- Faculty of Civil and Environmental Engineering, Technion—Israel Institute of Technology, Haifa, Israel
Over the last decades, nanofiltration (NF) membranes have been used to selectively remove certain solutes from water with recent interest targeting more challenging separations that require precise selectivity between solutes. In this perspective article, we aim to challenge (but not disprove) the prevalent notion that NF membranes are suitable for precise separations. We first provide and analyze selectivity data from the literature of three important separations including monovalent-divalent ion selectivity, separations involving organic molecules, and the more ambitious separations of ions with the same charge. We then introduce the terms rejection-based selectivity and transport-based selectivity to distinguish between the commonly “rough” separations pursued in NF (e.g., water softening) and the more visionary separations required for precise selectivity (e.g., transport of lithium through specific recognition sites), respectively. Using these terms, we discuss two major intrinsic limitations to achieve precise selectivity in NF systems; namely, the need for a solute-specific membrane that can transport simultaneously additional species (i.e., water and the complementary salt ion) and the detrimental effect of concentration polarization. We conclude with guidelines and principles to overcome these limitations.
1 Introduction
Since their first introduction in the late 1980s, nanofiltration (NF) membranes, a “looser” version of reverse osmosis (RO) membranes, have been proposed for applications involving the selective removal of specific solutes from water, such as water softening, sulfate removal, and concentration of organics in certain industries (Hilal et al., 2004; Van der Bruggen et al., 2008; Mohammad et al., 2015; Oatley-Radcliffe et al., 2017). More recently, increasing demands for fit-for-purpose water treatment and recovery of valuable elements from water have accelerated the race for the design of highly selective nanofiltration membranes for more challenging separations such as lithium and nutrient recovery and selective removal of contaminants from different waters (Epsztein et al., 2020; Duchanois et al., 2021; Zhao et al., 2021a; Zuo et al., 2021). While a certain (and important) progress has been reported, it is imperative to analyze the existing literature on solute-solute selectivity in NF, highlight current challenges and limitations, and introduce principles and pathways to overcome these limitations.
In this perspective, we aim to challenge (but not dismiss) the prevalent conception that nanofiltration membranes can achieve precise separations between solutes. We first review and analyze the general trends of achievable selectivities in NF for three main classes of important separations; namely, separation between ions of different charge, separations involving organics, and the highly challenging separation between ions of the same charge. We then discuss key challenges to achieve high solute-solute selectivity and introduce new guidelines to overcome these challenges. Our main takeaway message is that intrinsic limitations in the operation of current NF systems may require the design of a membrane with unique and different properties from those pursued so far.
2 Achievable solute-solute selectivity in nanofiltration membranes
To demonstrate the general picture of the achievable selectivity between different solutes (i.e., solute-solute selectivity) in NF, we extracted data from 43 references for three major classes of separations including separation of ions with different charge (Figure 1A), separations involving organics (Figure 1B), and the more challenging separation of ions with the same charge (Figures 1C,D). While selectivity in membrane processes can be expressed in different ways, the observed selectivity between two solutes, A and B, in NF is most commonly expressed using
where Ri is the observed rejection of solute i, which is defined as
where Cp and Cf are the concentrations of solute i in the permeate and feed solution, respectively. The real selectivity, which accounts for the increased concentration of accumulated solutes on the membrane surface, can be calculated from real rejections (instead of observed rejections) using the evaluated (Song and Elimelech, 1995) or measured (Sutzkover et al., 2000) solute concentrations on the membrane surface, Cm, instead of Cf in Eq. 2.
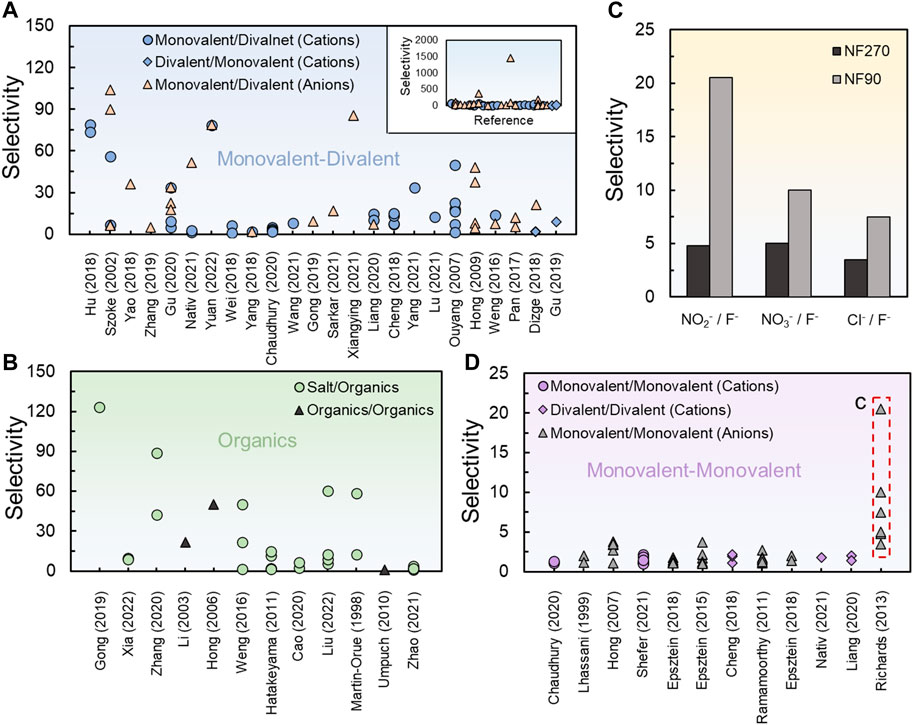
FIGURE 1. Solute-solute selectivity values reported in the literature in pressure-driven NF for (A) separation of ions with different charge (Szoke et al., 2003; Ouyang et al., 2008; Hong et al., 2009; Weng et al., 2016; Pan et al., 2017; Cheng et al., 2018; Dizge et al., 2018; Wei et al., 2018; Yang et al., 2018; Gong et al., 2019; Gu et al., 2019; Zhang et al., 2019; Chaudhury et al., 2020; Gu et al., 2020; Liang et al., 2020; Bian et al., 2021; Lu et al., 2021; Nativ et al., 2021; Sarkar et al., 2021; Wang et al., 2021; Yang et al., 2021; Hu et al., 2022; Li et al., 2022b; Yuan et al., 2022). The inset provides data for all the examined cases, while the main figure focuses on the common range of observed selectivities; (B) separations involving organic solutes (Martin-Orue et al., 1998; Li et al., 2003; Hong and Bruening, 2006; Umpuch et al., 2010; Hatakeyama et al., 2011; Weng et al., 2016; Gong et al., 2019; Cao et al., 2020; Zhang et al., 2020; Zhao et al., 2021b; Liu et al., 2022; Xia et al., 2022); (C,D) separation of ions with the same charge (Lhassani et al., 2001; Hong et al., 2007; Malaisamy et al., 2011; Richards et al., 2013; Epsztein et al., 2015; Epsztein et al., 2017; Epsztein et al., 2018; Chaudhury et al., 2020; Shefer et al., 2021). The selectivity values are based on Eq. 1. The data in (C) demonstrating the permeability-selectivity tradeoff for ion-ion separation was taken from (Richards et al., 2013).
The data in Figure 1 were achieved in pressure-driven NF experiments performed under different conditions (e.g., different concentration, pressure, pH, membrane type, single vs. mixed salt solutions, etc.) and therefore cannot be compared with each other; however, they are representative of the literature and hopefully catch the big picture of achievable selectivities in NF. In addition, many of the studies reviewed here did not account for concentration polarization; that is, the reported selectivity is based on observed rejection only, which hinders the understanding of the real membrane capabilities. We will discuss the underestimation of concentration polarization and its crucial role to obtain high solute-solute selectivity in NF in Section 3.
We note that the expression used in Eq. 1 to quantify the selectivity does not provide the complete picture regarding the quality of the separation from a practical point of view. More specifically, while this expression reflects the ratio of transmembrane passage between two solutes, it does not provide any information on the separation capabilities of the individual solute; that is, important evaluators of the separation of the individual solute such as rejection, permeability, or concentration in the permeate cannot be deduced from this term. For example, if the rejection of solute A is 99% and the rejection of solute B is 99.999%, the selectivity according to Eq. 1 is relatively high (1,000), but the actual separation is very poor, as both solutes will accumulate in the retentate stream with extremely little gain of pure solute A in the permeate stream. Nevertheless, the expression in Eq. 1 provides information on the ratio between the passage of two solutes, which is important to understand the ability of the membrane to distinguish between two solutes form a more fundamental perspective. We also note that the measurement of the selectivity becomes highly sensitive when rejections increase above 99% (e.g., a difference between 99% and 99.9% will lead to an order of magnitude difference in the selectivity), hindering the exact calculation of the selectivity.
2.1 Separation of ions with different charge
Separation between ions of different valence is perhaps the most popular solute-solute separation pursued by NF due to the relatively large size and/or charge difference of the ions to be separated (Figure 1A). Specifically, separation of divalent ions such as calcium, magnesium, and sulfate from the dominant monovalent ions in water systems (i.e., sodium and chloride) has been targeted in many studies and applications (Lu et al., 2022). More recently, lithium recovery from salt-lake brines, which contain high magnesium concentration, has been studied extensively (Sun et al., 2015; Li et al., 2019, Li Q. et al., 2022; Lyu et al., 2022). Efforts, therefore, mostly focus on maximizing the synergistic effect of size- and charge-based rejection by adjusting the membrane charge to be the same as the charge of the divalent ion (e.g., imparting a positive charge to the typically negative surface of polyamide membranes to enhance the removal of divalent cations) (Cheng et al., 2018). As will be discussed in later sections, this approach of increasing the rejection of the unwanted solute (i.e., rejection-based selectivity) may play an important role in the upper selectivity limit obtained for monovalent-divalent ion separation in NF.
Generally, most selectivity values for monovalent-divalent ion separation range from 0 to 100 (Figure 1A) with a few studies reporting higher values up to 2000 (Figure 1A, inset). This range of achievable selectivity can be very often satisfactory from a practical view, as the rejection of divalent ions can occasionally reach high values above 95%. For example, a sodium rejection of 10% and magnesium rejection of 95% results in a selectivity of 18, which in many cases can satisfy the softening requirements. While the reported monovalent-divalent ion selectivity demonstrates the potential of NF membranes to separate divalent ions from monovalent ions for various applications, a precise separation of these ions is still not within reach (e.g., high permeation of sodium with ultralow permeation of magnesium). For comparison, the ultra-selective potassium channel transports only one sodium ion per each 10,000 potassium ions traversing the channel, representing a selectivity value of 10,000 with high throughput of potassium (Doyle et al., 1998; Gouaux and MacKinnon, 2005). To put things in context, even if a membrane rejects 99.9% of one ion and 1% of the other ion, the selectivity will be 990. We will discuss the intrinsic limitations to reach ultrahigh selectivities in NF in Section 3.
2.2 Separations involving organic solutes
Due to their dense but relatively open structure that allows the permeation of certain solutes, NF membranes have been also widely applied for the selective removal of specific organic solutes over inorganic ions or other organic molecules. This type of selectivity, which mostly relies on size and charge differences between solutes, is needed in various environmental and industrial applications such as water and wastewater purification, recovery of pharmaceuticals and biomolecules, and concentration of products in the food industry (Mohammad et al., 2015). Our collected data from the literature of NF applied for separation of organics aims to provide the general picture of achievable selectivity in this area (Figure 1B).
Overall, good separation can be obtained with selectivity values similar to those achieved for monovalent-divalent ion separation. Such selectivity values are achievable for the selective separation of organic solutes from different organics or inorganic ions due to the relatively large size differences between different organic molecules and the larger size of organics compared to most inorganic ions, respectively. In addition, the pH dependency of the charge of many organic molecules (e.g., amino acids) opens opportunities to enhance size-based separations or induce the separation between similarly sized organics. However, as discussed for monovalent-divalent separation in Section 3.1, achieving ultrahigh selectivity values (i.e., four orders of magnitude and higher) for the separation of organics has not been reported. As we will discuss elaborately in Section 3, a partial reason for the limited selectivity achieved is the fact that the separation is pursued by tailoring the membrane to enhance the rejection of the unwanted solute (i.e., rejection-based selectivity) instead of designing a membrane that permits the transport of a specific solute (i.e., transport-based selectivity).
2.3 Separation of ions with the same charge
With the increased demand for resource recovery, fit-for-purpose water treatment, and other related applications, the separation between ions of the same charge, especially monovalent ions, has become a main research avenue in recent years (Epsztein et al., 2020; Zhao et al., 2021a; Shefer et al., 2022a; Shefer et al., 2022b; Pavluchkov et al., 2022). Since similarly charged ions often possess a similar hydrated size (Nightingale, 1959), separation mechanisms beyond simple size and charge exclusion are required to leverage the selectivity. Such mechanisms must involve more gentle, non-electrostatic interactions as demonstrated by many ion-selective biological channels (Gouaux and MacKinnon, 2005; Faucher et al., 2019; Epsztein et al., 2020). To establish these interactions in membrane separation, fabrication approaches with atomic precision are required. Unfortunately, current methods to fabricate polymeric NF membranes cannot reach such precision scales, where even the roughness of the membrane surface exceeds tens of nanometers (Freger, 2003). Nevertheless, due to the non-steric and non-electrostatic selectivity mechanisms that exist naturally under any condition, even state-of-the-art and other custom-made polymeric NF membranes exhibit a certain selectivity between ions of the same charge (Figures 1C,D).
As expected, the selectivity values obtained for the separation of similarly sized and charged ions (Figure 1D) are lower than those obtained for monovalent-divalent ion separation (Figure 1A) and fractionation of organics (Figure 1B), with most values ranging from 0 to 5. Since the current technology to improve the selectivity between ions of the same charge in polymeric NF membranes is limited, most of the data in Figure 1D represents experiments on commercial NF membranes and only a few studies that attempted to leverage the separation by fabricating NF membranes using the layer-by-layer assembly of polyelectrolytes. We note that over the last decade there has been a progress in the fabrication of channels and membranes that incorporate specific binding groups to enhance or hinder the transport of specific ions, which resulted in increased ion-ion selectivity (Sheng et al., 2014; Warnock et al., 2021; DuChanois et al., 2022). However, most of these studies did not test the selectivity in a pressure-driven NF and did not provide the obtained selectivity (or related parameters to measure this selectivity) as defined in this perspective; therefore, data from these studies were not included in Figure 1D. Increasing the selectivity between ions of the same charge for practical separations may be possible with further progress and technological developments in membrane fabrication, but will be still bounded by some intrinsic limitations governing the separation in NF systems as discussed in Section 3.
Interestingly, our analysis finds the selectivity between monovalent ions to be higher for denser NF membranes (Figure 1C) (Richards et al., 2013), most likely due to the same permeability-selectivity tradeoff that governs water-salt separation (Park et al., 2017); that is, a less permeable membrane reduces more the permeability of the larger species, increasing the selectivity between the two species. This finding is, to some extent, counterintuitive to the common notion that NF membranes can achieve higher solute-solute selectivity than RO membranes. However, from a practical view, the lower selectivity of NF is commonly compensated by the higher throughput of permeate that is rich with a desired solute compared to the almost solute-free (and therefore useless) permeate in RO.
3 Key challenges and solutions towards improved solute-solute selectivity
We showed in the previous section that most achievable solute-solute selectivity values reported in the literature of NF can reach the lower range of two orders of magnitude (100–150) with only a few exceptional cases that demonstrate selectivity value of three orders of magnitude (>1,000). These relatively low selectivity values compared to the four orders of magnitude selectivity observed for ion-selective biological channels mostly stem from the low specificity of polymeric NF membranes towards a certain solute. To better discuss the lack of specificity in current NF membranes, we introduce the terms rejection-based selectivity and transport-based selectivity, which represent two main approaches to achieve high selectivity. In most attempts reported in the literature to improve the selectivity of NF membranes, the rejection-based selectivity approach was used; that is, the rejection of the unwanted solute (e.g., magnesium in water softening) is increased with minimal or no increase in the rejection of the wanted solute (e.g., sodium in water softening) (Cheng et al., 2018). This approach suffers from two main drawbacks. First, rejection is based on repulsive forces between the membrane and permeating solutes and therefore it is more challenging to be tuned to act on a specific solute. For example, when magnesium removal is pursued by applying higher positive membrane charge, the rejection of other multivalent cations will also increase, so that the recovery of pure magnesium cannot be achieved. Second, we assert that a higher rejection of solute A can be achieved if efforts will be directed to tune the membrane to specifically transport solute B (i.e., transport-based selectivity, see below) instead of tuning the rejection of solute A.
Using transport-based selectivity, which is manifested in some prominent cases of ion-selective biological channels (Gouaux and MacKinnon, 2005), ultrahigh selectivity values can be achieved through high specificity of the membrane towards the desired solute to be transported. Applying this approach, a permeate containing exclusively the desired solute can be obtained with close to zero passage of unwanted solutes, overcoming the above-mentioned problems associated with rejection-based selectivity. Principles to achieve such specificity, which are based on decreasing the energy barrier for the target solute via compensatory interactions (i.e., interactions that thermodynamically stabilize the permeating solute within the pore), were discussed in detail elsewhere (Epsztein et al., 2020). While we believe that it is imperative to apply transport-based selectivity in NF membranes to reach ultrahigh selectivity values, two main limitations intrinsic to NF systems impose a significant challenge for the utilization of such approach.
3.1 A solute-specific membrane that transports simultaneously other species
As opposed to ion-exchange membranes or biological ion channels, which ideally permit the exclusive transport of anions or cations without water, NF membranes inherently must transport simultaneously multiple species including anions, cations, and (mostly) water. This mixture of species entering the membrane hinders a membrane design for transport-based selectivity that permits the transport of a specific solute via compensatory interactions. That is, how can a membrane be highly specific to lithium, for example, if it must also transport chloride and water? To overcome this inherent limitation, we propose a few principles for the design of an ideal ultra-selective NF membrane (Figure 2).
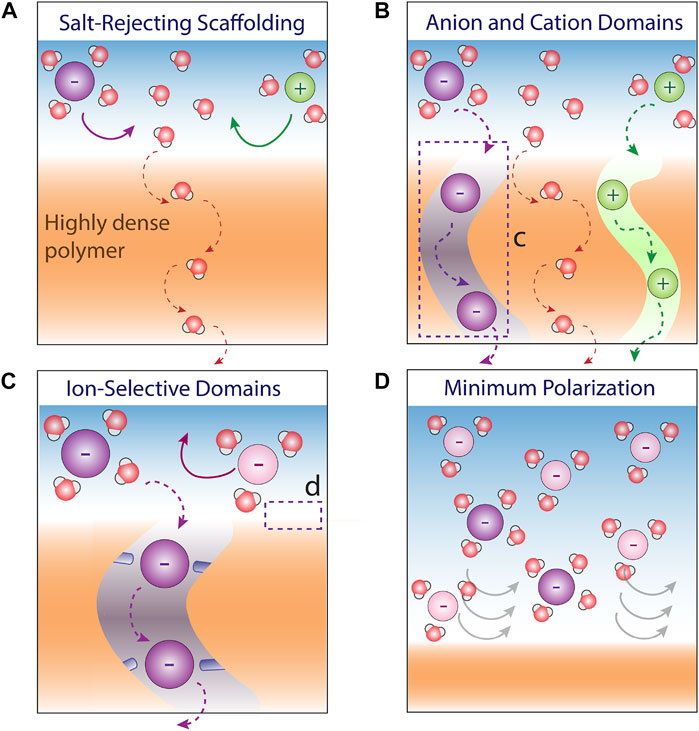
FIGURE 2. Guidelines and principles for the design of an ideal ultra-selective NF membrane. (A) To avoid permeation of unwanted solutes, the membrane backbone should be highly impermeable to salts; (B) To separate the treatment of anions and cations, anion and cation domains should be designed alternately; (C) Within each domain, precise selectivity can be applied using principles form ion-selective biological channels; and (D) Concentration polarization should be minimized to decrease the driving force of the less permeable species.
First, to have a membrane that is selective to the transport of a specific solute (and water), it will be essential to start with a scaffolding exhibiting ultrahigh salt rejection to ensure that only water and no solute can pass through (Figure 2A). Such scaffolding can be, in fact, a highly dense reverse osmosis membrane with ultrahigh water-salt selectivity (Barboiu, 2012). Next, to reduce the problem of separation into separation of ions with the same charge (e.g., separating lithium from sodium without the interference of chloride), alternating anion and cation domains (i.e., channels that transport exclusively anions or cations) must be designed within the scaffolding (Figure 2B). Such a charge-patterned mosaic membrane with alternating anion and cation domains was recently proposed for a different purpose using inkjet printing (Gao et al., 2016). These domains can be then decorated with selective recognition sites based on previously reported principles that provide the compensatory interactions for the target ion to discriminate between ions of the same charge (Figure 2C).
3.2 Counteracting driving force due to concentration polarization
According to the solution-diffusion model (Wijmans and Baker, 1995), the actual chemical potential gradient that moves solutes from one side of the membrane to the other is the concentration gradient across the membrane. This feature means that the selectivity between two solutes is interfered by the increasing driving force (i.e., concentration gradient) applied on the less permeable species along the filtration module. Such interference becomes even more prominent due to concentration polarization, which is manifested more severely in NF membranes with relatively high flux and especially in the case of highly rejected and heavy solutes with low diffusivity in solution due to their higher tendency to accumulate on the membrane surface (Déon et al., 2013). In the context of transport-based selectivity discussed here that can ideally prevent the passage of unwanted solutes, an unregulated increase in the surface concentration of the rejected solutes can substantially hamper the achievement of ultrahigh selectivity, which requires ultrahigh rejection (>99.9%) of these solutes. It is very likely, for example, that one of the factors that limit the selective rejection of divalent cations over sodium cation in softening processes is the high concentration polarization of the relatively heavy and less mobile divalent cations. Therefore, the premise for achieving ultrahigh selectivity for any kind of separation in pressure-driven NF is that the flow conditions are optimized to minimize concentration polarization effects (Figure 2D).
4 Outlook for precise separations in nanofiltration
Nanofiltration membranes, by their very nature, are designed to transport solvents (e.g., water) rather than solutes. That is, the major species to be transported through the membrane is the solvent. This property of NF membranes imposes a prominent challenge to make these membranes highly selective to a specific solute using the selectivity principles learned from nature (e.g., incorporating intrapore binding sites that stabilize the target solute in the membrane). This challenge becomes even higher in the specific case of ions, where the transport of a target ion must be accompanied by the complementary salt ion to maintain electroneutrality. On top of this inherent limitation, pressure-driven NF systems are characterized by concentration polarization that counteracts solute-solute selectivity by providing additional driving force to the rejected species. Our ongoing investigation shows how polarization effects can not only decrease the selectivity between solutes, but even reverse it. Depending on the specific needs, future membrane design should account for these limitations in different levels. For non-precise separations resulting from rejection-based selectivity (i.e., higher rejection of divalent ions compared to monovalent ions), minimizing concentration polarization should be addressed at high priority. For more advanced and precise separations that make use of transport-based selectivity (i.e., selective transport of a specific solute through recognition sites in the membrane), a more holistic approach should be taken to achieve ultrahigh selectivities that exceed by at least one order of magnitude the existing selectivity data. This approach requires the fabrication of a new class of NF membranes comprising multiple pathways for each type of species in the solution.
Data availability statement
The original contributions presented in the study are included in the article/Supplementary Material, further inquiries can be directed to the corresponding author.
Author contributions
RE—Conceptualization, Formal analysis, Resources, Funding acquisition, Writing—Review and Editing, Visualization.
Funding
This research was supported by the ISRAEL SCIENCE FOUNDATION (Grant No. 1269/21) and the “German-Israeli Foundation for Scientific research and Development”.
Conflict of interest
The author declares that the research was conducted in the absence of any commercial or financial relationships that could be construed as a potential conflict of interest.
Publisher’s note
All claims expressed in this article are solely those of the authors and do not necessarily represent those of their affiliated organizations, or those of the publisher, the editors and the reviewers. Any product that may be evaluated in this article, or claim that may be made by its manufacturer, is not guaranteed or endorsed by the publisher.
References
Barboiu, M. (2012). Artificial water channels. Angew. Chem. Int. Ed. Engl. 51, 11674–11676. doi:10.1002/anie.201205819
Bian, X., Zhang, Y., Gong, L., Zhu, Y., and Jin, J. (2021). Calcium ion coordinated polyamide nanofiltration membrane for ultrahigh perm-selectivity desalination. Chem. Res. Chin. Univ. 37, 1101–1109. doi:10.1007/s40242-021-1270-8
Cao, X. L., Yan, Y. N., Zhou, F. Y., and Sun, S. P. (2020). Tailoring nanofiltration membranes for effective removing dye intermediates in complex dye-wastewater. J. Memb. Sci. 595, 117476. doi:10.1016/j.memsci.2019.117476
Chaudhury, S., Wormser, E., Harari, Y., Edri, E., and Nir, O. (2020). Tuning the ion-selectivity of thin-film composite nanofiltration membranes by molecular layer deposition of alucone. ACS Appl. Mater. Interfaces 12, 53356–53364. doi:10.1021/acsami.0c16569
Cheng, W., Liu, C., Tong, T., Epsztein, R., Sun, M., Verduzco, R., et al. (2018). Selective removal of divalent cations by polyelectrolyte multilayer nanofiltration membrane: Role of polyelectrolyte charge, ion size, and ionic strength. J. Memb. Sci. 559, 98–106. doi:10.1016/j.memsci.2018.04.052
Déon, S., Dutournié, P., Fievet, P., Limousy, L., and Bourseau, P. (2013). Concentration polarization phenomenon during the nanofiltration of multi-ionic solutions: Influence of the filtrated solution and operating conditions. Water Res. 47, 2260–2272. doi:10.1016/j.watres.2013.01.044
Dizge, N., Epsztein, R., Cheng, W., Porter, C. J., and Elimelech, M. (2018). Biocatalytic and salt selective multilayer polyelectrolyte nanofiltration membrane. J. Memb. Sci. 549, 357–365. doi:10.1016/j.memsci.2017.12.026
Doyle, D. A., Cabral, J. M., Pfuetzner, R. A., Kuo, A., Gulbis, J. M., Cohen, S. L., et al. (1998). The structure of the potassium channel: Molecular basis of K+ conduction and selectivity. Science 280, 69–77. doi:10.1126/science.280.5360.69
Duchanois, R. M., Porter, C. J., Violet, C., Verduzco, R., and Elimelech, M. (2021). Membrane materials for selective ion separations at the water – energy nexus. Adv. Mater. 33, e2101312–e2101318. doi:10.1002/adma.202101312
DuChanois, R. M., Heiranian, M., Yang, J., Porter, C. J., Li, Q., Zhang, X., et al. (2022). Designing polymeric membranes with coordination chemistry for high-precision ion separations. Sci. Adv. 8, eabm9436–10. doi:10.1126/sciadv.abm9436
Epsztein, R., Nir, O., Lahav, O., and Green, M. (2015). Selective nitrate removal from groundwater using a hybrid nanofiltration–reverse osmosis filtration scheme. Chem. Eng. J. 279, 372–378. doi:10.1016/j.cej.2015.05.010
Epsztein, R., Cheng, W., Shaulsky, E., Dizge, N., and Elimelech, M. (2017). Elucidating the mechanisms underlying the difference between chloride and nitrate rejection in nanofiltration. J. Memb. Sci. 548, 694–701. doi:10.1016/j.memsci.2017.10.049
Epsztein, R., Shaulsky, E., Dizge, N., Warsinger, D. M., and Elimelech, M. (2018). Role of ionic charge density in Donnan exclusion of monovalent anions by nanofiltration. Environ. Sci. Technol. 52, 4108–4116. doi:10.1021/acs.est.7b06400
Epsztein, R., DuChanois, R. M., Ritt, C. L., Noy, A., and Elimelech, M. (2020). Towards single-species selectivity of membranes with subnanometre pores. Nat. Nanotechnol. 15, 426–436. doi:10.1038/s41565-020-0713-6
Faucher, S., Aluru, N., Bazant, M. Z., Blankschtein, D., Brozena, A. H., Cumings, J., et al. (2019). Critical knowledge gaps in mass transport through single-digit nanopores: A review and perspective. J. Phys. Chem. C 123, 21309–21326. doi:10.1021/acs.jpcc.9b02178
Freger, V. (2003). Nanoscale heterogeneity of polyamide membranes formed by interfacial polymerization. Langmuir 19, 4791–4797. doi:10.1021/LA020920Q
Gao, P., Hunter, A., Summe, M. J., and Phillip, W. A. (2016). A method for the efficient fabrication of multifunctional mosaic membranes by inkjet printing. ACS Appl. Mater. Interfaces 8, 19772–19779. doi:10.1021/acsami.6b06048
Gong, G., Wang, P., Zhou, Z., and Hu, Y. (2019). New insights into the role of an interlayer for the fabrication of highly selective and permeable thin-film composite nanofiltration membrane. ACS Appl. Mater. Interfaces 11, 7349–7356. doi:10.1021/acsami.8b18719
Gouaux, E., and MacKinnon, R. (2005). Principles of selective ion transport in channels and pumps. Science 310, 1461–1465. doi:10.1126/science.1113666
Gu, K., Wang, S., Li, Y., Zhao, X., Zhou, Y., and Gao, C. (2019). A facile preparation of positively charged composite nanofiltration membrane with high selectivity and permeability. J. Memb. Sci. 581, 214–223. doi:10.1016/j.memsci.2019.03.057
Gu, Z., Yu, S., Zhu, J., Li, P., Gao, X., and Zhang, R. (2020). Incorporation of lysine-modified UiO-66 for the construction of thin-film nanocomposite nanofiltration membrane with enhanced water flux and salt selectivity. Desalination 493, 114661. doi:10.1016/j.desal.2020.114661
Hatakeyama, E. S., Gabriel, C. J., Wiesenauer, B. R., Lohr, J. L., Zhou, M., Noble, R. D., et al. (2011). Water filtration performance of a lyotropic liquid crystal polymer membrane with uniform, sub-1-nm pores. J. Memb. Sci. 366, 62–72. doi:10.1016/j.memsci.2010.09.028
Hilal, N., Al-Zoubi, H., Darwish, N. A., Mohammad, A. W., and Abu Arabi, M. (2004). A comprehensive review of nanofiltration membranes: Treatment, pretreatment, modelling, and atomic force microscopy. Desalination 170, 281–308. doi:10.1016/j.desal.2004.01.007
Hong, S. U., and Bruening, M. L. (2006). Separation of amino acid mixtures using multilayer polyelectrolyte nanofiltration membranes. J. Memb. Sci. 280, 1–5. doi:10.1016/j.memsci.2006.04.028
Hong, S. U., Malaisamy, R., and Bruening, M. L. (2007). Separation of fluoride from other monovalent anions using multilayer polyelectrolyte nanofiltration membranes. Langmuir 23, 1716–1722. doi:10.1021/la061701y
Hong, S. U., Ouyang, L., and Bruening, M. L. (2009). Recovery of phosphate using multilayer polyelectrolyte nanofiltration membranes. J. Memb. Sci. 327, 2–5. doi:10.1016/j.memsci.2008.11.035
Hu, P., Yuan, B., Niu, Q. J., Chen, K., Xu, Z., Tian, B., et al. (2022). Modification of polyamide nanofiltration membrane with ultra-high multivalent cations rejections and mono-/divalent cation selectivity. Desalination 527, 115553. doi:10.1016/j.desal.2022.115553
Lhassani, A., Rumeau, M., Benjelloun, D., and Pontie, M. (2001). Selective demineralization of water by nanofiltration application to the defluorination of brackish water. Water Res. 35, 3260–3264. doi:10.1016/S0043-1354(01)00020-3
Li, S. L., Li, C., Liu, Y. S., Wang, X. L., and Cao, Z. A. (2003). Separation of L-glutamine from fermentation broth by nanofiltration. J. Memb. Sci. 222, 191–201. doi:10.1016/S0376-7388(03)00290-4
Li, Y., Zhao, Y. J., Wang, H., and Wang, M. (2019). The application of nanofiltration membrane for recovering lithium from salt lake brine. Desalination 468, 114081. doi:10.1016/j.desal.2019.114081
Li, Q., Liu, Y., Liu, Y., Ji, Y., Cui, Z., Yan, F., et al. (2022a). Mg2+/Li+ separation by electric field assisted nanofiltration:the impacts of membrane pore structure, electric property and other process parameters. J. Memb. Sci. 662, 120982. doi:10.1016/j.memsci.2022.120982
Li, Y., Wang, S., Li, H., Kang, G., Sun, Y., Yu, H., et al. (2022b). Preparation of highly selective nanofiltration membranes by moderately increasing pore size and optimizing microstructure of polyamide layer. J. Memb. Sci. 643, 120056. doi:10.1016/j.memsci.2021.120056
Liang, Y., Zhu, Y., Liu, C., Lee, K. R., Hung, W. S., Wang, Z., et al. (2020). Polyamide nanofiltration membrane with highly uniform sub-nanometre pores for sub-1 Å precision separation. Nat. Commun. 11, 2015–2019. doi:10.1038/s41467-020-15771-2
Liu, Z., Qiang, R., Lin, L., Deng, X., Yang, X., Zhao, K., et al. (2022). Thermally modified polyimide/SiO2 nanofiltration membrane with high permeance and selectivity for efficient dye/salt separation. J. Memb. Sci. 658, 120747. doi:10.1016/j.memsci.2022.120747
Lu, D., Ma, T., Lin, S., Zhou, Z., Li, G., An, Q., et al. (2021). Constructing a selective blocked-nanolayer on nanofiltration membrane via surface-charge inversion for promoting Li+ permselectivity over Mg2+. J. Memb. Sci. 635, 119504. doi:10.1016/j.memsci.2021.119504
Lu, D., Yao, Z., Jiao, L., Waheed, M., Sun, Z., and Zhang, L. (2022). Separation mechanism, selectivity enhancement strategies and advanced materials for mono-/multivalent ion-selective nanofiltration membrane. Adv. Membr. 2, 100032. doi:10.1016/j.advmem.2022.100032
Lyu, B., Wang, M., Jiang, J., and Jiang, Z. (2022). Molecular design of covalent − organic framework membranes for Li +/Mg 2 + separation : Significant charge effect. J. Memb. Sci. 662, 120976. doi:10.1016/j.memsci.2022.120976
Malaisamy, R., Talla-Nwafo, A., and Jones, K. L. (2011). Polyelectrolyte modification of nanofiltration membrane for selective removal of monovalent anions. Sep. Purif. Technol. 77, 367–374. doi:10.1016/j.seppur.2011.01.005
Martin-Orue, C., Bouhallab, S., and Garem, A. (1998). Nanofiltration of amino acid and peptide solutions: Mechanisms of separation. J. Memb. Sci. 142, 225–233. doi:10.1016/S0376-7388(97)00325-6
Mohammad, A. W., Teow, Y. H., Ang, W. L., Chung, Y. T., Oatley-Radcliffe, D. L., and Hilal, N. (2015). Nanofiltration membranes review: Recent advances and future prospects. Desalination 356, 226–254. doi:10.1016/j.desal.2014.10.043
Nativ, P., Leifman, O., Lahav, O., and Epsztein, R. (2021). Desalinated brackish water with improved mineral composition using monovalent-selective nanofiltration followed by reverse osmosis. Desalination 520, 115364. doi:10.1016/j.desal.2021.115364
Nightingale, E. R. (1959). Phenomenological theory of ion solvation. Effective radii of hydrated ions. J. Phys. Chem. 63, 1381–1387. doi:10.1021/j150579a011
Oatley-Radcliffe, D. L., Walters, M., Ainscough, T. J., Williams, P. M., Mohammad, A. W., and Hilal, N. (2017). Nanofiltration membranes and processes: A review of research trends over the past decade. J. Water Process Eng. 19, 164–171. doi:10.1016/j.jwpe.2017.07.026
Ouyang, L., Malaisamy, R., and Bruening, M. L. (2008). Multilayer polyelectrolyte films as nanofiltration membranes for separating monovalent and divalent cations. J. Memb. Sci. 310, 76–84. doi:10.1016/j.memsci.2007.10.031
Pan, Y., Xu, R., Lü, Z., Yu, S., Liu, M., and Gao, C. (2017). Enhanced both perm-selectivity and fouling resistance of poly(piperazine-amide) nanofiltration membrane by incorporating sericin as a co-reactant of aqueous phase. J. Memb. Sci. 523, 282–290. doi:10.1016/j.memsci.2016.10.011
Park, H. B., Kamcev, J., Robeson, L. M., Elimelech, M., and Freeman, B. D. (2017). Maximizing the right stuff: The trade-off between membrane permeability and selectivity. Science 356, eaab0530–1148. doi:10.1126/science.aab0530
Pavluchkov, V., Shefer, I., Peer-Haim, O., Blotevogel, J., and Epsztein, R. (2022). Indications of ion dehydration in diffusion-only and pressure-driven nanofiltration. J. Memb. Sci. 648, 120358. doi:10.1016/j.memsci.2022.120358
Richards, L. A., Richards, B. S., Corry, B., and Schäfer, A. I. (2013). Experimental energy barriers to anions transporting through nanofiltration membranes. Environ. Sci. Technol. 47, 1968–1976. doi:10.1021/es303925r
Sarkar, P., Modak, S., and Karan, S. (2021). Ultraselective and highly permeable polyamide nanofilms for ionic and molecular nanofiltration. Adv. Funct. Mater. 31, 2007054–2007058. doi:10.1002/adfm.202007054
Shefer, I., Peer-Haim, O., Leifman, O., and Epsztein, R. (2021). Enthalpic and entropic selectivity of water and small ions in polyamide membranes. Environ. Sci. Technol. 55, 14863–14875. doi:10.1021/ACS.EST.1C04956
Shefer, I., Lopez, K., Straub, A. P., and Epsztein, R. (2022a). Applying transition-state theory to explore transport and selectivity in salt-rejecting membranes: A critical review. Environ. Sci. Technol. 56, 7467–7483. doi:10.1021/acs.est.2c00912
Shefer, I., Peer-Haim, O., and Epsztein, R. (2022b). Limited ion-ion selectivity of salt-rejecting membranes due to enthalpy-entropy compensation. Desalination 541, 116041. doi:10.1016/j.desal.2022.116041
Sheng, C., Wijeratne, S., Cheng, C., Baker, G. L., and Bruening, M. L. (2014). Facilitated ion transport through polyelectrolyte multilayer films containing metal-binding ligands. J. Memb. Sci. 459, 169–176. doi:10.1016/j.memsci.2014.01.051
Song, L., and Elimelech, M. (1995). Theory of concentration polarization in crossflow filtration. Faraday Trans. 91, 3389–3398. doi:10.1039/FT9959103389
Sun, S. Y., Cai, L. J., Nie, X. Y., Song, X., and Yu, J. G. (2015). Separation of magnesium and lithium from brine using a Desal nanofiltration membrane. J. Water Process Eng. 7, 210–217. doi:10.1016/j.jwpe.2015.06.012
Sutzkover, I., Hasson, D., and Semiat, R. (2000). Simple technique for measuring the concentration polarization level in a reverse osmosis system. Desalination 131, 117–127. doi:10.1016/S0011-9164(00)90012-2
Szoke, S., Patzay, G., and Weiser, L. (2003). Characteristics of thin-film nanofiltration membranes at various pH-values. Desalination 151, 123–129. doi:10.1016/S0011-9164(02)00990-6
Umpuch, C., Galier, S., Kanchanatawee, S., and Balmann, H. R. D. (2010). Nanofiltration as a purification step in production process of organic acids: Selectivity improvement by addition of an inorganic salt. Process Biochem. 45, 1763–1768. doi:10.1016/j.procbio.2010.01.015
Van der Bruggen, B., Mänttäri, M., and Nyström, M. (2008). Drawbacks of applying nanofiltration and how to avoid them: A review. Sep. Purif. Technol. 63, 251–263. doi:10.1016/j.seppur.2008.05.010
Wang, L., Rehman, D., Sun, P. F., Deshmukh, A., Zhang, L., Han, Q., et al. (2021). Novel positively charged metal-coordinated nanofiltration membrane for lithium recovery. ACS Appl. Mater. Interfaces 13, 16906–16915. doi:10.1021/acsami.1c02252
Warnock, S. J., Sujanani, R., Zofchak, E. S., Zhao, S., Dilenschneider, T. J., Hanson, K. G., et al. (2021). Engineering Li/Na selectivity in 12-Crown-4–functionalized polymer membranes. Proc. Natl. Acad. Sci. U. S. A. 118, e2022197118. doi:10.1073/pnas.2022197118
Wei, C., He, Z., Lin, L., Cheng, Q., Huang, K., Ma, S., et al. (2018). Negatively charged polyimide nanofiltration membranes with high selectivity and performance stability by optimization of synergistic imidization. J. Memb. Sci. 563, 752–761. doi:10.1016/j.memsci.2018.06.046
Weng, X. D., Bao, X. J., Jiang, H. D., Chen, L., Ji, Y. L., An, Q. F., et al. (2016). pH-responsive nanofiltration membranes containing carboxybetaine with tunable ion selectivity for charge-based separations. J. Memb. Sci. 520, 294–302. doi:10.1016/j.memsci.2016.08.002
Wijmans, J. G., and Baker, R. W. (1995). The solution-diffusion model: a review. J. Memb. Sci. 107, 1–21. doi:10.1016/0376-7388(95)00102-i
Xia, D., Zhang, M., Tong, C., Wang, Z., Liu, H., and Zhu, L. (2022). In-situ incorporating zwitterionic nanocellulose into polyamide nanofiltration membrane towards excellent perm-selectivity and antifouling performances. Desalination 521, 115397. doi:10.1016/j.desal.2021.115397
Yang, Z., Zhou, Z. W., Guo, H., Yao, Z., Ma, X. H., Song, X., et al. (2018). Tannic acid/Fe3+ nanoscaffold for interfacial polymerization: Toward enhanced nanofiltration performance. Environ. Sci. Technol. 52, 9341–9349. doi:10.1021/acs.est.8b02425
Yang, Z., Fang, W., Wang, Z., Zhang, R., Zhu, Y., and Jin, J. (2021). Dual-skin layer nanofiltration membranes for highly selective Li+/Mg2+ separation. J. Memb. Sci. 620, 118862. doi:10.1016/j.memsci.2020.118862
Yuan, B., Wang, N., Zhao, S., Hu, P., Jiang, J., Cui, J., et al. (2022). Polyamide nanofiltration membrane fine-tuned via mixed matrix ultrafiltration support to maximize the sieving selectivity of Li+/Mg2+ and Cl–/SO42. Desalination 538, 115929. doi:10.1016/j.desal.2022.115929
Zhang, Z., Kang, G., Yu, H., Jin, Y., and Cao, Y. (2019). From reverse osmosis to nanofiltration: Precise control of the pore size and charge of polyamide membranes via interfacial polymerization. Desalination 466, 16–23. doi:10.1016/j.desal.2019.05.001
Zhang, Y., Song, Q., Liang, X., Wang, J., Jiang, Y., and Liu, J. (2020). High-flux, high-selectivity loose nanofiltration membrane mixed with zwitterionic functionalized silica for dye/salt separation. Appl. Surf. Sci. 515, 146005. doi:10.1016/j.apsusc.2020.146005
Zhao, Y., Tong, T., Wang, X., Lin, S., Reid, E. M., and Chen, Y. (2021a). Differentiating solutes with precise nanofiltration for next generation environmental separations: A review. Environ. Sci. Technol. 55, 1359–1376. doi:10.1021/acs.est.0c04593
Zhao, Y., Tong, X., and Chen, Y. (2021b). Fit-for-purpose design of nanofiltration membranes for simultaneous nutrient recovery and micropollutant removal. Environ. Sci. Technol. 55, 3352–3361. doi:10.1021/acs.est.0c08101
Keywords: ion selectivity, ion transport, water permeability, solute rejection, concentration polarization, reverse osmosis, polyamide membranes
Citation: Epsztein R (2022) Intrinsic limitations of nanofiltration membranes to achieve precise selectivity in water-based separations. Front. Membr. Sci. Technol. 1:1048416. doi: 10.3389/frmst.2022.1048416
Received: 19 September 2022; Accepted: 17 October 2022;
Published: 11 November 2022.
Edited by:
Santanu Karan, Central Salt and Marine Chemicals Research Institute (CSIR), IndiaReviewed by:
Gyorgy Szekely, King Abdullah University of Science and Technology, Saudi ArabiaNadir Dizge, Mersin University, Turkey
Copyright © 2022 Epsztein. This is an open-access article distributed under the terms of the Creative Commons Attribution License (CC BY). The use, distribution or reproduction in other forums is permitted, provided the original author(s) and the copyright owner(s) are credited and that the original publication in this journal is cited, in accordance with accepted academic practice. No use, distribution or reproduction is permitted which does not comply with these terms.
*Correspondence: Razi Epsztein, cmF6aWVwc3p0ZWluQHRlY2huaW9uLmFjLmls