- 1Department of Health Science and Technology, Graduate School of Convergence Science and Technology, Seoul National University, Seoul, Republic of Korea
- 2School of Mechanical Engineering, Korea University of Technology and Education, Cheonan-si, Republic of Korea
- 3Division of Pulmonary and Critical Care Medicine, Department of Internal Medicine, Seoul National University College of Medicine, Seoul National University Bundang Hospital, Seongnam-si, Republic of Korea
Inhalation of ultrafine particles, aerosol contaminants, and cigarette smoke can induce respiratory diseases. As humans are constantly exposed to various exogenous substances, it is crucial to study their impacts on respiratory diseases and airway dysfunction. Recently, organ-on-a-chip technology has been applied in many research studies to understand disease mechanisms, drug screening, and drug testing. The combination of organ-on-a-chip technology and the air-liquid interface (ALI) culture method is emerging as a new platform for realistically mimicking the microenvironment and physiological motions of the human lungs. Breathing motion can be simulated through cyclic stretching, while blood flow can be replicated using channel flow within the chip. The ALI system is critical for mucociliary differentiation, pseudostratified morphology, and epithelial barrier function development. The combination of organ-on-a-chip technology and ALI systems allows the integration of cyclic stretch as a breathing motion and microfluidic channels as circulatory systems. The chip system can also integrate the lung epithelial cells, extracellular matrix, and microstructures, providing microenvironments such as fibroblast, collagen, and immune cells to the epithelial cells. This review discusses chip systems as effective tools for recapitulating human lung environments and how they are applied in biological studies against various pulmonary diseases such as infections or inflammation, fibrosis, and malignancy.
1 Introduction
The respiratory system contains essential structures that are vital to airflow in and out of the body. At the same time, it is the entrance for harmful substances that might combine with the air and access the body through breathing. Disease arising from aerosol impurities is detected when considerable implications on the respiratory system have occurred. Respiratory diseases occur not only via the entrance of impurities through aerosol, but also by emerging viral infection or lung cancer. Current research in drug development and toxicology is making progress in simulating the physiological respiratory system. Furthermore, traditional pulmonary research using cell lines and animals does not fully replicate the functional and structural complexity of human tissues and organs. Considering the significance of recapitulating the respiratory system, the lung-on-a-chip and ALI culture method provides a breakthrough in understanding the respiratory system. The lung-on-a-chip and ALI culture method offers a realistic micro-environment of the human lung. The ALI systems show many advantages over 2D culture systems and submerged culture systems in reproducing the in vivo characteristics of the pulmonary epithelial cells. For this reason, this review paper presents an overview of the commercially available lung-on-a-chip and ALI combination to suggest the importance of organ-on-a-chip technology in mimicking the breathing process and lung fluids.
2 Important considerations for lung-on-chip systems
Structurally, the organs in the pulmonary system establish a continuous air passage system, enabling the inflow and outflow of air in the body. Therefore, for effective functional operations, the respiratory system is divided into two sections, the upper and lower respiratory tract. Each section comprises unique organs and cell components characterized by their unique functions, and it is important to effectively recapitulate the following characteristics in a lung-on-a-chip device.
2.1 Shear stress
Besides ensuring efficient passage of air, the upper respiratory tract also heats and humidifies the air flowing into the lower respiratory tract (Pierce and Worsnop, 1999). Therefore, maintaining the micro-environment of lung-on-a-chip with filtered and humidified air between exposures is crucial to best mimic these physiological conditions, including adequate mucus production and periciliary layers on the epithelial surface. The epithelium lining in the lung airways is exposed to continuous air-flow shear stress by the bi-directional airflow caused by respiration. The degree of shear stress varies across different parts of the lung due to differences in the size of the airway, air flow rate, and humidity. However, the role of shear stress in regulating the stability and development of the epithelium lining in the airways has not yet been made clear, even though the process of cyclic stretch is well understood to support the development and secretion of surfactant in alveolar cells (Edwards, 2001). A recent study revealed that airway epithelial cells exhibit more representative mucociliary differentiation under the bi-directional airflow when compared to the effects of constant uni-directional airflow in microfluidic devices (Park et al., 2023). Not only is the extent of airway cell differentiation influenced by mechanical transduction in microfluidic devices, but so is the composition of airway cells. A study utilized the Chip-S1® device (Emulate Bio, US), seeding Primary Human Bronchial Epithelial Cells (PBEC) from lung cancer patients in the top channel of the device, while endothelial cells were co-cultured in the lower channel. The device was calibrated to recapitulate physiological conditions by adjusting the airflow rate of about 1.2 μL/s, and they performed a bulk RNA-sequencing analysis. As a result, this study found that epithelial cells under ALI showed cell differentiation related to tissue development (Nawroth et al., 2023). In vivo, the lung tissue is supported by lung microvasculature, and the circulating blood applies shear stress to the endothelium. The endothelium plays a crucial role in controlling the movement of compounds, fluids, and pathogens. This regulation supports essential functions such as fluid balance, gas exchange, immune response, and molecular transport (Buchanan et al., 2014). Therefore, designing lung-on-a-chip devices that effectively mimic the shear stress of circulating blood can enhance our understanding of the physiological aspects of vasculatures with microfluidic devices.
2.2 Cyclic stretch
The alveoli ensure an adequate and efficient exchange of gases by expanding during inhalation and shrinking when exhalation occurs (Peate, 2018). Furthermore, the mechanical stretching of the alveoli is essential for the synthesis and secretion of pulmonary surfactant, which includes the four major surfactant proteins (SP): SP-A, SP-B, SP-C, and SP-D (Gutierrez et al., 1998). Given these dynamics, it is important to create in vitro lung models that not only emulate the natural physiological forces exerted on alveolar cells but also provide the ALI state of alveolar cells to closely mimic the lung physiology environment. A novel study introduced a lung-on-a-chip model that mimicked the pulmonary parenchymal environment and included cyclic strain induced by breathing motion. This lung-on-a-chip device is constructed from polydimethylsiloxane (PDMS) and impacts the cells to a maximum of 10% linear cyclic strain. The lung bronchial epithelial cell line 16HBE14o− cell was used to study epithelial barrier functions, including under cyclic strain. The FITC-sodium permeability test revealed that cyclic stretching applied to the cells increased the permeability of the epithelial barrier. The increased permeability showed a better simulation of the molecular exchange processes of the human lung (Stucki et al., 2015). Along with the epithelial barrier permeability, cyclic mechanical stretch also influences the intracellular uptake ability of alveolar epithelial cells. A recent study found that alveolar epithelial cells (A549) cultured under ALI conditions showed increased intracellular uptake to nano-sized particles delivered via aerosol, an effect attributed to the influence of cyclic stretch (10% linear, 0.33 Hz). In this experiment, a Cyclic In Vitro Cell-stretch system was used, which is a bioreactor designed to apply cyclic mechanical stretching on the BETA membrane for lung epithelial cell experiments under ALI conditions. This study used confocal laser scanning microscopy to assess how cyclic stretch affects the uptake of 100 nm nanoparticles by A549 cells, revealing that cyclic stretching significantly enhanced the internalization of these particles into the F-actin cytoskeleton deep within the cells (Doryab et al., 2021). The “alveolar lung-on-a-chip” model replicates human pulmonary alveoli function using a three-dimensional porous hydrogel of gelatin methacryloyl with an inverse opal structure affixed to a PDMS chip. In a study, this model featured primary human alveolar epithelial cells (hAECs) that undergo cyclic stretches simulating natural breathing movements, specifically applying an 8% cyclic stretch to emulate the physiological expansion and contraction of alveoli. In the alveolar lung-on-a-chip model, hAECs maintained viability and preserved epithelial structure under ALI conditions and simulated breathing for 48 h. Additionally, cyclic mechanical strains also enhanced the formation of tight junctions among the cells, as indicated by zonula occludens-1 (ZO-1) staining (Huang et al., 2021). To simulate ventilator-induced lung injury (VILI), immortalized murine lung alveolar epithelial cells (MLE-12) were seeded on a lung-on-a-chip composed of a thin stretchable poly(caprolactone) nanofibrous membrane and a cyclic pathophysiologic stretch (approximately 25%) was applied. VILI is characterized by an increase in tissue inflammation during the initial acute injury phase, often followed by a subsequent fibrotic response that can cause irreversible lung damage. It was found that pathologic stretch induced cell death and nuclear translocation of the mechanotransducers YAP/TAZ protein, signaling the cells’ activation of the stress response, survival, and repair mechanisms (Tas et al., 2021).
2.3 Air liquid interface
2.3.1 Signaling pathway involved in ALI
The impact of the ALI method on signaling pathways and cell differentiation mainly facilitates the activation of signaling pathways that are naturally involved in lung development and function, while submerged conditions inhibit cell differentiation. It has been suggested that the ALI method impacts lung cell differentiation by regulating key signaling pathways, especially Notch and Wnt signaling pathways.
Gerovac et al. (2014) investigated the critical role of the Notch signaling pathway in regulating the differentiation of lung cells. This study highlighted the importance of the ALI condition as the submersion method prevents ciliated cell differentiation of bronchial epithelial cells by making the cells hypoxic. It was supported by the observation that the transcription factor of hypoxic response mediator, HIF-1alpha and HIF-2alpha, starts to accumulate rapidly, and ciliated cell differentiation is reduced when the cell culture method was switched from ALI to the submerged or hypoxia condition. Under the submerged or hypoxia condition, those proteins trigger Notch signaling to repress MCI and FOXJ1 expression, factors necessary for ciliated cell differentiation (Gerovac et al., 2014). It concluded that the ciliated cell differentiation requires not only the establishment of ALI condition but also the interruption of the Notch signaling pathway. Schmid et al. (2017) observed the effect of the Wnt signaling pathway on the differentiation of ciliated lung epithelial cells under ALI conditions. It emphasized the importance of Wnt signaling modulation in the redifferentiation of the lung cells under ALI conditions for modeling the airway cell repair process. The enhanced β-catenin signaling, a component of the Wnt pathway, reduces the number of ciliated cells and leads to squamous changes in the epithelium. Conversely, inhibition of canonical Wnt signaling increases the number of ciliated cells (Schmid et al., 2017).
2.3.2 Effect of ALI culture on lung cells
The ALI method is advantageous in promoting cell differentiation and optimizing the histological and morphological characteristics of lung airway cells. Models including 3D culture under ALI conditions mimic better in vivo environment of the lung, which provides more physiological conditions (Figure 1). Primary cells are considered the most appropriate for simulating lung physiology, and the upper and lower lung parts are represented by normal human epithelial cells (NHBE) and hAECs, respectively. Comparing the cellular features after the ALI culture of these lung cells will be a starting point for validating in vitro ALI lung models. Under experimental conditions, ALI culture is an effective method for inducing ciliated airway epithelial cell differentiation. A study compared the degree of ciliogenesis in response to different volumes of apical media when NHBE cells were seeded on a transwell. As the volume of transwell apical media increased, the number of ciliated cells decreased, while the smallest amount of surface liquid resulted in the maximum stimulation of ciliogenesis (Gerovac et al., 2014). Not only did the number of ciliated cells increase after ALI cultures, but the number of mucous-producing cells also increased. When the bronchial primary cells were cultured under ALI, the pseudostratified and differentiated epithelial layers were formed, and the epithelial thickness was grown, reaching a height similar to that of actual human bronchial epithelial thickness (Leung et al., 2020). Representative cell lines from the lung bronchial region suitable for ALI culture include 16HBE14o- and Calu-3. Specifically, the 16HBE14o-cell line, which originates from NHBE, was transformed using an SV40 plasmid (Cozens et al., 1992). Unlike the results observed in NHBE cells under ALI conditions, a study comparing ALI and liquid-submerged conditions on the 16HBE14o-cell line showed higher transepithelial electrical resistance (TEER) values and more distinct expressions of junctional proteins under the liquid-submerged conditions (Ehrhardt et al., 2002). The Calu-3 cell line, originating from bronchial adenocarcinoma, lost some of its cell cycle checkpoint as a result of mutation. When grown in submerged conditions, the Calu-3 cell line showed higher TEER values than when cultured under ALI conditions. The study linked this TEER value difference to their morphological disparities in that the cells under ALI exhibited a folded morphology, whereas cells in liquid displayed a monolayer structure (Stentebjerg-Andersen et al., 2011). Additionally, it has been revealed that the Calu-3 cell line is unsuitable for studies of cilia function, a feature of ALI cultures, as it lacks basal cells and kinocilia (Lodes et al., 2020).
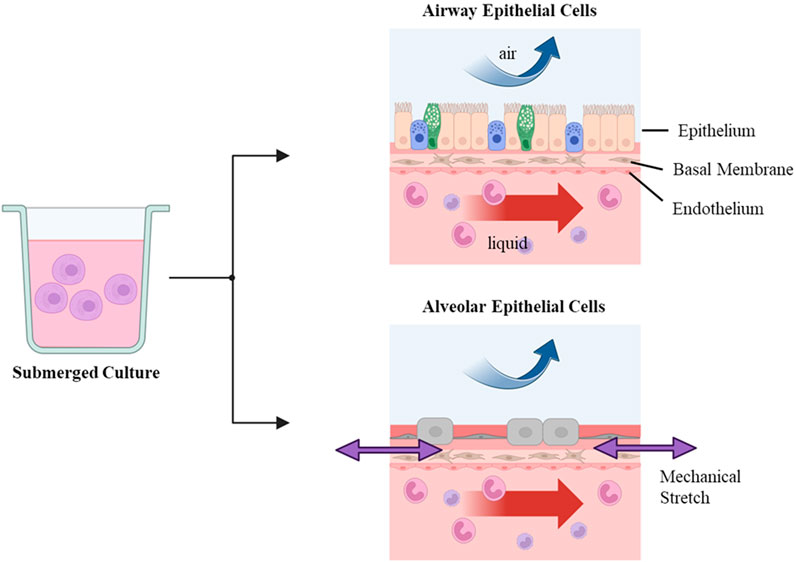
Figure 1. In vitro air-liquid interface (ALI) culture stage ALI culture can be realized by submerged culture and sequential air-exposing culture steps. Edits by BioRender.
In the case of the alveolar epithelium, it has been known that primary adult human alveolar type (AT) 2 cells cease to replicate when cultured in vitro and, within several days, start to trans-differentiate into AT1-like cells under ALI conditions. Therefore, to investigate the effects of ALI culture on alveolar cells, there is a need for cell lines that represent alveolar characteristics and can overcome long-term survivability. There are representative cell lines such as adenocarcinoma-derived NCI-H441, human alveolar epithelial lentivirus immortalized (hAELVi) cell line, and A549 that serve as in vitro models of the alveolar epithelial barrier. Lochbaum et al. (2020) demonstrated that ALI conditions enhanced TEER in NCI-H441 cells, and it was attributed to the strengthening of tight junctions rather than to alterations in active transcellular ion transport. Also, hAELVi cell lines showed enhanced TEER under ALI conditions, although it showed a heterogenous cell population due to the initial immortalization of a single donor human alveolar epithelial cells of CD326-positive (Kuehn et al., 2016). For A549 cell lines, a study conducted media tests to better replicate alveolar epithelial characteristics under ALI conditions. A study transitioned the A549 cell line from fetal bovine serum (FBS) media to two FBS-free media: X-
3 Commercially-available lung-on-chip systems
The lung-on-a-chip comprises a three-dimensional simulation of a human lung on a microchip that is capable of breathing. It is created from human lung and blood vessel cells and can replicate the inflammatory response brought on by microbial infections and it anticipates the absorption of airborne nanoparticles. Lung-on-a-chip is used to study the physiological behavior of primary human lung cells under respiratory disease models. The lung-on-a-chip enables the ability to emulate the physiology model of the lung and the identification of the most appropriate intervention for a lung disease problem (Shrestha et al., 2020). The co-culture systems enable the study of interactions between lung epithelial cells and endothelial cells incorporating lung-related fibroblasts and solubilized human lung extracellular matrix. To date, lung-on-a-chip prototypes have continued to evolve and are being used in the research fields to accurately replicate aspects of the respiratory and vascular system. Furthermore, they serve as models for respiratory infection and assess the potential treatment effects (Table 1). Table 1 lists commercially available lung-on-a-chip models that are optimal for the ALI culture system, enhancing the replication of the respiratory tract. Below is a brief description of relevant companies that provide lung-on-a-chip technology, organized in alphabetical order by company name.
• AlveoliX, founded in 2019 in Switzerland, specializes in developing organ-on-a-chip, which can be supported by the cloud-based exposure chamber (VITROCELL, Germany) as an inhalation tool. Sengupta et al. (2023) designed a model integrating a cloud-based exposure chamber with an AXLung-on-chip system, allowing precise aerosol delivery to lung cells under ALI and cyclic stretch conditions of the chip membrane. The study investigated the effects of toxic nanoparticles such as titanium dioxide, zinc oxide, and polyhexamethylene guanidine on lung epithelial barrier sensitivity, cytotoxicity, and inflammation. This advancement enhances the inhalation toxicology field with a deeper understanding of how inhaled particles interact with lung tissue (Sengupta et al., 2023). A study also used the AXLung-on-chip system to culture lung cells under ALI condition. Over the same period, the cells exhibited robust barrier formation and increased transcription levels of AT1 marker (AQP5) and AT2 cell marker (ATP-binding cassette sub-family A member 3, SP-C), exceeding the results from submerged conditions. This study confirmed that the ALI culture significantly enhanced the alveolar characteristics, making the model effective for simulating lung function (Sengupta et al., 2022).
• BEOnChip, founded in 2016 at the University of Zaragoza in Spain, specializes in the development of organ-on-a-chip devices using advanced materials such as lipophobic thermoplastic polymers. BEONchip was used in a study designed to effectively study how substances diffuse through the stratified epithelium. By using a stratified epithelium-on-a-chip model, nanoparticle permeability and Stroke radius were studied as their ability to cross the tissue barrier impacts their uptake into the bloodstream, which, in turn, can be useful for the development of drug efficiency and toxicological assessment (Fernández-Carro et al., 2023).
• BiomimiX, founded in Italy in 2017, developed uBeat Stretch Platform, which provides a physiological uniaxial strain of 10% to 3D microtissues, making it suitable for culturing tissues that undergo stretching stimuli in vivo (BiomimX, 2024).
• CN Bio, founded in 2009 by a UK company, commercialized organ-on-a-chip to provide a microfluidic system mimicking the bloodstream. This organ-on-a-chip consists of multiple bioreactor chambers, each outfitted with a lower microfluidic chamber that is connected to a fluid reservoir. A study established a dynamic flow condition by using CNBio’s PhysioMimix™, which allows continuous media perfusion in the basolateral compartment at a flow rate of 0.5 μL/s. In this experiment, NHBE monoculture and NHBE-normal human lung fibroblasts (NHLF) co-culture models were maintained under both static and flow systems with ALI conditions. Both models in flow system condition retained the ‘hairy’ structure of ciliated cells, while under static conditions, the cilia structure was absent. Additionally, the expression goblet cell marker (MUC5B) attained a higher flow condition when compared to static condition, suggesting that dynamic flow conditions promoted the differentiation and maturation of the models (Phan et al., 2023). Currently, a PhysioMimix lung-on-a-chip model has been utilized in collaboration with the FDA to help assess the impact of inhaled drugs under realistic conditions (CN Bio, 2024).
• Emulate, founded in 2014 by Harvard University, specializes in creating organ-on-a-chip and microfluidic systems. Si et al. (2021) demonstrated that an air-on-a-chip model has the capability to replicate the influenza A virus infection and mimic an immune response. Furthermore, it also tested repurposing approved drugs for potential anti-influenza therapeutics (Si et al., 2021). A study also used the emulate chip in an ALI lung-on-a-chip system to enhance the assessment of T-cell bispecific antibodies’ (TCBs) effects and safety on lung alveolar epithelial cells. This study assessed the toxicity of TCBs targeting the folate receptor 1 (FOLR1), expressed in lung epithelial cells. Consequently, the FOLR1 gene and protein expression were confirmed in this mature alveolus lung-chip model (Kerns et al., 2021).
• MIMETAS, founded in 2014 in the Netherlands, the company’s OrganoPlate platform features numerous adaptable microfluidic designs and specialized tools, allowing unique tissue cultures and applications. A study presented a lung tumor-on-a-chip model with 3D endothelium by using a three-lane OrganoPlate, populated with human umbilical vein endothelial cells (HUVECs), non-small cell lung carcinoma cells (HCC0827), and primary T cells. This system effectively simulates T-cell adhesion, extravasation, and migration, playing a vital role in understanding immune cell behavior within a tumor (Wisdom et al., 2023).
• REACT4LIFE, founded in 2016 in Italy, specializes in accommodating 3D tissue models of clinically relevant sizes and mimicking the blood flow. The organ-on-a-chip technology (MIVO system) is designed without PDMS to avoid unintended molecule binding. This design ensures that the MIVO system maintains physiological conditions, preventing immune cells from adhering to the walls (React4Life, 2024).
• Synvivo, founded in 2015 by a US company, commercialized organ-on-chip models to simulate microvasculature and organ structure. Liu et al. (2019) used lung-on-a-chip (SynVivo Inc.) to optimize the microfluidic model by integrating human bronchial epithelial cells with co-cultured endothelial cells. This model allowed an understanding of airway functionality and disease mechanism through imaging techniques such as micro-optical coherence tomography, enabling precise measurement of cilia movement, beat frequency, and mucociliary transport. The integration of these imaging technologies with the lung-on-a-chip model will enhance our comprehension of the respiratory system (Liu et al., 2019).
• TissUse, founded in 2010 in Germany, specializes in developing tissue-on-chip technology. A study used a HUMIMIC chip to optimize the co-culture model of a bronchial MucilAir tissue and liver spheroids to assess the effects of aerosol toxicity. This study tested aflatoxin B1, known as a hepatotoxic and carcinogenic compound, to explore the interaction between the lung and liver due to its metabolization occurring in both organs. Under the aflatoxin B1 exposure, the co-culture model demonstrated a decrease in functionality and viability of liver spheroid compared to the monoculture model, which highlights the importance of multi-organ models in better understanding the complex interactions affecting human health (Schimek et al., 2020).
4 Conclusion
Lung-on-a-chip technology enables the simulation of lung epithelial cells stretching the membrane and mimics the dynamic flow of fluids, closely resembling the lung’s in vivo conditions. The ALI culture technique is particularly beneficial for allowing the lung cells to differentiate more effectively compared to submerged cultures. Therefore, the combination of lung-on-a-chip and ALI exposure with microfluidics technology offers a more physiologically relevant model than traditional cell culture methods. These models are increasingly used for studying lung diseases, drug discovery, and toxicology studies due to their ability to mimic the human lung environment more accurately than traditional 2D cell cultures. The market for these models is expanding, driven by the need for more effective lung disease treatments, the rise of personalized medicine, and the shift away from animal testing. Technological advancements in this field, such as the use of organoids, microfluidics, and advanced imaging techniques, are enhancing the utility and accuracy of these models. Despite their promise, the development of in vitro lung models faces challenges such as complexity, high cost, and the need to replicate the intricate structure and function of human lungs. The development of ALI and the lung-on-a-chip model has opened new doors for understanding lung physiology and predicting human physiological responses to drugs and toxins. Therefore, though there are challenges such as high cost, complexity, and low experimental reproducibility, the development of organ-on-a-chip is necessary to enhance our understanding and treatment approaches.
Author contributions
SP: Writing–original draft, Writing–review and editing, Conceptualization, Data curation, Formal Analysis, Funding acquisition, Investigation, Methodology, Project administration, Resources, Software, Supervision, Validation, Visualization. CW: Conceptualization, Data curation, Formal Analysis, Funding acquisition, Investigation, Methodology, Project administration, Resources, Software, Supervision, Validation, Visualization, Writing–original draft, Writing–review and editing. Y-JC: Writing–review and editing, Writing–original draft, Visualization, Validation, Supervision, Software, Resources, Project administration, Methodology, Investigation, Funding acquisition, Formal Analysis, Data curation, Conceptualization.
Funding
The author(s) declare that financial support was received for the research, authorship, and/or publication of this article. This was supported by Bio Industrial Technology Development Program (20018463, Development of 3D lung and environment microphysiological platform for acute respiratory diseases) funded By the Ministry of Trade, Industry and Energy (MOTIE, Korea).
Acknowledgments
We gratefully acknowledge SP, CW, and Y-JC for their expert contributions, and thank Seoul National University, KOREATECH, and Seoul National University Bundang Hospital for their support and resources. Our sincere appreciation also goes to our funding agencies and our families for their invaluable support.
Conflict of interest
The authors declare that the research was conducted in the absence of any commercial or financial relationships that could be construed as a potential conflict of interest.
Publisher’s note
All claims expressed in this article are solely those of the authors and do not necessarily represent those of their affiliated organizations, or those of the publisher, the editors and the reviewers. Any product that may be evaluated in this article, or claim that may be made by its manufacturer, is not guaranteed or endorsed by the publisher.
References
BiomimX (2024). Home page. Available at: https://www.biomimx.com.
Buchanan, C. F., Verbridge, S. S., Vlachos, P. P., and Rylander, M. N. (2014). Flow shear stress regulates endothelial barrier function and expression of angiogenic factors in a 3D microfluidic tumor vascular model. Cell adhesion Migr. 8 (5), 517–524. doi:10.4161/19336918.2014.970001
Chary, A., Groff, K., Stucki, A. O., Contal, S., Stoffels, C., Cambier, S., et al. (2022). Maximizing the relevance and reproducibility of A549 cell culture using FBS-free media. Toxicol. Vitro 83, 105423. doi:10.1016/j.tiv.2022.105423
CN Bio (2024). Home page. Available at: https://cn-bio.com.
Cozens, A. L., Yezzi, M. J., Yamaya, M., Steiger, D., Wagner, J. A., Garber, S. S., et al. (1992). A transformed human epithelial cell line that retains tight junctions post crisis. Vitro Cell. Dev. Biology-Animal 28, 735–744. doi:10.1007/bf02631062
Doryab, A., Taskin, M. B., Stahlhut, P., Schröppel, A., Orak, S., Voss, C., et al. (2021). A bioinspired in vitro lung model to study particokinetics of nano-/microparticles under cyclic stretch and air-liquid interface conditions. Front. Bioeng. Biotechnol. 9, 616830. doi:10.3389/fbioe.2021.616830
Edwards, Y. S. (2001). Stretch stimulation: its effects on alveolar type II cell function in the lung. Comp. Biochem. Physiology Part A Mol. Integr. Physiology 129 (1), 245–260. doi:10.1016/s1095-6433(01)00321-x
Ehrhardt, C., Kneuer, C., Fiegel, J., Hanes, J., Schaefer, U., Kim, K. J., et al. (2002). Influence of apical fluid volume on the development of functional intercellular junctions in the human epithelial cell line 16HBE14o–: implications for the use of this cell line as an in vitro model for bronchial drug absorption studies. Cell tissue Res. 308, 391–400. doi:10.1007/s00441-002-0548-5
Fernandez-Carro, E., Salomon-Cambero, R., Armero, L., Castro-Abril, H. A., Ayensa-Jiménez, J., Martínez, M. A., et al. (2023). Nanoparticles Stokes radius assessment through permeability coefficient determination within a new stratified epithelium on-chip model. Artif. Cells, Nanomedicine, Biotechnol. 51 (1), 466–475. doi:10.1080/21691401.2023.2253534
Gerovac, B. J., Valencia, M., Baumlin, N., Salathe, M., Conner, G. E., and Fregien, N. L. (2014). Submersion and hypoxia inhibit ciliated cell differentiation in a notch-dependent manner. Am. J. Respir. Cell Mol. Biol. 51 (4), 516–525. doi:10.1165/rcmb.2013-0237OC
Gutierrez, J. A., Gonzalez, R. F., and Dobbs, L. G. (1998). Mechanical distension modulates pulmonary alveolar epithelial phenotypic expression in vitro. Am. J. Physiology-Lung Cell. Mol. Physiology 274 (2), L196–L202. doi:10.1152/ajplung.1998.274.2.l196
Huang, D., Liu, T., Liao, J., Maharjan, S., Xie, X., Pérez, M., et al. (2021). Reversed-engineered human alveolar lung-on-a-chip model. Proc. Natl. Acad. Sci. U. S. A. 118 (19), e2016146118. doi:10.1073/pnas.2016146118
Kerns, S. J., Belgur, C., Petropolis, D., Kanellias, M., Barrile, R., Sam, J., et al. (2021). Human immunocompetent Organ-on-Chip platforms allow safety profiling of tumor-targeted T-cell bispecific antibodies. Elife 10, e67106. doi:10.7554/elife.67106
Kuehn, A., Kletting, S., de Souza Carvalho-Wodarz, C., Repnik, U., Griffiths, G., Fischer, U., et al. (2016). Human alveolar epithelial cells expressing tight junctions to model the air-blood barrier. ALTEX 33, 251–260. doi:10.14573/altex.1511131
Leung, C., Wadsworth, S. J., Yang, S. J., and Dorscheid, D. R. (2020). Structural and functional variations in human bronchial epithelial cells cultured in air-liquid interface using different growth media. Am. J. Physiology-Lung Cell. Mol. Physiology 318 (5), L1063–L1073. doi:10.1152/ajplung.00190.2019
Liu, Z., Mackay, S., Gordon, D. M., Anderson, J. D., Haithcock, D. W., Garson, C. J., et al. (2019). Co-cultured microfluidic model of the airway optimized for microscopy and micro-optical coherence tomography imaging. Biomed. Opt. express 10 (10), 5414–5430. doi:10.1364/boe.10.005414
Lochbaum, R., Schilpp, C., Nonnenmacher, L., Frick, M., Dietl, P., and Wittekindt, O. H. (2020). Retinoic acid signalling adjusts tight junction permeability in response to air-liquid interface conditions. Cell. Signal. 65, 109421. doi:10.1016/j.cellsig.2019.109421
Lodes, N., Seidensticker, K., Perniss, A., Nietzer, S., Oberwinkler, H., May, T., et al. (2020). Investigation on ciliary functionality of different airway epithelial cell lines in three-dimensional cell culture. Tissue Eng. Part A 26 (7-8), 432–440. doi:10.1089/ten.tea.2019.0188
Nawroth, J. C., Roth, D., van Schadewijk, A., Ravi, A., Maulana, T. I., Senger, C. N., et al. (2023). Breathing on chip: dynamic flow and stretch accelerate mucociliary maturation of airway epithelium in vitro. Mater. Today Bio 21, 100713. doi:10.1016/j.mtbio.2023.100713
Park, S., Newton, J., Hidjir, T., and Young, E. W. (2023). Bidirectional airflow in lung airway-on-a-chip with matrix-derived membrane elicits epithelial glycocalyx formation. Lab a Chip 23 (16), 3671–3682. doi:10.1039/d3lc00259d
Peate, I. (2018). Anatomy and physiology, 10. The respiratory system. Br. J. Healthc. Assistants 12 (4), 178–181. doi:10.12968/bjha.2018.12.4.178
Phan, T. H., Shi, H., Denes, C. E., Cole, A. J., Wang, Y., Cheng, Y. Y., et al. (2023). Advanced pathophysiology mimicking lung models for accelerated drug discovery. Biomaterials Res. 27 (1), 35. doi:10.1186/s40824-023-00366-x
Pierce, R. J., and Worsnop, C. J. (1999). Upper airway function and dysfunction in respiration. Clin. Exp. Pharmacol. physiology 26 (1), 1–10. doi:10.1046/j.1440-1681.1999.02988.x
React4Life (2024). Home page. Available at: https://www.react4life.com.
Schimek, K., Frentzel, S., Luettich, K., Bovard, D., Rütschle, I., Boden, L., et al. (2020). Human multi-organ chip co-culture of bronchial lung culture and liver spheroids for substance exposure studies. Sci. Rep. 10 (1), 7865. doi:10.1038/s41598-020-64219-6
Schmid, A., Sailland, J., Novak, L., Baumlin, N., Fregien, N., and Salathe, M. (2017). Modulation of Wnt signaling is essential for the differentiation of ciliated epithelial cells in human airways. FEBS Lett. 591 (21), 3493–3506. doi:10.1002/1873-3468.12851
Sengupta, A., Dorn, A., Jamshidi, M., Schwob, M., Hassan, W., De Maddalena, L. L., et al. (2023). A multiplex inhalation platform to model in situ like aerosol delivery in a breathing lung-on-chip. Front. Pharmacol. 14, 1114739. doi:10.3389/fphar.2023.1114739
Sengupta, A., Roldan, N., Kiener, M., Froment, L., Raggi, G., Imler, T., et al. (2022). A new immortalized human alveolar epithelial cell model to study lung injury and toxicity on a breathing lung-on-chip system. Front. Toxicol. 4, 840606. doi:10.3389/ftox.2022.840606
Shrestha, J., Razavi Bazaz, S., Aboulkheyr Es, H., Yaghobian Azari, D., Thierry, B., Ebrahimi Warkiani, M., et al. (2020). Lung-on-a-chip: the future of respiratory disease models and pharmacological studies. Crit. Rev. Biotechnol. 40 (2), 213–230. doi:10.1080/07388551.2019.1710458
Si, L., Bai, H., Rodas, M., Cao, W., Oh, C. Y., Jiang, A., et al. (2021). A human-airway-on-a-chip for the rapid identification of candidate antiviral therapeutics and prophylactics. Nat. Biomed. Eng. 5 (8), 815–829. doi:10.1038/s41551-021-00718-9
Stentebjerg-Andersen, A., Notlevsen, I. V., Brodin, B., and Nielsen, C. U. (2011). Calu-3 cells grown under AIC and LCC conditions: implications for dipeptide uptake and transepithelial transport of substances. Eur. J. Pharm. Biopharm. 78 (1), 19–26. doi:10.1016/j.ejpb.2010.12.030
Stucki, A. O., Stucki, J. D., Hall, S. R., Felder, M., Mermoud, Y., Schmid, R. A., et al. (2015). A lung-on-a-chip array with an integrated bio-inspired respiration mechanism. Lab a Chip 15 (5), 1302–1310. doi:10.1039/c4lc01252f
Tas, S., Rehnberg, E., Bölükbas, D. A., Beech, J. P., Kazado, L. N., Svenningsson, I., et al. (2021). 3D printed lung on a chip device with a stretchable nanofibrous membrane for modeling ventilator induced lung injury. bioRxiv, 2021–2107. doi:10.1101/2021.07.02.450873
Wisdom, K. M., Suijker, J., Van den Broek, L., Sridharan, B., Grandhi, T. S. P., Cheng, A., et al. (2023). Lung tumor microphysiological system with 3D endothelium to evaluate modulators of T-cell migration. ALTEX-Alternatives animal Exp. 40 (4), 649–664. doi:10.14573/altex.2208121
Keywords: inhalation, lung-on-chip, air liquid interface, microphysiological systems, in vitro
Citation: Park S, Woo CG and Cho Y-J (2024) Revolutionizing respiratory health research: “commercially-available lung-on-a-chip and air-liquid interface systems”. Front. Lab. Chip. Technol. 3:1373029. doi: 10.3389/frlct.2024.1373029
Received: 19 January 2024; Accepted: 22 May 2024;
Published: 11 June 2024.
Edited by:
Lydia Sohn, University of California, Berkeley, United StatesReviewed by:
Andreas Stucki, PETA Science Consortium International e.V., GermanyCopyright © 2024 Park, Woo and Cho. This is an open-access article distributed under the terms of the Creative Commons Attribution License (CC BY). The use, distribution or reproduction in other forums is permitted, provided the original author(s) and the copyright owner(s) are credited and that the original publication in this journal is cited, in accordance with accepted academic practice. No use, distribution or reproduction is permitted which does not comply with these terms.
*Correspondence: Chang Gyu Woo, d29vQGtvcmVhdGVjaC5hYy5rcg==; Young-Jae Cho, bHVuZ2RyY2hvQHNudS5hYy5rcg==