- Department of Mechanical, Industrial and Systems Engineering, University of Rhode Island, Kingston, RI, United States
Effective environmental monitoring has become a worldwide concern, requiring the development of novel tools to deal with pollution risks and manage natural resources. However, a majority of current assessment methods are still costly and labor-intensive. Thanks to the rapid advancements in microfluidic technology over the past few decades, great efforts have been made to develop miniaturized tools for rapid and efficient environmental monitoring. Compared to traditional large-scale devices, microfluidic approaches provide several advantages such as low sample and energy consumption, shortened analysis time and adaptabilities to onsite applications. More importantly, it provides a low-cost solution for onsite environmental assessment leveraging the ubiquitous materials such as paper and plastics, and cost-effective fabrication methods such as inkjet printing and drawing. At present, devices that are disposable, reproducible, and capable of mass production have been developed and manufactured for a wide spectrum of applications related to environmental monitoring. This review summarizes the recent advances of low-cost microfluidics in the field of environmental monitoring. Initially, common low-cost materials and fabrication technologies are introduced, providing a perspective on the currently available low-cost microfluidic manufacturing techniques. The latest applications towards effective environmental monitoring and assessment in water quality, air quality, soil nutrients, microorganisms, and other applications are then reviewed. Finally, current challenges on materials and fabrication technologies and research opportunities are discussed to inspire future innovations.
1 Introduction
Environmental pollution has continuously been a major threat due to fast-growing anthropogenic activities resulting from civilization and industrialization (Xu et al., 2018; Lau et al., 2020; Podgorski and Berg, 2020; Santos et al., 2021). Associated burden of diseases and death arising from global air and water pollution poses a great challenge on public health, especially in underdeveloped regions and countries (Evans et al., 2014; Mahaqi et al., 2021; Yang et al., 2022). For instance, more than four millions of deaths related with gastrointestinal diseases may be attributed to water contamination in the United States (Colford et al., 2006). The contaminated water, if used for irrigation, can also induce food safety issues involving bacterial contamination (Hamilton et al., 2006). The pollution of oxides of nitrogen (NOx) was also found to play an important role in respiratory problems among children and adults in Nigeria (Komolafe et al., 2014). Other pollutants such as waterborne pathogens, heavy metals, and toxic gases from industrial disposal effluents are also major contributors to global water pollution (Yew et al., 2019). The existing evidence clearly speaks out the necessity of accurate pollution risk assessment for tracking pollution sources, determining long-term trends of pollution, and developing effective treatment methods. In particular, it is essential to conduct quantitative assessment on potential pollutants of various types of pollution (e.g., air, water and land pollution) (Pol et al., 2017).
Conventionally, the assessment of pollutants is carried out in centralized laboratories following the collection of samples (Ritchie et al., 2003). Indeed, these measurements could provide accurate and critical information about the pollutants. However, the use of bulky equipment makes them not adaptable to in situ and real-time assessment, thus hindering a universal and rapid environmental monitoring (Pena-Pereira et al., 2021). One promising solution to address this downside is the development of miniaturized and potentially field-deployable analytical tools using microfluidic technologies (Dhar and Lee, 2018). Thanks to the miniaturization of the fluid domain, microfluidics offers several unique advantages such as low sample consumption, high surface-to-volume ratio, and powerful fluid/particle manipulation abilities (McNeely et al., 1999; Zhu and Fang, 2013; Gao et al., 2020). However, as a technology benefiting from microelectromechanical systems (MEMS) microfabrication techniques, traditional microfluidic devices built on glass or silicon require complicated fabrication processes involving costly chemicals, materials, equipment, and trained personnel (Rai-Choudhury, 1997; Mao and Huang, 2012; Moreau, 2012; Song et al., 2018; Lin et al., 2020a). Moreover, a majority of microfluidic devices still do not bypass the requirements of external equipment and/or components (e.g., syringe pumps, heaters, valves, and others) to realize various functions (Lin et al., 2019a). As a result, the use of microfluidics, to a large extent, is limited in research and laboratories. In order to reduce the cost and minimize the dependency on external instrumentations, low-cost microfluidic devices made from cheap and ubiquitous materials received extensive attentions for various applications in the past decade (Faustino et al., 2016; Fan, 2018).
Recent research publications have indicated a constant growth in the field of low-cost microfluidics (Fan, 2018), as evidenced by the increasing number of relevant articles found on Google Scholar (Figure 1) using the keyword “low-cost microfluidics”. In particular, great efforts have been made to develop novel low-cost microfluidic devices by exploring various low-cost materials and fabrication techniques. For example, wax printing was applied on filter papers to create paper devices (Lin et al., 2016). Cloth was also applied because of the potentials to develop wearable sensors (Zhang et al., 2020). With advancements in 3D printing technologies, multi-layered microfluidic channels with complicated designs became achievable, which also opened new opportunities in various applications including environmental monitoring and assessment (Bhattacharjee et al., 2016; Yazdi et al., 2016).
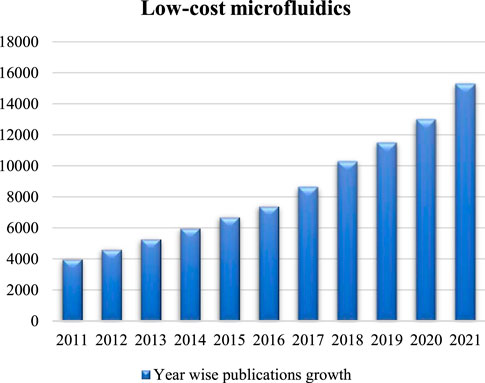
FIGURE 1. Year wise publications growth in regard to low-cost microfluidics from 2011 to 2021. Data were collected using Google Scholar.
Note that the low-cost feature highlighted here is indeed not a rigorous description. It is largely dependent on how engineers, researchers and scientists define it. In this review, we refer the low-cost microfluidics to devices and systems manufactured outside of cleanroom with all associated fabrication tools and materials readily accessible to most research laboratories. This definition was used by previous researchers when discussing upon low-cost microfluidics in review articles (Faustino et al., 2016; Fan, 2018). Although these devices and systems may not provide similar performance compared to the cleanroom-based counterparts at current stages, they hold promise in the global dissemination of the state-of-the-art environmental monitoring achievements when accuracy is not as significant as the accessibility to the analytical analysis tools (Raj M and Chakraborty, 2020). For example, the availability of clean water in developing countries remains a challenge; the low-cost monitoring of contaminants in water such as heavy metals and infectious microorganisms provide direct benefits towards improving local public health. To achieve this goal, cellulose paper, a porous and ubiquitous material has been employed to build sensors to monitor the water quality (Bhattacharjee et al., 2016). The porous structure of this material enables passive capillary actions without external driving mechanisms (Nightingale et al., 2015), while its portable nature also benefits in situ measurements. Therefore, besides environmental monitoring, these devices are also useful for many other applications such as the point-of-care (POC) diagnostics (Volpatti and Yetisen, 2014; Almeida et al., 2018; Dhar and Lee, 2018; Manisha et al., 2018; Jaywant and Arif, 2019).
In this review, we will start with the primary advances in the underlying materials and fabrication methods of low-cost microfluidic devices. Indeed, several good review papers have been published previously discussing the fabrication technologies for low-cost microfluidics and other major topics (Tomazelli Coltro et al., 2014; He et al., 2015; Faustino et al., 2016; Almeida et al., 2018; Fan, 2018), however, as a promising tool for ongoing and future onsite environmental monitoring and assessment, a comprehensive review with this specific focus is still beneficial. Finally, latest applications on water, air, soil quality and many others were introduced, along with conclusions, insights, and future perspectives.
2 Low-cost materials and fabrication methods
Over the past decades, a variety of low-cost materials have been explored to create microfluidic devices beyond glass and silicon (Tomazelli Coltro et al., 2014; Chen et al., 2016; Raj M and Chakraborty, 2020). In 2007, the Whitesides group developed the first modern microfluidic paper-based analytical device (µPAD) (Martinez et al., 2007), by which glucose and protein assays were performed on a cellulose paper. The COVID-19 pandemic also necessitated the development and applications of low-cost analytical analysis tools (Adyel, 2020; Patrício Silva et al., 2021). For example, the Flowflex COVID-19 Antigen Home Test is built on top of a lateral flow chromatographic immunoassay, in which samples can be directly placed on the test device and the results are displayed on control and test lines in a few minutes (Boelle et al., 2022). Besides paper, plastics are important materials used in low-cost microfluidics and have been used as substrates or housing that protects major components. Polyethylene terephthalate (PET), a common thermoplastic polymer used to make bottles and packages, was used as a flexible substrate for various applications such as the single-cell trapping reported by our group (Lin et al., 2019a). Indeed, other materials such as cloth, elastomers and biomaterials are also good candidates (McMillan et al., 2020; Zhang et al., 2020; Tien and Dance, 2021) and will be discussed below.
Besides low-cost materials, selection of the most appropriate fabrication method is fundamental to reduce the overall cost of the final devices. So far, many fabrication technologies have been explored and developed in the field of microfluidics (Niculescu et al., 2021; Scott and Ali, 2021). Conventional fabrication methods such as photolithography, reactive-ion etching, electron-beam lithography, and LIGA (lithography, electroplating, and molding) often rely on sophisticated equipment and expensive materials, therefore not suitable for low-cost microfluidics (Gale et al., 2018). On the other hand, fabrication methods such as wax printing, 3D printing and even drawing only require minimal investment on the equipment and materials, which attracted a lot of attention nowadays (Tomazelli Coltro et al., 2014; Fan, 2018). In this section, low-cost microfluidic materials and fabrication methods (Figure 2) are summarized and discussed.
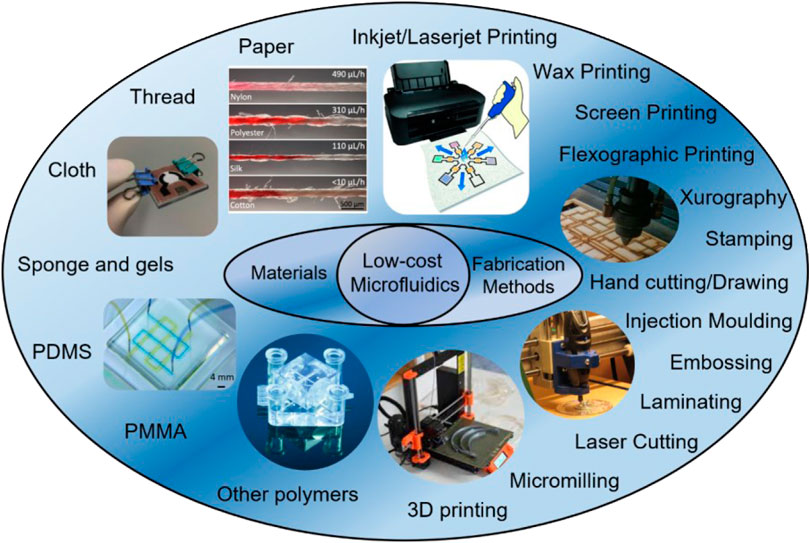
FIGURE 2. Summary of low-cost microfluidic materials and fabrication methods. Materials are showed in the left while fabrication methods are in the right. Commonly used low-cost materials are paper, thread, cloth, PDMS, and PMMA. Oftentimes used low-cost fabrication methods are 3D printing, micromilling, laser cutting, inkjet/laserjet printing, and xurography. Reproduced with permission from (Yamada et al., 2015), (Yang et al., 2017), (Au et al., 2016), (Jiang et al., 2020), (Rumaner et al., 2019).
2.1 Low-cost materials
At present, many low-cost materials have been explored to develop tools for environmental monitoring, including cellulose paper, thread, cloth and polymers (Gao et al., 2019a; Arroyo et al., 2020; Zhang et al., 2020; Zhou et al., 2021; Li et al., 2022). In fact, devices made from the first three materials have caught intensive attention and are often called paper-based analytical devices (µPADs), thread-based analytical devices (µTADs), and cloth-based analytical devices (µCADs), respectively. Polymeric materials such as polydimethylsiloxane (PDMS), polymethyl methacrylate (PMMA) and PET are also major players thanks to advantages in their mechanical properties, optical and thermal stabilities, as well as the versatility to different environmental applications (Fallahi et al., 2019; Nielsen et al., 2019).
2.1.1 Paper-based analytical devices (µPADs)
Paper is an inexpensive and ubiquitous resource that has been used in various applications for a long time (L Santana and Angela A Meireles, 2014; Kumar Gupta et al., 2019). Its properties (e.g., porosity, chemical composition, and wetting performance) are readily adjustable for different purposes (Glavan et al., 2013; Böhm et al., 2014; Gao et al., 2019b). Like other porous materials, the porous nature and high surface-to-volume ratio of the paper promote passive fluid driving and control. The fiber chemical composition (e.g., degree of polarity) can also be modified to enhance sample-paper interactions and plays a key role in device design and operation (Chitnis et al., 2011; Lim et al., 2019; Ma et al., 2019; Soum et al., 2019). Owing to a wide variety of paper types commercially available in the market, the correct property selection also saves time and labor for material treatments (Tomazelli Coltro et al., 2014; Xia et al., 2016). For example, nitrocellulose paper serves as a good substrate for covalent immobilization of the biomolecules due to the strong binding capability to proteins originated from the nitrate groups on their surfaces. Filter paper and chromatography paper can outperform other paper types in terms of uniform thickness and pore size (Tang et al., 2022).
Note that the paper material per se only provides the backbone of the devices, while analytical analysis taking place on papers is realized through incorporation of various sensing or detection methods (Adkins et al., 2015; Nishat et al., 2021). Existing detection methods can be categorized into several types including colorimetric, fluorescent, chemiluminescent, electrochemical, electro chemiluminescent and Raman sensing (Fu and Wang, 2018; Kaneta et al., 2019; Zheng et al., 2021a; Li et al., 2021). Review articles for in-depth discussions on advances in µPADs can be found in the following references: (Carrell et al., 2019; Kaneta et al., 2019; Lim et al., 2019; Soum et al., 2019).
2.1.2 Thread-based analytical devices(µTADs)
The µTADs are another successful application of porous materials for environmental monitoring and general analytical analysis (Agustini et al., 2016; Tan et al., 2021). Similar to µPADs, these devices are good candidates for low-cost applications. The existing industry worldwide also promotes the applications without complex material modifications (Farajikhah et al., 2019; Weng et al., 2019). Currently, a variety of threads are available for different applications, including natural (e.g., silk, wool, linen, etc.) and synthetic (e.g., polyester, polyether-polyurea, acrylic, etc.) threads (Oliveira et al., 2022). The flow characteristics and the detection methods employed in threads are similar to those employed in paper, since both are porous (Berthier et al., 2017; Tan et al., 2021). However, compared to paper devices, thread-based devices are more suitable for wearable applications since threads can be used to create clothing either by directly sewing, or having walls patterned onto cloth (Xiao et al., 2019; Tan et al., 2021; Xia et al., 2021).
The detection methods used in µTADs are similar to those used in µPADs. Conventional detection methods (e.g., fluorescence, electrochemical, Raman, etc.) are applicable to thread-based devices as well (Weng et al., 2019; Agustini et al., 2021). Moreover, distance and barcode-based detection are another two possible low-cost detection strategies (Tan et al., 2021). Distance-based detection relies on the fact that disparate wetting performances can be induced by different analytes for identification (Alsaeed and Mansour, 2020; Jarujamrus et al., 2020; Shimazu et al., 2022). Moreover, barcode detection can provide results of multiple analyte reactions (e.g., blood typing) that otherwise are difficult to achieve (Nilghaz et al., 2014). For a comprehensive review on thread devices, the readers are encouraged to read the suggested references: (Farajikhah et al., 2019; Weng et al., 2019; Tan et al., 2021; Xia et al., 2021).
2.1.3 Other porous materials
Similar to paper and thread, cloth also has a porous structure, thus most fabrication and analytical approaches used in the aforementioned porous materials can also be extended and exploited (Zheng et al., 2021b; Xu et al., 2021). Colorimetric method is the most popular method used in µCADs due to its simplicity and independence on external analysis tools (Bagherbaigi et al., 2014; Nilghaz et al., 2015; Li et al., 2018; Tasaengtong and Sameenoi, 2020). Electrochemical and chemiluminescence methods and their combination were explored as well (Jiang et al., 2020; Shang et al., 2020; Zheng et al., 2021b; Shang et al., 2022). Readers are encouraged to read more detailed review papers that summarizes fabrication, detection methods and performances of µCADs (Nilghaz et al., 2013; Zhang et al., 2020; Agustini et al., 2021). In addition, other low-cost materials have also been reported. For example, sponge was used for thedetection of heavy metal ions in environmental samples (Ding and Lisak, 2019), leveraging the strength of sponge structure and the coupling with other materials for better mechanical properties (Hu et al., 2022; Silva et al., 2022). A few examples of the applications of low-cost porous materials are shown in Figure 3.
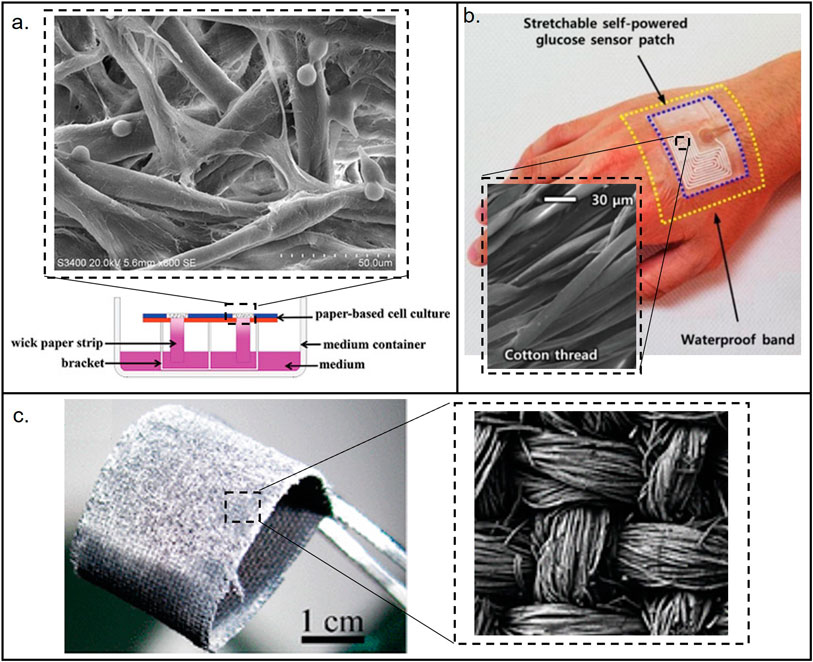
FIGURE 3. Materials used for the fabrication of low-cost porous (paper, thread, and cloth) microfluidic devices. (A) Paper-based device. Reproduced with permission from (Fu et al., 2021). (B) Thread-based device. Reproduced with permission from (Bae et al., 2022). (C) Cloth-based device. Reproduced with permission from (Wang et al., 2011). The images show the structural differences between the porous materials. Cotton and cloth have more organized structures than paper.
2.1.4 Polymers
Polymer is a type of material that consists of large molecules called repeating units (or mer) arranged in a periodic manner within the structure (Strobl and Strobl, 1997; Young and Lovell, 2011). Nature has generously provided us many polymeric materials such as wood and rubber (Carothers, 1936; Mark et al., 2004). The paper cellulose described above is indeed a type of polymer composed of glucose units (El Seoud and Heinze, 2005; Rose and Palkovits, 2011). Moreover, many synthetic polymers have been recently invented for various purposes (Hacker et al., 2019). For example, plastic is a large family of polymers, including polycarbonate (PC), polyethylene (PE), polypropylene (PP), polyethylene terephthalate (PETE or PET), polyvinyl chloride (PVC), acrylonitrile-butadiene-styrene (ABS) and many others (Young and Lovell, 2011; Maitz, 2015; Hacker et al., 2019). Oftentimes, to create microfluidic devices, the associated cost does not come from the materials themselves since they are cheap, instead, the fabrication methods such as photolithography that creates polymeric structures are responsible for the high cost (Chan et al., 2015; Faustino et al., 2016). In particular, PDMS is a popular polymeric material used in microfluidics (Haubert et al., 2006; Li et al., 2012; Raj M and Chakraborty, 2020). It offers several advantages over other materials such as cost-effectiveness, good biocompatibility and transparency, favorable elasticity and flexibility, inertness to chemicals and permeability to gases (Raj M and Chakraborty, 2020; Miranda et al., 2021). To create PDMS based devices, soft lithography has been considered as a gold standard. Specifically, a mold with desired pattern is created first, and then the PDMS mixture is poured onto the mold allowing the curing over time to create PDMS replicas with identical patterns. Though the method itself is low-cost, the molds are made from complex conventional photolithography, for which a cleanroom is indispensable (Tomazelli Coltro et al., 2014; Barocio et al., 2021). To reduce the cost and eliminate the needs of a cleanroom, other fabrication methods such as 3D printing and milling have been explored for mold manufacturing (Gale et al., 2018; Ruiz et al., 2020).
PMMA is another popular polymeric material used in microfluidics (Chen et al., 2019; Ma et al., 2020; Razavi Bazaz et al., 2020). As a thermoplastic, PMMA becomes pliable when heated up above the glass transition temperature. Therefore, similar to PDMS, PMMA devices can be made by molding, thus holding promise for mass production (Trotta et al., 2018; Ma et al., 2020). In addition, PMMA can be used as an UV-sensitive material on which the structures are created by the UV radiation (Fan et al., 2012). Thin plastic films such as the double sided tapes, PET films are also explored to create lab-on-a-foil devices (Focke et al., 2010; Bertana et al., 2018). Unlike the porous materials described above, the devices made on thin films are much similar to regular PDMS devices, on which microchannels can be created and active fluid and particle manipulation technologies can be integrated (Gale et al., 2018). Another important polymer that has been widely used nowadays are the photosensitive resins used in 3D printing techniques. Note that although traditional 3D printing resins possess good mechanical and physical properties, limitations still exist in terms of the molding performance if used as the molds and the biocompatibility for biology and medical purposes (Heuer et al., 2021). Other issues such as flow control issues, channel dimensional accuracy, solvent compatibility, surface roughness and low wettability are still the major concerns for broader applications (Gale et al., 2018; Mehta and Rath, 2021), though several studies have reported novel photopolymer formulations (resins) capable of potentially addressing these issues (Pranzo et al., 2018; Mehta and Rath, 2021). More comprehensive reviews of 3D printing materials for microfluidic devices can also help the readers understand the current status and future perspectives for this hot field (Gale et al., 2018; Weisgrab et al., 2019).
2.2 Low-cost fabrication methods
The cost associated with the development of microfluidic devices is not completely related with the material selection, the processing methodology used as fabrication method can modify the price dramatically. For instance, a PDMS microfluidic device fabricated under conventional photolithography shall have a different price than the same device fabricated using 3D printing (Au et al., 2016; Raj M and Chakraborty, 2020; Razavi Bazaz et al., 2020). In this section, we will summarize the low-cost fabrication methods and provide a perspective of their advantages and limitations. Figure 4 shows examples of materials used for low-cost devices and their associated fabrication methods.
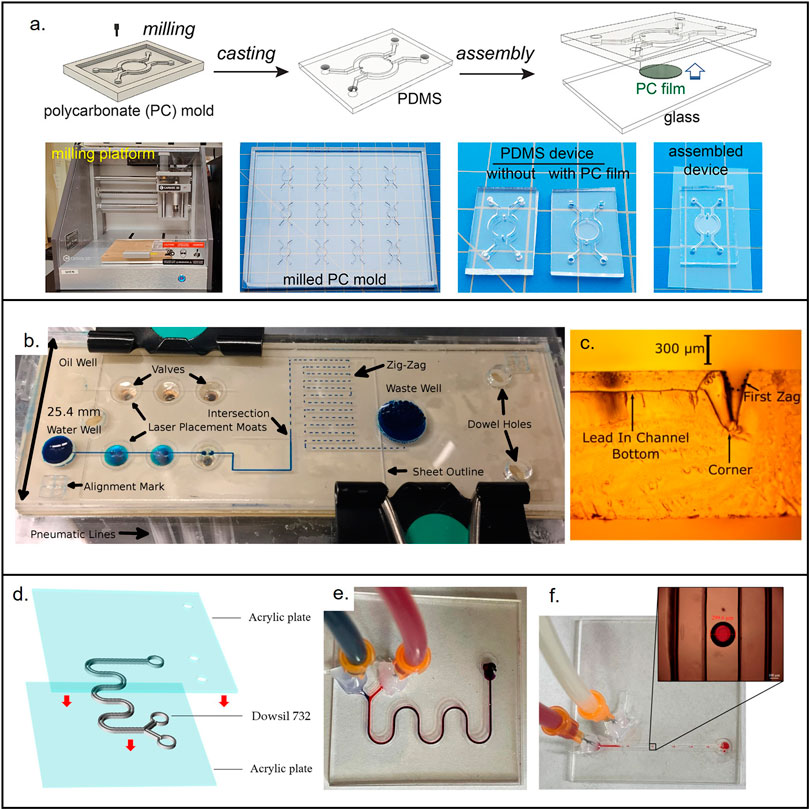
FIGURE 4. Materials used for the fabrication of low-cost non-porous microfluidic devices. (A) Microfluidic device made from PDMS casted in a micromilled mold. Reproduced with permission from (Oh et al., 2022). (B) PMMA micropump. Reproduced with permission from (Strike et al., 2018). (C) Cross section showing the laser (CO2) cut microchannel. Reproduced with permission from (Strike et al., 2018). (D) 3D printed device made out of novel resin (Dowsil 732) that enables end-use devices. Reproduced with permission from (Jin et al., 2022). (E) Micromixer used to test Dowsil 732. Reproduced with permission from (Jin et al., 2022). (F) Droplet generator used to test Dowsil 732. Reproduced with permission from (Jin et al., 2022).
2.2.1 Fabrication methods for porous materials
Since µPADs, µTADs and µCADs have similar structures, they do share similar fabrication approaches (Dou et al., 2015; Tan et al., 2021). One of the straightforward approaches is to cut paper into strips with desired dimensions, followed by loading essential reagents (Adkins et al., 2015; Akyazi et al., 2018; Weng et al., 2019). Here, the capillary action serves as the driving pump to spread samples from one end to another (Xie et al., 2019; Nishat et al., 2021). Hydrophobic fluid barriers can also be used to control the fluid flow in porous devices, turning a single piece of paper into a fluid managing platform (Soum et al., 2019; Liu et al., 2020; Liu et al., 2021). Different printers can be used to create hydrophobic walls that confine the fluid transport in between, most printers are simple, inexpensive, and suitable for large volume creation (mass production) (Tomazelli Coltro et al., 2014; Adkins et al., 2015). Inkjet printing is a popular printing fabrication technology, consisting of two main categories: powder based, or photopolymer based (Loo et al., 2019; Olmos et al., 2019; Aladese and Jeong, 2021; Khorsandi et al., 2021). Using this technique, hydrophobic inks are used to create the channel walls on paper-based devices (Fan et al., 2018; Lim et al., 2019; Wang et al., 2019). Note that inkjet printing is applicable for multiple types of paper, while laser printers provides rapid and large volume printing processes (Bamshad and Cho, 2021; Nishat et al., 2021).
In addition, flexographic printing has also been used for creating µPADs. This method provides a continuous nature of fabrication, which is critical in mass production (Caetano et al., 2018; Qian et al., 2022). The screen printing process has also been used yet it requires multiple steps and has low resolution (Morbioli et al., 2019; Soum et al., 2019; Dixon, 2020; Yehia et al., 2020). Moreover, wax screen printing is a technology that combines the advantages of wax printing and screen printing, offering a simple 2-step process at much lower costs than traditional wax printing technology (Morbioli et al., 2019). Wax printing has been widely used for creating channel walls in paper-based devices (Liu et al., 2015; Kamnoet et al., 2021), however, there are still some limitations for this technology such as the difficulty to create smaller size channels (Gale et al., 2018; Lin et al., 2022; Tesfaye and Hussen, 2022). The solvents may also soak into the wax and paper boundaries, thus compromising the functionality of the device (Szabo and Hess-Dunning, 2021; Chen et al., 2022a; Ruiz et al., 2022; Tran et al., 2022). Using stamps (ink imprinting) and pen writing (handwriting) are easy yet non-precise techniques to pattern 2D channels (Dornelas et al., 2015; Xia et al., 2016; Noviana et al., 2020). Plasma treatment can also be used to pattern channels using hand held corona treater (He et al., 2015; Zhu et al., 2016). The cross-sectional area of porous devices can be adjusted to control the flow motion (Soum et al., 2019; Modha et al., 2021), it is possible to cut paper and cloth with different inexpensive tools (i.e., scissors, razor blade) (Tomazelli Coltro et al., 2014; Xia et al., 2016). Those tools already exist in commercial versions, coupled to CNC machines and are able to execute a predefined cutting path based on the drawings (Gosset et al., 2018; Cortes-Medina et al., 2020; Guo et al., 2021). Similarly, xurography (digital craft cutter) can be used to cut other materials (e.g., polymeric sheet), as long as the material thickness is small (Speller et al., 2019; Caffiyar et al., 2020; Guo et al., 2021).
2.2.2 3D printing
3D printing technology has proven to be a cost-effective method for prototyping and engineering studies (Mehta and Rath, 2021; Jin et al., 2022). With the improvements of 3D printers, filaments and CAD technologies, 3D printing has emerged as a great tool to create microfluidic devices (Bhattacharjee et al., 2016; Gale et al., 2018; Mehta and Rath, 2021). Additive manufacturing constructs three-dimensional objects directly from the CAD designs using techniques such as fused deposition modeling (FDM) and stereolithography (SLA) (Gale et al., 2018; Weisgrab et al., 2019; Mehta and Rath, 2021). Moreover, this technique allows for the fabrication of the final enclosed device directly from the resin and also for the development of PDMS molds using specific resins (He et al., 2016; Razavi Bazaz et al., 2020; Mehta and Rath, 2021). The printed parts may also be bonded to other substrates or 3D printed parts using adhesive tapes or treatments such as UV bonding (Bressan et al., 2019; Razavi Bazaz et al., 2020; Wei et al., 2022). Owing to the fact that 3D printing does not require a cleanroom setting nor the skilled personnel, this method holds great promise for low-cost microfluidics, especially when non-conventional designs and multi-layered structures are needed (Raoufi et al., 2020; Razavi Bazaz et al., 2020; Su et al., 2020). However, current 3D printing techniques still have limitations such as clogging of the channels, poor quality of the surfaces and low resolution (He et al., 2016; Mehta and Rath, 2021). Despite having lower resolution than conventional cleanroom techniques, the resolution of 3D printers is already suitable for multiple microfluidic applications (He et al., 2016; Mehta and Rath, 2021). Although the selection of resins for printing transparent parts and molds is limited, with the rapid advances in this technology, 3D printing resins that promote better resolution, surface finishing and transparency would further enhance the capabilities of microfluidic devices; in fact, there are resins currently being developed with the specific purpose of fabricating microfluidic devices (e.g., Figure 4E) (He et al., 2016; Nielsen et al., 2020). For further discussion on 3D printing technologies applied to microfluidic devices manufacturing, the readers are encouraged to review the following references: (Chen et al., 2016; He et al., 2016; Enders et al., 2019; de Almeida Monteiro Melo Ferraz et al., 2020; Gonzalez et al., 2020; Nielsen et al., 2020; Mehta and Rath, 2021).
2.2.3 Micromilling
Unlike 3D printing, micromilling is a subtractive manufacturing technique that removes the materials from the bulk to create the desired structures. The prepared parts can be bonded to a substrate to create the final enclosed microfluidic device (Hossain and Rahman, 2018; Rahim and Ehsan, 2021), or it can be used as a mold for PDMS (Jiménez-Díaz et al., 2019; Javidanbardan et al., 2021). Similar to many other low-cost fabrication methods, micromilling does not require a cleanroom and is relatively fast, greatly expediting the manufacturing processes especially for prototyping tests (Faustino et al., 2016; Nguyen et al., 2019). Currently, many materials have been explored to create microfluidic devices using micromilling, among which PMMA and aluminum are two most popular materials (Jiménez-Díaz et al., 2019; Nguyen et al., 2019; Behroodi et al., 2020; Javidanbardan et al., 2021; Saptaji et al., 2021). The micromilled molds made of aluminum can be used for casting multiple times, which could further reduce the cost of the final device (Guckenberger et al., 2015; Nguyen et al., 2019).
On the other hand, micromilling has several limitations that should be considered. For example, the milling bits used in micromilling are prone to breaking especially when high resolution (e.g., 25 µm) is required (Charles et al., 2018; Leclerc, 2021). In addition, complex 3D features and designs may not be suitable for micromilling, even though customized milling bits may be able to create structures with preset shapes (Ku et al., 2018; Javidanbardan et al., 2021). Micromilling only removes the materials from external surfaces, therefore bonding with other substrates is inevitable to create enclosed microchannels. The bonding could be done mechanically (i.e., using screws), thermally (i.e., bonding two PMMA plates when heated up), or using surface treatments and adhesives such as the tapes (Kosoff et al., 2018; Owens and Hart, 2018; Madureira et al., 2019; Gonçalves et al., 2021).
2.2.4 Laser micromachining
Laser micromachining has also been employed for low-cost microfluidics (Mohammed et al., 2016; Persson et al., 2022). For example, CO2 laser is a widely used microfabrication method (Chen et al., 2019; Buchroithner et al., 2021; Nishat et al., 2021; Shin and Choi, 2021). During the fabrication process, the laser energy is focused on the region of interest of the workpieces, causing the materials to melt and evaporate. Typically, a CO2 laser with a wavelength of 10.6 µm are used (Mohammed et al., 2016; Persson et al., 2022). Indeed, sophisticated laser machine or reduced wavelength (e.g., femtosecond lasers) can be applied to further improve the cutting resolution, yet these methods are not suitable for low-cost microfluidics since extra costs are inevitably required (Elgohary et al., 2020; Saadat et al., 2020; Andriukaitis et al., 2022). When it comes to the materials used in laser micromachining, both hard materials such as glass and soft materials such as PMMA, cyclic olefin copolymer (COC) and even paper could be used (Islam et al., 2018; Lin et al., 2019b). Note that to avoid the cracks caused by thermal stress, surface coating could be applied on the glass slides (Chung et al., 2010). In addition, laser micromachining can be used to create both molds and final devices after bonding (Mahmud et al., 2018; Gao et al., 2019a; Ma et al., 2019). The bonding and assembly techniques used for laser cut devices are similar to those used for micromilled devices (Faustino et al., 2016; Mohammed et al., 2016; Nguyen et al., 2019; Persson et al., 2022).
2.2.5 Other fabrication methods
Thin plastic films can also be directly made into final devices via screen printing technology, or as simple as hand cutting (Gale et al., 2018; Nishat et al., 2021). Films and thin plastics can be fabricated at large scale using laminate manufacturing or roller imprinting (Focke et al., 2010; Su et al., 2016). Note that PMMA has been widely used in low-cost microfluidics, it has been used to create devices by micromilling, laser ablation, and by the injection molding (Kotz et al., 2020; Ma et al., 2020), thus holding promise in mass production (Trotta et al., 2018; Ma et al., 2020). It is also worth mentioning that the methods such as roller imprinting, injection molding and hot embossing do require a high resolution mold, which increases the initial cost but eventually can compensate towards low unit price (Shiu et al., 2008; Zhang et al., 2018; Zhang et al., 2019). Indeed, there are other fabrication methods explored for microfluidics, for example, microwire has been used to create devices but the performance is not as high as that of 3D printing (Jia et al., 2008; Kuo et al., 2013). Interested readers are encouraged to read the references (Tomazelli Coltro et al., 2014; Faustino et al., 2016; Raj M and Chakraborty, 2020). Table 1 compares the previously mentioned fabrication methods.
3 Latest environmental applications
In this section, we will review the latest low-cost microfluidic advancements in the field of environmental monitoring. There are three main subsections to summarize and discuss the devices used for water, air, and soil contamination detection.
3.1 Water quality monitoring
Effective water quality monitoring and assessment are of great importance and essential to public health. Low-cost microfluidic devices offer competitive performance as compared to sophisticated equipment in centralized laboratories yet are more cost-effective and provide simpler operation and more rapid analytical analysis (Jaywant and Arif, 2019; Saez et al., 2021). As a result, much effort has been made to develop more effective and low-cost microfluidic devices for efficient water quality monitoring for the assessment of different types of contaminants. This section provides the readers with an overview of the most recent advancements in this regard.
3.1.1 Heavy metal pollutants
Heavy metal pollution in water has received increasing attention over the past decades (Almeida et al., 2018; Santangelo et al., 2019; Kinuthia et al., 2020). It is reported that even at low concentrations, these contaminants can pose a great threat to the aquatic environment, ecosystem, and human health (Snyder et al., 2020; Barocio et al., 2021). Given such growing concerns, low-cost microfluidic devices can be an affordable tool for continuous water monitoring regarding heavy metal contamination worldwide. The burgeoning advancements are distinct as evidenced by continuous developments made over the past years, with many applications built on top of paper microfluidics (Almeida et al., 2018). To name a few, Wang et al. developed a μPAD with high detection accuracy and selectivity for lead ions (Pb2+) in drinking water. The device realized rapid visual quantitative detection by examining the extension length of the color bar in the particle dam (Wang et al., 2022). A similar device was designed to quantify silver (Ag+) contamination in freshwater, and it was reported to have a detection limit of 453.7 nM, high selectivity, and a high recovery rate of 96.8% (Wang et al., 2020). Jarujamrus et al. developed a μPAD to detect Mercury (Hg2+) in various water samples with the ability to instantly report Hg2+ concentration on-site by using a smartphone. The smartphone analyzer is responsive and user-friendly, which has enabled unskilled users to use this device to conduct sample analysis (Jarujamrus et al., 2018). Similar applications used μPADs for the detection of Cu2+ (Quinn et al., 2018; Sharifi et al., 2020).
Besides the detection of a single type of heavy metal, μPADs were also developed for the identification of multiple heavy metals simultaneously. Khoshbin et al. developed a paper-based aptasensor to detect Ag+ and Hg2+ within 10 min based on conformational changes of Ag+ -and Hg2+ specific aptamers. The concentration of the ions can be indicated by fluorescence recovery rate, with a limit of detection of 1.33 p.m. for Hg2+ and 1.01 p.m. for Ag+ (Khoshbin et al., 2020). Idros et al. used a μPAD to detect several major heavy metals, including Hg2+, Pb2+, Cr3+, Ni2+, Cu2+, and Fe3+ by applying different ligands loaded onto the test paper (Idros and Chu, 2018) (Figure 5A). Similarly, Kamnoet et al. capitalized on the colorimetric assays to identify multiple heavy metals including Cu2+, Co2+, Ni2+, Hg2+, and Mn2+ with a corresponding limit of detection of 0.32, 0.59, 5.87, 0.20, and 0.11 mg/L, respectively (Kamnoet et al., 2021).
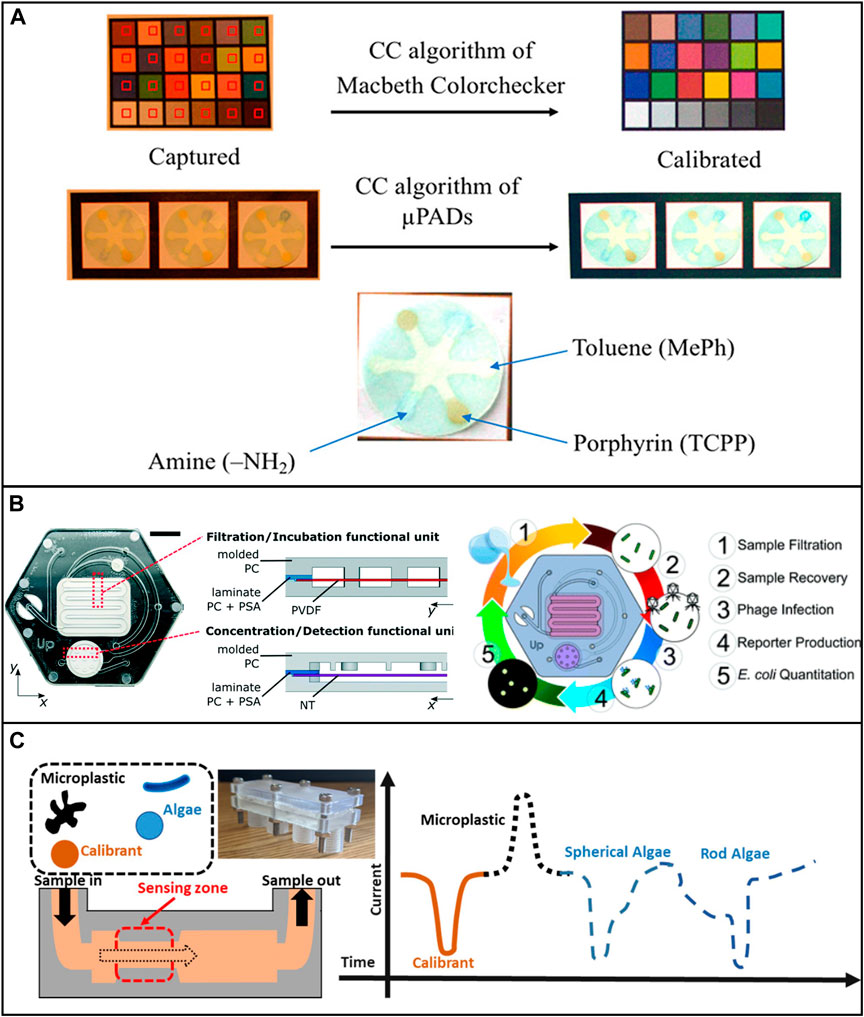
FIGURE 5. Low-cost microfluidic platforms for water quality monitoring. (A) Detection of Hg2+, Pb2+, Cr3+, Ni2+, Cu2+, and Fe3+ by applying different ligands loaded onto the test paper. Reproduced with permission from (Idros and Chu, 2018). (B) Microfluidic device for the identification of E. Coli in water samples. The device was fabricated using polycarbonate. Reproduced with permission from (Alonzo et al., 2022). (C) Characterizing algae with spherical and microplastics from tea bags with a 3D printed device. Reproduced with permission from (Pollard et al., 2020).
The porous nature of papers and capillary driving can limit associated fluid and particle manipulation. Herein, other materials such as polymers are also applied to fabricate microfluidic devices for more accurate heavy metal detection. For example, an epitaxial graphene sensor was combined with a 3D-printed microfluidic chip to detect Pb2+ and Cd2+ (Santangelo et al., 2019). In another study, a porous conductive carbon cloth was integrated with a microfluidic device to desalinate and recover valuable metal ions (Cu2+, Zn2+, Ni2+, Ag+, and Zn2+/Cu2+ mixtures) from wastewater samples (Allioux et al., 2018). Ding et al. successfully conducted heavy metal analysis by using a sponge-based microfluidic device that was integrated with ion-selective electrodes for sampling heavy metal ions (Cd2+ and Pb2+) and non-metal clinically related chemical ions, namely K+, Na+, and Cl− (Ding et al., 2021). Furthermore, a combined μCPAD was developed to detect Mercury and lead ions in water samples. The groups used cloth’s ductility and durability to endure the oscillation during fabrication to improve the producibility and life span of the device (Wang et al., 2022).
3.1.2 Non-metallic pollutants
Non-metal substances are more abundant pollutants in water and are highly complex by nature. Nowadays, portable microfluidic devices are playing a critical role in water quality analysis for a large variety of toxins, such as pharmaceutical residues, due to their many advantages (Barocio et al., 2021). Scala-Benuzzi et al. developed an electrochemical paper-based immunocapture assay (EPIA) to assess Ethinylestradiol quantitively in water samples. It was reported the test achieved a low detection limit of 0.1 ng/L and a linearity range of 0.5–120 ng/L (Scala-Benuzzi et al., 2018). In another study, chlorpyrifos pesticide was detected by using a lipase-embedded paper-based device (Sankar et al., 2020). The limit of detection and limit of quantification was found to be 0.065 mg/L and 0.198 mg/L, respectively. Interestingly, the wash water of cauliflower, grapes, coriander leaves, brinjal, and bitter guard could be used as samples (Sankar et al., 2020). Jemmeli et al. developed a highly sensitive paper-based electrochemical sensor to detect bisphenol A (BPA) in drinking water (Jemmeli et al., 2020). Mako et al. developed a μPAD to detect nitrite levels in drinking water (Mako et al., 2020). Peters et al., developed a μPAD to monitor total ammonia levels in freshwater (Peters et al., 2019). Similarly, μPAD was used for detecting phosphate in water samples (Sarwar et al., 2019; Racicot et al., 2020). Besides paper-based devices, Carvalho et al. developed a fully 3D printed thread-based microfluidic device to detect Nitrite in well water samples with high precision (Carvalho et al., 2021). Caetano et al. developed a textile thread-based microfluidic device combined with an electrochemical biosensor to detect phenol concentration in tap water (Caetano et al., 2018).
It is worth noting that, among all the non-metallic pollutants, microplastics have been drawing lots of research attention recently. Microfluidic devices can benefit microplastic-related research in many ways, such as microplastic identification and separation. However, only a few low-cost microfluidic devices have been developed for these applications. Pollard et al. developed a low-cost and high-throughput three-dimensional printed microfluidic resistive pulse sensor for characterizing algae with spherical and rod structures as well as microplastics from tea bags. The device can rapidly screen liquids at a volume rate of 1L/min in the presence of microplastic and algae (Pollard et al., 2020) (Figure 5C). Mesquita et al. developed a 3D printed microfluidic device for microplastic identification that improved the Nile Red staining process (Mesquita et al., 2022). It is suggested that researchers use the full potential of low-cost microfluidic devices to achieve reproducible and reliable long-term assessment of environmental microplastics.
3.1.3 Waterborne microorganisms
The presence of waterborne pathogens can cause severe illnesses. Continuous monitoring and in-situ studies of waterborne microorganisms are another rapidly growing research interest. In a recent study, Yin et al. developed a 3D-printed integrated microfluidic chip for colorimetric detection of SARS-CoV-2 and other human enteric pathogens in wastewater. The sensitivity of detection was reported to be 100 genome equivalent (GE)/mL for SARS-CoV-2 and 500 colony-forming units (CFU)/mL for other targeted human enteric pathogens (Yin et al., 2021). Schaumburg et al. designed a μPAD for waterborne bacteria detection which consists of two sequential pre-concentration steps. The detection limit of concentration was as low as 9.2 CFU/ml in laboratory samples and 920 CFU/ml in apple juice samples within ∼90 min (Schaumburg et al., 2019). Several studies successfully used μPAD and 3D printed microfluidic devices to detect E. coli in various water samples and achieved low detection limit, high sensitivity, and quick analysis (Sweet et al., 2019; Lin et al., 2020b; Snyder et al., 2020; Alonzo et al., 2022).
3.2 Air quality monitoring
Monitoring and controlling airborne microparticles are drawing attention due to the decreasing air quality across the globe. Microfluidic devices have proven the ability to sort and separate microparticles effectively, which shall be used in air quality monitoring for particle trapping and real-time concentration analysis. In this section, recent applications of low-cost microfluidic devices in the assessment of airborne micro particles are discussed.
3.2.1 Metallic and non-metallic pollutants
Airborne metal particles are one of the most representative harmful elements. Several studies have used μPAD to detect some typical airborne metal particles. Sun et al. developed a μPAD that realized on-site multiaxial quantification of airborne trace metals by implementing unmanned aerial vehicle in-air sampling (UAV). Data can be easily processed by a smartphone within 30 min (Sun et al., 2018). The same group later applied graphene oxide (GO) coating onto the paper and improved the detection limits for Fe, Cu, and Ni to 6.6, 5.1, and 9.9 ng respectively, which is comparable to the commercial coupled plasma (ICP) instruments (Sun et al., 2019) (Figure 6A). Jia et al. successfully used a μPAD to detect cobalt (Co), copper (Cu), and iron (Fe) in ambient air and street sediments with detection limits of 8.2, 45.8, and 186.0 ng (Jia et al., 2017).
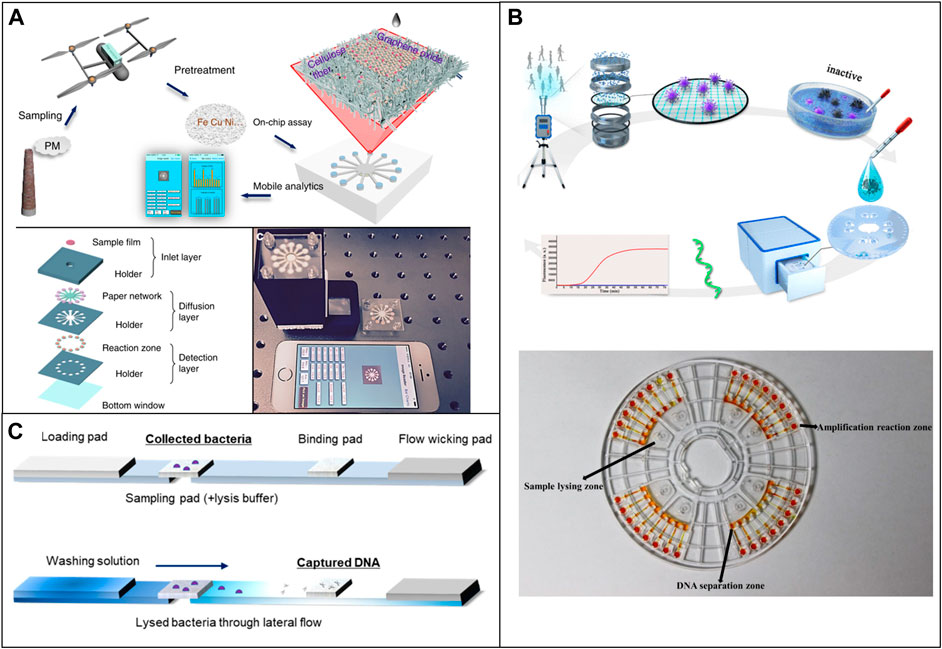
FIGURE 6. Low-cost microfluidic platforms for air quality monitoring. (A) Quantification of airborne trace metals, such as Fe, Cu, and Ni with μPAD. Reproduced with permission from (Sun et al., 2019). (B) Microfluidic device used for the identification of Covid in air samples. Reproduced with permission from (Xiong et al., 2021). (C) Detection of airborne bacteria with μPAD and a 3D printed device. Reproduced with permission from (Seok et al., 2021).
Unlike water applications, only a few devices used low-cost microfluidic devices for the detection of non-metallic airborne pollutants. For example, Guo et al. developed a smartphone-based microfluidic sensor to detect gaseous formaldehyde in the ambient. The microfluidic chip consists of two reagent reservoirs, a reaction reservoir, and a mixing column. The PTFE membrane was used to prevent the fluid from flowing out while the gas molecules enter. The system showed great selectivity against other ambient gas (Guo et al., 2018). Zhao et al. developed a 3D printed based microfluidic impactor for particular matter classification and concentration detection (Zhao et al., 2016). However, most of these applications used costly fabrication techniques, such as photolithography, and the integration with multiple sensors also increased the overall cost (Poenar, 2019).
3.2.2 Airborne microorganisms
Dias et al. used a μPAD to detect levoglucosan concentration in the ambient with a colorimetric method. The linear detection range is 0–64.8 μg m/L and the detection limit is 2 and 6 μg m/L. The device showed selectivity for levoglucosan with variation in colorimetric signal intensity lower than 8% (Dias et al., 2019). Seok et al. developed a μPAD combined with a 3D printed analysis kit for detection of airborne bacteria by collecting aerosols (Seok et al., 2021) (Figure 6C).
3.3 Soil quality monitoring
Soil is home to many types of microorganisms and nutrients and contains many types of toxic pollutants. Simplified detection methods and analysis devices for soil quality management are beneficial to agricultural development, ecosystem, and human health. This section reviews the recent applications of low-cost microfluidic approaches used for soil quality assessments.
3.3.1 Heavy metals and non-metallic pollutants
Ding et al. used an acidified μPAD integrated with potentiometric sensors for the detection of multiple heavy metal ions in the soil, street run-off, and multiple environmental samples (Ding et al., 2021). Similarly, an eco-friendlier metal-modified μPAD was developed for the same purpose (Silva et al., 2022). Xi et al. developed a centrifugal microfluidic system for pyrene extraction from soil (Xi et al., 2010). A similar centrifugal microfluidic device was also used for the detection of pesticide residues in vegetables and soil (Duford et al., 2013). Other soil nutrients can be detected using colorimetric microfluidic devices, most of these applications used μPAD, 3D printed devices, and a combination of low-cost fabrication techniques (Cheng et al., 2021).
3.3.2 Soil microorganisms
With the advantages of microfluidic platforms, the development of soil-on-a-chip has been growing to study soil biofilms and microorganisms’ ecological and biological impacts (Stanley et al., 2016; Wu et al., 2022). However, challenges and limitations still exist, such as the controlling of hydrophilic and hydrophobic surfaces in PDMS based devices, which highlighted the potential benefits of using porous membrane microchannels, which are normally fabricated with low costs.
3.4 Other microfluidic platforms for environmental applications
Microfluidic devices can be used for many other environmental applications. The burgeoning demand for reliable and reproducible devices that can be mass-produced makes low-cost microfluidic approaches more appealing. Readers are encouraged to read review papers in this regard and further implement low-cost fabrication techniques by combining different methodologies or converting existing designs to low-cost versions (Pol et al., 2017; Dhar and Lee, 2018; Pouyanfar et al., 2022). Table 2 summarizes the low-cost microfluidic platforms for environmental monitoring mentioned in this review regarding their substrate material, fabrication method, detection methods, and significant contributions and results.
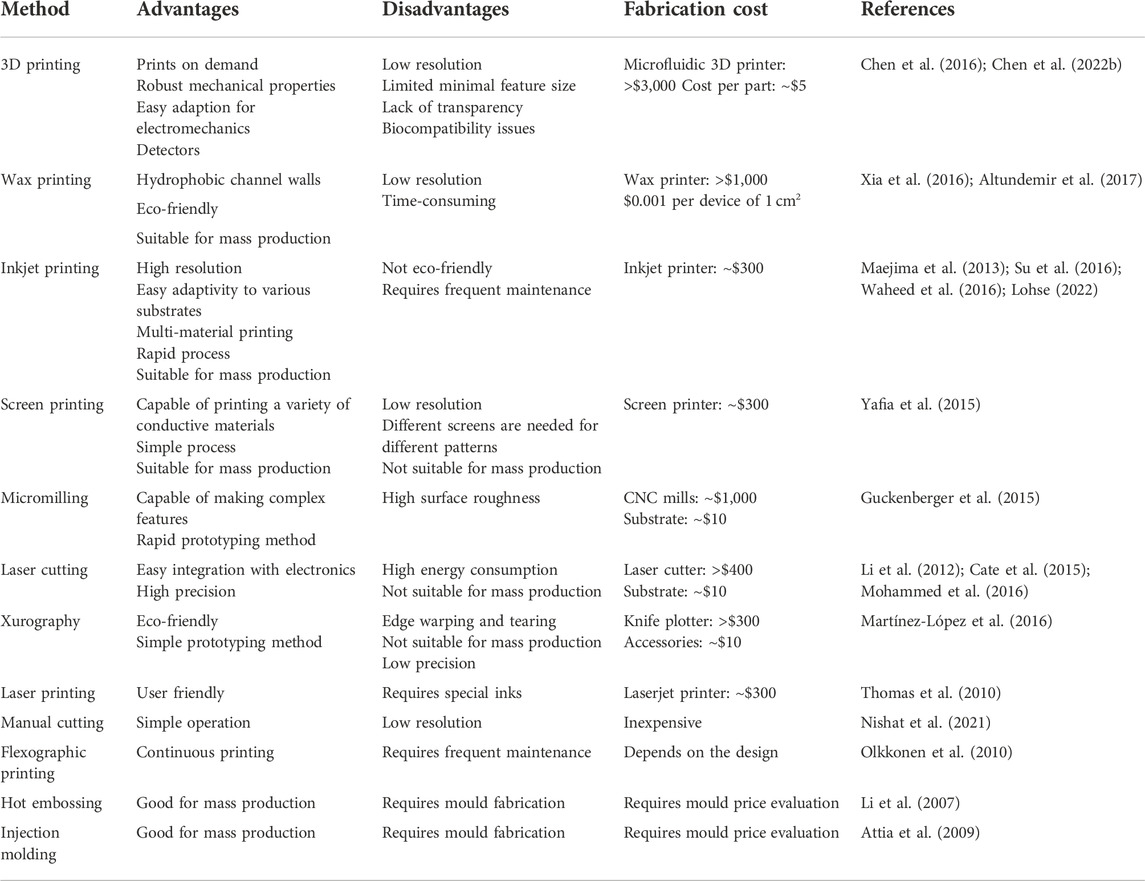
TABLE 1. Advantages, disavantages, and cost estimations for the aforementioned low-cost fabrication technologies.
4 Discussion and conclusion
Low-cost microfluidic technologies have grown over the years, especially because the materials and fabrication methods summarized here are useful to aid places with limited resources, proving to be a reliable substitute to expensive equipment and complex operation processes (Tomazelli Coltro et al., 2014; Morbioli et al., 2017). Among all the low-cost devices, paper is one of the most widely used, given its high availability and easy manufacturing techniques (Adkins et al., 2015; Xia et al., 2016). In addition, porous devices are attractive because they are user friendly, its capillary nature made it possible to eliminate the dependency on external flow control equipment (i.e., no necessity of pumps), easing the operation (Adkins et al., 2015; Nishat et al., 2021). However, porous devices lack the ability to provide equivalent abilities in fluid and particle manipulation as non-porous devices due to the passive nature of fluid wicking (Adkins et al., 2015; Razavi Bazaz et al., 2020).
In addition, a variety methods have been successfully used to create polymeric devices, including 3D printing, micromilling, laser cutting, xurography, injection moulding, and hot embossing (Attia et al., 2009; Martínez-López et al., 2016; Nguyen et al., 2019; Razavi Bazaz et al., 2020; Persson et al., 2022). Specifically, 3D printing has emerged as an inexpensive fabrication approach, providing acceptable resolution that is beneficial for the creation of complex microchannels (Razavi Bazaz et al., 2020; Carrasco-Correa et al., 2021). Xurography employs a knife cutter to cut structures in the materials, this simple method is useful for the fabrication of rapid tests through the employment of laminated devices (Gosset et al., 2018; Speller et al., 2019; Hong Tham Phan and Kim, 2022). However, the resolution is highly limited by the blade sizes and the method lacks the capabilities to fabricate thick devices (Martínez-López et al., 2016; Mohammed et al., 2016; Persson et al., 2022). Laser micromachining has its resolution heavily reliant on the laser quality and wavelength, in order to increase the resolution, expensive lasers are necessary, limiting its applications in low-cost microfluidics (Chen et al., 2018; Nishat et al., 2021; Shin and Choi, 2021). Mass production remains a key approach to reduce the final cost of a single unit, in this regard, several methods such as injection molding and hot embossing are good candidates (Li et al., 2007; Chan et al., 2015).
Despite great achievements made over the past years for low-cost microfluidics, current devices still do not possess competitive performance compared to devices made using traditional methods especially those based on the clean room fabrication techniques. In summary, the selection of the most appropriate material for certain applications is critical to achieve the desired performance for microfluidic devices. Though there is a large pool of potential materials available for selection, the goal of achieving low-cost, good quality, and efficient high-volume production remains to be the challenging triple constraints for creating a more competitive force for low-cost microfluidics regarding capability, reproducibility, and sustainability (He et al., 2016; Amin et al., 2017).
Environmental monitoring is a field that demands costly analysis techniques and have been benefited from low-cost microfluidic devices (Jokerst et al., 2012; Kung et al., 2019). Water quality monitoring is an important application of low-cost microfluidic devices, as freshwater management is essential to human life, although still limited in some places (Komolafe et al., 2014; Budlayan et al., 2022). In case of water contamination, low-cost microfluidic devices can assist tracking diseases since multiple tests can be performed using disposable or reusable devices (e.g., Covid on wastewater using 3D printed devices (Yin et al., 2021)). Instead of outsourcing tests, it is possible to continuously monitor water quality using portable tests (Berardi et al., 2008; Rizzo, 2010). Heavy metal detection in water samples using paper-based devices have been demonstrated in different devices: multiple heavy metals in coastal waters (Wang et al., 2021); in situ cadmium (Yuan et al., 2022) and Mercury (Budlayan et al., 2022) detection, indicating that most heavy metals can be identified using low-cost devices. Micromilling and 3D printed devices were explored for heavy metal detection (Santangelo et al., 2019; Huang et al., 2021) and though these devices were successful experimentally and demonstrated enough accuracy to be used in the lab, the dependency on external pumping systems and trained personnel still limit their market potential. When it comes to processing samples with large volumes, injection molded devices have emerged as a major player. For example, the detection of E. coli in earlier stages was performed using an injection molded device capable of processing 100 ml of water (Alonzo et al., 2022).
Air and soil quality are other important fields of environmental monitoring due to their direct relation with humans (Fenger, 1999; Akimoto, 2003). Different µPADs have been developed for heavy metal detection in soil street run-off samples (Ding et al., 2021), and air samples (Jia et al., 2017). Non-porous technologies have also been used for soil analysis, such as centrifugal microfluidic device for the detection of pesticide residues in vegetables and soil (Xi et al., 2010; Duford et al., 2013). Despite many successful applications in the air and soil monitoring areas, as evidenced in research publications, low-cost devices have yet to be widely commercially available in the market due to complexity of air and soil samples (Jokerst et al., 2012; Schulze et al., 2017). Samples are normally filtered and washed, which is time consuming (especially for air samples, which have to be captured in open space) hindering the use of low-cost technologies by the general public (Sun et al., 2018; Zhu et al., 2022). Though microfluidic devices have been widely used for environmental applications, not all devices can be considered user-friendly and low-cost (Tomazelli Coltro et al., 2014; Faustino et al., 2016). Especially for air and soil environmental analysis, as discussed above, this can be mainly due to the sample pre-processing and device operation (e.g., pump operation in 3D printed devices). More applications related to air and soil quality monitoring are encouraged and should be developed. Most of the low-cost devices reviewed in the paper used colorimetric detection method (Xia et al., 2016; Weng et al., 2019; Tabani et al., 2022). Though water is related to a lot of applications, there is a lack of standardization that could be beneficial to boost commercialization.
Cost reduction is critical to expand the usage of microfluidic devices in environmental monitoring. A combination of a few low-cost techniques (hybrid devices) was attempted for different purposes and should be further explored (Dou et al., 2015; Ruiz et al., 2020; Mehta and Rath, 2021). Hybrid devices can take advantage of commercially available technologies (Ruiz et al., 2020; Mehta and Rath, 2021; Wang et al., 2022). Smartphones have been used along with low-cost microfluidic devices to enhance accuracy and overall performance (Lopez-Ruiz et al., 2014; Sarwar et al., 2019; Yin et al., 2021). Smartphones were coupled with paper-based inkjet-printed devices for Cr3+ and Al3+ identification on water (Taheri and Khayatian, 2022), as well as Pb2+ (Cui et al., 2022). For the case of air quality control, drones were used to collect samples in different location and heights, and used smartphones for data processing within 30 min at a cost of $1.92 (Sun et al., 2018). The samples still needed to be pre-processed with acid solutions for final analysis, showing that this step requires more simplification. A filtration system was developed to be used in the field (hand powered), which is a good option to substitute pumping systems in devices that do not require flow rate precision (Quinn et al., 2018). With the advantages of microfluidic platforms, the development of soil-on-a-chip devices has been growing to study soil biofilms and ecological and biological impacts of microorganisms, which is of fundamental importance for the constant development of novel and better performing agricultural practices (Stanley et al., 2016; Wu et al., 2022). Overall, low-cost microfluidic devices have proven their capability to perform environmental monitoring assessment, furthermore, low-cost microfluidics have been contributing to the worldwide spread of microfluidic technologies, indicating that researchers should keep innovating towards more reliable cost-effective devices (Jokerst et al., 2012; Stanley et al., 2016; Budlayan et al., 2022).
Author contributions
PM and LG drafted the manuscript. YL drafted and revised the manuscript.
Funding
We acknowledge financial support from Rhode Island Foundation (8429_20210963).
Conflict of interest
The authors declare that the research was conducted in the absence of any commercial or financial relationships that could be construed as a potential conflict of interest.
Publisher’s note
All claims expressed in this article are solely those of the authors and do not necessarily represent those of their affiliated organizations, or those of the publisher, the editors and the reviewers. Any product that may be evaluated in this article, or claim that may be made by its manufacturer, is not guaranteed or endorsed by the publisher.
References
Adkins, J., Boehle, K., and Henry, C. (2015). Electrochemical paper-based microfluidic devices. Electrophor. [Internet] 36 (16), 1811–1824. Available from. doi:10.1002/elps.201500084
Adyel, T. M. (2020). Accumulation of plastic waste during COVID-19. Science 369 (6509), 1314–1315. doi:10.1126/science.abd9925
Agustini, D., Bergamini, M. F., and Marcolino-Junior, L. H. (2016). Low cost microfluidic device based on cotton threads for electroanalytical application. Lab. Chip 16 (2), 345–352. doi:10.1039/c5lc01348h
Agustini, D., Caetano, F. R., Quero, R. F., Fracassi da Silva, J. A., Bergamini, M. F., Marcolino-Junior, L. H., et al. (2021). Microfluidic devices based on textile threads for analytical applications: State of the art and prospects. Anal. Methods 13, 4830–4857. doi:10.1039/d1ay01337h
Akimoto, H. (2003). Global air quality and pollution. Science 302 (5651), 1716–1719. doi:10.1126/science.1092666
Akyazi, T., Basabe-Desmonts, L., and Benito-Lopez, F. (2018). Review on microfluidic paper-based analytical devices towards commercialisation. Anal. Chim. Acta 1001, 1–17. [Internet]Available from:. doi:10.1016/j.aca.2017.11.010
Aladese, A. D., and Jeong, H-H. (2021). Recent developments in 3D printing of droplet-based microfluidics. BioChip J. 15, 313–333. doi:10.1007/s13206-021-00032-1
Allioux, F-M., Kapruwan, P., Milne, N., Kong, L., Fattaccioli, J., Chen, Y., et al. (2018). Electro-capture of heavy metal ions with carbon cloth integrated microfluidic devices. Sep. Purif. Technol. 194, 26–32. [Internet]Available from:. doi:10.1016/j.seppur.2017.10.064
Almeida, M. I. G. S., Jayawardane, B. M., Kolev, S. D., and McKelvie, I. D. (2018). Developments of microfluidic paper-based analytical devices (μPADs) for water analysis: A review. Talanta 177, 176–190. [Internet]Available from:. doi:10.1016/j.talanta.2017.08.072
Alonzo, L. F., Hinkley, T. C., Miller, A., Calderon, R., Garing, S., Williford, J., et al. (2022). A microfluidic device and instrument prototypes for the detection of Escherichia coli in water samples using a phage-based bioluminescence assay. Lab. Chip 22 (11), 2155–2164. [Internet]Available from. doi:10.1039/D1LC00888A
Alsaeed, B., and Mansour, F. R. (2020). Distance-based paper microfluidics; principle, technical aspects and applications. Microchem. J. 155, 104664. [Internet]Available from:. doi:10.1016/j.microc.2020.104664
Altundemir, S., Uguz, A. K., and Ulgen, K. (2017). A review on wax printed microfluidic paper-based devices for international health. Biomicrofluidics 11 (4), 041501. [Internet]Available from. doi:10.1063/1.4991504
Amin, R., Joshi, A., and Tasoglu, S. (2017). Commercialization of 3D-printed microfluidic devices. J. 3D Print. Med. 1 (2), 85–89. [Internet]Available from. doi:10.2217/3dp-2016-0010
Andriukaitis, D., Vargalis, R., Šerpytis, L., Drevinskas, T., Kornyšova, O., Stankevičius, M., et al. (2022). Fabrication of microfluidic tesla valve employing femtosecond bursts. Micromachines 13 (8), 1180. doi:10.3390/mi13081180
Arroyo, M. J., Erenas, M. M., Orbe-Payá, I. D., Cantrell, K., Dobado, J. A., Ballester, P., et al. (2020). Thread based microfluidic platform for urinary creatinine analysis. Sensors Actuators B Chem. 305, 127407. doi:10.1016/j.snb.2019.127407
Attia, U. M., Marson, S., and Alcock, J. R. (2009). Micro-injection moulding of polymer microfluidic devices. Microfluid. Nanofluidics 7 (1), 1–28. doi:10.1007/s10404-009-0421-x
Au, A. K., Huynh, W., Horowitz, L. F., and Folch, A. (2016). 3D-Printed microfluidics. Angew. Chem. Int. Ed. 55 (12), 3862–3881. [Internet]Available from. doi:10.1002/anie.201504382
Bae, C. W., Chinnamani, M. V., Lee, E. H., and Lee, N-E. (2022). Stretchable non-enzymatic fuel cell-based sensor patch integrated with thread-embedded microfluidics for self-powered wearable glucose monitoring. Adv. Mater. Interfaces 9 (20), 2200492. [Internet]Available from. doi:10.1002/admi.202200492
Bagherbaigi, S., Córcoles, E. P., and Wicaksono, D. H. B. (2014). Cotton fabric as an immobilization matrix for low-cost and quick colorimetric enzyme-linked immunosorbent assay (ELISA). Anal. Methods 6 (18), 7175–7180. doi:10.1039/c4ay01071j
Bamshad, A., and Cho, H. J. (2021). Laserjet printed micro/nano sensors and microfluidic systems: A simple and facile digital platform for inexpensive, flexible, and low-volume devices. Adv. Mater. Technol. 6 (12), 2100401. doi:10.1002/admt.202100401
Barocio, M. E., Hidalgo-Vázquez, E., Kim, Y., Rodas-Zuluaga, L. I., Chen, W-N., Barceló, D., et al. (2021). Portable microfluidic devices for in-field detection of pharmaceutical residues in water: Recent outcomes and current technological situation – a short review. Case Stud. Chem. Environ. Eng. 3, 100069. [Internet]Available from:. doi:10.1016/j.cscee.2020.100069
Behroodi, E., Latifi, H., Bagheri, Z., Ermis, E., Roshani, S., and Salehi Moghaddam, M. (2020). A combined 3D printing/CNC micro-milling method to fabricate a large-scale microfluidic device with the small size 3D architectures: An application for tumor spheroid production. Sci. Rep. 10 (1), 22171–22184. doi:10.1038/s41598-020-79015-5
Berardi, L., Giustolisi, O., Kapelan, Z., and Savic, D. A. (2008). Development of pipe deterioration models for water distribution systems using EPR. J. Hydroinformatics 10 (2), 113–126. doi:10.2166/hydro.2008.000
Bertana, V., Potrich, C., Scordo, G., Scaltrito, L., Ferrero, S., Lamberti, A., et al. (2018). 3D-printed microfluidics on thin poly (methyl methacrylate) substrates for genetic applications. J. Vac. Sci. Technol. B, Nanotechnol. Microelectron. Mater. Process. Meas. Phenom. 36 (1), 01A106. doi:10.1116/1.5003203
Berthier, J., Brakke, K. A., Gosselin, D., Berthier, E., and Navarro, F. (2017). Thread-based microfluidics: Flow patterns in homogeneous and heterogeneous microfiber bundles. Med. Eng. Phys. 48, 55–61. [Internet]Available from:. doi:10.1016/j.medengphy.2017.08.004
Bhattacharjee, N., Urrios, A., Kang, S., and Folch, A. (2016). The upcoming 3D-printing revolution in microfluidics. Lab. Chip 16 (10), 1720–1742. doi:10.1039/c6lc00163g
Boelle, P. Y., Decormeille, G., Hermann, B., Heming, N., Jacq, G., Kamel, T., et al. (2022). Sanitary safety of the 2021 French intensive care society medical conference: A case/control study. Ann. Intensive Care 12 (1), 11. doi:10.1186/s13613-022-00986-x
Böhm, A., Carstens, F., Trieb, C., Schabel, S., and Biesalski, M. (2014). Engineering microfluidic papers: Effect of fiber source and paper sheet properties on capillary-driven fluid flow. Microfluid. Nanofluidics 16 (5), 789–799. doi:10.1007/s10404-013-1324-4
Bressan, L. P., Adamo, C. B., Quero, R. F., de Jesus, D. P., and da Silva, J. A. F. (2019). A simple procedure to produce FDM-based 3D-printed microfluidic devices with an integrated PMMA optical window. Anal. Methods 11 (8), 1014–1020. doi:10.1039/c8ay02092b
Buchroithner, B., Mayr, S., Hauser, F., Priglinger, E., Stangl, H., Santa-Maria, A. R., et al. (2021). Dual channel microfluidics for mimicking the blood–brain barrier. ACS Nano 15 (2), 2984–2993. doi:10.1021/acsnano.0c09263
Budlayan, M. L., Dalagan, J., Lagare-Oracion, J. P., Patricio, J., Arco, S., Latayada, F., et al. (2022). Detecting mercury ions in water using a low-cost colorimetric sensor derived from immobilized silver nanoparticles on a paper substrate. Environ. Nanotechnol. Monit. Manag. 18, 100736. [Internet]Available from:. doi:10.1016/j.enmm.2022.100736
Caetano, F. R., Carneiro, E. A., Agustini, D., Figueiredo-Filho, L. C. S., Banks, C. E., Bergamini, M. F., et al. (2018). Combination of electrochemical biosensor and textile threads: A microfluidic device for phenol determination in tap water. Biosens. Bioelectron. 99, 382–388. [Internet]Available from:. doi:10.1016/j.bios.2017.07.070
Caffiyar, M. Y., Lim, K. P., Basha, I. H. K., Hamid, N. H., Cheong, S. C., and Ho, E. T. W. (2020). Label-free, high-throughput assay of human dendritic cells from whole-blood samples with microfluidic inertial separation suitable for resource-limited manufacturing. Micromachines 11 (5), 514. doi:10.3390/mi11050514
Carothers, W. H. (1936). Polymers and polyfunctionality. Trans. Faraday Soc. 32, 39–49. doi:10.1039/tf9363200039
Carrasco-Correa, E. J., Simó-Alfonso, E. F., Herrero-Martínez, J. M., and Miró, M. (2021). The emerging role of 3D printing in the fabrication of detection systems. TrAC Trends Anal. Chem. 136, 116177. doi:10.1016/j.trac.2020.116177
Carrell, C., Kava, A., Nguyen, M., Menger, R., Munshi, Z., Call, Z., et al. (2019). Beyond the lateral flow assay: A review of paper-based microfluidics. Microelectron. Eng. 206, 45–54. [Internet]Available from:. doi:10.1016/j.mee.2018.12.002
Carvalho, R. M., Ferreira, V. S., and Lucca, B. G. (2021). A novel all-3D-printed thread-based microfluidic device with an embedded electrochemical detector: First application in environmental analysis of nitrite. Anal. Methods 13 (11), 1349–1357. [Internet]Available from. doi:10.1039/D1AY00070E
Cate, D. M., Adkins, J. A., Mettakoonpitak, J., and Henry, C. S. (2015). Recent developments in paper-based microfluidic devices. Anal. Chem. 87 (1), 19–41. doi:10.1021/ac503968p
Chan, H. N., Chen, Y., Shu, Y., Chen, Y., Tian, Q., and Wu, H. (2015). Direct, one-step molding of 3D-printed structures for convenient fabrication of truly 3D PDMS microfluidic chips. Microfluid. Nanofluidics 19 (1), 9–18. [Internet]Available from. doi:10.1007/s10404-014-1542-4
Charles, P. T., Wadhwa, V., Kouyate, A., Mesa-Donado, K. J., Adams, A. A., Deschamps, J. R., et al. (2018). A high aspect ratio bifurcated 128-microchannel microfluidic device for environmental monitoring of explosives. Sensors 18 (5), 1568. doi:10.3390/s18051568
Chen, C., Mehl, B. T., Munshi, A. S., Townsend, A. D., Spence, D. M., and Martin, R. S. (2016). 3D-printed microfluidic devices: Fabrication, advantages and limitations-a mini review. Anal. Methods 8 (31), 6005–6012. [Internet]. Available from:. doi:10.1039/c6ay01671e
Chen, C., Meng, H., Guo, T., Deshpande, S., and Chen, H. (2022). Development of paper microfluidics with 3D-printed PDMS barriers for flow control. ACS Appl. Mater Interfaces 14, 40286–40296. doi:10.1021/acsami.2c08541
Chen, C., Townsend, A. D., Hayter, E. A., Birk, H. M., Sell, S. A., and Martin, R. S. (2018). Insert-based microfluidics for 3D cell culture with analysis. Anal. Bioanal. Chem. 410 (12), 3025–3035. doi:10.1007/s00216-018-0985-y
Chen, H., Yang, B., and Yang, Z. (2022). “Chapter 1 - fabrication of microfluidic chips,” in Multidisciplinary microfluidic and nanofluidic lab-on-a-chip. Editors C. Yang, and P. C. H. Li (Elsevier), 3–35. [Internet]. Li X (James)Available at: https://www.sciencedirect.com/science/article/pii/B9780444594327000145.
Chen, X., Li, T., and Gao, Q. I. (2019). A novel method for rapid fabrication of PMMA microfluidic chip by laser cutting and sealing integration. Surf. Rev. Lett. 26 (08), 1950042. doi:10.1142/s0218625x19500422
Cheng, Y., Yang, R. M. H., Alejandro, F. M., Li, F., Balavandy, S. K., Wang, L., et al. (2021). 7 - current applications of colourimetric microfluidic devices (smart phone based) for soil nutrient determination. Smartphone-Based Detect. Devices 2021, 103–128. C. Hussain, [Internet]Available at: https://www.sciencedirect.com/science/article/pii/B9780128236963000106.
Chitnis, G., Ding, Z., Chang, C-L., Savran, C. A., and Ziaie, B. (2011). Laser-treated hydrophobic paper: An inexpensive microfluidic platform. Lab. Chip 11 (6), 1161–1165. doi:10.1039/c0lc00512f
Chung, C-K., Chang, H. C., Shih, T. R., Lin, S. L., Hsiao, E. J., Chen, Y. S., et al. (2010). Water-assisted CO2 laser ablated glass and modified thermal bonding for capillary-driven bio-fluidic application. Biomed. Microdevices 12 (1), 107–114. doi:10.1007/s10544-009-9365-x
Colford, J. M. J., Roy, S., Beach, M. J., Hightower, A., Shaw, S. E., and Wade, T. J. (2006). A review of household drinking water intervention trials and an approach to the estimation of endemic waterborne gastroenteritis in the United States. J. Water Health 4 (2), 71–88. doi:10.2166/wh.2006.018
Cortes-Medina, M., Avendano, A., Bushman, A., Chang, C-W., Menyhert, M., and Song, J. W. (2020). Microfluidic prototyping by xurography to engineer fully-lumenized microvessels in vitro. FASEB J. 34 (S1), 1. doi:10.1096/fasebj.2020.34.s1.06369
Cui, Y., Wang, R., Brady, B., and Wang, X. (2022). Fully inkjet-printed paper-based Pb2+ optodes for water analysis without interference from the chloramine disinfectant. Anal. Bioanal. Chem. 414, 7585–7595. Available from. doi:10.1007/s00216-022-04286-y
de Almeida Monteiro Melo Ferraz, M., Nagashima, J. B., Venzac, B., Le Gac, S., and Songsasen, N. (2020). 3D printed mold leachates in PDMS microfluidic devices. Sci. Rep. 10 (1), 994–999. doi:10.1038/s41598-020-57816-y
de Tarso Garcia, P., Garcia Cardoso, T. M., Garcia, C. D., Carrilho, E., and Tomazelli Coltro, W. K. (2014). A handheld stamping process to fabricate microfluidic paper-based analytical devices with chemically modified surface for clinical assays. Rsc Adv. 4 (71), 37637–37644. doi:10.1039/c4ra07112c
Dhar, B. C., and Lee, N. Y. (2018). Lab-on-a-chip technology for environmental monitoring of microorganisms. BioChip J. 12 (3), 173–183. doi:10.1007/s13206-018-2301-5
Dias, I. M., Cardoso, T. M. G., Coltro, W. K. T., and Urban, R. C. (2019). Paper-based analytical devices with colorimetric detection for determining levoglucosan in atmospheric particulate matter. Atmos. Environ. 213, 463–469. [Internet]Available from:. doi:10.1016/j.atmosenv.2019.06.040
Ding, R., Cheong, Y. H., Zhao, K., and Lisak, G. (2021). Acidified paper substrates for microfluidic solution sampling integrated with potentiometric sensors for determination of heavy metals. Sensors Actuators B Chem. 347, 130567. [Internet]Available from:. doi:10.1016/j.snb.2021.130567
Ding, R., and Lisak, G. (2019). Sponge-based microfluidic sampling for potentiometric ion sensing. Anal. Chim. Acta 1091, 103–111. doi:10.1016/j.aca.2019.09.024
Dixon, C. (2020). Printed digital microfluidics for diagnosis of disease. Canada: University of Toronto.
Dornelas, K. L., Dossi, N., and Piccin, E. (2015). A simple method for patterning poly (dimethylsiloxane) barriers in paper using contact-printing with low-cost rubber stamps. Anal. Chim. Acta 858, 82–90. doi:10.1016/j.aca.2014.11.025
Dou, M., Sanjay, S. T., Benhabib, M., Xu, F., and Li, X. (2015). Low-cost bioanalysis on paper-based and its hybrid microfluidic platforms. Talanta 145, 43–54. [Internet]Available from:. doi:10.1016/j.talanta.2015.04.068
Duford, D. A., Xi, Y., and Salin, E. D. (2013). Enzyme inhibition-based determination of pesticide residues in vegetable and soil in centrifugal microfluidic devices. Anal. Chem. 85 (16), 7834–7841. Available from. doi:10.1021/ac401416w
El Seoud, O. A., and Heinze, T. (2005). Organic esters of cellulose: New perspectives for old polymers. Polysaccharides I 186, 103–149. doi:10.1007/b136818
Elgohary, A., Block, E., Squier, J., Koneshloo, M., Shaha, R. K., Frick, C., et al. (2020). Fabrication of sealed sapphire microfluidic devices using femtosecond laser micromachining. Appl. Opt. 59 (30), 9285–9291. doi:10.1364/ao.400184
Enders, A., Siller, I. G., Urmann, K., Hoffmann, M. R., and Bahnemann, J. (2019). 3D printed microfluidic mixers—a comparative study on mixing unit performances. Small 15 (2), 1804326. doi:10.1002/smll.201804326
Evans, E., Gabriel, E. F. M., Coltro, W. K. T., and Garcia, C. D. (2014). Rational selection of substrates to improve color intensity and uniformity on microfluidic paper-based analytical devices. Analyst 139 (9), 2127–2132. doi:10.1039/c4an00230j
Fallahi, H., Zhang, J., Phan, H-P., and Nguyen, N-T. (2019). Flexible microfluidics: Fundamentals, recent developments, and applications. Micromachines 10 (12), 830. doi:10.3390/mi10120830
Fan, Y., Liu, Y., Li, H., and Foulds, I. G. (2012). Printed wax masks for 254 nm deep-UV pattering of PMMA-based microfluidics. J. Micromechanics Microengineering 22 (2), 027001. doi:10.1088/0960-1317/22/2/027001
Fan, Y., Wang, H., Liu, S., Zhang, B., and Zhang, Y. (2018). Milk carton with integrated paper-based microfluidics for milk quality rapid test. J. Food Saf. 38 (6), e12548. doi:10.1111/jfs.12548
Fan, Y. (2018). Low-cost microfluidics: Materials and methods. Micro & Nano Lett. 13 (10), 1367–1372. doi:10.1049/mnl.2018.5169
Farajikhah, S., Cabot, J. M., Innis, P. C., Paull, B., and Wallace, G. (2019). Life-saving threads: Advances in textile-based analytical devices. ACS Comb. Sci. 21 (4), 229–240. doi:10.1021/acscombsci.8b00126
Faustino, V., Catarino, S. O., Lima, R., and Minas, G. (2016). Biomedical microfluidic devices by using low-cost fabrication techniques: A review. J. Biomechanics 49 (11), 2280–2292. [Internet]Available from:. doi:10.1016/j.jbiomech.2015.11.031
Fenger, J. (1999). Urban air quality. Atmos. Environ. 33 (29), 4877–4900. doi:10.1016/s1352-2310(99)00290-3
Focke, M., Kosse, D., Müller, C., Reinecke, H., Zengerle, R., and von Stetten, F. (2010). Lab-on-a-Foil: Microfluidics on thin and flexible films. Lab. Chip 10 (11), 1365–1386. doi:10.1039/c001195a
Fu, L-M., and Wang, Y-N. (2018). Detection methods and applications of microfluidic paper-based analytical devices. TrAC Trends Anal. Chem. 107, 196–211. doi:10.1016/j.trac.2018.08.018
Fu, S., Zuo, P., and Ye, B. (2021). A Novel Wick Like Paper Based Microfluidic Device for 3D Cell Culture and Anti Cancer Drugs Screening. Biotechnol. J 16, 2000126.
Gale, B. K., Jafek, A. R., Lambert, C. J., Goenner, B. L., Moghimifam, H., Nze, U. C., et al. (2018). A review of current methods in microfluidic device fabrication and future commercialization prospects. Inventions 3, 60. doi:10.3390/inventions3030060
Gao, B., Li, X., Yang, Y., Chu, J., and He, B. (2019). Emerging paper microfluidic devices. Analyst 144 (22), 6497–6511. doi:10.1039/c9an01275c
Gao, B., Yang, Y., Liao, J., He, B., and Liu, H. (2019). Bioinspired multistructured paper microfluidics for POCT. Lab. Chip 19 (21), 3602–3608. doi:10.1039/c9lc00907h
Gao, Y., Wu, M., Lin, Y., and Xu, J. (2020). Acoustic microfluidic separation techniques and bioapplications: A review. Micromachines 11 (10), 921. doi:10.3390/mi11100921
Gerstl, F., Pongkitdachoti, U., Unob, F., and Baeumner, A. J. (2022). Miniaturized sensor for electroanalytical and electrochemiluminescent detection of pathogens enabled through laser-induced graphene electrodes embedded in microfluidic channels. Lab. Chip 22, 3721–3733. doi:10.1039/d2lc00593j
Glavan, A. C., Martinez, R. V., Maxwell, E. J., Subramaniam, A. B., Nunes, R. M. D., Soh, S., et al. (2013). Rapid fabrication of pressure-driven open-channel microfluidic devices in omniphobic RF paper. Lab. Chip 13 (15), 2922–2930. doi:10.1039/c3lc50371b
Gonçalves, I. M., Madureira, M., Miranda, I., Schütte, H., Moita, A., Minas, G., et al. (2021). Separation microfluidic device fabricated by micromilling techniques. Eng. Proc. 4 (1), 37.
Gonzalez, G., Chiappone, A., Dietliker, K., Pirri, C. F., and Roppolo, I. (2020). Fabrication and functionalization of 3D printed polydimethylsiloxane-based microfluidic devices obtained through digital light processing. Adv. Mater Technol. 5 (9), 2000374. doi:10.1002/admt.202000374
Gosset, A., Durrieu, C., Renaud, L., Deman, A-L., Barbe, P., Bayard, R., et al. (2018). Xurography-based microfluidic algal biosensor and dedicated portable measurement station for online monitoring of urban polluted samples. Biosens. Bioelectron. 117, 669–677. doi:10.1016/j.bios.2018.07.005
Guckenberger, D. J., de Groot, T. E., Wan, A. M. D., Beebe, D. J., and Young, E. W. K. (2015). Micromilling: A method for ultra-rapid prototyping of plastic microfluidic devices. Lab. Chip 15 (11), 2364–2378. doi:10.1039/c5lc00234f
Guo, W., Hansson, J., Gustafsson, L., and van der Wijngaart, W. (2021). “Bend-and-Bond” polymer microfluidic origami,” in 2021 IEEE 34th international conference on micro electro mechanical systems (MEMS) (IEEE), 222–225.
Guo, X-L., Chen, Y., Jiang, H-L., Qiu, X-B., and Yu, D-L. (2018). Smartphone-based microfluidic colorimetric sensor for gaseous formaldehyde determination with high sensitivity and selectivity. Sensors 18 (9), 3141. [Internet]Available from:. doi:10.3390/s18093141
Hacker, M. C., Krieghoff, J., and Mikos, A. G. (2019). “Synthetic polymers,” in Principles of regenerative medicine (Elsevier), 559–590.
Hamilton, A. J., Stagnitti, F., Premier, R., Boland, A-M., and Hale, G. (2006). Quantitative microbial risk assessment models for consumption of raw vegetables irrigated with reclaimed water. Appl. Environ. Microbiol. 72 (5), 3284–3290. doi:10.1128/aem.72.5.3284-3290.2006
Haubert, K., Drier, T., and Beebe, D. (2006). PDMS bonding by means of a portable, low-cost corona system. Lab. Chip 6 (12), 1548–1549. doi:10.1039/b610567j
He, Y., Wu, Y., Fu, J. Z., Gao, Q., and Qiu, J. J. (2016). Developments of 3D printing microfluidics and applications in chemistry and biology: A review. Electroanalysis 28 (8), 1658–1678. [Internet]Available from. doi:10.1002/elan.201600043
He, Y., Wu, Y., Fu, J-Z., and Wu, W-B. (2015). Fabrication of paper-based microfluidic analysis devices: A review. Rsc Adv. 5 (95), 78109–78127. doi:10.1039/c5ra09188h
Heuer, C., Preuß, J., Habib, T., Enders, A., and Bahnemann, J. (2021). 3D printing in biotechnology—an insight into miniaturized and microfluidic systems for applications from cell culture to bioanalytics. London. Eng. Life Sci. doi:10.1002/elsc.202100081
Hong Tham Phan, T., and Kim, S-J. (2022). Super-hydrophobic microfluidic channels fabricated via xurography-based polydimethylsiloxane (PDMS) micromolding. Chem. Eng. Sci. 258, 117768. doi:10.1016/j.ces.2022.117768
Hossain, M. M., and Rahman, T. (2018). Low cost micro milling machine for prototyping plastic microfluidic devices. Multidiscip. Digit. Publ. Inst. Proc. 2 (13), 707.
Hu, K., Ma, L., Wang, Z., Fernandez-Delgado, O., Garay, Y. E., Lopez, J. A., et al. (2022). Facile synthesis and integration of poly (vinyl alcohol) sponge-supported metal nanocatalysts on a microfluidic chip enable a new continuous flow multireactor nanocatalysis platform for high efficiency and reusability catalysis. ACS Sustain. Chem. Eng. 10 (32), 10579–10589. doi:10.1021/acssuschemeng.2c02060
Huang, W-H., Mai, V-P., Wu, R-Y., Yeh, K-L., and Yang, R-J. (2021). A microfluidic aptamer-based sensor for detection of mercury(II) and lead(II) ions in water. Micromachines 12, 1283. doi:10.3390/mi12111283
Idros, N., and Chu, D. (2018). Triple-indicator-based multidimensional colorimetric sensing platform for heavy metal ion detections. ACS Sensors 3 (9), 1756–1764. [Internet]Available from. doi:10.1021/acssensors.8b00490
Islam, M. M., Loewen, A., and Allen, P. B. (2018). Simple, low-cost fabrication of acrylic based droplet microfluidics and its use to generate DNA-coated particles. Sci. Rep. 8 (1), 8763–8774. doi:10.1038/s41598-018-27037-5
Jarujamrus, P., Meelapsom, R., Pencharee, S., Obma, A., Amatatongchai, M., Ditcharoen, N., et al. (2018). Use of a smartphone as a colorimetric analyzer in paper-based devices for sensitive and selective determination of mercury in water samples. Anal. Sci. 34 (1), 75–81. doi:10.2116/analsci.34.75
Jarujamrus, P., Prakobkij, A., Puchum, S., Chaisamdaeng, S., Meelapsom, R., Anutrasakda, W., et al. (2020). Acid–base titration using a microfluidic thread-based analytical device (μTAD). Analyst 145 (13), 4457–4466. doi:10.1039/d0an00522c
Javidanbardan, A., Azevedo, A. M., Chu, V., and Conde, J. P. (2021). A systematic approach for developing 3D high-quality PDMS microfluidic chips based on micromilling technology. Micromachines 13 (1), 6. doi:10.3390/mi13010006
Jaywant, S. A., and Arif, K. M. (2019). A comprehensive review of microfluidic water quality monitoring sensors. Sensors 19 (21), 4781. doi:10.3390/s19214781
Jemmeli, D., Marcoccio, E., Moscone, D., Dridi, C., and Arduini, F. (2020). Highly sensitive paper-based electrochemical sensor for reagent free detection of bisphenol A. Talanta 216, 120924. [Internet]Available from:. doi:10.1016/j.talanta.2020.120924
Jia, Y., Dong, H., Zheng, J., and Sun, H. (2017). Portable detection of trace metals in airborne particulates and sediments via μPADs and smartphone. Biomicrofluidics 11 (6), 064101. [Internet]Available from. doi:10.1063/1.5003308
Jia, Y., Jiang, J., Ma, X., Li, Y., Huang, H., Cai, K., et al. (2008). PDMS microchannel fabrication technique based on microwire-molding. Sci. Bull. 53 (24), 3928–3936. doi:10.1007/s11434-008-0528-6
Jiang, J., Wu, H., Su, Y., Liang, Y., Shu, B., and Zhang, C. (2020). Electrochemical cloth-based dna sensors (ECDSs): A new class of electrochemical gene sensors. Anal. Chem. 92 (11), 7708–7716. doi:10.1021/acs.analchem.0c00669
Jiménez-Díaz, E., Cano-Jorge, M., Zamarrón-Hernández, D., Cabriales, L., Páez-Larios, F., Cruz-Ramírez, A., et al. (2019). Micro–Macro: Selective integration of microfeatures inside low-cost macromolds for PDMS microfluidics fabrication. Micromachines 10 (9), 576. doi:10.3390/mi10090576
Jin, Y., Xiong, P., Xu, T., and Wang, J. (2022). Time-efficient fabrication method for 3D-printed microfluidic devices. Sci. Rep. 12 (1), 1233. [Internet]Available from. doi:10.1038/s41598-022-05350-4
Jokerst, J. C., Emory, J. M., and Henry, C. S. (2012). Advances in microfluidics for environmental analysis. Analyst 137 (1), 24–34. doi:10.1039/c1an15368d
Kamnoet, P., Aeungmaitrepirom, W., Menger, R. F., and Henry, C. S. (2021). Highly selective simultaneous determination of Cu(ii), Co(ii), Ni(ii), Hg(ii), and Mn(ii) in water samples using microfluidic paper-based analytical devices. Analyst 146 (7), 2229–2239. [Internet]Available from. doi:10.1039/D0AN02200D
Kaneta, T., Alahmad, W., and Varanusupakul, P. (2019). Microfluidic paper-based analytical devices with instrument-free detection and miniaturized portable detectors. Appl. Spectrosc. Rev. 54 (2), 117–141. doi:10.1080/05704928.2018.1457045
Khorsandi, D., Nodehi, M., Waqar, T., Shabani, M., Kamare, B., Zare, E. N., et al. (2021). Manufacturing of microfluidic sensors utilizing 3d printing technologies: A production system. J. Nanomater. 2021, 1–16. doi:10.1155/2021/5537074
Khoshbin, Z., Housaindokht, M. R., and Verdian, A. (2020). A low-cost paper-based aptasensor for simultaneous trace-level monitoring of mercury (II) and silver (I) ions. Anal. Biochem. 597, 113689. [Internet]Available from:. doi:10.1016/j.ab.2020.113689
Kinuthia, G. K., Ngure, V., Beti, D., Lugalia, R., Wangila, A., and Kamau, L. (2020). Levels of heavy metals in wastewater and soil samples from open drainage channels in nairobi, Kenya: Community health implication. Sci. Rep. 10 (1), 8434. [Internet]Available from. doi:10.1038/s41598-020-65359-5
Komolafe, A. A., Adegboyega, S. A-A., Anifowose, A. Y. B., Akinluyi, F. O., and Awoniran, D. R. (2014). Air pollution and climate change in lagos, Nigeria: Needs for proactive approaches to risk management and adaptation. Am. J. Environ. Sci. 10 (4), 412–423. doi:10.3844/ajessp.2014.412.423
Kosoff, D., Yu, J., Suresh, V., Beebe, D. J., and Lang, J. M. (2018). Surface topography and hydrophilicity regulate macrophage phenotype in milled microfluidic systems. Lab. Chip 18 (19), 3011–3017. doi:10.1039/c8lc00431e
Kotz, F., Mader, M., Dellen, N., Risch, P., Kick, A., Helmer, D., et al. (2020). Fused deposition modeling of microfluidic chips in polymethylmethacrylate. Micromachines 11 (9), 873. doi:10.3390/mi11090873
Ku, X., Zhang, Z., Liu, X., Chen, L., and Li, G. (2018). Low-cost rapid prototyping of glass microfluidic devices using a micromilling technique. Microfluid. Nanofluidics 22 (8), 82–88. doi:10.1007/s10404-018-2104-y
Kumar Gupta, P., Sai Raghunath, S., Venkatesh Prasanna, D., Venkat, P., Shree, V., Chithananthan, C., et al. (2019). An update on overview of cellulose, its structure and applications. Cellulose 201 (9). doi:10.5772/intechopen.84727
Kung, C-T., Hou, C-Y., Wang, Y-N., and Fu, L-M. (2019). Microfluidic paper-based analytical devices for environmental analysis of soil, air, ecology and river water. Sensors Actuators B Chem. 301, 126855. doi:10.1016/j.snb.2019.126855
Kuo, J. T. W., Kim, B. J., Hara, S. A., Lee, C. D., Gutierrez, C. A., Hoang, T. Q., et al. (2013). Novel flexible Parylene neural probe with 3D sheath structure for enhancing tissue integration. Lab. Chip 13 (4), 554–561. doi:10.1039/c2lc40935f
L Santana, Á., and Angela A Meireles, M. (2014). New starches are the trend for industry applications: A review. Food Public Health 4 (5), 229–241. doi:10.5923/j.fph.20140405.04
Lau, W. W. Y., Shiran, Y., Bailey, R. M., Cook, E., Stuchtey, M. R., Koskella, J., et al. (2020). Evaluating scenarios toward zero plastic pollution. Science 369 (6510), 1455–1461. doi:10.1126/science.aba9475
Leclerc, C. (2021). Experimental and computational analyses of a microfluidic chip fabricated through computer numerical control micromilling of stressed polystyrene sheets. Guelph, ON: University of Guelph.
Li, H., Liu, C., Wang, D., and Zhang, C. (2018). Programmable fluid transport on photolithographically micropatterned cloth devices: Towards the development of facile, multifunctional colorimetric diagnostic platforms. Sensors Actuators B Chem. 255, 2416–2430. doi:10.1016/j.snb.2017.08.215
Li, J. M., Liu, C., Qiao, H. C., Zhu, L. Y., Chen, G., and Dai, X. D. (2007). Hot embossing/bonding of a poly (ethylene terephthalate)(PET) microfluidic chip. J. Micromechanics Microengineering 18 (1), 015008. doi:10.1088/0960-1317/18/1/015008
Li, M., Li, S., Wu, J., Wen, W., Li, W., and Alici, G. (2012). A simple and cost-effective method for fabrication of integrated electronic-microfluidic devices using a laser-patterned PDMS layer. Microfluid. Nanofluidics 12 (5), 751–760. [Internet]Available from. doi:10.1007/s10404-011-0917-z
Li, Q., Yuan, Z., Zhang, C., Hu, S., Chen, Z., Wu, Y., et al. (2022). Tough, highly oriented, super thermal insulating regenerated all-cellulose sponge-aerogel fibers integrating a graded aligned nanostructure. Nano Lett. 22 (9), 3516–3524. doi:10.1021/acs.nanolett.1c03943
Li, W., Zhang, X., Li, T., Ji, Y., and Li, R. (2021). Molecularly imprinted polymer-enhanced biomimetic paper-based analytical devices: A review. Anal. Chim. Acta 1148, 238196. doi:10.1016/j.aca.2020.12.071
Lim, H., Jafry, A. T., and Lee, J. (2019). Fabrication, flow control, and applications of microfluidic paper-based analytical devices. Molecules 24 (16), 2869. doi:10.3390/molecules24162869
Lin, D., Li, B., Fu, L., Qi, J., Xia, C., Zhang, Y., et al. (2022). A novel polymer-based nitrocellulose platform for implementing a multiplexed microfluidic paper-based enzyme-linked immunosorbent assay. Microsystems Nanoeng. 8 (1), 53–10. doi:10.1038/s41378-022-00385-z
Lin, D., Li, B., Qi, J., Ji, X., Yang, S., Wang, W., et al. (2020). Low cost fabrication of microfluidic paper-based analytical devices with water-based polyurethane acrylate and their application for bacterial detection. Sensors Actuators B Chem. 303, 127213. [Internet]Available from:. doi:10.1016/j.snb.2019.127213
Lin, H., Zhao, Y., Lin, S., Wang, B., Yeung, C., Cheng, X., et al. (2019). A rapid and low-cost fabrication and integration scheme to render 3D microfluidic architectures for wearable biofluid sampling, manipulation, and sensing. Lab. Chip 19 (17), 2844–2853. doi:10.1039/c9lc00418a
Lin, S., Yu, Z., Chen, D., Wang, Z., Miao, J., Li, Q., et al. (2020). Progress in microfluidics-based exosome separation and detection technologies for diagnostic applications. Small 16 (9), 1903916. doi:10.1002/smll.201903916
Lin, Y., Gao, Y., Wu, M., Zhou, R., Chung, D., Caraveo, G., et al. (2019). Acoustofluidic stick-and-play micropump built on foil for single-cell trapping. Lab. Chip 19 (18), 3045–3053. doi:10.1039/c9lc00484j
Lin, Y., Gritsenko, D., Feng, S., Teh, Y. C., Lu, X., and Xu, J. (2016). Detection of heavy metal by paper-based microfluidics. Biosens. Bioelectron. 83, 256–266. doi:10.1016/j.bios.2016.04.061
Liu, J., Kong, X., Wang, H., Zhang, Y., and Fan, Y. (2020). Roll-to-roll wax transfer for rapid and batch fabrication of paper-based microfluidics. Microfluid. Nanofluidics 24 (1), 6–7. doi:10.1007/s10404-019-2310-2
Liu, J., Zhang, B., Zhang, Y., and Fan, Y. (2021). Fluid control with hydrophobic pillars in paper-based microfluidics. J. Micromechanics Microengineering 31 (12), 127002. doi:10.1088/1361-6439/ac35c9
Liu, M., Zhang, C., and Liu, F. (2015). Understanding wax screen-printing: A novel patterning process for microfluidic cloth-based analytical devices. Anal. Chim. Acta 891, 234–246. doi:10.1016/j.aca.2015.06.034
Lohse, D. (2022). Fundamental fluid dynamics challenges in inkjet printing. Annu. Rev. Fluid Mech. 54, 349–382. [Internet]Available from. doi:10.1146/annurev-fluid-022321-114001
Loo, J. F. C., Ho, A. H. P., Turner, A. P. F., and Mak, W. C. (2019). Integrated printed microfluidic biosensors. Trends Biotechnol. 37 (10), 1104–1120. doi:10.1016/j.tibtech.2019.03.009
Lopez-Ruiz, N., Curto, V. F., Erenas, M. M., Benito-Lopez, F., Diamond, D., Palma, A. J., et al. (2014). Smartphone-based simultaneous pH and nitrite colorimetric determination for paper microfluidic devices. Anal. Chem. 86 (19), 9554–9562. doi:10.1021/ac5019205
Ma, J., Yan, S., Miao, C., Li, L., Shi, W., Liu, X., et al. (2019). Paper microfluidics for cell analysis. Adv. Healthc. Mater. 8 (1), 1801084. doi:10.1002/adhm.201801084
Ma, X., Li, R., Jin, Z., Fan, Y., Zhou, X., and Zhang, Y. (2020). Injection molding and characterization of PMMA-based microfluidic devices. Microsyst. Technol. 26 (4), 1317–1324. doi:10.1007/s00542-019-04662-2
Madureira, M., Faustino, V., Schütte, H., Pinho, D., Minas, G., Gassmann, S., et al. (2019). “Red blood cells separation in a curved T-shaped microchannel fabricated by a micromilling technique,” in ECCOMAS thematic conference on computational vision and medical image processing (Springer), 585–593.
Maejima, K., Tomikawa, S., Suzuki, K., and Citterio, D. (2013). Inkjet printing: An integrated and green chemical approach to microfluidic paper-based analytical devices. RSC Adv. 3 (24), 9258–9263. [Internet]Available from. doi:10.1039/C3RA40828K
Mahaqi, A., Mehiqi, M., Moheghy, M. A., Moheghi, M. M., and Hussainzadeh, J. (2021). Nitrate pollution in kabul water supplies, Afghanistan; sources and chemical reactions: A review. Int. J. Environ. Sci. Technol. 19, 6925–6934. doi:10.1007/s13762-021-03551-4
Mahmud, M. A., Blondeel, E. J. M., Kaddoura, M., and MacDonald, B. D. (2018). Features in microfluidic paper-based devices made by laser cutting: How small can they be? Micromachines 9 (5), 220. doi:10.3390/mi9050220
Maitz, M. F. (2015). Applications of synthetic polymers in clinical medicine. Biosurface Biotribology 1 (3), 161–176. doi:10.1016/j.bsbt.2015.08.002
Mako, T. L., Levenson, A. M., and Levine, M. (2020). Ultrasensitive detection of nitrite through implementation of N-(1-Naphthyl)ethylenediamine-Grafted cellulose into a paper-based device. ACS Sensors 5 (4), 1207–1215. [Internet]Available from. doi:10.1021/acssensors.0c00291
Manisha, H., Priya Shwetha, P. D., and Prasad, K. S. (2018). “Low-cost paper analytical devices for environmental and biomedical sensing applications,” in Environmental, chemical and medical sensors (Springer), 315–341.
Mao, X., and Huang, T. J. (2012). Microfluidic diagnostics for the developing world. Lab. Chip 12 (8), 1412–1416. doi:10.1039/c2lc90022j
Mao, X., and Zhang, C. (2022). A microfluidic cloth-based photoelectrochemical analytical device for the detection of glucose in saliva. Talanta 238, 123052. [Internet]Available from:. doi:10.1016/j.talanta.2021.123052
Mark, J., Ngai, K., Graessley, W., Mandelkern, L., Samulski, E., Wignall, G., et al. (2004). Physical properties of polymers. Cambridge University Press.
Martinez, A. W., Phillips, S. T., Butte, M. J., and Whitesides, G. M. (2007). Patterned paper as a platform for inexpensive, low-volume, portable bioassays. Angew. Chem. 46 (8), 1318–1320. doi:10.1002/anie.200603817
Martínez-López, J. I., Mojica, M., Rodríguez, C. A., and Siller, H. R. (2016). Xurography as a rapid fabrication alternative for point-of-care devices: Assessment of passive micromixers. Sensors 16 (5), 705. [Internet]Available from:. doi:10.3390/s16050705
McMillan, A. H., Thomée, E. K., Dellaquila, A., Nassman, H., Segura, T., and Lesher-Pérez, S. C. (2020). Rapid fabrication of membrane-integrated thermoplastic elastomer microfluidic devices. Micromachines 11 (8), 731. doi:10.3390/mi11080731
McNeely, M. R., Spute, M. K., Tusneem, N. A., and Oliphant, A. R. (1999). “Hydrophobic microfluidics,” in Microfluidic devices and systems II. (Santa Clara, CA: International Society for Optics and Photonics), 210–220.
Mehta, V., and Rath, S. N. (2021). 3D printed microfluidic devices: A review focused on four fundamental manufacturing approaches and implications on the field of healthcare. Bio-Design Manuf. 4 (2), 311–343. [Internet]Available from. doi:10.1007/s42242-020-00112-5
Mesquita, P., Gong, L., and Lin, Y. (2022). A low-cost microfluidic method for microplastics identification: Towards continuous recognition. Micromachines 13 (4), 499. doi:10.3390/mi13040499
Miranda, I., Souza, A., Sousa, P., Ribeiro, J., Castanheira, E. M. S., Lima, R., et al. (2021). Properties and applications of PDMS for biomedical engineering: A review. J. Funct. Biomaterials 13 (1), 2. doi:10.3390/jfb13010002
Modha, S., Castro, C., and Tsutsui, H. (2021). Recent developments in flow modeling and fluid control for paper-based microfluidic biosensors. Biosens. Bioelectron. 178, 113026. doi:10.1016/j.bios.2021.113026
Mogera, U., Guo, H., Namkoong, M., Rahman, M. S., Nguyen, T., and Tian, L. (2022). Wearable plasmonic paper–based microfluidics for continuous sweat analysis. Sci. Adv. 8 (12), eabn1736. [Internet]eabn1736. Available from. doi:10.1126/sciadv.abn1736
Mohammed, M. I., Zainal Alam, M. N. H., Kouzani, A., and Gibson, I. (2016). Fabrication of microfluidic devices: Improvement of surface quality of CO2laser machined poly(methylmethacrylate) polymer. J. Micromechanics Microengineering 27 (1), 015021. [Internet]Available from. doi:10.1088/0960-1317/27/1/015021
Morbioli, G. G., Mazzu-Nascimento, T., Stockton, A. M., and Carrilho, E. (2019). “How are these devices manufactured?,” in Paper-based diagnostics (Springer), 89–122.
Morbioli, G. G., Mazzu-Nascimento, T., Stockton, A. M., and Carrilho, E. (2017). Technical aspects and challenges of colorimetric detection with microfluidic paper-based analytical devices (μPADs) - a review. Anal. Chim. Acta 970, 1–22. [Internet]Available from:. doi:10.1016/j.aca.2017.03.037
Moreau, W. M. (2012). Semiconductor lithography: Principles, practices, and materials. Springer Science & Business Media.
Nguyen, T-Q., Mah, J., Park, W-T., and Lee, S. (2019). “Rapid and versatile micromold fabrication using micromilling and nanopolishing for microfluidic devices,” in Fluids engineering division summer meeting (American Society of Mechanical Engineers (ASME)), V004T06A011.
Niculescu, A-G., Chircov, C., Bîrcă, A. C., and Grumezescu, A. M. (2021). Fabrication and applications of microfluidic devices: A review. Int. J. Mol. Sci. 22 (4), 2011. doi:10.3390/ijms22042011
Nielsen, A. V., Beauchamp, M. J., Nordin, G. P., and Woolley, A. T. (2020). 3D printed microfluidics. Annu. Rev. Anal. Chem. 13, 45–65. doi:10.1146/annurev-anchem-091619-102649
Nielsen, J. B., Hanson, R. L., Almughamsi, H. M., Pang, C., Fish, T. R., and Woolley, A. T. (2019). Microfluidics: Innovations in materials and their fabrication and functionalization. Anal. Chem. 92 (1), 150–168. doi:10.1021/acs.analchem.9b04986
Nightingale, A. M., Beaton, A. D., and Mowlem, M. C. (2015). Trends in microfluidic systems for in situ chemical analysis of natural waters. Sensors Actuators B Chem. 221, 1398–1405. doi:10.1016/j.snb.2015.07.091
Nilghaz, A., Bagherbaigi, S., Lam, C. L., Mousavi, S. M., Cόrcoles, E. P., and Wicaksono, D. H. B. (2015). Multiple semi-quantitative colorimetric assays in compact embeddable microfluidic cloth-based analytical device (μCAD) for effective point-of-care diagnostic. Microfluid. Nanofluidics 19 (2), 317–333. doi:10.1007/s10404-015-1545-9
Nilghaz, A., Ballerini, D. R., and Shen, W. (2013). Exploration of microfluidic devices based on multi-filament threads and textiles: A review. Biomicrofluidics 7 (5), 051501. [Internet]Available from. doi:10.1063/1.4820413
Nilghaz, A., Zhang, L., Li, M., Ballerini, D. R., and Shen, W. (2014). Understanding thread properties for red blood cell antigen assays: Weak ABO blood typing. ACS Appl. Mater Interfaces 6 (24), 22209–22215. doi:10.1021/am505849e
Nishat, S., Jafry, A. T., Martinez, A. W., and Awan, F. R. (2021). Paper-based microfluidics: Simplified fabrication and assay methods. Sensors Actuators B Chem. 336, 129681. [Internet]Available from:. doi:10.1016/j.snb.2021.129681
Noviana, E., Carrão, D. B., Pratiwi, R., and Henry, C. S. (2020). Emerging applications of paper-based analytical devices for drug analysis: A review. Anal. Chim. Acta 1116, 70–90. [Internet]Available from:. doi:10.1016/j.aca.2020.03.013
Oh, J. M., Begum, H. M., Liu, Y. L., Ren, Y., and Shen, K. (2022). Recapitulating tumor hypoxia in a cleanroom-free, liquid-pinning-based microfluidic tumor model. ACS Biomater. Sci. Eng. 8 (7), 3107–3121. [Internet]Available from. doi:10.1021/acsbiomaterials.2c00207
Oliveira, A. C. M., Araújo, D. A. G., Pradela-Filho, L. A., Takeuchi, R. M., Trindade, M. A. G., and Dos Santos, A. L. (2022). Threads in tubing: An innovative approach towards improved electrochemical thread-based microfluidic devices. Lab. Chip 22 (16), 3045–3054. doi:10.1039/d2lc00387b
Olkkonen, J., Lehtinen, K., and Erho, T. (2010). Flexographically printed fluidic structures in paper. Anal. Chem. 82 (24), 10246–10250. doi:10.1021/ac1027066
Olmos, C. M., Vaca, A., Rosero, G., Peñaherrera, A., Perez, C., de Sá Carneiro, I., et al. (2019). Epoxy resin mold and PDMS microfluidic devices through photopolymer flexographic printing plate. Sensors Actuators B Chem. 288, 742–748. doi:10.1016/j.snb.2019.03.062
Owens, C. E., and Hart, A. J. (2018). High-precision modular microfluidics by micromilling of interlocking injection-molded blocks. Lab. Chip 18 (6), 890–901. doi:10.1039/c7lc00951h
Patrício Silva, A. L., Prata, J. C., Walker, T. R., Duarte, A. C., Ouyang, W., Barcelò, D., et al. (2021). Increased plastic pollution due to COVID-19 pandemic: Challenges and recommendations. Chem. Eng. J. 405, 126683. doi:10.1016/j.cej.2020.126683
Pena-Pereira, F., Bendicho, C., Pavlović, D. M., Martín-Esteban, A., Díaz-Álvarez, M., Pan, Y., et al. (2021). Miniaturized analytical methods for determination of environmental contaminants of emerging concern–a review. Anal. Chim. Acta 1158, 238108. doi:10.1016/j.aca.2020.11.040
Persson, H., Park, S., Mohan, M., Cheung, K. K., Simmons, C. A., and Young, E. W. K. (2022). Rapid assembly of PMMA microfluidic devices with PETE membranes for studying the endothelium. Sensors Actuators B Chem. 356, 131342. [Internet]Available from:. doi:10.1016/j.snb.2021.131342
Peters, J. J., Almeida, M. G., O'Connor Šraj, L., McKelvie, I. D., and Kolev, S. D. (2019). Development of a micro-distillation microfluidic paper-based analytical device as a screening tool for total ammonia monitoring in freshwaters. Anal. Chim. Acta 1079, 120–128. [Internet]Available from:. doi:10.1016/j.aca.2019.05.050
Podgorski, J., and Berg, M. (2020). Global threat of arsenic in groundwater. Science 368 (6493), 845–850. doi:10.1126/science.aba1510
Poenar, D. P. (2019). Microfluidic and micromachined/MEMS devices for separation, discrimination and detection of airborne particles for pollution monitoring. Micromachines 10 (7), 483. [Internet]Available from:. doi:10.3390/mi10070483
Pol, R., Céspedes, F., Gabriel, D., and Baeza, M. (2017). Microfluidic lab-on-a-chip platforms for environmental monitoring. TrAC Trends Anal. Chem. 95, 62–68. doi:10.1016/j.trac.2017.08.001
Pollard, M., Hunsicker, E., and Platt, M. (2020). A tunable three-dimensional printed microfluidic resistive pulse sensor for the characterization of algae and microplastics. ACS Sensors 5 (8), 2578–2586. [Internet]Available from:. doi:10.1021/acssensors.0c00987
Pouyanfar, N., Harofte, S. Z., Soltani, M., Siavashy, S., Asadian, E., Ghorbani-Bidkorbeh, F., et al. (2022). Artificial intelligence-based microfluidic platforms for the sensitive detection of environmental pollutants: Recent advances and prospects. Trends Environ. Anal. Chem. 34, e00160. Available from:. doi:10.1016/j.teac.2022.e00160
Prabowo, B. A., Fernandes, E., and Freitas, P. (2022). A pump-free microfluidic device for fast magnetic labeling of ischemic stroke biomarkers. Anal. Bioanal. Chem. 414 (8), 2571–2583. [Internet]Available from:. doi:10.1007/s00216-022-03915-w
Pranzo, D., Larizza, P., Filippini, D., and Percoco, G. (2018). Extrusion-based 3D printing of microfluidic devices for chemical and biomedical applications: A topical review. Micromachines 9 (8), 374. doi:10.3390/mi9080374
Qian, S., Han, Y., Xu, F., Feng, D., Yang, X., Wu, X., et al. (2022). A fast, sensitive, low-cost electrochemical paper-based chip for real-time simultaneous detection of cadmium (Ⅱ) and lead (Ⅱ) via aptamer. Talanta 247, 123548. [Internet]Available from:. doi:10.1016/j.talanta.2022.123548
Quinn, C. W., Cate, D. M., Miller-Lionberg, D. D., Reilly, T., Volckens, J., and Henry, C. S. (2018). Solid-phase extraction coupled to a paper-based technique for trace copper detection in drinking water. Environ. Sci. Technol. 52 (6), 3567–3573. [Internet]Available from. doi:10.1021/acs.est.7b05436
Racicot, J. M., Mako, T. L., Olivelli, A., and Levine, M. (2020). A paper-based device for ultrasensitive, colorimetric phosphate detection in seawater. Sensors 20 (10), 2766. [Internet]Available from:. doi:10.3390/s20102766
Rahim, M. S., and Ehsan, A. A. (2021). Micro milling process for the rapid prototyping of microfluidic devices. Adv. Microfluid Nanofluids 1, 81–92. doi:10.5772/intechopen.91560
Rai-Choudhury, P. (1997). Handbook of microlithography, micromachining, and microfabrication: Microlithography, 1. Bellingham, WA: SPIE press.
Raj M, K., and Chakraborty, S. (2020). PDMS microfluidics: A mini review. J. Appl. Polym. Sci. 137 (27), 48958. doi:10.1002/app.48958
Raoufi, M. A., Razavi Bazaz, S., Niazmand, H., Rouhi, O., Asadnia, M., Razmjou, A., et al. (2020). Fabrication of unconventional inertial microfluidic channels using wax 3D printing. Soft Matter 16 (10), 2448–2459. doi:10.1039/c9sm02067e
Razavi Bazaz, S., Rouhi, O., Raoufi, M. A., Ejeian, F., Asadnia, M., Jin, D., et al. (2020). 3D printing of inertial microfluidic devices. Sci. Rep. 10 (1), 5929. [Internet]Available from. doi:10.1038/s41598-020-62569-9
Ritchie, J. C., Zimba, P. V., and Everitt, J. H. (2003). Remote sensing techniques to assess water quality. Photogrammetric Eng. Remote Sens. 69 (6), 695–704. doi:10.14358/pers.69.6.695
Rizzo, P. (2010). Water and wastewater pipe nondestructive evaluation and health monitoring: A review. Adv. Civ. Eng. 2010, 1–13. doi:10.1155/2010/818597
Rose, M., and Palkovits, R. (2011). Cellulose-based sustainable polymers: State of the art and future trends. Macromol. Rapid Commun. 32 (17), 1299–1311. doi:10.1002/marc.201100230
Ruiz, C., Kadimisetty, K., Yin, K., Mauk, M. G., Zhao, H., and Liu, C. (2020). Fabrication of hard–soft microfluidic devices using hybrid 3D printing. Micromachines 11 (6), 567. doi:10.3390/mi11060567
Ruiz, R. A., Gonzalez, J. L., Vazquez-Alvarado, M., Martinez, N. W., and Martinez, A. W. (2022). Beyond wax printing: Fabrication of paper-based microfluidic devices using a thermal transfer printer. Anal. Chem. 94 (25), 8833–8837. doi:10.1021/acs.analchem.2c01534
Rumaner, M., Horowitz, L., Ovadya, A., and Folch, A. (2019). Thread as a low-cost material for microfluidic assays on intact tumor slices. Micromachines 10 (7), 481. doi:10.3390/mi10070481
Saadat, M., Taylor, M., Hughes, A., and Hajiyavand, A. M. (2020). Rapid prototyping method for 3D PDMS microfluidic devices using a red femtosecond laser. Adv. Mech. Eng. 12 (12), 168781402098271. doi:10.1177/1687814020982713
Saez, J., Catalan-Carrio, R., Owens, R. M., Basabe-Desmonts, L., and Benito-Lopez, F. (2021). Microfluidics and materials for smart water monitoring: A review. Anal. Chim. Acta 1186, 338392. [Internet]Available from:. doi:10.1016/j.aca.2021.338392
Sankar, K., Lenisha, D., Janaki, G., Juliana, J., Kumar, R. S., Selvi, M. C., et al. (2020). Digital image-based quantification of chlorpyrifos in water samples using a lipase embedded paper based device. Talanta 208, 120408. [Internet]Available from:. doi:10.1016/j.talanta.2019.120408
Santangelo, M. F., Shtepliuk, I., Filippini, D., Puglisi, D., Vagin, M., Yakimova, R., et al. (2019). Epitaxial graphene sensors combined with 3D-printed microfluidic chip for heavy metals detection. Sensors 19 (10), 2393. [Internet]Available from:. doi:10.3390/s19102393
Santos, R. G., Machovsky-Capuska, G. E., and Andrades, R. (2021). Plastic ingestion as an evolutionary trap: Toward a holistic understanding. Science 373 (6550), 56–60. doi:10.1126/science.abh0945
Saptaji, K., Triawan, F., Sai, T. K., and Gebremariam, A. (2021). Deburring method of aluminum mould produced by milling process for microfluidic device fabrication. Indonesian J. Sci. Technol. 6 (1), 123–140. doi:10.17509/ijost.v6i1.31852
Sarwar, M., Leichner, J., Naja, G. M., and Li, C-Z. (2019). Smart-phone, paper-based fluorescent sensor for ultra-low inorganic phosphate detection in environmental samples. Microsystems Nanoeng. 5 (1), 56. [Internet].Available from. doi:10.1038/s41378-019-0096-8
Scala-Benuzzi, M. L., Raba, J., Soler-Illia, G. J. A. A., Schneider, R. J., and Messina, G. A. (2018). Novel electrochemical paper-based immunocapture assay for the quantitative determination of Ethinylestradiol in water samples. Anal. Chem. 90 (6), 4104–4111. [Internet]Available from. doi:10.1021/acs.analchem.8b00028
Schaumburg, F., Carrell, C. S., and Henry, C. S. (2019). Rapid bacteria detection at low concentrations using sequential immunomagnetic separation and paper-based isotachophoresis. Anal. Chem. 91 (15), 9623–9630. [Internet]Available from. doi:10.1021/acs.analchem.9b01002
Schulze, F., Gao, X., Virzonis, D., Damiati, S., Schneider, M. R., and Kodzius, R. (2017). Air quality effects on human health and approaches for its assessment through microfluidic chips. Genes (Basel) 8 (10), 244. doi:10.3390/genes8100244
Scott, S. M., and Ali, Z. (2021). Fabrication methods for microfluidic devices: An overview. Micromachines 12 (3), 319. doi:10.3390/mi12030319
Seok, Y., Lee, J., and Kim, M-G. (2021). Paper-based airborne bacteria collection and DNA extraction kit. Biosensors 11 (10), 375. [Internet]Available from. doi:10.3390/bios11100375
Shang, Q., Su, Y., Liang, Y., Lai, W., Jiang, J., Wu, H., et al. (2022). Correction to: Ultrasensitive cloth-based microfluidic chemiluminescence detection of Listeria monocytogenes hlyA gene by hemin/G-quadruplex DNAzyme and hybridization chain reaction signal amplification. Anal. Bioanal. Chem. 414 (13), 4011. doi:10.1007/s00216-022-04042-2
Shang, Q., Su, Y., Liang, Y., Lai, W., Jiang, J., Wu, H., et al. (2020). Ultrasensitive cloth-based microfluidic chemiluminescence detection of Listeria monocytogenes hlyA gene by hemin/G-quadruplex DNAzyme and hybridization chain reaction signal amplification. Anal. Bioanal. Chem. 412 (15), 3787–3797. doi:10.1007/s00216-020-02633-5
Sharifi, H., Tashkhourian, J., and Hemmateenejad, B. (2020). A 3D origami paper-based analytical device combined with PVC membrane for colorimetric assay of heavy metal ions: Application to determination of Cu(II) in water samples. Anal. Chim. Acta 1126, 114–123. [Internet]Available from:. doi:10.1016/j.aca.2020.06.006
Shimazu, R., Tomimuro, K., Ni, Y., Malegori, C., Hamedpour, V., Hiruta, Y., et al. (2022). Microfluidic thread-based analytical devices for point-of-care detection of therapeutic antibody in blood. Sensors Actuators B Chem. 352, 131002. doi:10.1016/j.snb.2021.131002
Shin, J. H., and Choi, S. (2021). Open-source and do-it-yourself microfluidics. Sensors Actuators B Chem. 347, 130624. doi:10.1016/j.snb.2021.130624
Shiu, P. P., Knopf, G. K., Ostojic, M., and Nikumb, S. (2008). Rapid fabrication of tooling for microfluidic devices via laser micromachining and hot embossing. J. Micromechanics Microengineering 18 (2), 025012. doi:10.1088/0960-1317/18/2/025012
Silva, R., Ahamed, A., Cheong, Y. H., Zhao, K., Ding, R., and Lisak, G. (2022). Non-equilibrium potentiometric sensors integrated with metal modified paper-based microfluidic solution sampling substrates for determination of heavy metals in complex environmental samples. Anal. Chim. Acta 1197, 339495. doi:10.1016/j.aca.2022.339495
Snyder, S. A., Boban, M., Li, C., VanEpps, J. S., Mehta, G., and Tuteja, A. (2020). Lysis and direct detection of coliforms on printed paper-based microfluidic devices. Lab. Chip 20 (23), 4413–4419. [Internet]Available from. doi:10.1039/D0LC00665C
Song, Y., Lin, B., Tian, T., Xu, X., Wang, W., Ruan, Q., et al. (2018). Recent progress in microfluidics-based biosensing. Anal. Chem. 91 (1), 388–404. doi:10.1021/acs.analchem.8b05007
Soum, V., Park, S., Brilian, A. I., Kwon, O-S., and Shin, K. (2019). Programmable paper-based microfluidic devices for biomarker detections. Micromachines 10 (8), 516. doi:10.3390/mi10080516
Speller, N. C., Morbioli, G. G., Cato, M. E., Cantrell, T. P., Leydon, E. M., Schmidt, B. E., et al. (2019). Cutting edge microfluidics: Xurography and a microwave. Sensors Actuators B Chem. 291, 250–256. [Internet]Available from:. doi:10.1016/j.snb.2019.04.004
Stanley, C. E., Grossmann, G., Casadevall i Solvas, X., and deMello, A. J. (2016). Soil-on-a-Chip: Microfluidic platforms for environmental organismal studies. Lab. Chip 16 (2), 228–241. [Internet]Available from. doi:10.1039/C5LC01285F
Strike, Z., Ghofrani, K., and Backhouse, C. (2018). CO2 Laser-Based Rapid Prototyping of Micropumps. Micromachines 9. doi:10.3390/mi9050215
Su, R., Wen, J., Su, Q., Wiederoder, M. S., Koester, S. J., Uzarski, J. R., et al. (2020). 3D printed self-supporting elastomeric structures for multifunctional microfluidics. Sci. Adv. 6 (41), eabc9846. doi:10.1126/sciadv.abc9846
Su, W., Cook, B. S., Fang, Y., and Tentzeris, M. M. (2016). Fully inkjet-printed microfluidics: A solution to low-cost rapid three-dimensional microfluidics fabrication with numerous electrical and sensing applications. Sci. Rep. 6 (1), 35111. [Internet]Available from. doi:10.1038/srep35111
Sun, H., Jia, Y., Dong, H., and Fan, L. (2019). Graphene oxide nanosheets coupled with paper microfluidics for enhanced on-site airborne trace metal detection. Microsystems Nanoeng. 5 (1), 4. [Internet]Available from. doi:10.1038/s41378-018-0044-z
Sun, H., Jia, Y., Dong, H., Fan, L., and Zheng, J. (2018). Multiplex quantification of metals in airborne particulate matter via smartphone and paper-based microfluidics. Anal. Chim. Acta 1044, 110–118. [Internet]Available from. doi:10.1016/j.aca.2018.07.053
Sweet, E. C., Liu, N., Chen, J., and Lin, L. (2019). “Entirely-3D printed microfluidic platform for on-site detection of drinking waterborne pathogens,” in 2019 IEEE 32nd international conference on micro electro mechanical systems. Seoul, South Korea: MEMS, 79–82.
Szabo, E., and Hess-Dunning, A. (2021). Irreversible, self-aligned microfluidic packaging for chronic implant applications. J. Micromechanics Microengineering 31 (9), 095011. doi:10.1088/1361-6439/ac1994
Tabani, H., Samkumpim, T., Alahmad, W., Dorabadizare, F., and Varanusupakul, P. (2022). In-tube gel electro-membrane combined with microfluidic paper-based device: A green and miniaturized extraction mode for the chromium speciation. Adv. Sample Prep. 3, 100036. [Internet]Available from:. doi:10.1016/j.sampre.2022.100036
Taheri, H., and Khayatian, G. (2022). Smartphone-based microfluidic chip modified using pyrrolidine-1-dithiocarboxylic acid for simultaneous colorimetric determination of Cr3+ and Al3+ ions. Spectrochimica Acta Part A Mol. Biomol. Spectrosc. 272, 121000. doi:10.1016/j.saa.2022.121000
Tan, W., Powles, E., Zhang, L., and Shen, W. (2021). Go with the capillary flow. Simple thread-based microfluidics. Sensors Actuators B Chem. 334, 129670. [Internet]Available from:. doi:10.1016/j.snb.2021.129670
Tang, R., Xie, M. Y., Li, M., Cao, L., Feng, S., Li, Z., et al. (2022). Nitrocellulose membrane for paper-based biosensor. Appl. Mater. Today 26, 101305. doi:10.1016/j.apmt.2021.101305
Tasaengtong, B., and Sameenoi, Y. (2020). A one-step polymer screen-printing method for fabrication of microfluidic cloth-based analytical devices. Microchem. J. 158, 105078. doi:10.1016/j.microc.2020.105078
Tesfaye, T., and Hussen, A. (2022). Microfluidic paper-based analytical device (µPAD) fabricated by wax screen printing technique for the determination of nitrite and nitrate ion in water samples. Microfluid. Nanofluidics 26 (3), 22–13. doi:10.1007/s10404-022-02520-8
Thomas, M. S., Millare, B., Clift, J. M., Bao, D., Hong, C., and Vullev, V. I. (2010). Print-and-peel fabrication for microfluidics: what’s in it for biomedical applications? Ann. Biomed. Eng. 38 (1), 21–32. doi:10.1007/s10439-009-9831-x
Tien, J., and Dance, Y. W. (2021). Microfluidic biomaterials. Adv. Healthc. Mater. 10 (4), 2001028. doi:10.1002/adhm.202001028
Tomazelli Coltro, W. K., Cheng, C-M., Carrilho, E., and de Jesus, D. P. (2014). Recent advances in low-cost microfluidic platforms for diagnostic applications. Electrophoresis 35 (16), 2309–2324. [Internet]Available from. doi:10.1002/elps.201400006
Tran, B. T., Rijiravanich, P., Puttaraksa, N., and Surareungchai, W. (2022). Wax gates in laminated microfluidic paper-based immunosensors. Microchem. J. 178, 107343. doi:10.1016/j.microc.2022.107343
Trotta, G., Volpe, A., Ancona, A., and Fassi, I. (2018). Flexible micro manufacturing platform for the fabrication of PMMA microfluidic devices. J. Manuf. Process. 35, 107–117. doi:10.1016/j.jmapro.2018.07.030
Volpatti, L. R., and Yetisen, A. K. (2014). Commercialization of microfluidic devices. Trends Biotechnol. 32 (7), 347–350. doi:10.1016/j.tibtech.2014.04.010
Waheed, S., Cabot, J. M., Macdonald, N. P., Lewis, T., Guijt, R. M., Paull, B., et al. (2016). 3D printed microfluidic devices: Enablers and barriers. Lab. Chip 16 (11), 1993–2013. doi:10.1039/c6lc00284f
Wang, G., Chu, L. T., Hartanto, H., Utomo, W. B., Pravasta, R. A., and Chen, T-H. (2020). Microfluidic particle dam for visual and quantitative detection of lead ions. ACS Sensors 5 (1), 19–23. [Internet]Available from:. doi:10.1021/acssensors.9b01945
Wang, L., Li, B., Wang, J., Qi, J., Li, J., Ma, J., et al. (2022). A rotary multi-positioned cloth/paper hybrid microfluidic device for simultaneous fluorescence sensing of mercury and lead ions by using ion imprinted technologies. J. Hazard. Mater. 428, 128165. [Internet]Available from:. doi:10.1016/j.jhazmat.2021.128165
Wang, M., Song, Z., Jiang, Y., Zhang, X., Wang, L., Zhao, H., et al. (2021). A three-dimensional pinwheel-shaped paper-based microfluidic analytical device for fluorescence detection of multiple heavy metals in coastal waters by rational device design. Anal. Bioanal. Chem. 413 (12), 3299–3313. Available from. doi:10.1007/s00216-021-03269-9
Wang, Y., Deng, R., Yang, L., and Bain, C. D. (2019). Fabrication of monolayers of uniform polymeric particles by inkjet printing of monodisperse emulsions produced by microfluidics. Lab. Chip 19 (18), 3077–3085. doi:10.1039/c9lc00588a
Wang, Z., Wang, H., Liu, B., Qiu, W., Zhang, J., Ran, S., et al. (2011). Transferable and flexible nanorod-assembled TiO2 cloths for dye-sensitized solar cells, photodetectors, and photocatalysts. ACS Nano 5, 8412–8419.
Wei, L., Fang, G., Kuang, Z., Cheng, L., Wu, H., Guo, D., et al. (2022). 3D-printed low-cost fabrication and facile integration of flexible epidermal microfluidics platform. Sensors Actuators B Chem. 353, 131085. doi:10.1016/j.snb.2021.131085
Weisgrab, G., Ovsianikov, A., and Costa, P. F. (2019). Functional 3D printing for microfluidic chips. Adv. Mater Technol. 4 (10), 1900275. doi:10.1002/admt.201900275
Weng, X., Kang, Y., Guo, Q., Peng, B., and Jiang, H. (2019). Recent advances in thread-based microfluidics for diagnostic applications. Biosens. Bioelectron. 132, 171–185. [Internet]Available from:. doi:10.1016/j.bios.2019.03.009
Wu, S., Wu, Y., Cao, B., Huang, Q., and Cai, P. (2022). An invisible workforce in soil: The neglected role of soil biofilms in conjugative transfer of antibiotic resistance genes. Crit. Rev. Environ. Sci. Technol. 52 (15), 2720–2748. [Internet]Available from. doi:10.1080/10643389.2021.1892015
Xi, Y., Duford, D. A., and Salin, E. D. (2010). Automated liquid–solid extraction of pyrene from soil on centrifugal microfluidic devices. Talanta 82 (3), 1072–1076. [Internet]Available from:. doi:10.1016/j.talanta.2010.06.007
Xia, J., Khaliliazar, S., Hamedi, M. M., and Sonkusale, S. (2021). Thread-based wearable devices. MRS Bull. 46 (6), 502–511. doi:10.1557/s43577-021-00116-1
Xia, Y., Si, J., and Li, Z. (2016). Fabrication techniques for microfluidic paper-based analytical devices and their applications for biological testing: A review. Biosens. Bioelectron. 77, 774–789. [Internet]Available from:. doi:10.1016/j.bios.2015.10.032
Xiao, G., He, J., Chen, X., Qiao, Y., Wang, F., Xia, Q., et al. (2019). A wearable, cotton thread/paper-based microfluidic device coupled with smartphone for sweat glucose sensing. Cellulose 26 (7), 4553–4562. doi:10.1007/s10570-019-02396-y
Xiong, H., Ye, X., Li, Y., Qi, J., Fang, X., Kong, J., et al. (2021). Efficient Microfluidic-Based Air Sampling/Monitoring Platform for Detection of Aerosol SARS-CoV-2 On-site. Anal. Chem. 93, 4270–4276. doi:10.1021/acs.analchem.0c05154
Xie, L., Zi, X., Zeng, H., Sun, J., Xu, L., and Chen, S. (2019). Low-cost fabrication of a paper-based microfluidic using a folded pattern paper. Anal. Chim. Acta 1053, 131–138. doi:10.1016/j.aca.2018.12.001
Xu, B., Qin, T., Zhang, J., Ding, Y., Su, Y., Wu, J., et al. (2021). Cloth-based microfluidic analytical devices by laser-induced hydrophilization technique. Sensors Actuators B Chem. 341, 129998. doi:10.1016/j.snb.2021.129998
Xu, X., Nie, S., Ding, H., and Hou, F. F. (2018). Environmental pollution and kidney diseases. Nat. Rev. Nephrol. 14 (5), 313–324. doi:10.1038/nrneph.2018.11
Yafia, M., Shukla, S., and Najjaran, H. (2015). Fabrication of digital microfluidic devices on flexible paper-based and rigid substrates via screen printing. J. Micromechanics Microengineering 25 (5), 057001. [Internet]Available from. doi:10.1088/0960-1317/25/5/057001
Yamada, K., Henares, T. G., Suzuki, K., and Citterio, D. (2015). Paper-based inkjet-printed microfluidic analytical devices. Angew. Chem. Int. Ed. 54 (18), 5294–5310. doi:10.1002/anie.201411508
Yang, X., Zhang, T., Zhang, X., Chu, C., and Sang, S. (2022). Global burden of lung cancer attributable to ambient fine particulate matter pollution in 204 countries and territories, 1990–2019. Environ. Res. 204, 112023. doi:10.1016/j.envres.2021.112023
Yang, J., Li, K., Zhu, L., and Tang, W. (2017). Fabrication of PDMS microfluidic devices with 3D wax jetting. Rsc Adv. 7, 3313–3320.
Yazdi, A. A., Popma, A., Wong, W., Nguyen, T., Pan, Y., and Xu, J. (2016). 3D printing: An emerging tool for novel microfluidics and lab-on-a-chip applications. Microfluid. Nanofluidics 20 (3), 50–18. doi:10.1007/s10404-016-1715-4
Yehia, A. M., Saad, A. S., and Tantawy, M. A. (2020). USB multiplex analyzer employing screen-printed silver electrodes on paper substrate; a developed design for dissolution testing. J. Pharm. Biomed. Analysis 186, 113272. doi:10.1016/j.jpba.2020.113272
Yew, M., Ren, Y., Koh, K. S., Sun, C., and Snape, C. (2019). A review of state-of-the-art microfluidic technologies for environmental applications: Detection and remediation. Glob. Challenges 3 (1), 1800060. doi:10.1002/gch2.201800060
Yin, K., Ding, X., Xu, Z., Li, Z., Wang, X., Zhao, H., et al. (2021). Multiplexed colorimetric detection of SARS-CoV-2 and other pathogens in wastewater on a 3D printed integrated microfluidic chip. Sensors Actuators B Chem. 344, 130242. [Internet]Available from:. doi:10.1016/j.snb.2021.130242
Yuan, Y., Jia, H., and Wang, J. (2022). A microfluidic electrochemical sensing platform for in situ detection of trace cadmium ions. Anal. Methods 14, 3802–3813. doi:10.1039/d2ay01016j
Zhang, C., Su, Y., Liang, Y., and Lai, W. (2020). Microfluidic cloth-based analytical devices: Emerging technologies and applications. Biosens. Bioelectron. 168, 112391. doi:10.1016/j.bios.2020.112391
Zhang, H., Fang, F., Gilchrist, M. D., and Zhang, N. (2018). Filling of high aspect ratio micro features of a microfluidic flow cytometer chip using micro injection moulding. J. Micromechanics Microengineering 28 (7), 075005. doi:10.1088/1361-6439/aab7bf
Zhang, N., Liu, J., Zhang, H., Kent, N. J., Diamond, D., and D Gilchrist, M. (2019). 3D printing of metallic microstructured mould using selective laser melting for injection moulding of plastic microfluidic devices. Micromachines 10 (9), 595. doi:10.3390/mi10090595
Zhao, J., Liu, M., Liang, L., Wang, W., and Xie, J. (2016). Airborne particulate matter classification and concentration detection based on 3D printed virtual impactor and quartz crystal microbalance sensor. Sensors Actuators A Phys. 238, 379–388. [Internet]Available from:. doi:10.1016/j.sna.2015.12.029
Zheng, L., Liu, Y., and Zhang, C. (2021). A sample-to-answer, wearable cloth-based electrochemical sensor (WCECS) for point-of-care detection of glucose in sweat. Sensors Actuators B Chem. 343, 130131. doi:10.1016/j.snb.2021.130131
Zheng, W., Wang, K., Xu, H., Zheng, C., Cao, B., Qin, Q., et al. (2021). Strategies for the detection of target analytes using microfluidic paper-based analytical devices. Anal. Bioanal. Chem. 413 (9), 2429–2445. doi:10.1007/s00216-021-03213-x
Zhou, M., Shi, X., Li, X., Xiao, G., Liang, L., Ju, J., et al. (2021). Constructing silk fibroin-based three-dimensional microfluidic devices via a tape mask-assisted multiple-step etching technique. ACS Appl. Bio Mater 4 (11), 8039–8048. doi:10.1021/acsabm.1c00948
Zhu, X., Wang, K., Yan, H., Liu, C., Zhu, X., and Chen, B. (2022). Microfluidics as an emerging platform for exploring soil environmental processes: A critical review. Environ. Sci. Technol. 56 (2), 711–731. Available from:. doi:10.1021/acs.est.1c03899
Zhu, Y., and Fang, Q. (2013). Analytical detection techniques for droplet microfluidics—a review. Anal. Chim. Acta 787, 24–35. doi:10.1016/j.aca.2013.04.064
Keywords: microfluidics, environmental monitoring, low-cost materials, low-cost fabrication methods, water/air/soil quality
Citation: Mesquita P, Gong L and Lin Y (2022) Low-cost microfluidics: Towards affordable environmental monitoring and assessment. Front. Lab. Chip. Technol. 1:1074009. doi: 10.3389/frlct.2022.1074009
Received: 19 October 2022; Accepted: 14 November 2022;
Published: 01 December 2022.
Edited by:
Samar Damiati, University of Sharjah, United Arab EmiratesReviewed by:
Bernhard Schuster, University of Natural Resources and Life Sciences Vienna, AustriaHyundoo Hwang, Bredis Inc., South Korea
Copyright © 2022 Mesquita, Gong and Lin. This is an open-access article distributed under the terms of the Creative Commons Attribution License (CC BY). The use, distribution or reproduction in other forums is permitted, provided the original author(s) and the copyright owner(s) are credited and that the original publication in this journal is cited, in accordance with accepted academic practice. No use, distribution or reproduction is permitted which does not comply with these terms.
*Correspondence: Yang Lin, eWFuZ2xpbkB1cmkuZWR1