- School of Engineering, Department of Biomedical Engineering, University of Glasgow, Glasgow, United Kingdom
1 Introduction
Every year, bench scientists in the biomedical and agricultural fields consume approximately 1,000 kg of plastic (Urbina et al., 2015), 30 times the average person in Europe (Eurostat, 2018). The climate crisis has urged laboratories to strive to improve sustainability practices and programs such as ‘My Green Lab’ (Green Lab Certification, 2013) and “Lab Efficiency Assessment Framework” (LEAF) (LEAF, 2018) are supporting laboratory changes through education, resources, and certifications. Lab-on-a-chip (LoC) technologies enable miniaturized laboratory analyses including drug screening, clinical diagnostics, and environmental and food safety monitoring. By their nature, they bring important advantages compared to macro-scale counterparts, including low sample volume and reagent consumption, rapid reactions, high throughput, and small dimensions allowing to use less material for manufacturing and providing portability (Agrawal et al., 2022). However, most commercially available microfluidic devices comprise non-biodegradable plastics with significant carbon dioxide equivalent (CO2-eq) (Eurostat, 2014) footprints. More than half of the published diagnostic LoC devices are made of polydimethylsiloxane (PDMS), preventing scaling and efficient production (Ongaro et al., 2022). Their portability has the potential to facilitate their use in remote areas, outside laboratory settings, raising concerns around health and environmental risks due to impractical, unavailable, or challenging waste disposal regulations and practices. Recent studies have investigated possible sustainable materials and reagent alternatives (Agrawal et al., 2022; Ongaro et al., 2022), however the environmental burden of LoC devices throughout their life cycle remains largely unexamined. Whilst substantial efforts in choosing LoC materials to reduce single-use non-biodegradable plastics are required to enable efficient scalability and reduce toxic chemicals, similarly, design and (bio)physical principles used to develop LoC need to be considered. Innovation in manufacturing technologies and the incorporation of life cycle assessments (LCA) from the outset of new LoC research can reduce downstream human health and environmental impacts (Visotsky et al., 2017). However, the lack of systematic studies on the non-green aspects of LoC systems limits our understanding and ability to improve their environmental performance effectively. Further research is needed to address this gap and provide guidance for the design and implementation of sustainable LoCs.
In this paper, I explore recent achievements around LoC sustainability and potential LoC life cycle sustainable improvements. I also propose that environmental impact analyses and sustainable materials should take center stage in new LoC research.
2 Environmental impact analysis
A product’s environmental hotspots can be identified using several tools (Table 1), but the most comprehensive is LCA (Luz et al., 2018). LCA quantifies potential impacts on the natural environment, human health, and resources during each step of the product life cycle (Caro, 2019). Since the LoC market is anticipated to grow by 20% year-on-year (Sridhar et al., 2022), there is an important need for guidelines to develop and dispose these technologies. The basis of a sustainable ‘self-assessment’ is incorporated into a ‘Design for Sustainability’ approach (Clark et al., 2009; Ceschin and Gaziulusoy, 2016) that can be integrated from the early stages of LoC design. These new initiatives could focus on reducing non-renewable energy and water consumption, waste production and harmful chemicals, and on developing longer-lasting designs (Ongaro et al., 2022). Such approaches, however, focus mainly on the consumable elements of LoCs (Agrawal et al., 2022; Ongaro et al., 2022), i.e., the materials and reagents involved, and their disposal. Therefore, LCA covering all cradle-to-grave aspects (Figure 1) should be advocated by the scientific community and encouraged by policymakers. Usually, LCA assesses products’ effects after their design and implementation, but embedding LCA into the initial stage of product design can significantly enhance the overall product’s sustainability (Visotsky et al., 2017). Luz et al. (2018) have recently proposed a novel approach to integrate LCA into the product development process, which can enhance the strategic planning of activities.
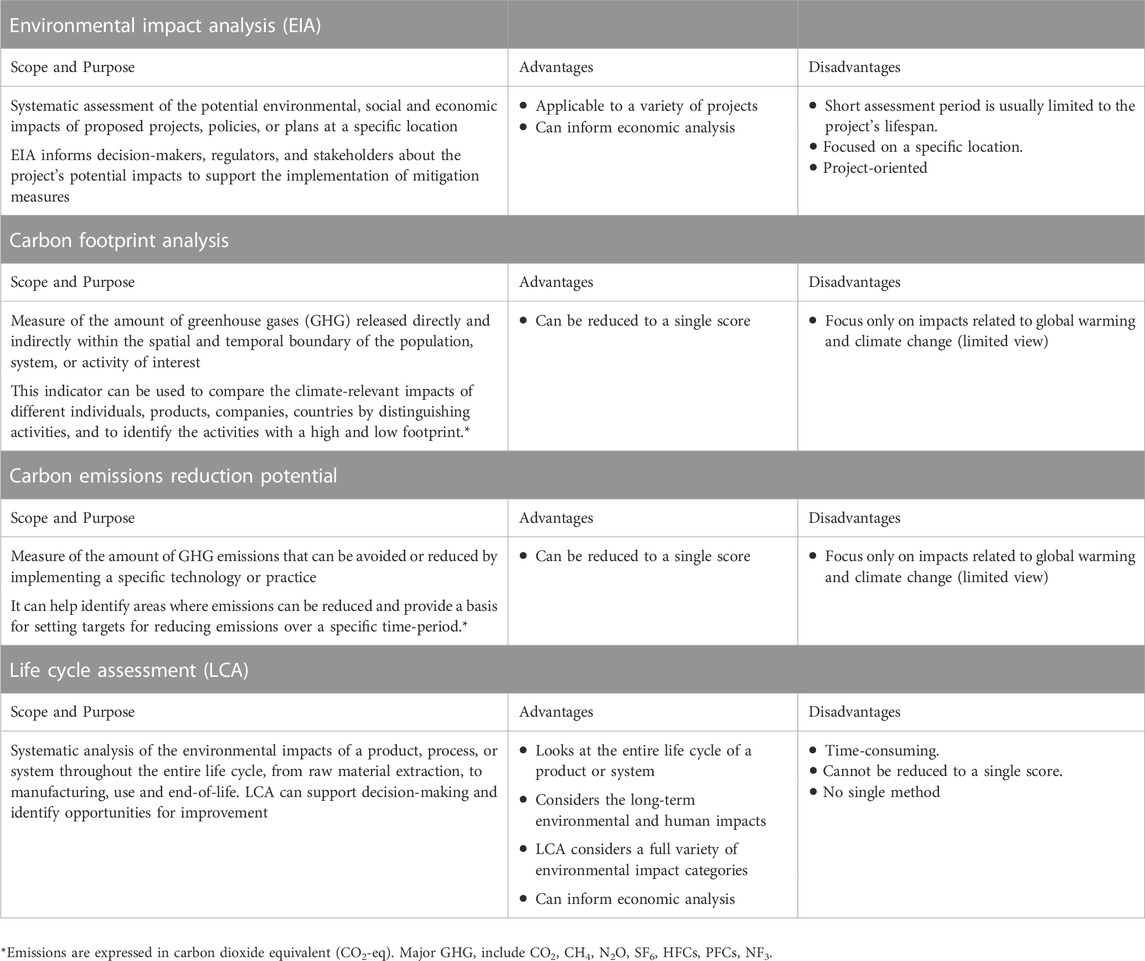
TABLE 1. Summary of environmental analysis tools, including environmental impact analysis (Wackernagel and Rees, 1996), carbon footprint analysis (ISO, 2018), carbon emissions reduction potential (Yang, 2010) and LCA (ISO, 2006a; 2006b). LCA offers a global view of the product’s impacts on the natural environment, human health and resource depletion, and is the most powerful tool to support decision-makers (Luz et al., 2018; Torabi and Ahmadi, 2020).
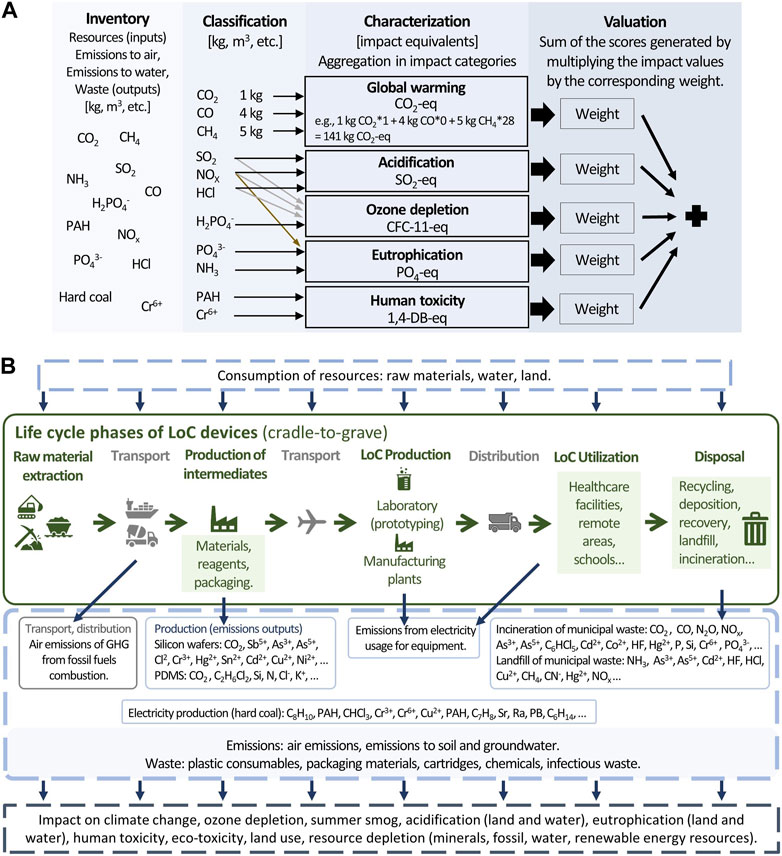
FIGURE 1. (A). Diagram of the stages of life cycle inventory and impact assessment in a LCA. LCA identifies the inputs and outputs of material flows during each step of the product life cycle, including the manufacturing processes, distribution, consumption, and waste management practices (Caro, 2019). Each input and output is assigned to impact categories, and the magnitude of the impacts is obtained via characterisation factors (Guinée, 2002; Thinkstep). (B). Schematic of the life cycle of LoC devices (European Commission, 2010) and some examples of emissions generated during each phase.
3 Sustainable consumables for LoC
3.1 Current materials and possible alternatives
Following the advent of microelectronics in the 1960s, the first substrate used for fabrication of LoCs was silicon (Foret et al., 2013). To circumvent the hazardous chemicals involved during fabrication and fulfil the high demand for low-cost devices, several polymers, including PDMS and poly (methyl methacrylate) (PMMA) have been used for their simple prototyping. Most commercially-available microfluidic devices are currently composed of these (and others) non-biodegradable plastics that have a large CO2-eq footprints (Ongaro et al., 2022). In the past decade, a variety of sustainable alternatives have been proposed (Keçili and Hussain, 2021; Damiati et al., 2022; Ongaro et al., 2022), but more research on how designs could be modified to accommodate different material strategies should take place.
Bio-derived and biodegradable (B&B) polymers such as polylactide (PLA) (Bettinger and Borenstein, 2010; Wang et al., 2010; Ongaro et al., 2020; 2018), polyDL-lactic-co-glycolide (PLGA) (Domachuk et al., 2010), zein (maize proteins) (Hsiao and Luecha, 2011; Luecha et al., 2011), shellac (Lausecker et al., 2016), and silk fibroin (Bettinger et al., 2007), have been successfully used both in biomedical and environmental applications. Other low-cost and biodegradable materials, such as paper and cotton, have been extensively used for detection of bioanalytes and pathogens (Agustini et al., 2021). Gelatin has also been used as a bio-based and biodegradable microfluidic platform in cell culture (Paguirigan and Beebe, 2006) and, most recently, as casing for lateral flow strips in a water-dispersible SARS-CoV-2 antigen test (Okos diagnostics, 2020). Interestingly, wood has been introduced in microfabrication processes, but some limitations around mechanical and chemical properties still need to be addressed (Ongaro et al., 2022).
3.2 Chemical and waste reduction
The impact of LoC does not simply arise from substrates, casing and cartridges. Greener options for solvents, like chloroform or acetone, and reagents, should be used in the future (Keçili and Hussain, 2021; Agrawal et al., 2022; Ongaro et al., 2022). For chemical analysis, green solvents include ionic liquids, deep eutectic solvents, and supercritical fluids (Keçili and Hussain, 2021; Agrawal et al., 2022). Clinical diagnostic assays often require chaotropic reagents, highly toxic to humans and marine life, with specific, high CO2-eq disposal procedures. Promising new methods have recently been introduced at a macroscale level in laboratory settings, e.g., by Merck (Merk), or integrated into microfluidic platforms for DNA extraction from E. coli or whole blood (Yoza et al., 2002; Nakagawa et al., 2005; Hagan et al., 2009).
A significant benefit of LoCs is the reduction in sample and reagents volumes, but also packaging material, compared to equivalent macroscale analytical processes. Importantly, LoCs allow automation, and it is critical to increase access to such capability so that researchers and users are able to harness benefits arising from increased reproducibility and lower number of analyses required in optimization processes (Keçili and Hussain, 2021).
4 Beyond consumables for LoC
4.1 Environmental considerations concerning bio-based and bioderived plastics, and fabrication methods
Using materials that are recycled or derived from biological sources for single-use LoCs can help to reduce CO2-eq emissions and plastic pollution at the point of use. However, material selection should also consider how the material is produced and disposed. For example, while B&B polymers, such as PLA, are praised substitutes for regular plastics, their production competes with food production, they require specific conditions for degradation, and generate methane gas during composting (Atiwesh et al., 2021). The same applies to cotton, whose crops are water-intensive and often use fertilizers with ecotoxicological effects (Naderi Mahdei et al., 2022). Consequently, not all the suggested alternatives are necessarily better for the environment than conventional plastics at all stages of their life cycle, Supplementary Materials (Walker and Rothman, 2020; Naser et al., 2021; Rosenboom et al., 2022). Moreover, fabrication processes, such as photolithography, laser printing, wax printing, hot embossing, and plasma oxidation (Ongaro et al., 2022; Sridhar et al., 2022), make use of chemicals and energy sources that contribute to the overall environmental impact of LoCs. For example, if gelatin represents a natural and biodegradable alternative to plastics, gelatin devices are often generated using photolithography (Paguirigan and Beebe, 2006), which uses chemicals that are toxic, carcinogenic, or derived from non-renewable sources (Mullen and Morris, 2021).
Substrates’ impact during the whole life cycle should be part of the design process before integrating specific materials in LoCs. Nonetheless, this task is complex and, importantly, not integrated in the training of LoC scientists or in their collaborative networks. There is therefore a urgent need to identify and compare the environmental hotspots of LoC substrates, and specific fabrication methodologies through LCA.
4.2 IT and digitalization
The integration of cloud computing and artificial intelligence potentiates LoC functionality, usability, and performance, particularly outside centralized laboratory facilities. For example, for healthcare applications, mobile technologies can support local decision making, offer guidelines for treatment, and accelerate the implementation of surveillance programs and global health policies (Fleming et al., 2021; Guo et al., 2021). Digitally connected LoCs can minimize the need for transportation, infrastructure, and logistics commonly associated with transporting individuals or specialized personnel (doctors, technicians) to centralized facilities (Ongaro et al., 2022). This potentially reduces the greenhouse gases emissions of LoC significantly. In the future, the advancement of wearable devices for continuous monitoring of biosignals will further reduce transport-related emissions.
Nevertheless, while mobile solutions are a key part of our emissions management strategy to achieve the United Nations Sustainable Development Goals (UN, 2015; Fleming et al., 2021), internet and digital tools have a significant environmental footprint, as they require precious/rare metals for production, consume large amounts of energy for the operation of data centers, and generate e-waste that is not properly recycled (UNEP, 2022). Therefore, the environmental impact of digital platforms, challenges around security, and the interoperability of data generated by LoC analysis should be key considerations. It is essential to encourage service providers, users, and policymakers to adopt more sustainable practices for their digital platforms.
4.3 Laboratory assessment frameworks
Research laboratories, where LoC devices are first conceived, are energy intensive and produce high levels of chemical and/or hazardous waste, when compared to regular office buildings (Lopez et al., 2017). This has prompted local actions and the creation of certification programs to incentivize greener practices, such as minimizing waste, optimizing energy use, choosing greener alternatives for reagents and consumables, and optimizing people management and research quality.
A recent report efficiently grouped all the sustainable initiatives that have been embraced by different research institutes (Winter et al., 2023), in an effort to increase awareness and education. Accreditation programs like LEAF and My Green Lab have boosted the implementation of these sustainable practices and created positive feedback loops and multiplying effects, that could reach also higher levels of society (Winter et al., 2023). Researchers should advocate institutional policies that support and incentivize sustainability, such as carbon budgeting, green procurement, and CO2-eq offsetting.
5 Discussion
Miniaturized LoC technologies have several advantages compared to their macroscale counterparts, stretching from reducing the amounts of sample and toxic chemicals, to minimization of packaging materials and better controlled energy transfer. However, the Lancet Commission for Pollution and Health reported that as many as one in six deaths worldwide since 2015 have arisen from a contaminated environment (with more than 90% of these deaths in low- and middle-income countries, which have borne the greatest economic and social burden (Fuller et al., 2022)). If, as a community, we want to increase access to chemical analysis and diagnostics to promote efficiency and reduce mortality and morbidity/illness, then we need to commit to a holistic approach for the reduction of LoC environmental impacts. Advocating for policy changes could speed up the shift to a low-carbon economy by promoting environmental practices in companies, through incentives like subsidies, clear emissions reduction goals that companies must meet, and strong national waste management infrastructures. Promoting sustainable informed choices can be institutionally supported, as illustrated by the European Union digital “product passport” (CIRPASS, 2023) that will make available to businesses, governments and consumers the information on a product’s origin, durability, composition, environmental and carbon footprint, reuse, repair and dismantling possibilities, and end-of-life handling.
The possibility of creating a circular economy to minimize raw materials use in LoCs is still a way away, but it would be a game-changer for waste management in the sector (Ongaro et al., 2022). As scientists, our mission includes preventing unnecessary deaths and creating a better planet, not just for ourselves but for future generations and for all creatures and life. To do this, we need to stimulate conversation and persist until sustainability becomes an integral attribute in the delivery of green products.
Author contributions
GC conceptualized and wrote the manuscript.
Funding
GC acknowledges support from Engineering and Physical Sciences Research Council (EPSRC) studentship EP/R513222/1.
Acknowledgments
The author thanks Dr Julien Reboud and Prof. Jonathan M. Cooper, University of Glasgow, for their help in editing.
Conflict of interest
The author declares that the research was conducted in the absence of any commercial or financial relationships that could be construed as a potential conflict of interest.
Publisher’s note
All claims expressed in this article are solely those of the authors and do not necessarily represent those of their affiliated organizations, or those of the publisher, the editors and the reviewers. Any product that may be evaluated in this article, or claim that may be made by its manufacturer, is not guaranteed or endorsed by the publisher.
Supplementary material
The Supplementary Material for this article can be found online at: https://www.frontiersin.org/articles/10.3389/frlct.2023.1239134/full#supplementary-material
SUPPLEMENTARY MATERIAL S1 | Examples of studies that compare different textile materials and plastics under different aspects, in terms of global warming potential impact category in kg CO2-equivalents.
References
Agrawal, A., Yıldız, Ü. Y., Hussain, C. G., Kailasa, S. K., Keçili, R., and Hussain, C. M. (2022). Greenness of lab-on-a-chip devices for analytical processes: advances & future prospects. J. Pharm. Biomed. Analysis 219, 114914. doi:10.1016/j.jpba.2022.114914
Agustini, D., Caetano, F. R., Quero, R. F., Fracassi Da Silva, J. A., Bergamini, M. F., Marcolino-Junior, L. H., et al. (2021). Microfluidic devices based on textile threads for analytical applications: state of the art and prospects. Anal. Methods 13, 4830–4857. doi:10.1039/D1AY01337H
Atiwesh, G., Mikhael, A., Parrish, C. C., Banoub, J., and Le, T.-A. T. (2021). Environmental impact of bioplastic use: A review. Heliyon 7, e07918. doi:10.1016/j.heliyon.2021.e07918
Bettinger, C. J., and Borenstein, J. T. (2010). Biomaterials-based microfluidics for engineered tissue constructs. Soft Matter 6, 4999. doi:10.1039/c0sm00247j
Bettinger, C. J., Cyr, K. M., Matsumoto, A., Langer, R., Borenstein, J. T., and Kaplan, D. L. (2007). Silk fibroin microfluidic devices. Adv. Mater. 19, 2847–2850. doi:10.1002/adma.200602487
Caro, D. (2019). “Carbon footprint,” in Encyclopedia of Ecology (Amsterdam, Netherlands: Elsevier), 252–257.
Ceschin, F., and Gaziulusoy, I. (2016). Evolution of design for sustainability: from product design to design for system innovations and transitions. Des. Stud. 47, 118–163. doi:10.1016/j.destud.2016.09.002
CIRPASS (2023). Ecosystem digital product passport (CIRPASS) prepares the ground for gradual piloting and deployment of the digital product passports (DPPs). Available at: https://cirpassproject.eu/(accessed 13, June, 23).
Clark, G., Kosoris, J., Hong, L., and Crul, M. (2009). Design for sustainability: current Trends in sustainable product design and development. Sustainability 1, 409–424. doi:10.3390/su1030409
Damiati, L. A., El-Yaagoubi, M., Damiati, S. A., Kodzius, R., Sefat, F., and Damiati, S. (2022). Role of polymers in microfluidic devices. Polymers 14, 5132. doi:10.3390/polym14235132
Domachuk, P., Tsioris, K., Omenetto, F. G., and Kaplan, D. L. (2010). Bio-microfluidics: biomaterials and Biomimetic designs. Adv. Mater. 22, 249–260. doi:10.1002/adma.200900821
European Commission, J. R. C. (2010). International Reference life cycle data system (ILCD) Handbook:general guide for life cycle assessment: Detailed guidance. First Edition. LU: Publications Office.
Eurostat, E. E. A. (2014). Glossary: carbon dioxide equivalent. Available at: https://ec.europa.eu/eurostat/statistics-explained/index.php?title=Glossary:Carbon_dioxide_equivalent (accessed 15, June, 23).
Eurostat (2018). How much plastic packaging waste do you produce? Available at: https://ec.europa.eu/eurostat/web/products-eurostat-news/-/edn-20180422-1 (accessed 13, June, 23).
Fleming, K. A., Horton, S., Wilson, M. L., Atun, R., DeStigter, K., Flanigan, J., et al. (2021). The Lancet Commission on diagnostics: transforming access to diagnostics. Lancet 398, 1997–2050. doi:10.1016/S0140-6736(21)00673-5
Foret, F., Smejkal, P., and Macka, M. (2013). “Miniaturization and microfluidics,” in Liquid Chromatography (Elsevier), 453–467. doi:10.1016/B978-0-12-415807-8.00020-1
Fuller, R., Landrigan, P. J., Balakrishnan, K., Bathan, G., Bose-O’Reilly, S., Brauer, M., et al. (2022). Pollution and health: A progress update. Lancet Planet. Health 6, e535–e547. doi:10.1016/S2542-5196(22)00090-0
Green Lab Certification, My (2013). My green lab certification. Available at: https://www.mygreenlab.org/green-lab-certification.html (accessed 4, June, 23).
J. B. Guinée (Editor) (2002). Handbook on life cycle assessment: Operational guide to the ISO standards, Eco-efficiency in industry and science (Dordrecht ; Boston: Kluwer Academic Publishers).
Guo, X., Khalid, M. A., Domingos, I., Michala, A. L., Adriko, M., Rowel, C., et al. (2021). Smartphone-based DNA diagnostics for malaria detection using deep learning for local decision support and blockchain technology for security. Nat. Electron. 4, 615–624. doi:10.1038/s41928-021-00612-x
Hagan, K. A., Meier, W. L., Ferrance, J. P., and Landers, J. P. (2009). Chitosan-Coated Silica as a Solid phase for RNA Purification in a microfluidic device. Anal. Chem. 81, 5249–5256. doi:10.1021/ac900820z
Hsiao, A., Luecha, J., Kokini, J., and Liu, L. (2011). Green microfluidics made of corn proteins. Annu. Int. Conf. IEEE Eng. Med. Biol. Soc. IEEE Eng. Med. Biol. Soc. Annu. Int. Conf. 2011, 8400–8403. doi:10.1109/IEMBS.2011.6092072
ISO (2006a). Environmental management — life cycle assessment — principles and framework. Available at: https://www.iso.org/standard/37456.html.
ISO (2006b). Environmental management — life cycle assessment — Requirements and guidelines. Available at: https://www.iso.org/standard/38498.html.
ISO (2018). Greenhouse gases — carbon footprint of products — Requirements and guidelines for quantification. Available at: https://www.iso.org/obp/ui/#iso:std:iso:14067.
Keçili, R., and Hussain, C. M. (2021). Green micro total analysis systems (GμTAS) for environmental samples. Trends Environ. Anal. Chem. 31, e00128. doi:10.1016/j.teac.2021.e00128
Lausecker, R., Badilita, V., Gleißner, U., and Wallrabe, U. (2016). Introducing natural thermoplastic shellac to microfluidics: A green fabrication method for point-of-care devices. Biomicrofluidics 10, 044101. doi:10.1063/1.4955062
LEAF (2018). Leaf - laboratory efficiency assessment framework. Available at: https://www.ucl.ac.uk/sustainable/leaf-laboratory-efficiency-assessment-framework (accessed 4, June, 23).
Lopez, J. B., Jackson, D., Gammie, A., and Badrick, T. (2017). Reducing the environmental impact of clinical laboratories. Clin. Biochem. Rev. 38, 3–11.
Luecha, J., Hsiao, A., Brodsky, S., Liu, G. L., and Kokini, J. L. (2011). Green microfluidic devices made of corn proteins. Lab. Chip 11, 3419. doi:10.1039/c1lc20726a
Luz, L. M. D., Francisco, A. C. D., Piekarski, C. M., and Salvador, R. (2018). Integrating life cycle assessment in the product development process: A methodological approach. J. Clean. Prod. 193, 28–42. doi:10.1016/j.jclepro.2018.05.022
Merk Single Spin DNA and RNA Purification Kits for green labs. Available at: https://www.sigmaaldrich.com/GB/en/technical-documents/technical-article/protein-biology/protein-purification/genelute-e-sustainability (accessed 28, April, 23).
Mullen, E., and Morris, M. A. (2021). Green Nanofabrication Opportunities in the Semiconductor industry: A life cycle Perspective. Nanomaterials 11, 1085. doi:10.3390/nano11051085
Naderi Mahdei, K., Esfahani, S. M. J., Lebailly, P., Dogot, T., Van Passel, S., and Azadi, H. (2022). Environmental impact assessment and efficiency of cotton: the case of Northeast Iran. Environ. Dev. Sustain. doi:10.1007/s10668-022-02490-5
Nakagawa, T., Tanaka, T., Niwa, D., Osaka, T., Takeyama, H., and Matsunaga, T. (2005). Fabrication of amino silane-coated microchip for DNA extraction from whole blood. J. Biotechnol. 116, 105–111. doi:10.1016/j.jbiotec.2004.08.018
Naser, A. Z., Deiab, I., and Darras, B. M. (2021). Poly(lactic acid) (PLA) and polyhydroxyalkanoates (PHAs), green alternatives to petroleum-based plastics: A review. RSC Adv. 11, 17151–17196. doi:10.1039/D1RA02390J
Okos diagnostics (2020). Gelassette. Available at: https://www.okosdiagnostics.com/(accessed 4, April, 23).
Ongaro, A. E., Di Giuseppe, D., Kermanizadeh, A., Miguelez Crespo, A., Mencattini, A., Ghibelli, L., et al. (2020). Polylactic is a sustainable, low Absorption, low Autofluorescence alternative to other plastics for microfluidic and Organ-on-chip applications. Anal. Chem. 92, 6693–6701. doi:10.1021/acs.analchem.0c00651
Ongaro, A. E., Keraite, I., Liga, A., Conoscenti, G., Coles, S., Schulze, H., et al. (2018). Laser Ablation of poly(lactic acid) Sheets for the rapid Prototyping of sustainable, single-use, disposable Medical Microcomponents. ACS Sustain. Chem. Eng. 6, 4899–4908. doi:10.1021/acssuschemeng.7b04348
Ongaro, A. E., Ndlovu, Z., Sollier, E., Otieno, C., Ondoa, P., Street, A., et al. (2022). Engineering a sustainable future for point-of-care diagnostics and single-use microfluidic devices. Lab. Chip 22, 3122–3137. doi:10.1039/D2LC00380E
Paguirigan, A., and Beebe, D. J. (2006). Gelatin based microfluidic devices for cell culture. Lab. Chip 6, 407. doi:10.1039/b517524k
Rosenboom, J.-G., Langer, R., and Traverso, G. (2022). Bioplastics for a circular economy. Nat. Rev. Mater. 7, 117–137. doi:10.1038/s41578-021-00407-8
Sridhar, A., Kapoor, A., Kumar, P. S., Ponnuchamy, M., Sivasamy, B., and Vo, D.-V. N. (2022). Lab-on-a-chip technologies for food safety, processing, and packaging applications: A review. Environ. Chem. Lett. 20, 901–927. doi:10.1007/s10311-021-01342-4
Thinkstep (2012). GaBi Paper Clip Tutorial Part 1: introduction to LCA and modelling using GaBi. Available at: https://sphera.com/product-sustainability-software/ (Accessed April 20, 2023).
Torabi, F., and Ahmadi, P. (2020). “Battery technologies,” in Simulation of Battery systems (Elsevier), 1–54. doi:10.1016/B978-0-12-816212-5.00005-2
UN (2015). Sustainable development goals (SDGs). Available at: https://sdgs.un.org/goals (accessed 4, April, 23).
UNEP (2022). The growing footprint of digitalisation. Available at: https://www.unep.org/resources/emerging-issues/growing-footprint-digitalisation (accessed 5, March, 23).
Urbina, M. A., Watts, A. J. R., and Reardon, E. E. (2015). Labs should cut plastic waste too. Nature 528, 479. doi:10.1038/528479c
Visotsky, D., Patel, A., and Summers, J. (2017). Using design Requirements for environmental assessment of products: A Historical based method. Procedia CIRP 61, 69–74. doi:10.1016/j.procir.2016.11.149
Wackernagel, M., and Rees, W. E. (1996). Our ecological footprint: Reducing human impact on the earth, new catalyst bioregional series. Gabriola Island: New Society Publishers. Philadelphia, PA.
Walker, S., and Rothman, R. (2020). Life cycle assessment of bio-based and fossil-based plastic: A review. J. Clean. Prod. 261, 121158. doi:10.1016/j.jclepro.2020.121158
Wang, J., Bettinger, C. J., Langer, R. S., and Borenstein, J. T. (2010). Biodegradable microfluidic scaffolds for tissue engineering from amino alcohol-based poly(ester amide) elastomers. Organogenesis 6, 212–216. doi:10.4161/org.6.4.12909
Winter, N., Marchand, R., Lehmann, C., Nehlin, L., Trapannone, R., Rokvić, D., et al. (2023). The paradox of the life sciences: how to address climate change in the lab: how to address climate change in the lab. EMBO Rep. 24, e56683. doi:10.15252/embr.202256683
Yang, H. (2010). Carbon efficiency, carbon reduction potential, and economic development in the People’s Republic of China: A Total factor production model. China: Asian Dev. Bank.
Keywords: lab-on-a-chip, sustainability, lab efficiency assessment framework, my green lab, life cycle assessment, environmental impact analysis
Citation: Core G (2023) Lab-on-a-chip—fostering a sustainable future. Front. Lab. Chip. Technol. 2:1239134. doi: 10.3389/frlct.2023.1239134
Received: 12 June 2023; Accepted: 14 August 2023;
Published: 28 August 2023.
Edited by:
Yiqiang Fan, Beijing University of Chemical Technology, ChinaReviewed by:
Guoxia Zheng, Dalian University, ChinaVigneswaran Narayanamurthy, Technical University of Malaysia Malacca, Malaysia
Copyright © 2023 Core. This is an open-access article distributed under the terms of the Creative Commons Attribution License (CC BY). The use, distribution or reproduction in other forums is permitted, provided the original author(s) and the copyright owner(s) are credited and that the original publication in this journal is cited, in accordance with accepted academic practice. No use, distribution or reproduction is permitted which does not comply with these terms.
*Correspondence: Giulia Core, Zy5jb3JlLjFAcmVzZWFyY2guZ2xhLmFjLnVr