- 1Cell Therapy Core, Vitalant Research Institute, San Francisco, CA, United States
- 2Department of Laboratory Medicine, Medical Center, University of California, San Francisco, San Francisco, CA, United States
- 3Research Division, Faculty of Medicine, National Autonomous University of Mexico, Mexico City, Mexico
- 4Laboratorio de Investigación en Inmunología y Proteómica, Hospital Infantil de México Federico Gómez, Mexico City, Mexico
Hematopoiesis is a process by which all blood cells are formed. The mechanisms controlling it have been studied for decades. Surprisingly, while hematopoietic stem cells are among the most extensively studied stem cell types, the complete understanding of how they are regulated during development, adulthood, or in non-homeostatic conditions remains elusive. In this review, our primary focus is on research findings that explore where hematopoietic precursors are found in adults outside their primary niches in the bone marrow. This phenomenon is termed extramedullary hematopoiesis (EMH). Early in development hematopoietic stem cells migrate through different regions within and outside the embryo and later the fetus. Although, the primary home for hematopoietic progenitors is the adult bone marrow, it is now recognized that other adult organs may act as hematopoietic progenitor reservoirs both in mice and humans. The first reports about this topic were principally originated from clinical observations, in cases where the bone marrow was malfunctioning, leading to an aberrant hematopoiesis outside the bone marrow. It is worth highlighting that those extramedullary organs, like the small intestine or fat tissue, contain subsets of fully functioning hematopoietic progenitors demonstrated by both in vitro and in vivo studies. Nonetheless, there are still some unanswered questions regarding the source of these cells, how they differ in function compared to their counterparts in the bone marrow, and the specific roles they play within the tissues where they are located.
1 Introduction
Stem cells are a unique cell type with the remarkable ability to serve as building blocks for all cell types in the body. The studies of Till and McCulloch were instrumental in shaping our understanding of stem cells, and their work continues to have a lasting impact on both basic research and clinical applications in the field of regenerative medicine and beyond McCulloch and Till (1). Over the past half-century, researchers have discovered combinations of physical properties, cell-surface markers, and devised assays to measure stem cell function that allow for the identification and separation of stem cell populations in adult tissues like the skin, intestinal epithelium, brain, and the hematopoietic system.
The best characterized stem cells are the hematopoietic stem cells (HSCs), which reside primarily within the bone marrow (BM) during adulthood. Murine HSCs are distinguished by the absence of cell-surface markers present on lineage (Lin)-committed hematopoietic cells. Additionally, these cells express high levels of the stem cell factor receptor (c-kit, CD117) (2) and stem-cell antigen 1 (Sca1) (3, 4); this Lin−c-kit+Sca1+ subset is commonly referred to as LSK cells (4, 5).
HSCs account for 0.00125–0.00425% (12–42 cells per million) of whole BM in mice (6). These HSCs are comprised of two main types: Long-term HSCs (LT-HSCs) or dormant HSCs and short-term HSCs (ST-HSCs) or activated HSCs. These populations of HSCs can be differentiated by expression of the anti-adhesive sialomucin CD34 (7); mouse LT-HSCs do not express CD34 until they become activated ST-HSCs (6, 8, 9). Other methods of HSC isolation often begin with enrichment of LSK cells, a heterogeneous population of hematopoietic precursors, with only a small subset consisting of LT-HSCs. According to flow cytometry analysis, the frequency of LT-HSCs within the LSK population is approximately 10% (10, 11). However, in vivo analyses may vary in the frequencies of long-term reconstituting cells measured among LSK cells: frequencies as low as 2% (12) or as high as 20% (13) having been reported. Frequency variation in the BM progenitor pool may be related to mouse age (14), sex (15), and strain variation (16, 17). For example, the frequency of LT-HSCs cells increases with age (10). In addition to CD34, other markers of HSCs that have been used to differentiate LSK cells are the SLAM family receptors (CD150 and CD48) (13), CD90 (5), and the capacity of HSCs to remove intravital dyes like Rhodamine-123 (3, 18).
In humans, the HSC population, similar to mice, is characterized by the absence of maturity markers and the expression of CD34 (Lin−CD34+) (19). Furthermore, HSCs are found to be more abundant among CD34+ cells expressing CD4 (20), CD90 (21), CD133 (22), and EPCR (23), while lacking expression of CD38 (24–26), CD45RA (27), and CD71 (28). Similar to murine HSCs, there exists a rare subset of HSCs characterized by the absence of an extensive lineage marker panel and CD34 (29). These CD34− HSCs have been identified in human cord blood and are known to express GPI-80 (30), CD90 (31), CD93 (31), and CD133 (32). While transplant success using CD34 selection suggests the presence of functional HSCs within the CD34+ fractions of neonatal and adult cells, experimental evidence indicates that pre-natal CD34− HSCs may be more primitive than their CD34+ counterparts (31, 33).
2 Hematopoiesis: from development to adulthood
During embryonic development, the earliest hematopoietic progenitor cells emerge in the yolk sac, appearing around day 17 in humans and embryonic day 7.5 (E7.5) in mice (34). These progenitors, originating from the mesodermal layer, form clusters known as “blood islands” within the yolk sac, closely associated with endothelial and hematopoietic cells. Human yolk sac development progresses through three phases: 1) a formative period, 2) a functional period, and 3) a period of regression. During the functional phase, which typically occurs during the second stage, the yolk sac comprises cells of both mesodermal and endodermal origin, housing endothelial and early hematopoietic cells (35). In humans, at 3–4 post-conception week (PCWs), the first generation of hematopoietic cells proliferate in the yolk sac and extraembryonic mesenchyme, primarily consisting of “primitive” erythroblasts (megaloblasts) which are present in circulating blood from 4 PCWs onward (36). Shortly after the initiation of blood island formation and primitive hematopoietic progenitor generation, endothelial cells within the para-aortic splanchnopleura region begin producing definitive HSCs. In the mouse, this process typically takes place around E9.5 (37), while in humans, it occurs briefly around Carnegie Stages (CS) 9–12 which is equivalent to 19–27 days of embryonic development (38). Subsequently, the para-aortic splanchnopleura region gives rise to most tissues in the aorta-gonad-mesonephros (AGM) (37–39), which emerges approximately after E10.5 in mouse and after the 27th day of pregnancy in humans as an active site of hematopoiesis (38, 40–42).
In mice, HSCs are present in the placenta at the same time as they are in the AGM region (43). Similarly, hematopoietic progenitors are present in the human placenta at approximately 5–6 PCWs (CS 14–16) (44–46). Human HSCs capable of long-term multilineage engraftment in immunodeficient mice were detected from placenta as early as 6 weeks of gestation (CS 17) (45). The human chorion, an extra-embryonic tissue that shares a developmental origin with placenta but is much less vascularized, also contains hematopoietic precursors and HSCs at 5–6 weeks of gestation capable of long-term reconstitution (46, 47). Hematopoietic progenitors in the early stages of placental/chorionic development may originate from extra-embryonic sources. A recent study demonstrated that primitive erythromyeloid progenitors in the placenta are distinct from definitive hematopoietic stem cells due to their absence of HLF expression and their limited lineage commitment primarily to the erythromyeloid lineage (48).
As embryonic development progresses, the liver becomes another important hematopoietic niche. Intraembryonic HSCs are located within the fetal liver of rodents (49) and humans (50) shortly after the liver has formed. It is known that HSCs migrate from the AGM to fetal liver, although the exact migration pathways are still not fully understood (51) it is known to be guided in response to stromal CXCL12 (52).
In mice, the liver begins to form as a diverticulum from the floor of the embryonic gut around E9 (53). Subsequently, the murine liver bud experiences accelerated growth as it becomes vascularized (54). It is believed that primitive progenitors from the yolk sac migrate to the fetal liver through the vitelline vein around E11 (55). By this stage, the murine liver harbors primitive erythromyeloid progenitors derived from the yolk sac (56). Additionally, AGM-derived HSCs begin to populate the murine fetal liver starting at E11, coinciding with maximal AGM activity (57). Subsequently, fetal liver cells at E12 undergo a significant expansion, up to 38-fold, and exhibit long-term reconstitution capability when transplanted into an irradiated mouse, indicating the presence of definitive HSCs by E12, as opposed to E10 and E11 cells, which did not result in reconstitution (58). This observation aligns with the decrease in HSCs observed in the AGM region by E12 (57).
An early and noteworthy investigation illustrated various hematopoietic cell lineages within the human embryonic liver as early as 5 PCW (CS 14–15), delineating their morphological characteristics (59). However, the cells may have comprised circulating primitive erythro-myeloid progenitors and their progeny, as they do not express CD34 and have been observed as early as 23 days of development (CS 10) (50). Recent studies indicate that the liver is initially populated by molecularly defined HSCs around 6 PCWs (CS 16) (60). Although CD34+ progenitors are detected in the human fetal liver as early as 30 days of development (CS 13) (50), several investigations indicate that transplantable HSCs are first present in the embryonic liver shortly thereafter, at 5–6 PCWs (CS 14–16) (61–64).
From this time forward until midgestation, the human liver is the primary intra-embryonic hematopoietic organ (65). Erythropoiesis dominates the hematopoietic output of the liver in order to meet the demands of an expanding blood volume (66, 67). Indeed, flow cytometric analysis of whole fetal liver indicate that CD235a in vitro erythroid cells are the dominant cell type in the liver during midgestation (68). In addition to erythropoiesis, liver hematopoiesis includes elements of myeloid and lymphoid precursors (26, 69–71). Single-cell transcriptome profiling of human fetal liver further confirmed the presence of HSCs and MPPs with the capability to generate functional innate lymphoid cells, T-, and B-lymphocytes, natural killer (NK), and CD34+ myeloid progenitors (72).
Interestingly, some long-lived myeloid cells are found in adult tissues like the lungs or peritoneal cavity. These so-called “tissue resident macrophages” emerge from the yolk-sac instead of progenitors derived from the AGM Gomez (56) or fetal liver (73). Also, some lymphoid populations like the B-1 B cells originate in the yolk-sac, which represent part of the humoral immune response but not the classic adaptive immune system. Similarly to tissue resident macrophages, this subset of B cells are rarely found circulating in the blood stream (74), and undergo self-renewal in the periphery (75). It is noteworthy that the nature of these yolk-sac derived leukocytes have more in common with innate immunity than adaptive immunity as reviewed in (76). While the presence of B-1 cells in humans has been controversial (74).However, a recent comprehensive comparative single-cell sequencing analysis of human fetal and adult tissues has presented compelling evidence, according to these authors, supporting the origin of human fetal B-1 cells (77).
Finally, the definitive hematopoietic organ, the BM, becomes hematopoietic at E15.5 in mouse (78) and at 10.5 PCW in humans (79). Although the first HPSCs in the murine bone marrow are found as early as E15.5 and E16 (80) the amount of LT-HSCs found a these stages is limited and steadily increasing until birth (78). The mouse BM is seeded by HSCs originating in the fetal liver (81). In humans fetal BM is also colonized by circulating hematopoietic precursors derived from fetal liver found in relative abundance during early gestational periods compared to neonates (82, 83). Human fetal BM is first found in the long bones of the fetus as these bones are the first to become large enough to contain a marrow. A recent investigation revealed that although HSCs with defined phenotypes are present in the human BM at 10 PCW, they attain full functionality only after reaching 12 PCWs (84). The BM becomes the dominant site of hematopoiesis early in the second half of gestation as the marrow increases in size relative to the liver.
It is worth mention that the fetal spleen is an important hematopoietic tissue in mice bridging the peri-natal period when hematopoiesis diminishes in the liver and before the BM forms. Humans and mice differ in this regard as the spleen contains but an insignificant number of hematopoietic precursors at any time during gestation (85, 86). Figure 1 illustrates the temporal migration of HSCs in human and mouse as well as the tissues where hematopoiesis is normally found.
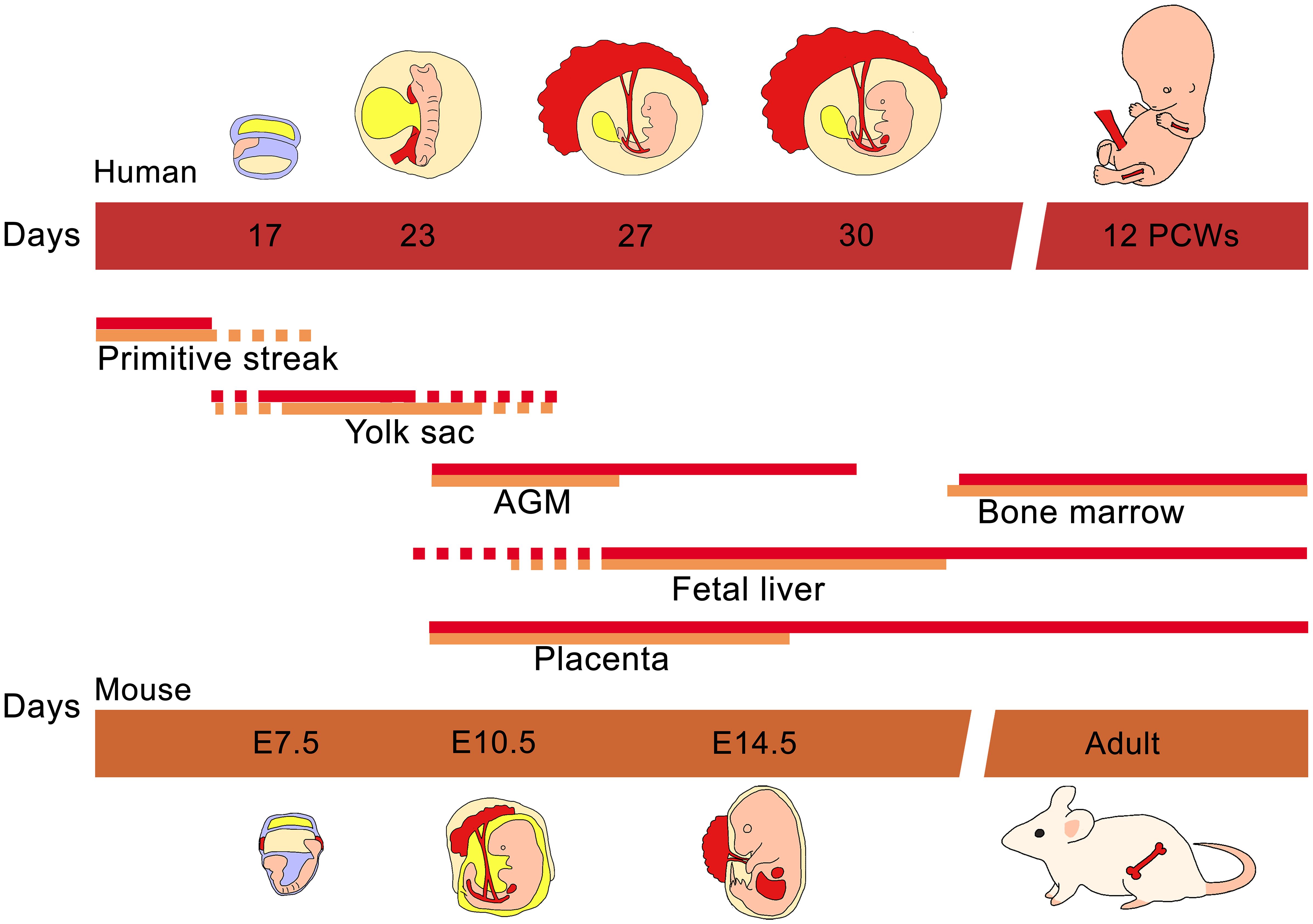
Figure 1 Ontogeny of hematopoiesis in humans and mice. The early hematopoietic progenitor cells emerged in the primitive yolk sac of both human and mouse. While the embryo continues its development, the mesoderm gives rise to vascular structures like the AGM where the definitive hematopoietic cells are produced. Almost at the same time the placenta and embryonic liver are colonized by AGM progenitors. Finally fetal liver hematopoietic cells migrate to populate the bone marrow.
3 Adult extramedullary hematopoiesis
EMH can occur in response to both homeostatic and pathologic conditions, such as acute infection or inflammation. Organs like the spleen and liver can start producing blood cells due to local production of hematopoietic growth factors like GM-CSF, TNFα, and IL-3 (87, 88). In chronic pathologies, the BM may lose its ability to function effectively, leading to increased reliance on organs like the liver, spleen, or even the lungs for blood cell production. In mice, splenic EMH readily occur when the capacity of the BM to produce blood cells is exceeded, recalling the significant role the spleen plays during the perinatal period in hematopoietic development. However, large mammals possessing substantial bone marrow reserves may not demonstrate a comparable propensity to transition blood cell production from the bone marrow to the spleen.
Myelofibrosis, diffuse osseous metastatic disease, leukemia, sickle cell disease, and thalassemia are among the primary factors contributing to human extramedullary hematopoiesis. (89). Many reports have found the presence of hematopoietic progenitors in extramedullary organs due to defective hematopoiesis in the BM due to any of these diseases (Table 1). Interestingly, some cases of EMH are found after inflammatory conditions such as inflamed joints (115), or lung infection (116, 117). Table 1 summarizes reports were EMH is found secondary to disease.
Pathological EMH is a secondary problem diagnosed principally with radiological studies, computed tomography (CT) or magnetic resonance imaging (MRI) (Table 1). Following resection, the anomalous mass is examined by pathologists, the most common techniques used to determine a hematological signature include staining for glycophorin (GPA, CD235a) for erythroid progenitors and Giemsa (118) or H&E (Hematoxylin and Eosin) staining for morphology (89). Sometimes immunohistochemistry (IHC) (104, 106, 111) or flow cytometry (FC) (98) with antibodies against known progenitor and mature immune cells or colloidal scans (93, 105) are used.
Although limited, there are some reports showing that HSPCs are present in tissues outside the BM under homeostatic conditions both in human and animal models. Furthermore, it has been established that these cells exhibit re-populating properties similar to those found in the BM. For example, tissues like the small intestine in humans contain hematopoietic progenitors expressing CD34 and CD45 (119). Later work showed not only a distinct phenotype but also confirmed the presence of hematopoietic colony-forming cells using clonal analysis in vitro (CFU-c). Additionally, it demonstrated the potential for long-term chimerism in individuals receiving intestinal allografts (120).
Another potential host for hematopoietic progenitors in humans is the stromal-vascular fraction (SVF) of adipose tissue. In pathological conditions such as chronic myeloid leukemia, adipose tissue has the potential to transform into a hematopoietic niche. In this context, leukemic cells express a fatty acid transporter to enhance their communication with the adipose microenvironment and evade chemotherapy (121). Under homeostatic conditions, this tissue harbors a subset highly enriched in CD34+ and VEGFR2+ (KDR) cells with absent CD45 expression. Interestingly, despite the lack of CD45 expression, these cells were able to originate hematopoietic colonies in a CFU-c assay (122). Notably, murine SVF within adipose tissue contains similar progenitors defined by a LSK phenotype and are capable of long-term multi-lineage reconstitution when transplanted into a recipient (123).
Among other organs known to exhibit pathology associated EMH, the lungs are included. While there is no conclusive evidence confirming the presence of HSCs in human lungs, there is evidence supporting their existence in mice. Initial observations in mouse lungs have revealed the occurrence of a cell subset, VEGFR2+CD31+ cells, which can produce endothelial cells, smooth muscle cells and form hematopoietic colonies in vitro (124). Anatomical descriptions of lung CD34+Sca1+-kit+ cells showed these cells are located in close proximity to blood vessels, and possibly belong to a unknown subset of hematopoietic progenitors (125). Furthermore it has been reported that mouse lungs are active hematopoietic organs, indeed they account for 50% of platelet production and are a source of hematopoietic progenitor and HSCs with a phenotype and repopulating activity similar to its counterparts in the murine BM (116).
Since the lungs are not classically known to be hematopoietic niches, it has been hypothesized that lung vasculature may function as an adaptive niche for HSCs, principally ST-HSCs, since LT-HSCs were not found by Lefrançais et al. in the lungs (116, 126). The lungs harbor more than 50 different cell types (127), the most likely subset to be nurturing HSCs are pericytes or distinct blood vessels, further work is needed to elucidate this question.
Besides the lungs, reports have indicated that the liver and spleen also display EMH associated with pathologies. Over the last decade, numerous studies have revealed that both organs harbor functional hematopoietic progenitors along with the necessary microenvironment to sustain them. A recent report has indicated the presence of an LSK population in the mouse spleen, along with its ability to expand when the Txl1 gene is overexpressed in splenic stromal cells (128). As for hepatic hematopoiesis, there is evidence that supports that liver sinusoid endothelial cells provide an appropriate hematopoietic environment that supports the proliferation and differentiation of hematopoietic progenitors in the mouse (129). Moreover, adult murine liver was shown to support human HSC engraftment, showing long-term multilineage hematopoiesis (130). In addition, donor chimerism after liver transplantation is a common finding, this comes from having a significant amount of donor-derived hematopoietic cells in the liver graft (131, 132). Liver-derived hematopoietic engraftment has even been documented to lead to a complete blood group change in the recipient (133).
In summary, although EMH has been observed under both homeostatic and emergency conditions, it remains uncertain whether extramedullary precursors are remnants from early ontogeny or if they come from and are constantly replenished from the BM (Figure 2). Consequently, we wish to highlight the importance of thoroughly researching all mechanisms involved in these phenomena.
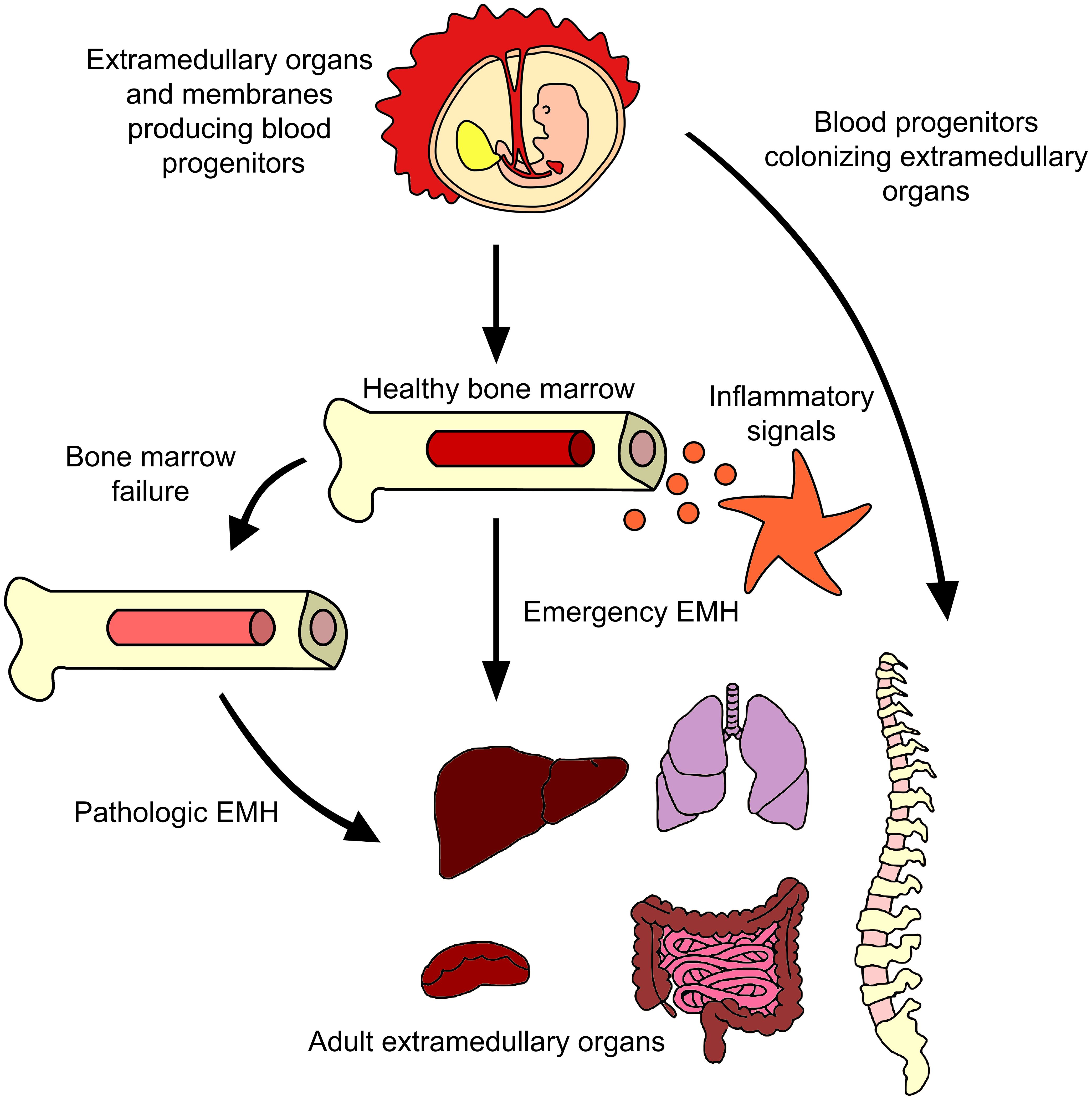
Figure 2 Extramedullary hematopoiesis in adults. Many reports have described the generation of progenitor cells outside the BM in adults. The major evidence points to BM failure and inflammation being the causes of some blood progenitors to emigrate from BM to organs like liver or spleen, although evidence of lungs or paraspinal EMH have been described. Murine models showed the presence of functional hematopoietic precursos in those tissues even without a disease or inflammatory insult. It remains a mystery if those cells came from BM or have a prenatal origin.
4 Molecules controlling hematopoiesis
The HSCs in the BM require a special microenvironment (niche) to maintain stemness. In the BM, two types of niches have been identified. First, the endosteal niche located in the inner cellular lining within bone cavity in contact with BM of trabecular bone, which is called endosteum. This region comprises specialized osteoblasts, CXCL12-abundant reticular cells (CARs), osteoclasts, and mesenchymal stromal cells (MSCs). Secondly, we have the vascular niche where HSCs are found next to CARs adjacent to BM sinusoids (134).
The molecules secreted from niche cells promote HSC maintenance. These include stem cell factor (SCF, KL) (135–136), CXCL12 (137, 138), angiopoietin 1 (139), and thrombopoietin (TPO) (140). Further detailed analysis of the BM niche showed that approximately 80% of dividing and non-dividing hematopoietic progenitors reside in close proximity to a sinusoid and nearly all HSCs are in contact with leptin receptor and CXCL12 high expressing niche cells (LEPR+CXCL12hi). The rest of the BM hematopoietic progenitors are located near arterioles (10%) and transition zones (10%) with only a few HSCs residing adjacent to the endosteum (141). In general, it is well-established that BM HSCs primarily inhabit these perivascular environments.
Other molecules are produced by a wide variety of cells that are essential for the regulation of hematopoiesis such as: SCF, notch ligands, bone morphogenic protein, transforming growth factor β (TGF-β), TPO, fibroblast growth factors, and insulin-like growth factor 2 as reviewed by (142). These molecules are necessary for maintaining and activating the resident HSCs on demand.
On the other hand, HSC and progenitor proliferation and differentiation is primarily regulated by cytokines like erythropoietin, granulocyte-monocyte colony-stimulating factor (GM-CSF) (143), α-interferon (INF-α) (144), and γ-interferon (INF-γ) (145). Hematopoiesis activated by inflammatory signals is often referred to as “emergency hematopoiesis” and it has been widely studied in pathogen associated inflammation (146).
Furthermore, factors produced during inflammatory processes in the mouse stimulate hematopoietic progenitors, some Toll-like receptor (TLR) ligands like Pam3CSK4 and lipopolysaccharide (LPS) promotes myelopoiesis in vitro (147). IFN-γ promotes activation and proliferation of HSCs both in vitro and in vivo (145). TLR3 ligand, poly(I:C), and its downstream effector INF-α also promotes the proliferation of dormant HSCs in vivo but chronic treatment caused the opposite effect (144). Other pro-inflammatory cytokines like G-CSF, interleukin (IL)-6, IL-11, and IL-12 promote not only HSC proliferation and differentiation but also HSC mobilization from BM to peripheral blood (148). The mobilization of HSCs is a mechanism by which sites of EMH may be seeded with hematopoietic precursors.
There are multiple cell sources of hematopoietic growth factors beyond innate immune cells that may contribute to EMH. In addition to the immune response, various cell types assist in the restoration and replenishment of hematopoietic niches. In vitro experiments involving the cultivation of HSCs with endothelial cells (ECs) or their soluble products have demonstrated that factors secreted by ECs are essential for enhancing the numbers of CFU-c (149). These factors are only partly defined. It was described that ECs are the main source of G-CSF in BM during inflammation, a molecule known to promote myelopoiesis (150). ECs also secrete IFN-γ and TNF-α, which promote MSC production of IL-13, another hematopoietic regulator in mice (151, 152). BM derived MSCs are affected by infection and inflammation, they respond to cytotoxic CD8+ T effector-cell signals and produce high levels of IL-6, thus promoting myelopoiesis (153). BM-MSCs primed by IFN-γ secretes hepatocyte growth factor, prostaglandin E2, that are known by its immune-suppressive effect but also stimulates growth of hematopoietic progenitors (154, 155). While BM niche molecules are known and widely described, our understanding of hematopoietic regulation outside the BM remains less understood.
5 EHM regulation by soluble factors
Hematopoietic progenitors respond to multiple signals, both in steady state and during inflammation, some of these signals are secreted molecules derived by numerous cell types throughout life as described before (156). Furthermore, the same pro-inflammatory molecules that promotes blood progenitor growth and mobilization in BM are linked to EMH in adults, such as GM-CSF, G-CSF, IL-3, IL-6 and IFN-γ (87, 157). Unfortunately, it is unknown which molecules outside the BM niche are specifically regulating the hematopoietic progenitors found in the extramedullary sites. While there are a number of potential candidates, some of them are discussed in more detail below:
5.1 Stromal derived factor 1
Certain soluble factors exhibit their function not only within the BM but also in extramedullary organs. For instance, stromal derived factor 1 (SDF-1), also recognized as CXCL12, is one such factor. Liver ECs sustain hematopoietic precursors both in vitro and in vivo by secreting SDF-1 and expressing adhesion molecules like CD34 similar to yolk sac endothelium capable of supporting hematopoietic proliferation and differentiation (129, 158).
During periods of stress, it is observed that blood progenitors migrate from the bone marrow to extramedullary tissues. In the spleen of mice, perisinusoidal ECs and tcf21+ stromal cells are found in the red pulp, where they produce SCF and SDF-1 (159). Moreover, it has been shown that a humanized mouse model injected with adenovectors expressing human SDF-1 promoted mobilization of HSCs into the spleen and peripheral blood, leading to an increased number of circulating human CD41+ cells (160). In addition, human spleens exhibiting extramedullary hematopoiesis showed significantly higher expression of SDF-1 compared to EMH-negative cases (161).
5.2 Thymic stromal lymphopoietin
Another possible EMH mediator is the thymic stromal lymphopoietin (TSLP). TSLP is a cytokine, that plays a crucial role in the regulation of the immune system and inflammation (162). TSLP is primarily produced at barrier surfaces in the body, such as the, gut, and lungs (163). Moreover, TSLP is involved in initiating and modulating immune responses at these barrier sites (164).
TSLP receptor is expressed by BM precursors and CD34+ cells in the periphery, the administration of exogenous TSLP to mice increased the percentages and numbers of Lin−CD34+ckit+ cells in the spleen, TSLP is mostly produced by epithelial and stromal cells and is involved in type 2 cytokine-mediated inflammation (165).
5.3 Isthmin-1
The regulation of extramedullary hematopoiesis (EMH) remains poorly understood, especially during homeostasis. While stress conditions offer some insights, questions persist about the origin and presence of resident blood precursors in extramedullary organs during steady-state conditions. As mentioned before, murine lungs contain fully functional hematopoietic progenitors (116). Nonetheless, a comprehensive characterization of the hematopoietic niche in the lungs has yet to be achieved, and certain researchers speculate that pericytes and ECs could potentially serve as niches for hematopoietic cells within the lung (126). Isthmin-1 is expressed by lung progenitors cells that are of hematopoietic and endothelial origin in the mouse (166). This soluble protein is related to NODAL subfamily of TGF-β, thus regulating embryonic development (167, 168). Additionally, Isthmin-1 plays a signaling role in promoting the development and functionality of hematopoietic tissues in the embryonic zebrafish (169). Hence, Ishtmin-1 has the potential to influence the formation of the hematopoietic microenvironment in other species and possibly outside the BM.
5.4 Adrenomedullin
Adrenomedullin (ADM) is a peptide hormone that plays a crucial role in regulating various physiological processes in the human body. It was first discovered in the adrenal medulla, which is why it is named “adrenomedullin” (170). This peptide has shown to improve in vitro expansion of cord blood HSCs in addition to classic cytokines such as SCF, TPO, EPO, FL3, GM-CSF, and GCSF (171, 172). Moreover, it has been demonstrated that ADM, of endothelial origin, is capable of maintaining HSCs in combination with SCF, TPO, and FLT3 ligand in vitro (173). Additionally, some primary BM stromal cell lines with the molecular signature Lin−CD45−CD271+PDGFRαlow/− also express ADM and are able to support human cord blood CD34+ cells (174).
While there is no conclusive evidence connecting ADM to the extramedullary support of hematopoietic progenitors, it is plausible that this peptide plays a role in EMH, given its widespread presence in organs such as the kidneys (175), heart (176), lungs (177), and blood vessels (173, 178).
5.5 Glial-derived neurotrophic factor
Another potential regulator of hematopoietic progenitors is the glial cell-derived neurotrophic factor (GDNF). GDNF, a member of the neurotrophic factor family, is a protein pivotal for the development, upkeep, and viability of numerous nerve cell types, particularly within the peripheral and central nervous systems (179). Recently, is has been shown that GNDF regulate hematopoiesis via RET signaling. Grey et al. demonstrated that the glial family receptor, RET, present on the surface of HSCs, contributes to prolonged cellular growth, increased stress resilience, and enhanced cell survival during in vitro expansion (180). When HSCs are exposed to RET ligand, GNDF, and its coreceptor complex, they exhibit enhanced progenitor function during primary transplantation and improved long-term HSC function during secondary transplantation. Human umbilical cord MSCs, when isolated, have the capacity to generate and release GDNF, contributing to tissue repair. Initially focused on nerve repair, this capability now extends to potentially include hematopoietic cells (181). Interestingly, murine BM HSCs also express RET and respond to it signaling partners, including GDNF (182). RET is not only expressed by BM cells but also for gut-associated lymphoid tissue, its signaling is necessary for Peyer’s patch formation (183). Generally, GDNF production and function is widely described within communication between neurons and their targets both in central as well as peripheral nervous system (184). It is noteworthy that GDNF can also be synthesized by MSCs and influence other types of cells such as salivary stem cells (185) or endothelial cells (186). It is conceivable that GDNF may exert an influence on hematopoietic precursors outside the bone marrow, analogous to its role in intestinal organogenesis.
6 Conclusions
In summary, the significance of extramedullary hematopoiesis (EMH) extends from embryonic development to adulthood, showcasing the dynamic changes in hematopoietic locations. Throughout development, the regulation of hematopoiesis by crucial cell types and molecules shapes the transition from EMH to bone marrow hematopoiesis. Some of these developmental EMH sites may maintain conditions conducive to hosting primitive and definitive hematopoietic progenitors. Recent research revealed that hematopoiesis originating from embryonic hemogenic ECs continues into adulthood, as evidenced even in 12-month-old mice (187). Notably, this research demonstrated that the majority of MPPs, T cells, and B-2 cells within the first month after birth were fetal EC-derived. Whether a similar phenomenon occurs in humans remains to be elucidated. Thus, understanding the origin of HSPCs as well as the variances in niches between normal and aberrant sites is vital, as it influences our comprehension of hematopoietic cell replenishment mechanisms. While BM niche is extensively characterized, with knowledge about cellular components and soluble factors, much remains unknown about the factors influencing adult EMH sites.
Most stem cell niches are situated near blood vessels and stromal cells (188–190). HSCs are no exception (191). Considering the nature of EMH sites, it is highly likely that the cells supporting these hematopoietic precursors are primarily endothelial and stromal cells. There is evidence showing that the vascular fraction of murine extramedullary adult organs like brain, heart, lung and liver are able to maintain LSK cells in vitro (192). In this context, the reliance on endothelial and stromal cells within EMH sites underscores the pivotal role of these cellular components in sustaining hematopoietic potential beyond the bone marrow.
7 Future perspectives
Nevertheless, there exist numerous inquiries that require further resolution. Are these possible niches similar to one another? What distinctions exist between the BM vascular niche and the vascular fraction of the organs displaying typical EMH? Additional research is needed to uncover the mechanisms that regulate the EMH niche-like functions. Undoubtedly, it is crucial to assess and contrast the various cell types participating in EMH with those of the perivascular and/or endosteal cells found in the BM niche.
The more recent models for investigating native hematopoiesis, exemplified by studies such as (193), hold promise for significant advancements in our understanding of hematopoietic processes. These models often incorporate advanced technologies, such as single-cell sequencing and sophisticated imaging techniques, allowing for a finer resolution of cellular interactions and dynamics within the hematopoietic microenvironment.
For instance, in a study where a high-resolution characterization of the bone marrow (BM) niche was conducted in both healthy conditions and acute myeloid leukemia (AML), researchers established a singlecell gene expression database encompassing 339,381 BM cells. The findings revealed significant alterations in cell type proportions and gene expression in AML, suggesting a comprehensive disruption of the entire niche (194). Such high-resolution analyses have the potential to enhance our comprehension of the pathological and homeostatic distinctions within extramedullary niches, spanning from development to adulthood.
Author contributions
GR-T: Conceptualization, Investigation, Writing – original draft, Writing – review & editing. MM: Funding acquisition, Supervision, Writing – review & editing. RV-R: Conceptualization, Supervision, Writing – review & editing.
Funding
The author(s) declare financial support was received for the research, authorship, and/or publication of this article. This work was supported, in part, by the National Institute of Allergy and Infectious Diseases (R01AI168322) of the National Institutes of Health.
Conflict of interest
The authors declare that the research was conducted in the absence of any commercial or financial relationships that could be construed as a potential conflict of interest.
The author(s) declared that they were an editorial board member of Frontiers, at the time of submission. This had no impact on the peer review process and the final decision.
Publisher’s note
All claims expressed in this article are solely those of the authors and do not necessarily represent those of their affiliated organizations, or those of the publisher, the editors and the reviewers. Any product that may be evaluated in this article, or claim that may be made by its manufacturer, is not guaranteed or endorsed by the publisher.
Author disclaimer
The content is solely the responsibility of the authors and does not necessarily represent the official views of the National Institute of Allergy and Infectious Diseases or the National Institutes of Health.
References
1. McCulloch EA, Till JE. The radiation sensitivity of normal mouse bone marrow cells, determined by quantitative marrow transplantation into irradiated mice. Radiat Res. (1960) 13:115–25. doi: 10.2307/3570877
2. Okada S, Nakauchi H, Nagayoshi K, Nishikawa S, Si N, Miura Y, et al. Enrichment and characterization of murine hematopoietic stem cells that express c-kit molecule. Blood. (1991) 78:1706–12. doi: 10.1182/blood.V78.7.1706.1706
3. Spangrude GJ, Heimfeld S, Weissman IL. Purification and characterization of mouse hematopoietic stem cells. Science. (1988) 241:58–62. doi: 10.1126/SCIENCE.2898810
4. Okada S, Nakauchi H, Nagayoshi K, lchi Nishikawa S, Miura Y, Suda T. In vivo and In vitro stem cell function of c-kit- and Sca-1–positive murine hematopoietic cells. Blood. (1992) 80:3044–50. doi: 10.1182/blood.V80.12.3044.3044
5. Ikuta K, Weissman IL. Evidence that hematopoietic stem cells express mouse c-kit but do not depend on steel factor for their generation. Proc Natl Acad Sci. (1992) 89:1502–6. doi: 10.1073/pnas.89.4.1502
6. Wilson A, Oser GM, Jaworski M, Blanco-Bose WE, Laurenti E, Adolphe C, et al. Dormant and self-renewing hematopoietic stem cells and their niches. Ann New York Acad Sci. (2007) 1106:64–75. doi: 10.1196/annals.1392.021
7. Ogawa M, Tajima F, Ito T, Sato T, Laver JH, Deguchi T. CD34 expression by murine hematopoietic stem cells. developmental changes and kinetic alterations. Ann New York Acad Sci. (2001) 938:139–45. doi: 10.1111/j.1749-6632.2001.tb03583.x
8. Osawa M, Hanada K, Hamada H, Nakauchi H. Long-term lymphohematopoietic reconstitution by a single CD34-low/negative hematopoietic stem cell. Science. (1996) 273:242–5. doi: 10.1126/science.273.5272.242
9. Ito T, Tajima F, Ogawa M. Developmental changes of CD34 expression by murine hematopoietic stem cells. Exp Hematol. (2000) 28:1269–73. doi: 10.1016/S0301-472X(00)00535-X
10. Kowalczyk MS, Tirosh I, Heckl D, Rao TN, Dixit A, Haas BJ, et al. Single-cell RNA-seq reveals changes in cell cycle and differentiation programs upon aging of hematopoietic stem cells. Genome Res. (2015) 25:1860–72. doi: 10.1101/gr.192237.115
11. Pietras E, Reynaud D, Kang YA, Carlin D, Calero-Nieto F, Leavitt A, et al. Functionally distinct sub- sets of lineage-biased multipotent progenitors control blood production in normal and regenerative conditions. Cell Stem Cell. (2015) 17:35–46. doi: 10.1016/j.stem.2015.05.003
12. Li C, Johnson G. Murine hematopoietic stem and progenitor cells: I. enrichment biologic characterization. Blood. (1995) 85:1472–9. doi: 10.1182/blood.v85.6.1472.bloodjournal8561472
13. Kiel MJ, Yilmaz OH, Iwashita T, Yilmaz OH, Terhorst C, Morrison SJ. SLAM family receptors distinguish hematopoietic stem and progenitor cells and reveal endothelial niches for stem cells. Cell. (2005) 121:1109–21. doi: 10.1016/j.cell.2005.05.026
14. Dykstra B, Olthof S, Schreuder J, Ritsema M, de Haan G. Clonal analysis reveals multiple functional defects of aged murine hematopoietic stem cells. J Exp Med. (2011) 208:2691–703. doi: 10.1084/jem.20111490
15. Smith JNP, Cordova BA, Richardson B, Christo KF, Campanelli J, Broncano AV, et al. Sex-specific niche signaling contributes to sexual dimorphism following stem cell transplantation. bioRxiv. (2022). doi: 10.1101/2022.06.13.495897
16. de Haan G, Nijhof W, Van Zant G. Mouse strain-dependent changes in frequency and proliferation of hematopoietic stem cells during aging: correlation between lifespan and cycling activity. Blood. (1997) 89:1543–50. doi: 10.1182/blood.v89.5.1543
17. Spangrude GJ, Brooks DM. Mouse strain variability in the expression of the hematopoietic stem cell antigen ly-6A/E by bone marrow cells. Blood. (1993) 82:3327–32. doi: 10.1182/blood.v82.11.3327
18. Goodell MA, Rosenzweig M, Kim H, Marks DF, DeMaria M, Paradis G, et al. Dye efflux studies suggest that hematopoietic stem cells expressing low or undetectable levels of CD34 antigen exist in multiple species. Nat Med. (1997) 3:1337–45. doi: 10.1038/nm1297-1337
19. Andrews RG, Singer JW, Bernstein ID. Human hematopoietic precursors in long-term culture: single CD34+ cells that lack detectable t cell, b cell, and myeloid cell antigens produce multiple colony- forming cells when cultured with marrow stromal cells. J Exp Med. (1990) 172:355–8. doi: 10.1084/jem.172.1.355
20. Muench MO, Roncarolo MG, Namikawa R. Phenotypic and functional evidence for the expression of CD4 by hematopoietic stem cells isolated from human fetal liver. Blood. (1997) 89:1364–75. doi: 10.1182/blood.V89.4.1364
21. Baum CM, Weissman IL, Tsukamoto AS, Buckle AM, Peault B. Isolation of a candidate human hematopoietic stem-cell population. Proc Natl Acad Sci United States America. (1992) 89:2804–8. doi: 10.1073/pnas.89.7.2804
22. Yin AH, Miraglia S, Zanjani ED, Almeida-Porada G, Ogawa M, Leary AG, et al. AC133, a novel marker for human hematopoietic stem and progenitor cells. Blood. (1997) 90:5002–12. doi: 10.1182/blood.V90.12.5002
23. Anjos-Afonso F, Buettner F, Mian SA, Rhys H, Perez-Lloret J, Garcia-Albornoz M, et al. Single cell analyses identify a highly regenerative and homogenous human CD34+ hematopoietic stem cell population. Nat Commun. (2022) 13:2048. doi: 10.1038/s41467-022-29675-w
24. Terstappen LW, Huang S, Safford M, Lansdorp PM, Loken MR. Sequential generations of hematopoietic colonies derived from single nonlineage-committed CD34+CD38- progenitor cells. Blood. (1991) 77:1218–27.
25. Issaad C, Croisille L, Katz A, Vainchenker W, Coulombel L. A murine stromal cell line allows the proliferation of very primitive human CD34++/CD38– progenitor cells in long-term cultures and semisolid assays. Blood. (1993) 81:2916–24. doi: 10.1182/blood.V81.11.2916.2916
26. Muench MO, Cupp J, Polakoff J, Roncarolo MG. Expression of CD33, CD38, and HLA-DR on CD34+ human fetal liver progenitors with a high proliferative potential. Blood. (1994) 83:3170–81. doi: 10.1182/blood.V83.11.3170.3170
27. Lansdorp PM, Sutherland HJ, Eaves CJ. Selective expression of CD45 isoforms on functional subpopulations of CD34+ hemopoietic cells from human bone marrow. J Exp Med. (1990) 172:363—–366. doi: 10.1084/jem.172.1.363
28. Lansdorp PM, Dragowska W. Long-term erythropoiesis from constant numbers of CD34+ cells in serum-free cultures initiated with highly purified progenitor cells from human bone marrow. J Exp Med. (1992) 175:1501–9. doi: 10.1084/jem.175.6.1501
29. Abe T, Matsuoka Y, Nagao Y, Sonoda Y, Hanazono Y. CD34-negative hematopoietic stem cells show distinct expression profiles of homing molecules that limit engraftment in mice and sheep. Int J Hematol. (2017) 106:631–7. doi: 10.1007/s12185-017-2290-5
30. Matsuoka Y, Sumide K, Kawamura H, Nakatsuka R, Fujioka T, Sonoda Y. GPI-80 expression highly purifies human cord blood–derived primitive CD34-negative hematopoietic stem cells. Blood. (2016) 128:2258–60. doi: 10.1182/blood-2016-03-704668
31. Anjos-Afonso F, Currie E, Palmer HG, Foster KE, Taussig DC, Bonnet D. CD34(-) cells at the apex of the human hematopoietic stem cell hierarchy have distinctive cellular and molecular signatures. Cell Stem Cell. (2013) 13:161–74. doi: 10.1016/j.stem.2013.05.025
32. Takahashi M, Matsuoka Y, Sumide K, Nakatsuka R, Fujioka T, Kohno H, et al. CD133 is a positive marker for a distinct class of primitive human cord blood-derived CD34-negative hematopoietic stem cells. Leukemia. (2014) 28:1308–15. doi: 10.1038/leu.2013.326
33. Sumide K, Matsuoka Y, Kawamura H, Nakatsuka R, Fujioka T, Asano H, et al. A revised road map for the commitment of human cord blood CD34-negative hematopoietic stem cells. Nat Commun. (2018) 9:2202. doi: 10.1038/s41467-018-04441-z
34. Baron MH, Isern J, Fraser ST. The embryonic origins of erythropoiesis in mammals. Blood. (2012) 119:4828–37. doi: 10.1182/blood-2012-01-153486
35. Enders AC, King BF. The human yolk sac and yolk sac tumors (Springer berlin heidelberg), chap. Dev Hum Yolk Sac. (1993). doi: 10.1007/978-3-642-77852-02
36. Migliaccio G, Migliaccio AR. The human yolk sac and yolk sac tumors (Springer berlin heidelberg), chap. Kinetics Hematopoiesis Hum Yolk Sac. (1993), 70–83. doi: 10.1007/978-3-642-77852-04
37. Garcia-Porrero JA, Godin IE, Dieterlen-Lièvre F. Potential intraembryonic hemogenic sites at pre-liver stages in the mouse. Anat Embryology. (1995) 192:425–35. doi: 10.1007/BF00240375
38. Tavian M, Robin C, Coulombel L, Peault B. The human embryo, but not its yolk sac, generates lympho-myeloid stem cells: Mapping multipotent hematopoietic cell fate in intraembryonic mesoderm. Immunity. (2001) 15:487–95. doi: 10.1016/S1074-7613(01)00193-5
39. Godin I, Dieterlen-Lièvre F, Cumano A. Emergence of multipotent hemopoietic cells in the yolk sac and paraaortic splanchnopleura in mouse embryos, beginning at 8. 5 days postcoitus. Proc Natl Acad Sci. (1995) 92:773–7. doi: 10.1073/pnas.92.3.773
40. Godin IE, Garcia-Porrero JA, Coutinho A, Dieterlen-Lièvre F, Marcos MAR. Para-aortic splanchnopleura from early mouse embryos contains B1a cell progenitors. Nature. (1993) 364:67–70. doi: 10.1038/364067a0
41. Medvinsky AL, Samoylina NL, Müller AM, Dzierzak EA. An early pre-liver intraembryonic source of CFU-s in the developing mouse. Nature. (1993) 364:64–7. doi: 10.1038/364064a0
42. Medvinsky A, Dzierzak E. Definitive hematopoiesis is autonomously initiated by the agm region. Cell. (1996) 86:897–906. doi: 10.1016/S0092-8674(00)80165-8
43. Gekas C, Dieterlen-Lièvre F, Orkin SH, Mikkola HKAA. The placenta is a niche for hematopoietic stem cells. Dev Cell. (2005) 8:365–75. doi: 10.1016/j.devcel.2004.12.016
44. Challier JC, Galtier M, Cortez A, Bintein T, Rabreau M, Uzan S. Immunocytological evidence for hematopoiesis in the early human placenta. Placenta. (2005) 26:282–8.
45. Robin C, Bollerot K, Mendes S, Haak E, Crisan M, Cerisoli F, et al. Human placenta is a potent hematopoietic niche containing hematopoietic stem and progenitor cells throughout development. Cell Stem Cell. (2009) 5:385–95. doi: 10.1016/j.stem.2009.08.020
46. Bárcena A, Muench MO, Kapidzic M, Gormley M, Goldfien GA, Fisher SJ. Human placenta and chorion: potential additional sources of hematopoietic stem cells for transplantation. Transfusion. (2011) 51 Suppl 4:94–105. doi: 10.1111/j.1537-2995.2011.03372.x
47. Muench MO, Kapidzic M, Gormley M, Gutierrez AG, Ponder KL, Fomin ME, et al. The human chorion contains definitive hematopoietic stem cells from the fifteenth week of gestation. Development. (2017) 144:1399–411. doi: 10.1242/dev.138438
48. Thomas JR, Appios A, Calderbank EF, Yoshida N, Zhao X, Hamilton RS, et al. Primitive haematopoiesis in the human placenta gives rise to macrophages with epigenetically silenced HLA-DR. Nat Commun. (2023) 14:1764. doi: 10.1038/s41467-023-37383-2
49. Sánchez MJ, Holmes A, Miles C, Dzierzak E. Characterization of the first definitive hematopoietic stem cells in the agm and liver of the mouse embryo. Immunity. (1996) 5:513–25. doi: 10.1016/s1074-7613(00)80267-8
50. Tavian M, Hallais MF, Péault B, Peault B. Emergence of intraembryonic hematopoietic precursors in the pre-liver human embryo. Development. (1999) 126:793 LP–803. doi: 10.1242/dev.126.4.793
51. Tavian M, Péault B. Embryonic development of the human hematopoietic system. Int J Dev Biol. (2005) 49:243–50. doi: 10.1387/ijdb.041957mt
52. Wright DE, Bowman EP, Wagers AJ, Butcher EC, Weissman IL. Hematopoietic stem cells are uniquely selective in their migratory response to chemokines. J Exp Med. (2002) 195:1145–54. doi: 10.1084/jem.20011284
53. Johnson GR, Jones RO. Differentiation of the mammalian hepatic primordium in vitro: I. morphogenesis and the onset of haematopoiesis. Development. (1973) 30:83–96. doi: 10.1242/dev.30.1
54. Matsumoto K, Yoshitomi H, Rossant J, Zaret KS. Liver organogenesis promoted by endothelial cells prior to vascular function. Science. (2001) 294:559–63. doi: 10.1126/science.1063889
55. Zovein AC, Turlo KA, Ponec RM, Lynch MR, Chen KC, Hofmann JJ, et al. Vascular remodeling of the vitelline artery initiates extravascular emergence of hematopoietic clusters. Blood. (2010) 116:3435–44. doi: 10.1182/blood-2010-04-279497
56. Gomez Perdiguero E, Klapproth K, Schulz C, Busch K, Azzoni E, Crozet L, et al. Tissue-resident macrophages originate from yolk-sac-derived erythro-myeloid progenitors. Nature. (2015) 518:547–51. doi: 10.1038/nature13989
57. Kumaravelu P, Hook L, Morrison AM, Ure J, Zhao S, Zuyev S, et al. Quantitative developmental anatomy of definitive haematopoietic stem cells/long-term repopulating units (HSC/RUs): role of the aorta-gonad-mesonephros (AGM) region and the yolk sac in colonisation of the mouse embryonic liver. Development. (2002) 129:4891–9. doi: 10.1242/dev.129.21.4891
58. Ema H, Nakauchi H. Expansion of hematopoietic stem cells in the developing liver of a mouse embryo. Blood. (2000) 95:2284–8. doi: 10.1182/blood.V95.7.2284
59. Migliaccio G, Migliaccio AR, Petti S, Mavilio F, Russo G, Lazzaro D, et al. Human embryonic hemopoiesis. kinetics of progenitors and precursors underlying the yolk sac–-liver transition. J Clin Invest. (1986) 78:51–60. doi: 10.1172/JCI112572
60. Calvanese V, Capellera-Garcia S, Ma F, Fares I, Liebscher S, Ng ES, et al. Mapping human haematopoietic stem cells from haemogenic endothelium to birth. Nature. (2022) 604:534–40. doi: 10.1038/s41586-022-04571-x
61. Oberlin E, Fleury M, Clay D, Petit-Cocault L, Candelier JJ, Mennesson B, et al. VE-cadherin expression allows identification of a new class of hematopoietic stem cells within human embryonic liver. Blood. (2010) 116:4444–55. doi: 10.1182/blood-2010-03-272625
62. Ivanovs A, Rybtsov S, Welch L, Anderson RA, Turner ML, Medvinsky A. Highly potent human hematopoietic stem cells first emerge in the intraembryonic aorta-gonad-mesonephros region. J Exp Med. (2011) 208:2417–27. doi: 10.1084/jem.20111688
63. Easterbrook J, Rybtsov S, Gordon-Keylock S, Ivanovs A, Taoudi S, Anderson RA, et al. Analysis of the spatiotemporal development of hematopoietic stem and progenitor cells in the early human embryo. Stem Cell Rep. (2019) 12:1056–68. doi: 10.1016/j.stemcr.2019.03.003
64. Ivanovs A, Rybtsov S, Anderson RA, Medvinsky A. Vast self-renewal potential of human agm region hscs dramatically declines in the umbilical cord blood. Stem Cell Rep. (2020) 15:811–6. doi: 10.1016/j.stemcr.2020.08.008
65. Golfier F, Bárcena A, Cruz J, Harrison MR, Muench MO. Mid-trimester fetal livers are a rich source of CD34+/++ cells for transplantation. Bone Marrow Transplant. (1999) 24:451–61. doi: 10.1038/sj.bmt.1701940
66. Rowley PT, Ohlsson-Wilhelm BM, Farley BA. Erythroid colony formation from human fetal liver. Proc Natl Acad Sci. (1978) 75:984–8. doi: 10.1073/pnas.75.2.984
67. Muench MO, Namikawa R. Disparate regulation of human fetal erythropoiesis by the microenvironments of the liver and bone marrow. Blood Cells Molecules Dis. (2001) 27:377–90. doi: 10.1006/bcmd.2001.0393
68. Muench MO. Stem cells and progenitors in liver development. Vol. 1. Morgan & Claypool Life Science Publishers (2012) p. 1–126. doi: 10.4199/c00070ed1v01y201212scb003
69. Kamps WA, Cooper MD. Microenvironmental studies of pre-b and b cell development in human and mouse fetuses. J Immunol. (1982) 129:526–31.
70. Rosenthal P, Rimm IJ, Umiel T, Griffin JD, Osathanondh R, Schlossman SF, et al. Ontogeny of human hematopoietic cells: analysis utilizing monoclonal antibodies. J Immunol. (1983) 131:232–7. doi: 10.4049/jimmunol.131.1.232
71. Bárcena A, Muench MO, Galy AHM, Cupp J, Roncarolo MG, Phillips JH, et al. Phenotypic and functional analysis of t-cell precursors in the human fetal liver and thymus: CD7 expression in the early stages of t- and myeloid-cell development. Blood. (1993) 82:3401–14. doi: 10.1182/blood.V82.11.3401.3401
72. Popescu DM, Botting RA, Stephenson E, Green K, Webb S, Jardine L, et al. Decoding human fetal liver haematopoiesis. Nature. (2019) 574:365–71. doi: 10.1038/s41586-019-1652-y
73. Schneider E, Moreau G, Arnould A, Vasseur F, Khodabaccus N, Dy M, et al. Increased fetal and extramedullary hematopoiesis in fas-deficient C57BL/6-lpr/lpr mice. Blood. (1999) 94:2613–21.
74. Griffin DO, Holodick NE, Rothstein TL. Human B1 cells in umbilical cord and adult peripheral blood express the novel phenotype CD20+CD27+CD43+CD70-. J Exp Med. (2011) 208:67–80. doi: 10.1084/jem.20101499
75. Ito C, Yamazaki H, Yamane T. Earliest hematopoietic progenitors at embryonic day 9 preferentially generate b-1 b cells rather than follicular b or marginal zone b cells. Biochem Biophys Res Commun. (2013) 437:307–13. doi: 10.1016/j.bbrc.2013.06.073
76. Smith FL, Baumgarth N. B-1 cell responses to infections. Curr Opin Immunol. (2019) 57:23–31. doi: 10.1016/j.coi.2018.12.001
77. Suo C, Dann E, Goh I, Jardine L, Kleshchevnikov V, Park JE, et al. Mapping the developing human immune system across organs. Science. (2022) 376. doi: 10.1126/science.abo0510
78. Hall TD, Kim H, Dabbah M, Myers JA, Crawford JC, Morales-Hernandez A, et al. Murine fetal bone marrow does not support functional hematopoietic stem and progenitor cells until birth. Nat Commun. (2022) 13:5403. doi: 10.1038/s41467-022-33092-4
79. Charbord P, Tavian M, Humeau L, Peault B. Early ontogeny of the human marrow from long bones: an immunohistochemical study of hematopoiesis and its microenvironment. Blood. (1996) 87:4109–19. doi: 10.1182/blood.V87.10.4109.bloodjournal87104109
80. Liu Y, Chen Q, Jeong HW, Koh BI, Watson EC, Xu C, et al. A specialized bone marrow micro- environment for fetal haematopoiesis. Nat Commun. (2022) 13:1327. doi: 10.1038/s41467-022-28775-x
81. Zanjani ED, Ascensao JL, Tavassoli M. Liver-derived fetal hematopoietic stem cells selectively and preferentially home to the fetal bone marrow. Blood. (1993) 81:399–404. doi: 10.1182/blood.v81.2
82. Wyrsch A, dalle Carbonare V, Jansen W, Chklovskaia E, Nissen C, Surbek D, et al. Umbilical cord blood from preterm human fetuses is rich in committed and primitive hematopoietic progenitors with high proliferative and self-renewal capacity. Exp Hematol. (1999) 27:1338–45. doi: 10.1016/S0301-472X(99)00059-4
83. Campagnoli C, Fisk N, Overton T, Bennett P, Watts T, Roberts I. Circulating hematopoietic progenitor cells in first trimester fetal blood. Blood. (2000) 95:1967–72. doi: 10.1182/blood
84. Zheng Z, He H, Tang XT, Zhang H, Gou F, Yang H, et al. Uncovering the emergence of HSCs in the human fetal bone marrow by single-cell RNA-seq analysis. Cell Stem Cell. (2022) 29:1562–79. doi: 10.1016/j.stem.2022.10.005
85. Thomas DB. Is the spleen a preferential site of blood cell production in the human fetus? Ital J Anat embryology. (1995) 100:245–51.
86. Calhoun DA, Li Y, Braylan RC, Christensen RD. Assessment of the contribution of the spleen to granulocytopoiesis and erythropoiesis of the mid-gestation human fetus. Early Hum Dev. (1996) 46:217–27. doi: 10.1016/0378-3782(96)01765-3
87. Kim C. Homeostatic and pathogenic extramedullary hematopoiesis. J Blood Med. (2010) 13. doi: 10.2147/jbm.s7224
88. Espín-Palazón R, Stachura D, Campbell C, García-Moreno D, Del Cid N, Kim A, et al. Proinflammatory signaling regulates hematopoietic stem cell emergence. Cell. (2014) 159:1070–85. doi: 10.1016/j.cell.2014.10.031
89. Roberts A, Shetty A, Mellnick V, Pickhardt P, Bhalla S, Menias C. Extramedullary haematopoiesis: radiological imaging features. Clin Radiol. (2016) 71:807–14. doi: 10.1016/j
90. Li R, Reddy VVB, Palmer CA. Extramedullary hematopoiesis: an unusual finding in subdural hematomas. Case Rep Pathol. (2011) 2011:1–3. doi: 10.1155/2011/718585
91. Shimony N, Cagnano E, Kanner AA. Extramedullary hematopoiesis presenting as spontaneous recurrent chronic subdural hematoma: Case report and review of the literature. Interdiscip Neurosurgery: Advanced Techniques Case Manage. (2018) 14:47–9.
92. Eskazan AE, Ar MC, Baslar Z. Intracranial extramedullary hematopoiesis in patients with thalassemia: a case report and review of the literature. Transfusion. (2012) 52:1715–20. doi: 10.1111/j.1537-2995
93. Singer A, Quencer R. Intracranial extramedullary hematopoiesis: a rare cause of headaches. J Neuroimaging. (2014) 24:524–7. doi: 10.1111/jon.12029
94. Mattei TA, Higgins M, Joseph F, Mendel E. Ectopic extramedullary hematopoiesis: Evaluation and treatment of a rare and benign paraspinal/epidural tumor. J Neurosurgery: Spine. (2013) 18:236–42. doi: 10.3171/2012.12.SPINE12720
95. Katchi T, Kolandaivel K, Khattar P, Farooq T, Islam H, Liu D. Extramedullary hematopoiesis presented as cytopenia and massive paraspinal masses leading to cord compression in a patient with hereditary persistence of fetal hemoglobin. biomark Res. (2016) 4:17–7. doi: 10.1186/s40364-016-0071-6
96. Wang A, Carberry N, Solli E, Gillick J, Islam H, Hillard V. Spinal cord compression secondary to extramedullary hematopoiesis: case report and review of the literature. Case Rep Oncol. (2016) 9:290–7. doi: 10.1159/000446473
97. La VT, Diatte M, Gaston J, Dick D, Sweiss R, Pakbaz Z. Spinal cord compression due to extramedullary hematopoiesis in a patient with e-beta-thalassemia managed without radiation or surgery. J Community Hosp Internal Med Perspect. (2018) 8:246–9. doi: 10.1080/20009666.2018.1490141
98. Abdulla MA, Yassin MA, Abdelrazek M, Mudawi D, Ibrahim F, Soliman DS, et al. A persistent cough as atypical clinical presentation of intrathoracic extramedullary hematopoiesis (EMH) in a female with thalassemia intermedia. Acta Biomedica Atenei Parmensis. (2018) 89:41–6. doi: 10.23750/abm
99. An J, Weng Y, He J, Li Y, Huang S, Cai S, et al. Intrathoracic extramedullary hematopoiesis presenting as tumor-simulating lesions of the mediastinum in α-thalassemia: A case report. Oncol Lett. (2015) 10:1993–6.
100. Pinato DJ, Tan W, Gately A. An unexpected cause of pulmonary cannonball lesion. J Thorac Oncol. (2014) 9:259–9. doi: 10.1097/JTO.0b013e3182a85298
101. Monga V, Silverman M. Pulmonary extramedullary hematopoiesis involving the pulmonary artery. Hematol Rep. (2015) 7:9–11. doi: 10.4081/hr.2015.5714
102. Chute DJ, Fowler DR. Fatal hemothorax due to rupture of an intrathoracic extramedullary hematopoietic nodule. Am J Forensic Med Pathol. (2004) 25:74–7. doi: 10.1097/01.paf.0000113859.48471.49
103. Rumi E, Passamonti F, Boveri E, De Amici M, Astori C, Braschi M, et al. Dyspnea secondary to pulmonary hematopoiesis as presenting symptom of myelofibrosis with myeloid metaplasia. Am J Hematol. (2006) 81:124–7. doi: 10.1002/ajh.20509
104. Ozbudak IH, Shilo K, Hale S, Aguilera NS, Galvin JR, Franks TJ. Alveolar airspace and pulmonary artery involvement by extramedullary hematopoiesis: a unique manifestation of myelofibrosis. Arch Pathol Lab Med. (2008) 132:99–103. doi: 10.5858/2008-132-99-aaapai
105. Wiener MD, Halvorsen RA, Vollmer RT, Foster WL, Roberts L. Focal intrahepatic extramedullary hematopoiesis mimicking neoplasm. Am J Roentgenology. (1987) 149:1171–2. doi: 10.2214/ajr.149.6.1171
106. Paunović M, Vukčević B, Terzić Z, Magdelinić M, Vučković L, Raonić J, et al. Cavernous hemangioma of the liver with extramedullary hematopoiesis and malignant cells embolization in an elderly patient: a case report. Acta Med Medianae. (2020) 59:114–9. doi: 10.5633/amm.2020.0216
107. Cao D, Wang Y. Gastrointestinal: Intrahepatic periportal masses with uncommon computed tomography patterns: Hepatic extramedullary hematopoiesis of primary myelofibrosis. J Gastroenterol Hepatol. (2020) 35:1857–7. doi: 10.1111/jgh.15069
108. Navarro M, Crespo C, Pérez L, Martínez C, Galant J, González I. Massive intrahepatic extramedullary hematopoiesis in myelofibrosis. Abdominal Imaging. (2000) 25:184–6. doi: 10.1007/s002619910041
109. Laxer RM, Roberts EA, Gross KR, Britton JR, Cutz E, Dimmick J, et al. Liver disease in neonatal lupus erythematosus. J Pediatr. (1990) 116:238–42. doi: 10.1016/S0022-3476(05)82880-X
110. Conor O’keane J, Wolf BC, Neiman RS. The pathogenesis of splenic extramedullary hematopoiesis in metastatic carcinoma. Cancer. (1989) 63:1539–43. doi: 10.1002/1097-0142(19890415)63:8⟨1539::AID-CNCR2820630814⟩3.0.CO;2-5
111. Sato S, Tamai Y, Okada S, Kannbe E, Takeda K, Tanaka E. Atraumatic splenic rupture due to ectopic extramedullary hematopoiesis after autologous stem cell transplantation in a patient with al amyloidosis. Internal Med. (2018) 57:399–402. doi: 10.2169/internalmedicine.9018-17
112. Jantunen E, Hänninen A, Naukkarinen A, Vornanen M, Lahtinen R. Gray platelet syndrome with splenomegaly and signs of extramedullary hematopoiesis: A case report with review of the literature. Am J Hematol. (1994) 46:218–24. doi: 10.1002/ajh.2830460311
113. Robinson C, Boyce AM, Estrada A, Kleiner DE, Mathew R, Stanton R, et al. Bone marrow failure and extramedullary hematopoiesis in McCune-albright syndrome. Osteoporosis Int. (2018) 29:237–41. doi: 10.1007/s00198-017-4217-7
114. Fan N, Lavu S, Hanson CA, Tefferi A. Extramedullary hematopoiesis in the absence of myeloproliferative neoplasm: Mayo clinic case series of 309 patients. Blood Cancer J. (2018) 8:119. doi: 10.1038/s41408-018-0156-6
115. Regan-Komito D, Swann JW, Demetriou P, Cohen ES, Horwood NJ, Sansom SN, et al. GM-CSF drives dysregulated hematopoietic stem cell activity and pathogenic extramedullary myelopoiesis in experimental spondyloarthritis. Nat Commun. (2020) 11:155. doi: 10.1038/s41467-019-13853-4
116. Lefrançais E, Ortiz-Muñoz G, Caudrillier A, Mallavia Bn, Liu F, Sayah DM, et al. The lung is a site of platelet biogenesis and a reservoir for haematopoietic progenitors. Nature. (2017) 544:105–9. doi: 10.1038/nature21706
117. Griseri T, McKenzie B, Schiering C, Powrie F. Dysregulated hematopoietic stem and progenitor cell activity promotes interleukin-23-driven chronic intestinal inflammation. Immunity. (2012) 37:1116–29. doi: 10.1016/j.immuni.2012.08.025
118. Baldane S, Sivgin S, Kaynar L, Ozaslan E, Yildirim A, Canoz O, et al. Pulmonary extramedullary hematopoiesis mimicking plasmacytoma in a patient with multiple myeloma. Romanian J Of Internal Med. (2015) 53:355–8. doi: 10.1515/rjim-2015-0046
119. Lynch L, O’Donoghue D, Dean J, O’Sullivan J, O’Farrelly C, Golden-Mason L. Detection and characterization of hemopoietic stem cells in the adult human small intestine. J Immunol (Baltimore Md.. (2006) 1950) 176:5199–204.
120. Fu J, Zuber J, Martinez M, Shonts B, Obradovic A, Wang H, et al. Human intestinal allografts contain functional hematopoietic stem and progenitor cells that are maintained by a circulating pool. Cell Stem Cell. (2019) 24:227–39. doi: 10.1016/j.stem.2018.11.007
121. Ye H, Adane B, Khan N, Sullivan T, Minhajuddin M, Gasparetto M, et al. Leukemic stem cells evade chemotherapy by metabolic adaptation to an adipose tissue niche. Cell Stem Cell. (2016) 19:23–37. doi: 10.1016/j.stem.2016.06.001
122. Miñana MD, Carbonell-Uberos F, Mirabet V, Marín S, Encabo A. IFATS collection: identification of hemangioblasts in the adult human adipose tissue. Stem Cells. (2008) 26:2696–704. doi: 10.1634/stemcells.2007-0988
123. Han J, Koh YJ, Moon HR, Ryoo HG, Cho CH, Kim I, et al. Adipose tissue is an extramedullary reservoir for functional hematopoietic stem and progenitor cells. Blood. (2010) 115:957–64. doi: 10.1182/blood-2009–05-219923
124. Xu Y, Sun P, Wang J, Li Z, Gao R, Wang X, et al. Differentiation of CD45-/CD31+ lung side population cells into endothelial and smooth muscle cells in vitro. Int J Mol Med. (2019) 43:1128–38. doi: 10.3892/ijmm.2019.4053
125. Popescu LM, Gherghiceanu M, Suciu LC, Manole CG, Hinescu ME. Telocytes and putative stem cells in the lungs: Electron microscopy, electron tomography and laser scanning microscopy. Cell Tissue Res. (2011) 345:391–403. doi: 10.1007/s00441-011-1229-z
126. Borges I, Sena I, Azevedo P, Andreotti J, Almeida V, Paiva A, et al. Lung as a niche for hematopoietic progenitors. Stem Cell Rev Rep. (2017) 13:567–74. doi: 10.1007/s12015-017-9747-z
127. Travaglini KJ, Nabhan AN, Penland L, Sinha R, Gillich A, Sit RV, et al. A molecular cell atlas of the human lung from single-cell RNA sequencing. Nature. (2020) 587:619–25. doi: 10.1038/s41586-020-2922-4
128. Oda A, Tezuka T, Ueno Y, Hosoda S, Amemiya Y, Notsu C, et al. Niche-induced extramedullary hematopoiesis in the spleen is regulated by the transcription factor Tlx1. Sci Rep. (2018) 8:1–16. doi: 10.1038/s41598-018-26693-x
129. Cardier JE, Barbera´-Guillem E. Extramedullary hematopoiesis in the adult mouse liver is associated with specific hepatic sinusoidal endothelial cells. Hepatology. (1997) 26:165–75. doi: 10.1002/hep
130. Muench MO, Beyer AI, Fomin ME, Thakker R, Mulvaney US, Nakamura M, et al. The adult livers of immunodeficient mice support human hematopoiesis: evidence for a hepatic mast cell population that develops early in human ontogeny. PloS One. (2014) 9:e97312. doi: 10.1371/journal.pone.0097312
131. Ayala R, Grande S, Albizua E, Crooke A, Meneu JC, Moreno A, et al. Long-term follow-up of donor chimerism and tolerance after human liver transplantation. Liver Transplant. (2009) 15:581–91. doi: 10.1002/lt.21736
132. Wang XQ, Lo CM, Chen L, Cheung CK, Yang ZF, Chen YX, et al. Hematopoietic chimerism in liver transplantation patients and hematopoietic stem/progenitor cells in adult human liver. Hepatology. (2012) 56:1557–66. doi: 10.1002/hep.25820
133. Alexander SI, Smith N, Hu M, Verran D, Shun A, Dorney S, et al. Chimerism and tolerance in a recipient of a deceased-donor liver transplant. New Engl J Med. (2008) 358:369–74. doi: 10.1056/NEJMoa0707255
134. Morrison SJ, Scadden DT. The bone marrow niche for haematopoietic stem cells. Nature. (2014) 505:327–34. doi: 10.1038/nature12984
135. Ding L, Saunders TL, Enikolopov G, Morrison SJ. Endothelial and perivascular cells maintain haematopoietic stem cells. Nature. (2012) 481:457–62. doi: 10.1038/nature10783
136. Comazzetto S, Murphy MM, Berto S, Jeffery E, Zhao Z, Morrison SJ. Restricted hematopoietic progenitors and erythropoiesis require SCF from leptin receptor+ niche cells in the bone marrow. Cell Stem Cell. (2019) 24:477–86. doi: 10.1016/j.stem.2018.11.022
137. Sugiyama T, Kohara H, Noda M, Nagasawa T. Maintenance of the hematopoietic stem cell pool by CXCL12-CXCR4 chemokine signaling in bone marrow stromal cell niches. Immunity. (2006) 25:977–88. doi: 10.1016/j.immuni.2006.10.016
138. Greenbaum A, Hsu YMS, Day RB, Schuettpelz LG, Christopher MJ, Borgerding JN, et al. CXCL12 in early mesenchymal progenitors is required for haematopoietic stem-cell maintenance. Nature. (2013) 495:227–30. doi: 10.1038/nature11926
139. Arai F, Hirao A, Ohmura M, Sato H, Matsuoka S, Takubo K, et al. Tie2/angiopoietin-1 signaling regulates hematopoietic stem cell quiescence in the bone marrow niche. Cell. (2004) 118:149–61. doi: 10.1016/j.cell.2004.07.004
140. Yoshihara H, Arai F, Hosokawa K, Hagiwara T, Takubo K, Nakamura Y, et al. Thrombopoietin/MPL signaling regulates hematopoietic stem cell quiescence and interaction with the osteoblastic niche. Cell Stem Cell. (2007) 1:685–97. doi: 10.1016/j.stem.2007.10.020
141. Acar M, Kocherlakota KS, Murphy MM, Peyer JG, Oguro H, Inra CN, et al. Deep imaging of bone marrow shows non-dividing stem cells are mainly perisinusoidal. Nature. (2015) 526:126–30. doi: 10.1038/nature15250
142. Zhang CC, Lodish HF. Cytokines regulating hematopoietic stem cell function. Curr Opin Hematol. (2008) 15:307–11. doi: 10.1097/MOH.0b013e3283007db5
143. Morrison SJ, Wright DE, Weissman IL. Cyclophosphamide/granulocyte colony-stimulating factor induces hematopoietic stem cells to proliferate prior to mobilization. Proc Natl Acad Sci United States America. (1997) 94:1908–13. doi: 10.1073/pnas.94.5.1908
144. Essers MAG, Offner S, Blanco-Bose WE, Waibler Z, Kalinke U, Duchosal MA, et al. IFNα activates dormant haematopoietic stem cells in vivo. Nature. (2009) 458:904–8. doi: 10.1038/nature07815
145. Baldridge MT, King KY, Boles NC, Weksberg DC, Goodell MA. Quiescent haematopoietic stem cells are activated by IFN-γ in response to chronic infection. Nature. (2010) 465:793–7. doi: 10.1038/nature09135
146. Boiko JR, Borghesi L. Hematopoiesis sculpted by pathogens: Toll-like receptors and inflammatory mediators directly activate stem cells. Cytokine. (2012) 57:1–8.
147. Nagai Y, Garrett KP, Ohta S, Bahrun U, Kouro T, Akira S, et al. Toll-like receptors on hematopoietic progenitor cells stimulate innate immune system replenishment. Immunity. (2006) 24:801–12. doi: 10.1016/j.immuni.2006.04.008
148. Thomas J, Liu F, Link DC. Mechanisms of mobilization of hematopoietic progenitors with granulocyte colony-stimulating factor. Curr Opin Hematol. (2002) 9:183–9. doi: 10.1097/00062752-200205000-00002
149. Muramoto GG, Chen B, Cui X, Chao NJ, Chute JP. Vascular endothelial cells produce soluble factors that mediate the recovery of human hematopoietic stem cells after radiation injury. Biol Blood Marrow Transplant. (2006) 12:530–40. doi: 10.1016/j.bbmt.2005.12.039
150. Boettcher S, Gerosa RC, Radpour R, Bauer J, Ampenberger F, Heikenwalder M, et al. Endothelial cells translate pathogen signals into g-CSF-driven emergency granulopoiesis. Blood. (2014) 124:1393–403. doi: 10.1182/blood-2014-04-570762
151. Chou KJ, Hsu CY, Huang CW, Chen HY, Ou SH, Chen CL, et al. Secretome of hypoxic endothelial cells stimulates bone marrow-derived mesenchymal stem cells to enhance alternative activation of macrophages. Int J Mol Sci. (2020) 21:4409–9. doi: 10.3390/ijms21124409
152. Jacobsen SE, Okkenhaug C, Veiby OP, Caput D, Ferrara P, Minty A. Interleukin 13: novel role in direct regulation of proliferation and differentiation of primitive hematopoietic progenitor cells. J Exp Med. (1994) 180:75–82. doi: 10.1084/jem.180.1.75
153. Schürch CM, Riether C, Ochsenbein AF. Cytotoxic CD8+ t cells stimulate hematopoietic progenitors by promoting cytokine release from bone marrow mesenchymal stromal cells. Cell Stem Cell. (2014) 14:460–72. doi: 10.1016/j.stem.2014.01.002
154. Mizuno K, Higuchi O, Ihle JN, Nakamura T. Hepatocyte growth factor stimulates growth of hematopoietic progenitor cells. Biochem Biophys Res Commun. (1993) 194:178–86. doi: 10.1006/bbrc.1993.1801
155. Prasanna SJ, Gopalakrishnan D, Shankar SR, Vasandan AB. Pro-inflammatory cytokines, IFNγ and TNFα, influence immune properties of human bone marrow and wharton jelly mesenchymal stem cells differentially. PloS One. (2010) 5:9016–6. doi: 10.1371/journal.pone.0009016
156. Morrison SJ, Spradling AC. Stem cells and niches: mechanisms that promote stem cell maintenance throughout life. Cell. (2008) 132:598–611. doi: 10.1016/j.cell.2008.01.038
157. Yang X, Chen D, Long H, Zhu B. The mechanisms of pathological extramedullary hematopoiesis in diseases. Cell Mol Life Sci. (2020) 77:2723–38. doi: 10.1007/s00018-020-03450-w
158. Mendt M, Cardier JE. Stromal-derived factor-1 and its receptor, CXCR4, are constitutively expressed by mouse liver sinusoidal endothelial cells: Implications for the regulation of hematopoietic cell migration to the liver during extramedullary hematopoiesis. Stem Cells Dev. (2012) 21:2142–51. doi: 10.1089/scd.2011.0565
159. Inra CN, Zhou BO, Acar M, Murphy MM, Richardson J, Zhao Z, et al. A perisinusoidal niche for extramedullary haematopoiesis in the spleen. Nature. (2015) 527:466–71. doi: 10.1038/nature15530
160. Perez LE, Alpdogan O, Shieh JH, Wong D, Merzouk A, Salari H, et al. Increased plasma levels of stromal-derived factor-1 (SDF-1/CXCL12) enhance human thrombopoiesis and mobilize human colony-forming cells (CFC) in NOD/SCID mice. Exp Hematol. (2004) 32:300–7. doi: 10.1016/j.exphem.2003.12.005
161. Miwa Y, Hayashi T, Suzuki S, Abe S, Onishi I, Kirimura S, et al. Up-regulated expression of CXCL12 in human spleens with extramedullary haematopoiesis. Pathology. (2013) 45:408–16. doi: 10.1097/PAT.0b013e3283613dbf
162. Ziegler SF, Roan F, Bell BD, Stoklasek TA, Kitajima M, Han H. The biology of thymic stromal lymphopoietin (TSLP). Adv Pharmacol. (2013) 66:129–55. doi: 10.1016/B978-0-12-404717-4.00004-4
163. Cianferoni A, Spergel J. The importance of TSLP in allergic disease and its role as a potential therapeutic target. Expert Rev Clin Immunol. (2014) 10:1463–74. doi: 10.1586/1744666X
164. Zhang Y, Zhou B. Functions of thymic stromal lymphopoietin in immunity and disease. Immunologic Res. (2012) 52:211–23. doi: 10.1007/s12026-012-8264-z
165. Siracusa MC, Saenz SA, Tait Wojno ED, Kim BS, Osborne LC, Ziegler CG, et al. Thymic stromal lymphopoietin-mediated extramedullary hematopoiesis promotes allergic inflammation. Immunity. (2013) 39:1158–70. doi: 10.1016/j.immuni.2013.09.016
166. Rivera-Torruco G, Martínez-Mendiola CA, Angeles-Floriano T, Jaimes-Ortega GA, Maravillas- Montero JL, García-Contreras R, et al. Isthmin 1 is expressed by progenitor-like cells in the lung: phenotypical analysis of isthmin 1+ hematopoietic stem-like cells in homeostasis and during infection. J Immunol Res. (2022) 2022:1–13. doi: 10.1155/2022/2909487
167. Osório L, Wu X, Zhou Z. Distinct spatiotemporal expression ofISM1during mouse and chick development. Cell Cycle. (2014) 13:1571–82. doi: 10.4161/cc.28494
168. Osório L, Wu X, Wang L, Jiang Z, Neideck C, Sheng G, et al. ISM1 regulates NODAL signaling and asymmetric organ morphogenesis during development. J Cell Biol. (2019) 218:2388–402. doi: 10.1083/jcb.201801081
169. Berrun A, Harris E, Stachura DL. Isthmin 1 (ism1) is required for normal hematopoiesis in developing zebrafish. PloS One. (2018) 13:e0196872. doi: 10.1371/journal.pone.0196872
170. Kitamura K, Kangawa K, Kawamoto M, Ichiki Y, Nakamura S, Matsuo H, et al. Adrenomedullin: a novel hypotensive peptide isolated from human pheochromocytoma. Biochem Biophys Res Commun. (1993) 192:553–60. doi: 10.1006/bbrc.1993.1451
171. Del Pup L, Belloni AS, Carraro G, De Angeli S, Parnigotto PP, Nussdorfer GG. Adrenomedullin is expressed in cord blood hematopoietic cells and stimulates their clonal growth. Int J Mol Med. (2003) 11:157–60. doi: 10.3892/ijmm.11.2.157
172. De Angeli S, Del Pup L, Febas E, Conconi MT, Tommasini M, Di Liddo R, et al. Adrenomedullin and endothelin-1 stimulate in vitro expansion of cord blood hematopoietic stem cells. Int J Mol Med. (2004) 14:1083–6. doi: 10.3892/ijmm.14.6.1083
173. Chute JP, Muramoto GG, Dressman HK, Wolfe G, Chao NJ, Lin S. Molecular profile and partial functional analysis of novel endothelial cell-derived growth factors that regulate hematopoiesis. Stem Cells. (2006) 24:1315–27. doi: 10.1634/stemcells.2005-0029
174. Li H, Zacharaki D, Ghazanfari R, Ekblom M, Méndez-Ferrer S, Scheding S. Primary Linneg/CD45neg/CD271pos/PDGFRαlow/Neg stroma stem cells in adult human bone marrow show a distinct molecular signature and potent hematopoiesis-supporting function. Blood. (2013) 122:3699. doi: 10.1182/blood.V122.21.3699.3699
175. Nishikimi T. Adrenomedullin in the kidney-renal physiological and pathophysiological roles. Curr Medicinal Chem. (2007) 14:1689–99. doi: 10.2174/092986707780830943
176. Tomoda Y, Kikumoto K, Isumi Y, Katafuchi T, Tanaka A, Kangawa K, et al. Cardiac fibroblasts are major production and target cells of adrenomedullin in the heart in vitro. Cardiovasc Res. (2001) 49:721–30. doi: 10.1016/S0008-6363(00)00291-1
177. Martinez A, Miller MJ, Unsworth EJ, Siegfried JM, Cuttitta F. Expression of adrenomedullin in normal human lung and in pulmonary tumors. Endocrinology. (1995) 136:4099–105. doi: 10.1210/endo.136.9.7649118
178. Sugo S, Minamino N, Kangawa K, Miyamoto K, Kitamura K, Sakata J, et al. Endothelial cells actively synthesize and secrete adrenomedullin. Biochem Biophys Res Commun. (1994) 201:1160–6. doi: 10.1006/bbrc.1994.1827
179. Lin LFH, Doherty DH, Lile JD, Bektesh S, Collins F. GDNF: a glial cell line-derived neurotrophic factor for midbrain dopaminergic neurons. Science. (1993) 260:1130–2. doi: 10.1126/science.8493557
180. Grey W, Chauhan R, Piganeau M, Huerga Encabo H, Garcia-Albornoz M, McDonald NQ, et al. Activation of the receptor tyrosine kinase RET improves long-term hematopoietic stem cell outgrowth and potency. Blood. (2020) 136:2535–47. doi: 10.1182/blood.2020006302
181. Guo ZY, Sun X, Xu XL, Zhao Q, Peng J, Wang Y. Human umbilical cord mesenchymal stem cells promote peripheral nerve repair via paracrine mechanisms. Neural Regener Res. (2015) 10:651–8. doi: 10.4103/1673-5374.155442
182. Fonseca-Pereira D, Arroz-Madeira S, Rodrigues-Campos M, Barbosa IA, Domingues RG, Bento T, et al. The neurotrophic factor receptor RET drives haematopoietic stem cell survival and function. Nature. (2014) 514:98–101. doi: 10.1038/nature13498
183. Veiga-Fernandes H, Coles MC, Foster KE, Patel A, Williams A, Natarajan D, et al. Tyrosine kinase receptor RET is a key regulator of peyer’s patch organogenesis. Nature. (2007) 446:547–51. doi: 10.1038/nature05597
184. Cintron-Colon AF, Almeida-Alves G, Boynton AM, Spitsbergen JM. GDNF synthesis, signaling, and retrograde transport in motor neurons. Cell Tissue Res. (2020) 382:47–56. doi: 10.1007/s00441-020-03287-6
185. Xiao N, Lin Y, Cao H, Sirjani D, Giaccia AJ, Koong AC, et al. Neurotrophic factor GDNF promotes survival of salivary stem cells. J Clin Invest. (2014) 124:3364–77. doi: 10.1172/JCI74096
186. Zhong Z, Gu H, Peng J, Wang W, Johnstone BH, March KL, et al. GDNF secreted from adipose- derived stem cells stimulates VEGF-independent angiogenesis. Oncotarget. (2016) 7:36829–41. doi: 10.18632/oncotarget.9208
187. Kobayashi M, Wei H, Yamanashi T, Azevedo Portilho N, Cornelius S, Valiente N, et al. HSC- independent definitive hematopoiesis persists into adult life. Cell Rep. (2023) 42:112239. doi: 10.1016/j.celrep.2023.112239
188. Crisan M, Yap S, Casteilla L, Chen CW, Corselli M, Park TS, et al. A perivascular origin for mesenchymal stem cells in multiple human organs. Cell Stem Cell. (2008) 3:301–13. doi: 10.1016/j.stem.2008.07.003
189. Tavazoie M, van der Veken L, Silva-Vargas V, Louissaint M, Colonna L, Zaidi B, et al. A specialized vascular niche for adult neural stem cells. Cell Stem Cell. (2008) 3:279–88.
190. Xiao Y, Woo WM, Nagao K, Li W, Terunuma A, Ys M, et al. Perivascular hair follicle stem cells associate with a venule annulus. J Invest Dermatol. (2013) 133:2324–31. doi: 10.1038/jid.2013.167
191. Agarwala S, Kim KY, Phan S, Ju S, Kong YE, Castillon GA, et al. Defining the ultrastructure of the hematopoietic stem cell niche by correlative light and electron microscopy. eLife. (2022) 11:e64835. doi: 10.7554/elife.64835
192. Li W, Johnson SA, Shelley WC, Yoder MC. Hematopoietic stem cell repopulating ability can be maintained in vitro by some primary endothelial cells. Exp Hematol. (2004) 32:1226–37. doi: 10.1016/j.exphem.2004.09.001
193. Rodriguez-Fraticelli AE, Wolock SL, Weinreb CS, Panero R, Patel SH, Jankovic M, et al. Clonal analysis of lineage fate in native haematopoiesis. Nature. (2018) 553:212–6. doi: 10.1038/nature25168
Keywords: hematopoiesis, stem cell, embryonic development, hematopoietic progenitor cells, extramedullary hematopoiesis
Citation: Rivera-Torruco G, Muench MO and Valle-Rios R (2024) Exploring extramedullary hematopoiesis: unraveling the hematopoietic microenvironments. Front. Hematol. 3:1371823. doi: 10.3389/frhem.2024.1371823
Received: 17 January 2024; Accepted: 02 May 2024;
Published: 28 May 2024.
Edited by:
Emanuele Azzoni, University of Milano Bicocca, ItalyReviewed by:
Perpetua Pinto-do-Ó, Universidade do Porto, PortugalLakshmi Sandhow, INSERM U1016 Institut Cochin, France
Copyright © 2024 Rivera-Torruco, Muench and Valle-Rios. This is an open-access article distributed under the terms of the Creative Commons Attribution License (CC BY). The use, distribution or reproduction in other forums is permitted, provided the original author(s) and the copyright owner(s) are credited and that the original publication in this journal is cited, in accordance with accepted academic practice. No use, distribution or reproduction is permitted which does not comply with these terms.
*Correspondence: Guadalupe Rivera-Torruco, Z3RvcnJ1Y29Adml0YWxhbnQub3Jn