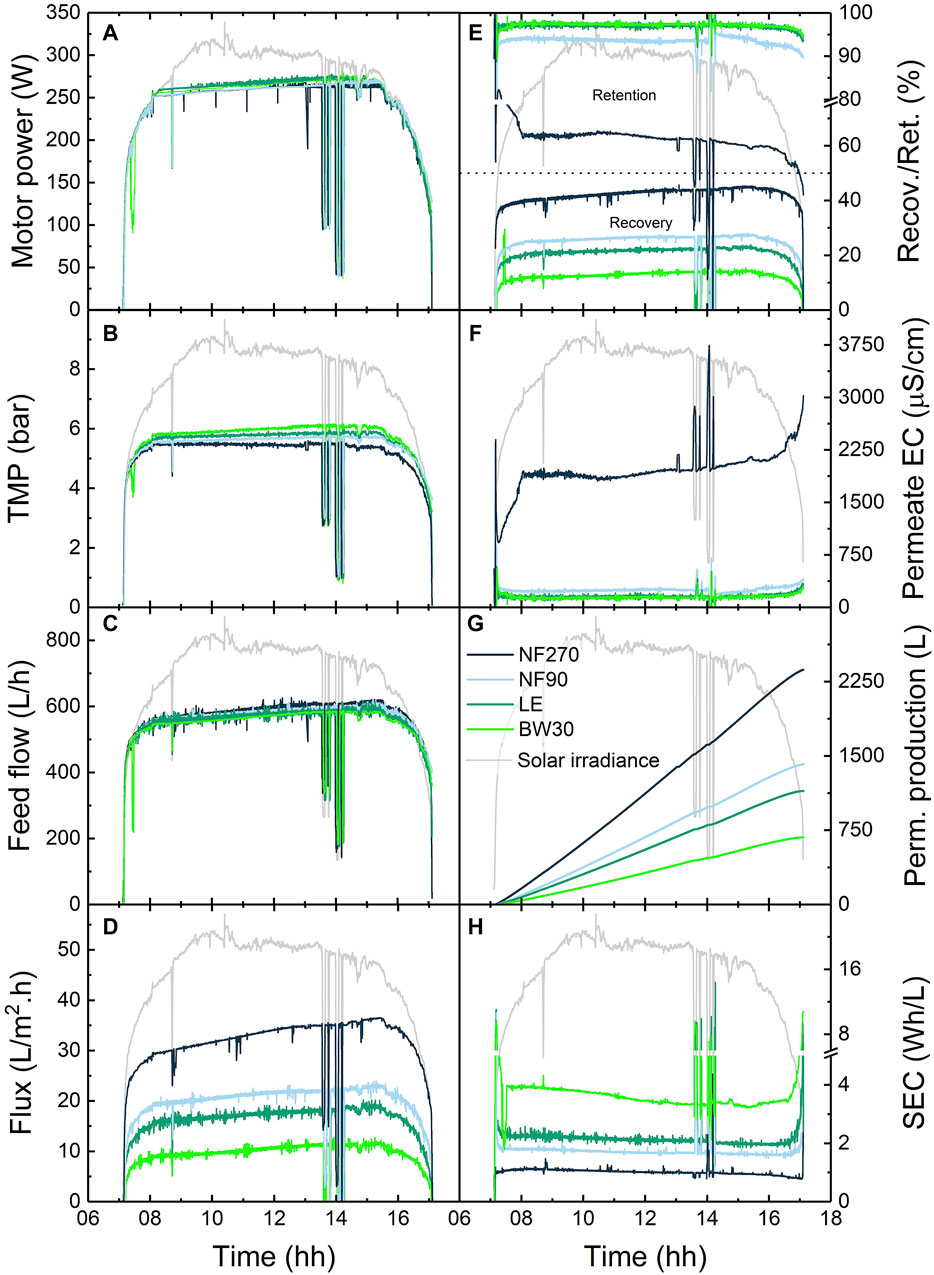
Figure 10. Effect of different membrane modules on motor power (A), transmembrane pressure (B), feed flow (C), flux (D) retention and recovery (E) permeate EC (F) permeate production (G), specific energy consumption (H).
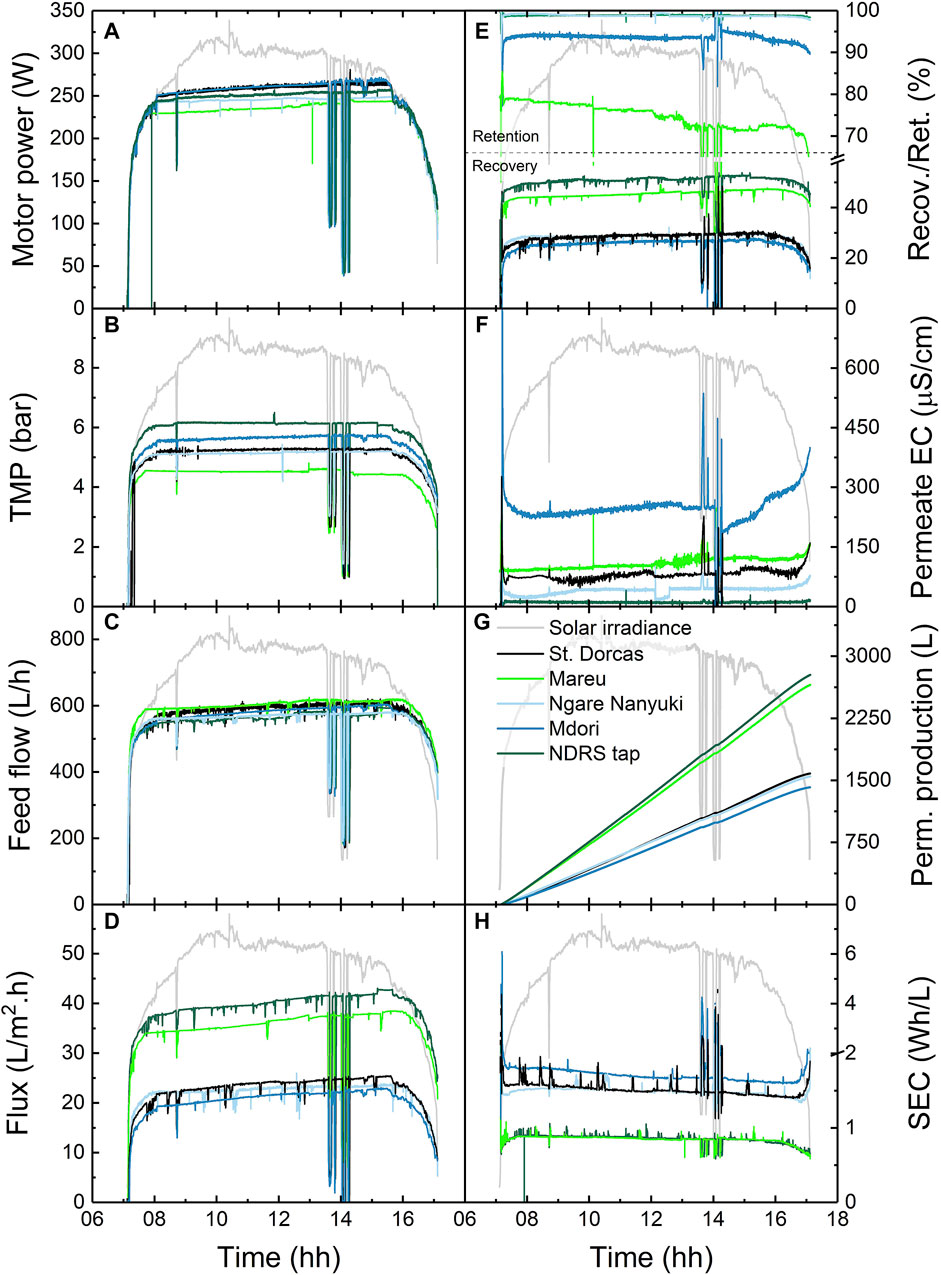
Figure 11. Effect of different feed water types on motor power (A), transmembrane pressure (B), feed flow (C), flux (D) retention and recovery (E) permeate EC (F) permeate production (G), specific energy consumption (H).
An advanced and conventional exergy analysis was conducted on a BWRO plant which had been operating intermittently for 14 years to identify where and how exergy destructions occur in the system in order to make recommendations for better energy performance (Fellaou et al., 2021). A schematic diagram of the plant is presented in Figure 12. Exergy is defined as the maximum or in some cases, the minimum amount of work that can be done by a system during the processes of being brought to thermodynamic equilibrium with its environment. In simple terms, it is a measure of the quality of an energy or how useful an energy can be. Energy cannot be destroyed but exergy can be destroyed or consumed in real applications due to irreversibilities that occur (Banerjee et al., 2019). They report that, according to conventional exergy analysis, the cartridge filters had the highest efficiency (about 98%), followed by the feed pump (about 62%), and then the high-pressure pump (about 59%). The RO system (membranes) had the least efficiency which was about 18% and there is a significant exergy destruction within the RO system that is unavoidable (about 19.78 kW). However, the unavoidable exergy destruction which occurs can be reduced by employing the right chemical cleaning processes to control membrane fouling, using membranes with improved properties and reducing pressure drop to the minimum, among others. Furthermore, 70.61%, 92.94% and 7.83% of the exergy destruction in the high-pressure pump, feed pump and RO system respectively, are avoidable according to advanced exergy analysis. The exergy destruction associated with the pumps can be reduced by using pumps that have higher performance efficiencies and energy recovery devices. Operating conditions as well as pressure control valves are other components which have been identified to contribute to exergy destruction in BWRO plants (Sutariya and Amaliar, 2023). A SWM treatment plant relies heavily on energy for its operation and these studies break down the exergies of the various main components of the plant. This information is critical for optimization of the processes in terms of energy consumption and improvement.
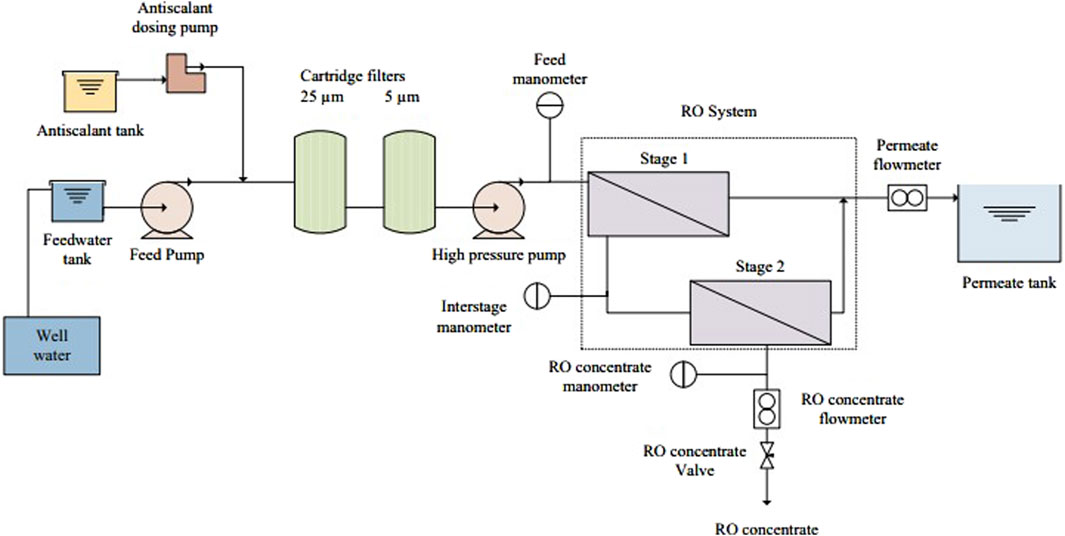
Figure 12. Schematic diagram of the unit operations of the BWRO plant (source (Fellaou et al., 2021).
The impact of adding an energy recovery device (ERD) to a medium scale BWRO plant was evaluated by (Alsarayreh et al., 2020). The ERD’s impact was studied at 2 different operating efficiencies, that is 80% and 90%. In this study, the ERD is used to recover the energy contained in the concentrate stream of a first RO stage and use it to feed the permeate of the first stage to a second RO stage. They observed that by including an ERD in the plant design, a reduction of between 47% and 54% in total energy consumption is obtained when the device operates at an efficiency of 80% and 90% respectively. They do also state that there will be an initial increase in the cost of production when an ERD is installed, however, over the long term, this cost will be reduced due to the reduction in cost of input energy required to operate the plant. Over the years, the SEC of desalination plants have reduced significantly. One of the factors that account for this is the introduction of ERDs. This study shows that existing plants can be reconfigured for the installation of an ERD for a reduction in the plant’s energy input requirement. However, there are different types of ERDs, and they operate with different principles and varying efficiencies and recoveries. Apart from the efficiency of the ERD, authors did not state which ERD their study was based on.
5.6 Chlorine exposure
Polyamide membranes, which are normally packed into SWM tend to fail when it is in contact with chlorine. Chlorine is an oxidant hence can be used as a pre-treatment unit operation to prevent biofouling of membranes (Yao et al., 2019). Although steps are taken to remove the chlorine from the feed water before it gets into contact with the membrane, the residue can cause eventual failure of the membrane. The tolerance of membranes to chlorine is denoted by the time of exposure and the chlorine concentration. This was however not validated by some studies. It was observed that other factors may contribute, in addition to chlorine concentration and exposure time to the chlorine tolerance of thin film composite polyamide membranes (García-Pacheco et al., 2019). Based on this, Xie et al. (2022) sought to determine the synergistic effect of chlorine and iron on an NF TFC membrane (NF90 from Dow Filmtec™). They observed that between chlorine concentration and exposure time, concentration was a more dominant factor in the degradation of the membrane. Furthermore, they observed that degradation was more pronounced (increased water flux and reduced salt rejection) in the presence of iron as compared to when the membranes were exposed to only chlorine. Although more studies covering other pH regimes and iron species such as Fe3+ are required for system optimization for a wide range of feed water quality, this study is quite important especially for groundwater treatment. According to the WHO, iron is the most abundant metal in the earth’s crust after aluminuim, and easily forms compounds with sulfur and oxygen to end up in the environment, with pronounced presence in groundwater around the world (WHO, 2017). Iron can also find its way into water at the treatment plant in the form of coagulants and catalysts and when equipment and piping systems corrode. Chlorine is also often used as a disinfectant in water treatment. Hence knowing their synergistic effect on membrane degradation is crucial.
6 Opportunities
Spiral wound module water treatment systems are considered as energy efficient systems, however, in practice, they use quite a significant amount of energy, especially the high-pressure pumps, as compared to what it can theoretically be (Shaaban and Yahya, 2017). Hence, further studies are still required to reduce the amount of energy required by the systems, integrate less expensive sources of energy to power the systems or use sustainable energy sources effectively and efficiently. Renewable energy has been proposed Ghazi et al. (2022) as an alternative to energy sources such as electrical, thermal and fossil fuels which are not environmentally friendly and, in most cases, not sustainable. Wind and solar energy are the common sources of renewable energy that have been used to power spiral wound module systems such as RO and NF (Ghazi et al., 2022). These are however controlled by weather patterns and may not supply energy continuously. This results in fluctuations in energy supply and sometimes intermittent operation is employed. There is a need to develop efficient and durable energy storage systems to maximize their potential.
Furthermore, incorporation of flexibility into the system design of plants to be able to operate with varying feed water characteristics is very important, since factors such as seasonal changes tend to affect groundwater quality. Artificial intelligence (AI) for modelling and optimizing operational parameters will be a beneficial tool in this regard (Al Aani et al., 2019). Artificial neural networks (ANNs), an AI tool was used by Roehl et al. (2018) to obtain information on fouling in the first stage of a full-scale RO plant. ANNs were used to develop models that can predict the occurrence and mechanism of fouling based on data that had been gathered over a 6-year period from the RO plant. Similar approach, that is the use of ANNs or other AI tools such as genetic algorithm (GA) can be used to develop models to predict other performance indicators and optimize process parameters in groundwater treatment without the limitations that theoretical models are faced with. In the same vein, there is a need to improve pre-treatment technologies to produce feed water of high quality to protect the modules to increase their service life.
Thin film composite membranes that are packed into spiral wound modules are often saddled with a selectivity and permeability trade-off. Developing membranes that overcome this challenge is still an area of great research interest. Artificial water channels have been proposed in this regard, however, there is still a challenge of up-scaling with these membranes (Fuwad et al., 2019; Abaie et al., 2021; Di Vincenzo et al., 2021a; Huang et al., 2021). There is a need for further and extensive research in incorporating water channels into thin film composite membranes to obtain membranes with high selectivity and permeability without trade-offs for full scale desalination purposes. This will go a long way in contributing to overall high system performance. In the same vein, the membranes are often saddled with limited selectivity for some applications. Improving the selectivity will contribute to improving the separation efficiency of the modules. Some researchers are adopting and coupling the interfacial polymerization process with unconventional materials and other techniques to make tailored membranes. Popova et al. (2024) carried out the interfacial polymerization process with a spin coater and used a track-etched MF membrane as a support instead of the commonly used sponge-like UF and MF supports. The membranes had a high performance for rejection of bacteria-sized particles.
Feed spacers play a major role in the performance of SWM, hence enhancing their hydrodynamic and mass transfer properties is of great importance. Advanced modelling, simulation, manufacturing, and characterization techniques such as, computational fluid dynamics, computer aided design software, x-ray computed tomography and 3-dimensional printing can be used to develop, optimize, and fabricate feed spacers for enhanced performance (Bucs et al., 2018). Yu et al. (2022) proposes an interesting design for an arch feed spacer that has a hole in the node. This design exhibited relatively higher flow velocity distribution and fewer dead zones, resulting in less accumulation of foulants.
As stated earlier, fouling is one of the challenges with the use of spiral wound membranes. Developing membranes with high resistance to fouling will extend the service life of the modules and contribute to long term cost reduction for operating these systems. Y. Chen et al. (2022) made SWMs from membranes whose surface they had modified with tethered poly (acylic acid) using an atmospheric pressure plasma-induced graft polymerization process. The modules recorded lower fouling propensity and total permeability recovery after water cleaning as compared to a Dow SW30 module.
The United Nations (UN) has several agencies, programmes and funds that champion the course of water and sanitation issues. Hence, the UN established UN-Water, as a tool that serves to coordinate the efforts of its members and partners that work on water and sanitation related issues. UN-water is used by the UN to basically have a single front in terms of the deliverables on water and sanitation. UN-water publishes a yearly report known as the United Nations World Water Development Report (WWDR) and launches it on world water day. According to the 2021 report, the world is not on track to make goal 6 of the sustainable development goals (SDGs) a reality (UN Water, 2021). The SDG 6 mainly focuses on providing safe water and sanitation for all. This calls for urgent and conscientious efforts to work towards attaining the SDG 6. A search of the google scholar database using the keywords “desalination” and “groundwater”, and “desalination” and “seawater” indicated that there have been more efforts on treating seawater as compared to groundwater for over 2 decades as shown in Figure 13. Treating more groundwater to make it safely available to end-users could go a long way to add up to the efforts being made to attain the SDG 6. The 2022 WWDR highlights this clearly by placing emphasis on the use of groundwater (UN Water, 2022).
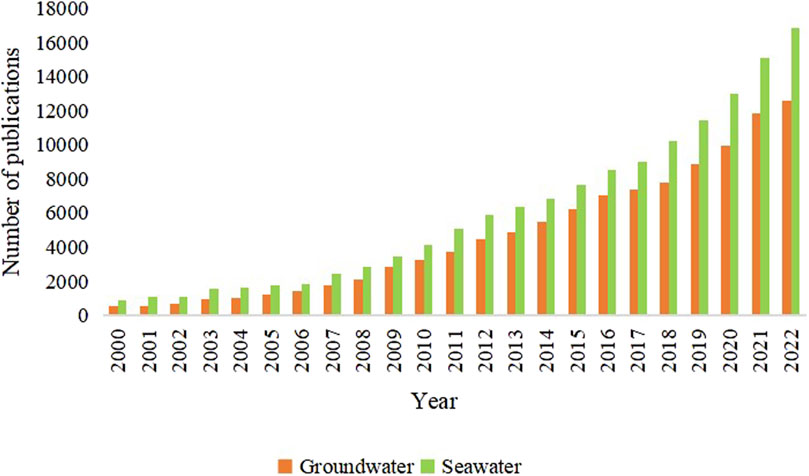
Figure 13. Annual scientific publications on the desalination of groundwater and seawater as contained in the google scholar database.
7 Conclusion
Pressure-driven membrane processes such as nanofiltration and reverse osmosis are an advanced water treatment technology that have the potential to be used to provide safe water for domestic purposes and for industrial applications from groundwater sources as well as from seawater. As noted early on, a lot of efforts have been made in treating seawater with spiral wound membrane modules as compared to groundwater. However, there are several nations that are landlocked and do not readily have access to seawater. Aside from this, nations that are not landlocked have regions where seawater desalination would not be commercially viable. Luckily, groundwater has been identified as the largest source of readily available fresh water source that can be relied upon in the wake of water scarcity and looming water crises that the world is faced with. Hence it would be beneficial to invest more efforts in treating groundwater with this advanced technology as contaminants such as heavy metals, minerals and organic micropollutants (components of fertilizers, pesticides, cosmetics, drugs, etc.), amongst others can be removed from the water. Furthermore, due to the low osmotic pressures of groundwater, higher recovery rates (60%–90%) are attainable at much lower operating pressures (below 10 bars) as compared to seawater. Similarly, higher permeate flow rates are attainable, although this is at the expense of high rejection.
Although the spiral wound membrane module, a key part of the pressure-driven membrane plant has evolved and become more advanced over the years from when it was first developed in the 1960s, there are still knowledge gaps that need to be filled to obtain optimum performance. These include but not limited to improving pre-treatment processes, developing membranes with high selectivity and permeability with less susceptibility to fouling, integration of renewable and sustainable energy sources into the plant set-up, improving energy storage and recovery systems, and developing innovative and environmentally friendly waste handling and disposal processes. This paper discussed the applications of spiral wound membranes to remove contaminants from groundwater for domestic and industrial applications. Consequently, application modes, process optimization, challenges and opportunities were presented. The review indicates that pressure-driven membrane treatment is currently the state-of-the-art method for providing water of suitable quality from groundwater, but more still needs to be done to improve module and plant performance.
Author contributions
RT: Conceptualization, Writing–original draft. PB: Supervision, Writing–review and editing. FA: Supervision, Writing–review and editing. EN: Writing–review and editing. SO-K: Funding acquisition, Supervision, Writing–review and editing.
Funding
The author(s) declare that financial support was received for the research, authorship, and/or publication of this article. This work was funded by Institut de Recherche pour le Développement (IRD) and Agence Française de Développement (AFD), through the ACE Partner project. Assistance was received from the African Membrane Society to cover the publication cost of this article.
Conflict of interest
The authors declare that the research was conducted in the absence of any commercial or financial relationships that could be construed as a potential conflict of interest.
Publisher’s note
All claims expressed in this article are solely those of the authors and do not necessarily represent those of their affiliated organizations, or those of the publisher, the editors and the reviewers. Any product that may be evaluated in this article, or claim that may be made by its manufacturer, is not guaranteed or endorsed by the publisher.
References
Abaie, E., Xu, L., and Shen, Y. (2021). Bioinspired and biomimetic membranes for water purification and chemical separation: a review. Front. Environ. Sci. Eng. 15 (6), 124. doi:10.1007/s11783-021-1412-8
Abbasnia, A., Yousefi, N., Mahvi, A. H., Nabizadeh, R., Radfard, M., Yousefi, M., et al. (2019). Evaluation of groundwater quality using water quality index and its suitability for assessing water for drinking and irrigation purposes: case study of Sistan and Baluchistan province (Iran). Hum. Ecol. Risk Assess. Int. J. 25 (4), 988–1005. doi:10.1080/10807039.2018.1458596
Ahdab, Y. D., and Lienhard, J. H. (2021). Desalination of brackish groundwater to improve water quality and water supply. Glob. Groundw. 2021, 559–575. doi:10.1016/B978-0-12-818172-0.00041-4
Ahmed, K., and Abdelamir, S. H. (2022). Development of biological processes for the removal of iron from water intended for the production of drinking water—case of ferruginous waters in Algeria. Int. J. Hydrology Sci. Technol. 13 (4), 457–465. doi:10.1504/IJHST.2022.123165
Al Aani, S., Bonny, T., Hasan, S. W., and Hilal, N. (2019). Can machine language and artificial intelligence revolutionize process automation for water treatment and desalination? Desalination 458, 84–96. doi:10.1016/j.desal.2019.02.005
Alhadidi, A., Kemperman, A. J. B., Schurer, R., Schippers, J. C., Wessling, M., and van der Meer, W. G. J. (2012). Using SDI, SDI+ and MFI to evaluate fouling in a UF/RO desalination pilot plant. Desalination 285, 153–162. doi:10.1016/j.desal.2011.09.049
Alnumani, A., Matin, A., Farooque, M., Green, T., Falath, W., Aljundi, I., et al. (2024). Osmotic-induced cleaning is effective in long-term fouling mitigation of reverse osmosis elements at pilot plant level. Desalination 578, 117336. doi:10.1016/j.desal.2024.117336
Al-Obaidi, M. A., Alsarayreh, A. A., Al-Hroub, A. M., Alsadaie, S., and Mujtaba, I. M. (2018). Performance analysis of a medium-sized industrial reverse osmosis brackish water desalination plant. Desalination 443, 272–284. doi:10.1016/j.desal.2018.06.010
Alsarayreh, A. A., Al-Obaidi, M. A., Al-Hroub, A. M., Patel, R., and Mujtaba, I. M. (2020). Evaluation and minimisation of energy consumption in a medium-scale reverse osmosis brackish water desalination plant. J. Clean. Prod. 248, 119220. doi:10.1016/j.jclepro.2019.119220
Ameen, F., Dawoud, T., and Arif, I. A. (2022). Purification treatment of polluted groundwater using wheat straw inoculated with microalgae. Algal Res. 62, 102639. doi:10.1016/j.algal.2022.102639
Anis, S. F., Hashaikeh, R., and Hilal, N. (2019). Reverse osmosis pretreatment technologies and future trends: a comprehensive review. Desalination 452, 159–195. doi:10.1016/j.desal.2018.11.006
Balster, J. (2015). “Capillary Membrane Module,” in Encyclopedia of Membranes. Editors E. Drioli, and L. Giorno (Springer), 1–2. doi:10.1007/978-3-642-40872-4_1581-1
Banerjee, A., Rakshit, N., and Ray, S. (2019). Structural dynamic models. Encycl. Ecol. 2019, 206–212. doi:10.1016/B978-0-12-409548-9.11202-3
Bouhadjar, S. I., Kopp, H., Britsch, P., Deowan, S. A., Hoinkis, J., and Bundschuh, J. (2019). Solar powered nanofiltration for drinking water production from fluoride-containing groundwater – a pilot study towards developing a sustainable and low-cost treatment plant. J. Environ. Manag. 231, 1263–1269. doi:10.1016/j.jenvman.2018.07.067
Boussouga, Y.-A., Richards, B. S., and Schäfer, A. I. (2021). Renewable energy powered membrane technology: system resilience under solar irradiance fluctuations during the treatment of fluoride-rich natural waters by different nanofiltration/reverse osmosis membranes. J. Membr. Sci. 617, 118452. doi:10.1016/j.memsci.2020.118452
Bucs, S., Farhat, N., Kruithof, J. C., Picioreanu, C., van Loosdrecht, M. C. M., and Vrouwenvelder, J. S. (2018). Review on strategies for biofouling mitigation in spiral wound membrane systems. Desalination 434, 189–197. doi:10.1016/j.desal.2018.01.023
Burkart, M. R., and Stoner, J. D. (2001). “Chapter 6—nitrogen in groundwater associated with agricultural systems,” in Nitrogen in the environment: sources, problems and management. Editors R. F. Follett, and J. L. Hatfield (Elsevier Science), 123–145. doi:10.1016/B978-044450486-9/50008-X
Buyanjargal, A., Kang, J., Sleep, B. E., and Jeen, S.-W. (2021). Sequential treatment of nitrate and phosphate in groundwater using a permeable reactive barrier system. J. Environ. Manag. 300, 113699. doi:10.1016/j.jenvman.2021.113699
Cadotte, J. E., Petersen, R. J., Larson, R. E., and Erickson, E. E. (1980). A new thin-film composite seawater reverse osmosis membrane. Desalination 32, 25–31. doi:10.1016/S0011-9164(00)86003-8
Chen, A. S. C., Wang, L., Sorg, T. J., and Lytle, D. A. (2020a). Removing arsenic and co-occurring contaminants from drinking water by full-scale ion exchange and point-of-use/point-of-entry reverse osmosis systems. Water Res. 172, 115455. doi:10.1016/j.watres.2019.115455
Chen, B.-Z., Ju, X., Liu, N., Chu, C.-H., Lu, J.-P., Wang, C., et al. (2020b). Pilot-scale fabrication of nanofiltration membranes and spiral-wound modules. Chem. Eng. Res. Des. 160, 395–404. doi:10.1016/j.cherd.2020.06.011
Chen, Y., Kim, S., Kim, Y., Walker, J. S., Wolfe, T., Coleman, K., et al. (2022). Scale up of polyamide reverse osmosis membranes surface modification with tethered poly(acrylic acid) for fabrication of low fouling spiral-wound elements. Desalination 536, 115762. doi:10.1016/j.desal.2022.115762
Chew, Y. T., and Yong, W. F. (2021). Recent advances of thin film nanocomposite membranes: effects of shape/structure of nanomaterials and interfacial polymerization methods. Chem. Eng. Res. Des. 172, 135–158. doi:10.1016/j.cherd.2021.06.003
Cipollina, A., Tzen, E., Subiela, V., Papapetrou, M., Koschikowski, J., Schwantes, R., et al. (2015). Renewable energy desalination: performance analysis and operating data of existing RES desalination plants. Desalination Water Treat. 55 (11), 3120–3140. doi:10.1080/19443994.2014.959734
Di Vincenzo, M., Tiraferri, A., Musteata, V.-E., Chisca, S., Deleanu, M., Ricceri, F., et al. (2021a). Tunable membranes incorporating artificial water channels for high-performance brackish/low-salinity water reverse osmosis desalination. Proc. Natl. Acad. Sci. 118 (37), e2022200118. doi:10.1073/pnas.2022200118
Di Vincenzo, M., Tiraferri, A., Musteata, V.-E., Chisca, S., Sougrat, R., Huang, L.-B., et al. (2021b). Biomimetic artificial water channel membranes for enhanced desalination. Nat. Nanotechnol. 16 (2), 190–196. doi:10.1038/s41565-020-00796-x
Du, J. R., Zhang, X., Feng, X., Wu, Y., Cheng, F., and Ali, M. E. A. (2020). Desalination of high salinity brackish water by an NF-RO hybrid system. Desalination 491, 114445. doi:10.1016/j.desal.2020.114445
Efraty, A. (2009). Apparatus for continuous closed circuit desalination under variable pressure with a single container. Patent US 7,628,921 B2.
Elmenshawy, M. R., Shalaby, S. M., Elshinnawy, A. I., and Gado, T. A. (2023). Groundwater desalination for agricultural purposes using reverse osmosis and nanofiltration technologies: case study wadi El-natrun, Egypt. Process Saf. Environ. Prot. 180, 669–685. doi:10.1016/j.psep.2023.10.033
Fantaye, S. M., Wolde, B. B., Haile, A. T., and Taye, M. T. (2023). Estimation of shallow groundwater abstraction for irrigation and its impact on groundwater availability in the Lake Tana sub-basin, Ethiopia. J. Hydrology Regional Stud. 46, 101365. doi:10.1016/j.ejrh.2023.101365
Fellaou, S., Ruiz-Garcia, A., and Gourich, B. (2021). Enhanced exergy analysis of a full-scale brackish water reverse osmosis desalination plant. Desalination 506, 114999. doi:10.1016/j.desal.2021.114999
Freire-Gormaly, M., and Bilton, A. M. (2018). Experimental quantification of the effect of intermittent operation on membrane performance of solar powered reverse osmosis desalination systems. Desalination 435, 188–197. doi:10.1016/j.desal.2017.09.013
Futterlieb, M., ElSherbiny, I. M. A., Tuczinski, M., Lipnizki, J., and Panglisch, S. (2021). Limits of high recovery inland desalination: closed-circuit reverse osmosis – a viable option? Chem. Ing. Tech. 93 (9), 1359–1368. doi:10.1002/cite.202100042
Fuwad, A., Ryu, H., Malmstadt, N., Kim, S. M., and Jeon, T.-J. (2019). Biomimetic membranes as potential tools for water purification: preceding and future avenues. Desalination 458, 97–115. doi:10.1016/j.desal.2019.02.003
García-Pacheco, R., Landaburu-Aguirre, J., Lejarazu-Larrañaga, A., Rodríguez-Sáez, L., Molina, S., Ransome, T., et al. (2019). Free chlorine exposure dose (ppm·h) and its impact on RO membranes ageing and recycling potential. Desalination 457, 133–143. doi:10.1016/j.desal.2019.01.030
Geise, G. M., Lee, H.-S., Miller, D. J., Freeman, B. D., McGrath, J. E., and Paul, D. R. (2010). Water purification by membranes: the role of polymer science. J. Polym. Sci. Part B Polym. Phys. 48 (15), 1685–1718. doi:10.1002/polb.22037
Ghazi, Z. M., Rizvi, S. W. F., Shahid, W. M., Abdulhameed, A. M., Saleem, H., and Zaidi, S. J. (2022). An overview of water desalination systems integrated with renewable energy sources. Desalination 542, 116063. doi:10.1016/j.desal.2022.116063
Greenlee, L. F., Lawler, D. F., Freeman, B. D., Marrot, B., and Moulin, P. (2009). Reverse osmosis desalination: water sources, technology, and today’s challenges. Water Res. 43 (9), 2317–2348. doi:10.1016/j.watres.2009.03.010
Grossi, L. B., Neves, E. F. O., Lange, L. C., and Amaral, M. C. S. (2024). Sustainability in reverse osmosis membranes waste management: environmental and socioeconomic assessment. Desalination 575, 117338. doi:10.1016/j.desal.2024.117338
Gu, B., Adjiman, C. S., and Xu, X. Y. (2017). The effect of feed spacer geometry on membrane performance and concentration polarisation based on 3D CFD simulations. J. Membr. Sci. 527, 78–91. doi:10.1016/j.memsci.2016.12.058
Gu, H., Plumlee, M. H., Boyd, M., Hwang, M., and Lozier, J. C. (2021). Operational optimization of closed-circuit reverse osmosis (CCRO) pilot to recover concentrate at an advanced water purification facility for potable reuse. Desalination 518, 115300. doi:10.1016/j.desal.2021.115300
Haidari, A. H., Blankert, B., Timmer, H., Heijman, S. G. J., and van der Meer, W. G. J. (2017). PURO: a unique RO-design for brackish groundwater treatment. Desalination 403, 208–216. doi:10.1016/j.desal.2015.09.015
Haidari, A. H., Heijman, S. G. J., and van der Meer, W. G. J. (2018). Optimal design of spacers in reverse osmosis. Sep. Purif. Technol. 192, 441–456. doi:10.1016/j.seppur.2017.10.042
Haris, S., Qiu, X., Klammler, H., and Mohamed, M. M. A. (2020). The use of micro-nano bubbles in groundwater remediation: a comprehensive review. Groundw. Sustain. Dev. 11, 100463. doi:10.1016/j.gsd.2020.100463
Hassan, Y., Mousa, H. M., Abd El-sadek, M. S., and Abdel-Jaber, G. T. (2020). Membrane technology for groundwater purification: a review. SVU-International J. Eng. Sci. Appl. 1 (1), 8–21. doi:10.21608/svusrc.2020.51369.1002
Hauptfeld, E., Pelkmans, J., Huisman, T. T., Anocic, A., Snoek, B. L., von Meijenfeldt, F. A. B., et al. (2022). A metagenomic portrait of the microbial community responsible for two decades of bioremediation of poly-contaminated groundwater. Water Res. 221, 118767. doi:10.1016/j.watres.2022.118767
Hosseinipour, E., Park, K., Burlace, L., Naughton, T., and Davies, P. A. (2022). A free-piston batch reverse osmosis (RO) system for brackish water desalination: experimental study and model validation. Desalination 527, 115524. doi:10.1016/j.desal.2021.115524
Huang, L.-B., Di Vincenzo, M., Li, Y., and Barboiu, M. (2021). Artificial water channels: towards biomimetic membranes for desalination. Chem. – A Eur. J. 27 (7), 2224–2239. doi:10.1002/chem.202003470
Imbrogno, J., and Belfort, G. (2016). Membrane desalination: where are we, and what can we learn from fundamentals? Annu. Rev. Chem. Biomol. Eng. 7 (1), 29–64. doi:10.1146/annurev-chembioeng-061114-123202
Ionics Freshwater Ltd (2010). Desalination ionics freshwater limited. Avaliable at: http://www.ionicsfreshwater.com/desalination.
Ismail, A. F., Khulbe, K. C., and Matsuura, T. (2019a). “Chapter 5—RO membrane module,” in Reverse osmosis. Editors A. F. Ismail, K. C. Khulbe, and T. Matsuura (Elsevier), 117–141. doi:10.1016/B978-0-12-811468-1.00005-0
Ismail, A. F., Khulbe, K. C., and Matsuura, T. (2019b). “Chapter 8—RO membrane fouling,” in Reverse osmosis. Editors A. F. Ismail, K. C. Khulbe, and T. Matsuura (Elsevier), 189–220. doi:10.1016/B978-0-12-811468-1.00008-6
Jiang, W., Wei, Y., Gao, X., Gao, C., and Wang, Y. (2015). An innovative backwash cleaning technique for NF membrane in groundwater desalination: fouling reversibility and cleaning without chemical detergent. Desalination 359, 26–36. doi:10.1016/j.desal.2014.12.025
Jiang, W., Xu, X., Johnson, D., Lin, L., Wang, H., and Xu, P. (2022). Effectiveness and mechanisms of electromagnetic field on reverse osmosis membrane scaling control during brackish groundwater desalination. Sep. Purif. Technol. 280, 119823. doi:10.1016/j.seppur.2021.119823
Johnson, J., and Busch, M. (2010). Engineering aspects of reverse osmosis module design. Desalination Water Treat. 15 (1–3), 236–248. doi:10.5004/dwt.2010.1756
Johnson, J. E. (2013). “Design and construction of commercial spiral wound modules,” in Encyclopedia of membrane science and technology. Editors E. M. V. Hoek, and V. V. Tarabara (Wiley), 1–21. doi:10.1002/9781118522318.emst071
Karabelas, A. J., Koutsou, C. P., Kostoglou, M., and Sioutopoulos, D. C. (2018a). Analysis of specific energy consumption in reverse osmosis desalination processes. Desalination 431, 15–21. doi:10.1016/j.desal.2017.04.006
Karabelas, A. J., Koutsou, C. P., and Sioutopoulos, D. C. (2018b). Comprehensive performance assessment of spacers in spiral-wound membrane modules accounting for compressibility effects. J. Membr. Sci. 549, 602–615. doi:10.1016/j.memsci.2017.12.037
Karmal, I., Mohareb, S., El housse, M., Hafid, N., Hadfi, A., Belattar, M., et al. (2020). Structural and morphological characterization of scale deposits on the reverse osmosis membranes: case of brackish water demineralization station in Morocco. Groundw. Sustain. Dev. 11, 100483. doi:10.1016/j.gsd.2020.100483
Karunakaran, A., Chaturvedi, A., Ali, J., Singh, R., Agarwal, S., and Garg, M. C. (2022). Response surface methodology-based modeling and optimization of chromium removal using spiral-wound reverse-osmosis membrane setup. Int. J. Environ. Sci. Technol. 19 (7), 5999–6010. doi:10.1007/s13762-021-03422-y
Kerdi, S., Qamar, A., Vrouwenvelder, J. S., and Ghaffour, N. (2018). Fouling resilient perforated feed spacers for membrane filtration. Water Res. 140, 211–219. doi:10.1016/j.watres.2018.04.049
Khanzada, N. K., Khan, S. J., and Davies, P. A. (2017). Performance evaluation of reverse osmosis (RO) pre-treatment technologies for in-land brackish water treatment. Desalination 406, 44–50. doi:10.1016/j.desal.2016.06.030
Kim, J., Park, K., Yang, D. R., and Hong, S. (2019). A comprehensive review of energy consumption of seawater reverse osmosis desalination plants. Appl. Energy 254, 113652. doi:10.1016/j.apenergy.2019.113652
Kim, S.-J., Oh, B. S., Yu, H.-W., Kim, L. H., Kim, C.-M., Yang, E.-T., et al. (2015). Foulant characterization and distribution in spiral wound reverse osmosis membranes from different pressure vessels. Desalination 370, 44–52. doi:10.1016/j.desal.2015.05.013
Kostoglou, M., and Karabelas, A. J. (2009). On the fluid mechanics of spiral-wound membrane modules. Industrial Eng. Chem. Res. 48 (22), 10025–10036. doi:10.1021/ie901129j
Koutsou, C. P., Karabelas, A. J., and Goudoulas, T. B. (2013). Characteristics of permeate-side spacers of spiral wound membrane modules. Desalination 322, 131–136. doi:10.1016/j.desal.2013.05.015
Koutsou, C. P., Karabelas, A. J., and Kostoglou, M. (2015). Membrane desalination under constant water recovery – the effect of module design parameters on system performance. Sep. Purif. Technol. 147, 90–113. doi:10.1016/j.seppur.2015.04.012
Lawler, W., Alvarez-Gaitan, J., Leslie, G., and Le-Clech, P. (2015). Comparative life cycle assessment of end-of-life options for reverse osmosis membranes. Desalination 357, 45–54. doi:10.1016/j.desal.2014.10.013
Lee, C.-K., Park, C., Woo, Y. C., Choi, J.-S., and Kim, J.-O. (2020). A pilot study of spiral-wound air gap membrane distillation process and its energy efficiency analysis. Chemosphere 239, 124696. doi:10.1016/j.chemosphere.2019.124696
Lee, S. (2020). Performance comparison of spiral-wound and plate-and-frame forward osmosis membrane module. Membranes 10 (11), 318. doi:10.3390/membranes10110318
Lee, T., Rahardianto, A., and Cohen, Y. (2019). Multi-cycle operation of semi-batch reverse osmosis (SBRO) desalination. J. Membr. Sci. 588, 117090. doi:10.1016/j.memsci.2019.05.015
Lesimple, A., Ahmed, F. E., and Hilal, N. (2020). Remineralization of desalinated water: methods and environmental impact. Desalination 496, 114692. doi:10.1016/j.desal.2020.114692
Lew, B., Tarnapolski, O., Afgin, Y., Portal, Y., Ignat, T., Yudachev, V., et al. (2020). Exploratory ranking analysis of brackish groundwater desalination for sustainable agricultural production: a case study of the Arava Valley in Israel. J. Arid Environ. 174, 104078. doi:10.1016/j.jaridenv.2019.104078
Li, M. (2023). Cyclic simulation and energy assessment of closed-circuit RO (CCRO) of brackish water. Desalination 545, 116149. doi:10.1016/j.desal.2022.116149
Li, W.-L., Fu, P., Lin, W.-T., Zhang, Z.-L., Luo, X.-W., Yu, Y.-H., et al. (2024). High-performance thin-film composite (TFC) membranes with 2D nanomaterial interlayers: an overview. Results Eng. 21, 101932. doi:10.1016/j.rineng.2024.101932
Liang, S., Mora, R., Huang, Q., Casson, R., Wang, Y., Woodard, S., et al. (2022). Field demonstration of coupling ion-exchange resin with electrochemical oxidation for enhanced treatment of per- and polyfluoroalkyl substances (PFAS) in groundwater. Chem. Eng. J. Adv. 9, 100216. doi:10.1016/j.ceja.2021.100216
Lim, Y. J., Goh, K., Kurihara, M., and Wang, R. (2021). Seawater desalination by reverse osmosis: current development and future challenges in membrane fabrication – a review. J. Membr. Sci. 629, 119292. doi:10.1016/j.memsci.2021.119292
Lin, W., Zhang, Y., Li, D., Wang, X., and Huang, X. (2021). Roles and performance enhancement of feed spacer in spiral wound membrane modules for water treatment: a 20-year review on research evolvement. Water Res. 198, 117146. doi:10.1016/j.watres.2021.117146
Liu, C. J., McKay, G., Jiang, D., Tenorio, R., Cath, J. T., Amador, C., et al. (2021). Pilot-scale field demonstration of a hybrid nanofiltration and UV-sulfite treatment train for groundwater contaminated by per- and polyfluoroalkyl substances (PFASs). Water Res. 205, 117677. doi:10.1016/j.watres.2021.117677
Loeb, S., and Sourirajan, S. (1963). “Sea water demineralization by means of an osmotic membrane,” in Saline water conversion—II (Washington, DC, United States: American Chemical Society), 117–132. doi:10.1021/ba-1963-0038.ch009
Madduri, S., Sodaye, H. S., Debnath, A. K., Adak, A. K., and Prasad, T. L. (2023). Transformation of brackish water Reverse Osmosis membranes to nanofiltration & ultrafiltration membranes by NaOCl treatment: kinetic and characterization studies. J. Water Process Eng. 56, 104549. doi:10.1016/j.jwpe.2023.104549
Madhura, L., Kanchi, S., Sabela, M. I., Singh, S., Bisetty, K., and Inamuddin, R. (2018). Membrane technology for water purification. Environ. Chem. Lett. 16 (2), 343–365. doi:10.1007/s10311-017-0699-y
Mengesha, A., and Sahu, O. (2022). Sustainability of membrane separation technology on groundwater reverse osmosis process. Clean. Eng. Technol. 7, 100457. doi:10.1016/j.clet.2022.100457
Mokarizadeh, H., Moayedfard, S., Maleh, M. S., Mohamed, S. I. G. P., Nejati, S., and Esfahani, M. R. (2021). The role of support layer properties on the fabrication and performance of thin-film composite membranes: the significance of selective layer-support layer connectivity. Sep. Purif. Technol. 278, 119451. doi:10.1016/j.seppur.2021.119451
Moudjeber, D. E., Mahmoudi, H., Djennad, M., Sioutopoulos, D. C., Mitrouli, S. T., and Karabelas, A. J. (2014). Brackish water desalination in the Algerian Sahara—plant design considerations for optimal resource exploitation. Desalination Water Treat. 52 (22–24), 4040–4052. doi:10.1080/19443994.2013.875947
Nativ, P., Leifman, O., Lahav, O., and Epsztein, R. (2021). Desalinated brackish water with improved mineral composition using monovalent-selective nanofiltration followed by reverse osmosis. Desalination 520, 115364. doi:10.1016/j.desal.2021.115364
Nunes, S. P., and Peinemann, K.-V. (2006). Membrane technology: in the chemical industry. John Wiley & Sons.
Pal, P. (2017). “Chapter 5—Water Treatment by Membrane-Separation Technology,” in Industrial Water Treatment Process Technology. Editor P. Pal (Butterworth-Heinemann). Available at: https://www.sciencedirect.com/science/article/pii/B9780128103913000059.
Park, H.-G., Cho, S.-G., Kim, K.-J., and Kwon, Y.-N. (2016). Effect of feed spacer thickness on the fouling behavior in reverse osmosis process—a pilot scale study. Desalination 379, 155–163. doi:10.1016/j.desal.2015.11.011
Park, K., Burlace, L., Dhakal, N., Mudgal, A., Stewart, N. A., and Davies, P. A. (2020). Design, modelling and optimisation of a batch reverse osmosis (RO) desalination system using a free piston for brackish water treatment. Desalination 494, 114625. doi:10.1016/j.desal.2020.114625
Park, K., and Davies, P. A. (2021). A compact hybrid batch/semi-batch reverse osmosis (HBSRO) system for high-recovery, low-energy desalination. Desalination 504, 114976. doi:10.1016/j.desal.2021.114976
Poeter, E., Fan, Y., Cherry, J., Wood, W., and Mackay, D. (2020). Natural chemical constituents in groundwater. Avaliable at: https://books.gw-project.org/groundwater-in-our-water-cycle/chapter/natural-chemical-constituents-in-groundwater/.
Popova, A., Boivin, S., Shintani, T., and Fujioka, T. (2024). Development of high-integrity reverse osmosis membranes for enhanced removal of microorganisms. Desalination 572, 117155. doi:10.1016/j.desal.2023.117155
Qiu, T., and Davies, P. A. (2012a). Comparison of configurations for high-recovery inland desalination systems. Water 4 (3), 690–706. doi:10.3390/w4030690
Qiu, T. Y., and Davies, P. A. (2012b). Longitudinal dispersion in spiral wound RO modules and its effect on the performance of batch mode RO operations. Desalination 288, 1–7. doi:10.1016/j.desal.2011.11.054
Rackley, S. A. (2017). “8—Membrane separation systems,” in Carbon Capture and Storage. 2nd Edn, Editor S. A. Rackley (Butterworth-Heinemann). Available at: https://www.sciencedirect.com/science/article/pii/B9780128120415000088.
Rastogi, N. K., and Nayak, C. A. (2011). “21—Membranes for forward osmosis in industrial applications,” in Advanced Membrane Science and Technology for Sustainable Energy and Environmental Applications. Editors A. Basile, and S. P. Nunes (Woodhead Publishing), 680–717. doi:10.1533/9780857093790.5.680
Reid, C. E., and Breton, E. J. (1959). Water and ion flow across cellulosic membranes. J. Appl. Polym. Sci. 1 (2), 133–143. doi:10.1002/app.1959.070010202
Roehl, E. A., Ladner, D. A., Daamen, R. C., Cook, J. B., Safarik, J., Phipps, D. W., et al. (2018). Modeling fouling in a large RO system with artificial neural networks. J. Membr. Sci. 552, 95–106. doi:10.1016/j.memsci.2018.01.064
Ruiz-García, A., Melián-Martel, N., and Mena, V. (2018). Fouling characterization of RO membranes after 11years of operation in a brackish water desalination plant. Desalination 430, 180–185. doi:10.1016/j.desal.2017.12.046
Ruiz-García, A., and Nuez, I. (2020). Long-term intermittent operation of a full-scale BWRO desalination plant. Desalination 489, 114526. doi:10.1016/j.desal.2020.114526
Ruiz-García, A., and Nuez, I. (2021). A time-dependent model of pressure drop in reverse osmosis spiral wound membrane modules. IFAC-PapersOnLine 54 (3), 158–163. doi:10.1016/j.ifacol.2021.08.235
Ruiz-García, A., Nuez, I., Carrascosa-Chisvert, M. D., and Santana, J. J. (2020). Simulations of BWRO systems under different feedwater characteristics. Analysis of operation windows and optimal operating points. Desalination 491, 114582. doi:10.1016/j.desal.2020.114582
Sanna, A., Buchspies, B., Ernst, M., and Kaltschmitt, M. (2021). Decentralized brackish water reverse osmosis desalination plant based on PV and pumped storage—technical analysis. Desalination 516, 115232. doi:10.1016/j.desal.2021.115232
Schwinge, J., Neal, P. R., Wiley, D. E., Fletcher, D. F., and Fane, A. G. (2004). Spiral wound modules and spacers: review and analysis. J. Membr. Sci. 242 (1), 129–153. doi:10.1016/j.memsci.2003.09.031
Semiat, R. (2010). “Water purification: materials and technologies,” in Encyclopedia of materials: science and technology. Editors K. H. J. Buschow, R. W. Cahn, M. C. Flemings, B. Ilschner, E. J. Kramer, S. Mahajanet al. (Elsevier), 1–4. doi:10.1016/B978-008043152-9.02230-2
Shaaban, S., and Yahya, H. (2017). Detailed analysis of reverse osmosis systems in hot climate conditions. Desalination 423, 41–51. doi:10.1016/j.desal.2017.09.002
Singh, R. (2015). “Chapter 2—water and membrane treatment,” in Membrane technology and engineering for water purification. Editor R. Singh (Butterworth-Heinemann), 81–178. doi:10.1016/B978-0-444-63362-0.00002-1
Speight, J. G. (2020). “Remediation technologies,” in Natural water remediation (Elsevier), 263–303. doi:10.1016/B978-0-12-803810-9.00008-5
Srivastava, A., Singh, R., Rajput, V. D., Minkina, T., Agarwal, S., and Garg, M. C. (2022). A systematic approach towards optimization of brackish groundwater treatment using nanofiltration (NF) and reverse osmosis (RO) hybrid membrane filtration system. Chemosphere 303, 135230. doi:10.1016/j.chemosphere.2022.135230
Srivastava, A., K, A., Nair, A., Ram, S., Agarwal, S., Ali, J., et al. (2021). Response surface methodology and artificial neural network modelling for the performance evaluation of pilot-scale hybrid nanofiltration (NF) & reverse osmosis (RO) membrane system for the treatment of brackish ground water. J. Environ. Manag. 278, 111497. doi:10.1016/j.jenvman.2020.111497
Stillwell, A., and Webber, M. (2016). Predicting the specific energy consumption of reverse osmosis desalination. Water 8 (12), 601. doi:10.3390/w8120601
Sutariya, B., and Amaliar, G. (2023). Thermodynamic performance assessment of a community-scale brackish water reverse osmosis plant using exergy analysis. AQUA - Water Infrastructure, Ecosyst. Soc. 72 (10), 1867–1880. doi:10.2166/aqua.2023.105
Sutariya, B., Patel, K., and Karan, S. (2022). Effects of manual interventions in the winding process on the performance of spiral wound membrane module. DESALINATION WATER Treat. 251, 1–6. doi:10.5004/dwt.2021.27858
Tabi, R. N., Agyemang, F. O., Mensah-Darkwa, K., Arthur, E. K., Gikunoo, E., and Momade, F. (2021). Zeolite synthesis and its application in water defluorination. Mater. Chem. Phys. 261, 124229. doi:10.1016/j.matchemphys.2021.124229
Tian, C., Chen, J., Bai, Z., Wang, X., Dai, R., and Wang, Z. (2023). Recycling of end-of-life polymeric membranes for water treatment: closing the loop. J. Membr. Sci. Lett. 3 (2), 100063. doi:10.1016/j.memlet.2023.100063
Verma, A. K., Oren, Y., Gilron, J., and Ronen, Z. (2022). An investigation on the ion exchange membrane bioreactor with a spiral wound contactor for nitrate removal from contaminated groundwater. Desalination 542, 116080. doi:10.1016/j.desal.2022.116080
Wang, L. K., Chen, J. P., Hung, Y.-T., and Shammas, N. K. (2011). Membrane and desalination technologies. Totowa, New Jersey: Humana Press. doi:10.1007/978-1-59745-278-6
Wang, Q., Sun, J., Xue, W., Zhao, G., Ding, W., Zhang, K., et al. (2023). Effect of carbon nanotube nanochannel on the separation performance of thin-film nanocomposite (TFN) membranes. Desalination 546, 116216. doi:10.1016/j.desal.2022.116216
Wang, Z., Deshmukh, A., Du, Y., and Elimelech, M. (2020). Minimal and zero liquid discharge with reverse osmosis using low-salt-rejection membranes. Water Res. 170, 115317. doi:10.1016/j.watres.2019.115317
WHO (2017). Guidelines for drinking-water quality: fourth edition incorporating first addendum (4th ed + 1st add). Geneva: World Health Organization. Avaliable at: https://apps.who.int/iris/handle/10665/254637.
Wright, N. C., and Winter, A. G. (2019). Design of spiral-wound electrodialysis modules. Desalination 458, 54–65. doi:10.1016/j.desal.2019.01.010
Xie, Y., Yang, L., Chen, X., Zhao, H., Cao, G., Li, X., et al. (2022). The role of iron present in water environment in degradation of polyamide membranes by free chlorine. J. Membr. Sci. 651, 120458. doi:10.1016/j.memsci.2022.120458
Xue, Y. L., Zhang, R., Cao, B., and Li, P. (2021). “Chapter 20—tubular membranes and modules,” in Hollow fiber membranes. Editors T.-S. Chung, and Y. Feng (Elsevier), 431–448. doi:10.1016/B978-0-12-821876-1.00011-1
Yao, Y., Zhang, W., Du, Y., Li, M., Wang, L., and Zhang, X. (2019). Toward enhancing the chlorine resistance of reverse osmosis membranes: an effective strategy via an end-capping technology. Environ. Sci. Technol. 53 (3), 1296–1304. doi:10.1021/acs.est.8b06006
Yasukawa, M., Suzuki, T., and Higa, M. (2018). “Chapter 1 - salinity gradient processes: thermodynamics, applications, and future prospects,” in Membrane-based salinity gradient processes for water treatment and power generation. Editors S. Sarp, and N. Hilal (Elsevier), 3–56. doi:10.1016/B978-0-444-63961-5.00001-8
Yu, J., Chen, D., Wu, J. J., Wang, B., and Field, R. W. (2022). Arch-type feed spacer with wide passage node design for spiral-wound membrane filtration with reduced energy cost. Desalination 540, 115980. doi:10.1016/j.desal.2022.115980
Keywords: groundwater purification, polymeric membranes, brackish water reverse osmosis, nanofiltration, membrane processes
Citation: Tabi RN, Boakye P, Agyemang FO, Nxumalo EN and Oduro-Kwarteng S (2024) A review of spiral wound membrane modules and processes for groundwater treatment. Front. Membr. Sci. Technol. 3:1343651. doi: 10.3389/frmst.2024.1343651
Received: 24 November 2023; Accepted: 29 March 2024;
Published: 19 April 2024.
Edited by:
Abdoulaye Doucouré, Strategic Planning and Professional Development, United StatesReviewed by:
Bhaumik Sutariya, Council of Scientific and Industrial Research (CSIR), IndiaGlenn Lipscomb, University of Toledo, United States
Copyright © 2024 Tabi, Boakye, Agyemang, Nxumalo and Oduro-Kwarteng. This is an open-access article distributed under the terms of the Creative Commons Attribution License (CC BY). The use, distribution or reproduction in other forums is permitted, provided the original author(s) and the copyright owner(s) are credited and that the original publication in this journal is cited, in accordance with accepted academic practice. No use, distribution or reproduction is permitted which does not comply with these terms.
*Correspondence: Rita Namoe Tabi, bmFtb2UyMUBnbWFpbC5jb20=