- Sanofi, Rare Blood Disorders, Rare and Neurological Diseases, Cambridge, MA, United States
Efmoroctocog alfa, a recombinant factor VIII Fc fusion protein referred to herein as rFVIIIFc, is an extended half-life factor replacement therapy approved for use in patients with hemophilia A. Previous studies have shown that rFVIIIFc has an immunoregulatory effect on monocyte-derived macrophages. This study provides novel findings and an understanding of how rFVIIIFc modulates monocyte differentiation into osteoclasts. rFVIIIFc was found to engage with Fc-gamma receptors (FcγR) on the monocyte surface, leading to increased inhibitory FcγR signaling in cells. Monocyte differentiation into osteoclasts in vitro was inhibited in a concentration-dependent manner following rFVIIIFc treatment, with the interaction between the Fc domain of rFVIIIFc and FcγRII on monocytes playing a role in this effect. The C1 and C2 domains of rFVIIIFc were also found to play a role in inhibiting osteoclast formation. rFVIIIFc treatment of monocytes skewed their differentiation from osteoclasts into a group of less differentiated monocytes with unique myeloid cell phenotypes. The results of this study suggest that rFVIIIFc has a unique immune-regulatory effect on monocyte differentiation, inhibiting osteoclast formation. We propose a “double touchpoint” model for rFVIIIFc interaction with monocytes, with both the Fc domain and domains of FVIII binding to the monocyte surface. Further study is needed to determine if this immune-regulatory effect has any potential benefit on the bone and joint health of patients with hemophilia A receiving rFVIIIFc.
Introduction
Hemophilia A is characterized by deficiency of factor VIII (FVIII) activity. Patients with hemophilia A, particularly those with severe disease (<1 IU/dL FVIII), experience bleed-related symptoms, including spontaneous bleeds into joints and soft tissues, and excessive bleeds with trauma or surgery (1). Prophylactic FVIII replacement therapy is the standard of care in hemophilia A, with the goal of preventing bleeds and preserving musculoskeletal function and joint health (1).
Efmoroctocog alfa is a recombinant FVIII Fc fusion protein (referred to herein as rFVIIIFc; Eloctate®, Sanofi, Waltham, MA/Elocta®, Sobi, Stockholm, Sweden) was the first extended half-life factor approved for individuals of all ages with hemophilia A in all clinical scenarios (2), demonstrating long-term efficacy and safety and improvement in joint health (3–5). rFVIIIFc consists of recombinant FVIII fused to the Fc portion of immunoglobulin (Ig)G1, allowing for half-life extension by taking advantage of the natural Fc recycling pathway mediated by neonatal Fc receptor (FcRn) in endothelial endosomes (6, 7). Preclinical studies suggest the Fc domain of rFVIIIFc has immunomodulatory effects (8–10). These include inducing alternative macrophage polarization of monocyte-derived macrophages in vitro (8), and inducing regulatory T-cell activation by Treg epitopes in the Fc domain to attenuate immunogenicity of rFVIIIFc and inflammation in vivo (9).
Hemophilic arthropathy is a debilitating complication in hemophilia, with approximately 80% of patients exhibiting joint damage (11). It is caused by spontaneous and repeated bleeds into the joints leading to chronic inflammation in the joint cavity, synovial pannus growth, destruction of cartilage, and subchondral bone erosion (11). Studies have described a high prevalence of decreased bone mineral density (BMD) and osteoporosis in patients with hemophilia (12–19) and in carriers of hemophilia (20). Decreased BMD is also associated with hemophilic arthropathy (16, 21–23). Therefore, there is a need to improve joint health and minimize BMD loss over time in those with hemophilia A, aside from controlling bleeds.
Bone mass in humans is actively maintained by bone remodeling, which is a balance between bone formation by osteoblasts and bone resorption by osteoclasts; disrupted balance contributes to pathogenesis of various bone disorders (24, 25). Osteoclasts are giant multi-nucleated cells that differentiate from mononuclear cells of monocyte/macrophage lineage, have bone-resorbing activity, and exclusively reside in the bone remodeling compartment (25–27). Osteoclastogenesis requires 2 essential factors, macrophage colony-stimulating factor (M-CSF) and receptor activator of nuclear factor κβ ligand (RANKL), which are sufficient to generate functional monocyte-derived osteoclasts in vitro (28–30).
Our previous study showed that rFVIIIFc, but not recombinant FVIII (rFVIII), skewed a type of monocyte-derived macrophage to a regulatory phenotype, dependent on rFVIIIFc-Fc receptor interactions (8). In this study, we aimed to determine whether rFVIIIFc has a regulatory effect on monocyte differentiation into osteoclasts in vitro.
Materials and methods
Recombinant proteins and biologics
FVIII proteins were produced and purified in-house as described previously (7), including B-domain deleted rFVIII, B-domain deleted rFVIIIFc, and rFVIIIFc-N297A. Non-targeting human IgG1 (hIgG1) control was from BioXcell (Lebanon, NH). M-CSF and RANKL (Peprotech, Rocky Hill, NJ) were used for culture and differentiation of monocytes into osteoclasts. Blocking Fc-gamma receptors (FcγR) (31–36) was achieved with the following: anti-cluster of differentiation (CD)16 antibodies (clone 3G8) and isotype control from Biolegend (San Diego, CA); anti-CD32 antibodies (clone IV3) and isotype control from BioXcell (Lebanon, NH); antigen-binding fragment (Fab) of anti-CD64 antibody (10.1 clone) and isotype Fab control from Ancell (Bayport, MN). Blocking of various domains on FVIII proteins was achieved with purified domain specific anti-FVIII monoclonal antibodies (GMA8004, 8017, 8010, 8020, 8014), from Green Mountain Antibodies (Burlington, VT).
Cell culture and treatment of cells
CD14+ blood monocytes were isolated from peripheral blood mononuclear cells of healthy donors and then purified using positive selection kits from Miltenyi Biotec (Auburn, CA) or StemCell Technologies (Cambridge, MA), per manufacturers’ protocols. Purified CD14+ monocytes were collected in RPMI1640 supplemented with GlutaMAX, 1% penicillin/streptomycin, and 10% fetal bovine serum (FBS) (Thermo Fisher Scientific, Waltham, MA). For evaluation of monocyte-FVIII interaction and signaling, purified monocytes were incubated with various proteins as indicated for each experiment at 37°C in a cell culture incubator, and cells were lifted for flow cytometry analysis of cell surface markers or for cell lysate collection. For monocyte differentiation into osteoclasts, 2.5×106 or 5×106 purified CD14+ monocytes were plated in 24- or 12-well plates, treated with various proteins as indicated for each experiment, and then were cultured with 25 ng/mL M-CSF and 100 ng/mL RANKL for 7 days. Fresh media and growth factors were provided after 3 days. After 7 days cells were subjected to various analyses, including staining for tartrate-resistant acid phosphatase (TRAP) activity, RNA extraction, or cell dissociation for flow cytometry analysis. To block the FcγRs on the monocyte surface before cell differentiation, monocytes were first incubated with the appropriate FcγR antibodies or controls for 30 minutes, followed by addition of indicated treatments. To block FVIII domains on rFVIIIFc, each respective anti-FVIII GMA antibody was incubated with rFVIIIFc at 1:1 ratio at 250 nM or with vehicle as the control for 10 minutes at room temperature, and the mixtures were then applied to cells with a final concentration of 25 nM rFVIIIFc.
Cell-based assays and analysis
Intracellular signaling in monocytes was evaluated in cell lysates using enzyme-linked immunosorbent assays (ELISA) for p-Syk, (Cell Signalling Technology Danvers, MA), p-SHP1 and p-SHP2 (RayBiotech life, Peachtree Corners, GA) per manufacturers’ protocols. TRAP activity after 7 days of culture was evaluated using the Acid Phosphatase, Leukocyte (TRAP) Kit (Sigma, St Louis, MO) per the manufacturer’s protocol but without hematoxylin staining. Images were taken using a Nikon Eclipse microscope at 10x magnification. To assess osteoclast bone resorption activity, monocytes were cultured on bone slices (Nordic Bioscience, Denmark), with a modified culture and differentiation method to enhance cell survival. Purified monocytes were cultured in a 12-well plate with their respective treatments for 1 day. Cells were then lifted and transferred to bone slices in a 96-well plate at 2×106 cells for a further 7-day culture. The cells were then washed away, and bone slices were stained with toluidine blue (Sigma, St Louis, MO) to visualize lacunae. For gene expression evaluation, total RNA was isolated from differentiated cells using the RNeasy Mini Kit (Qiagen, Germantown, MD) and reverse transcribed into cDNA using the SuperScript VILO Kit (Thermo Fisher Scientific, Waltham, MA). Gene expression was assessed using Taqman Gene Expression Master Mix (Thermo Fisher Scientific, Waltham, MA) and the predesigned Taqman gene expression assays for human receptor activator of nuclear factor kappa-B (RANK), nuclear factor of activated T cells (NFATC1), cathepsin K (CATK), TRAP, matrix metalloproteinase 9 (MMP9) with an endogenous control gene (36B4). Data were analyzed by delta Ct method on an Applied Biosystems 7500 Fast DX instrument per manufacturer’s instructions (Thermo Fisher Scientific, Waltham, MA).
For flow cytometry analysis of cell surface markers, differentiated cells were collected using non-enzymatic tissue dissociation buffer (Sigma, St Louis, MO). Cells were stained in a phosphate-buffered saline containing 2% FBS, 1 mM ethylenediaminetetraacetic acid, and 0.1% sodium azide, for monocyte/osteoclast analysis with an anti-CD14, anti-CD51/61, anti-CD32, and anti-CD64 antibody cocktail (Biolegend), or for analysis of broad myeloid phenotype with a cocktail containing anti-CD14, anti-CD16, anti-CD51/61, anti-CD32, anti-CD64, anti-CD163, anti-CD33, anti-CD35, anti-CD44, anti-CD11b, and anti-CD172a/b (Biolegend), along with LIVE/DEAD Fixable Violet Dead Cell Stain (Thermo Fisher Scientific). Flow cytometry data were acquired on a BD Canto or LSRII flow cytometer (BD Biosciences, Franklin Lake, NJ) and data were analyzed using FlowJo software (TreeStar, Ashland, OR) including plots and dimension reduction and t-distributed stochastic neighbor embedding (tSNE) analysis.
Statistical analysis
Statistical analyses were performed using paired t-test between two groups in Prism 8 (GraphPad, San Diego, CA). Data are presented as mean ± standard error of the mean (SEM). Statistics were considered significant if P values were <0.05.
Results
rFVIIIFc uniquely engages FcγRs on the CD14+ monocyte cell surface and leads to a change in intracellular signaling of FcγRs
Since rFVIIIFc, but not rFVIII, drives alternative activation of monocyte-derived macrophages through Fc-FcR interaction (8), we examined whether rFVIIIFc can directly bind monocytes by detecting the presence of cell membrane-bound rFVIIIFc. To this end, CD14+ monocytes purified from healthy donor blood were incubated with rFVIIIFc or rFVIII+hIgG1 and then an anti-human Fc antibody or anti-FVIII antibody (GMA8017) was used to detect human Fc domain or FVIII, respectively, on the monocyte cell surface (Figures 1A–D). The membrane-bound Fc domain was detected abundantly on monocytes incubated with rFVIIIFc after 15 minutes through to 2 hours compared with cells incubated with rFVIII+hIgG1 or vehicle (Figure 1A). After 15 minutes incubation, 3.3 (100.5) nM and higher concentrations of rFVIIIFc resulted in a significantly (P<0.05) greater proportion of cells with membrane-bound Fc domain compared with cells incubated with rFVIII+hIgG1 (Figure 1B). Membrane-bound FVIII could be detected on monocytes incubated with rFVIII or rFVIIIFc (Figure 1C). A slightly greater but variable proportion of the cells incubated with rFVIIIFc were found to be FVIII-bound than those incubated with rFVIII+hIgG1 (Figure 1D). These results indicate that both rFVIIIFc and rFVIII bind to the monocyte cell surface, but rFVIIIFc binds more efficiently than rFVIII.
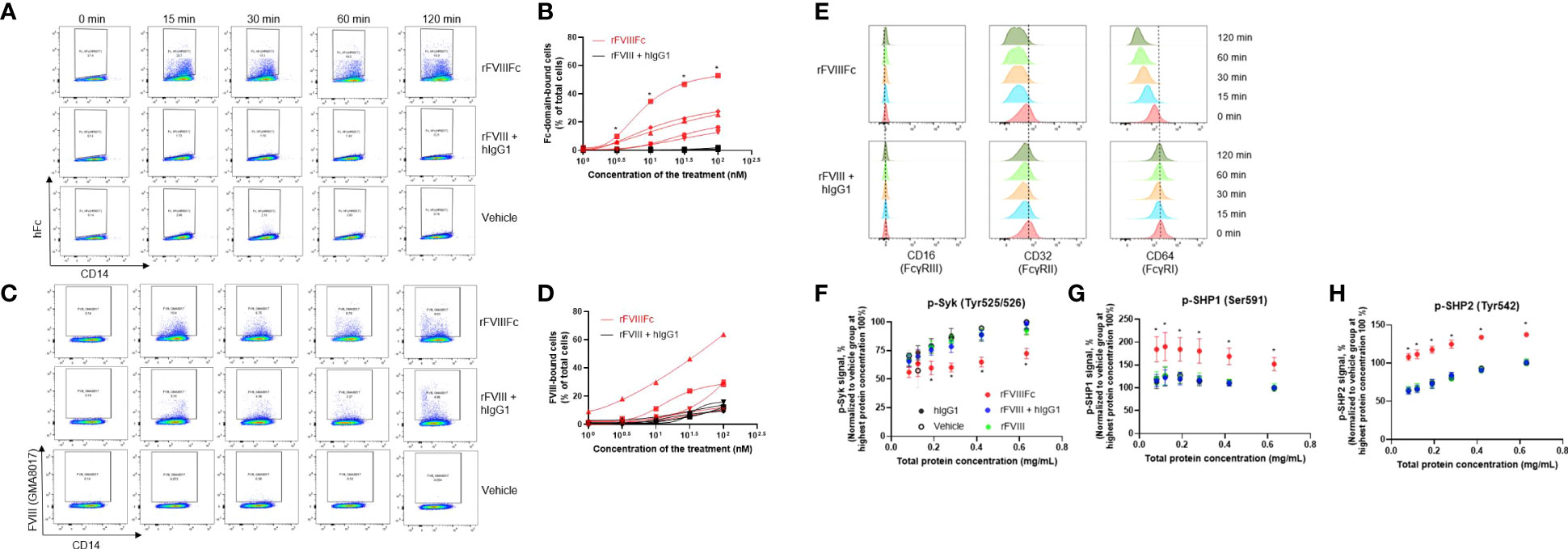
Figure 1 rFVIIIFc incubation with purified CD14+ blood monocytes leads to binding to cell surface, engagement of FcγRs, and changes in intracellular signaling. Representative flow cytometry plots showing membrane-bound Fc on monocyte cell surface detected by an anti-Fc antibody (A), or membrane-bound FVIII on monocyte cell surface detected by an anti-FVIII antibody (C), after incubation with 50 nM rFVIIIFc, rFVIII + hIgG1, or vehicle control for indicated time. Proportion of Fc-domain–bound cells (B) and FVIII-bound cells (D) following incubations with varying concentrations of rFVIIIFc or FVIII + hIgG1 for 15 minutes. Each line represents the results from an individual donor, n=5. Statistical testing performed using the paired t-test between the 2 groups at each concentration: *P < 0.05. (E) Representative flow cytometry plots showing the detection of each FcγR on the monocyte cell surface after incubation with 50 nM rFVIIIFc or rFVIII + hIgG for indicated time. Relative levels of phosphorylated Syk (F), SHP1 (G), and SHP2 (H) detected by the respective ELISAs in the serial diluted cell lysate. Cell lysates were collected from the monocytes following incubation with 50 nM of rFVIIIFc, rFVIII + hIgG1, rFVIII, hIgG1, or vehicle control for 15 minutes. Each cell lysate was adjusted to the same starting protein concentration and then was titrated down for a semi-quantitative ELISA. Data from each individual donor were normalized to the signal from the respective vehicle-incubated group at the starting concentration (100%). Data are presented as mean ± SEM, n=5. Statistical testing performed using a paired t-test between rFVIIIFc with any of the 4 groups at each concentrations: *P < 0.05.
Next, we assessed whether rFVIIIFc binding to monocytes could be linked with engagement of FcγR receptors on the monocyte cell surface. FcγRs comprises 3 types, FcγRI (CD64), FcγRII (CD32), and FcγRIII (CD16), and are known to have different expression levels on various monocyte subsets (37, 38). In this study, nearly all isolated CD14+ monocytes showed high FcγRII and FcγRI expression and low FcγRIII expression (Figure 1E), a classical monocyte phenotype. Three antibodies targeting each of the FcγRs known to compete with Fc binding sites on FcγRs (31–36) were used to assess FcγR engagement by rFVIIIFc. After monocyte incubation with rFVIIIFc or rFVIII+hIgG1, levels of FcγRIII did not change, but levels of both FcγRII and FcγRI gradually decreased, which could be attributed to the bound rFVIIIFc hindering detection of the receptors or subsequent internalization of the bound receptors. These results indicate that FcγRII and FcγRI were uniquely engaged by the Fc domain of rFVIIIFc, not by that of monomeric hIgG, suggesting additional mechanisms are involved in rFVIIIFc engaging the FcγRs.
As Fc-FcγR engagement could lead to FcγR signaling (37), we next examined the phosphorylation of Syk, an activatory signaling pathway downstream of FcγRI and FcγRII, in CD14+ monocytes after incubation with rFVIIIFc or rFVIII+hIgG1. Surprisingly, only rFVIIIFc caused a significant (P<0.05) reduction in p-Syk levels (Figure 1F). This prompted us to examine the inhibitory signaling pathway mediated by SHP1 or SHP2 downstream of FcγRII in CD14+ monocytes (37). Only rFVIIIFc caused a significant (P<0.05) increase in the levels of phosphorylated SHP1 and SHP2 in the monocytes (Figures 1G, H). Phosphorylated SHP1 and SHP2 are activated phosphatases that could dephosphorylate active p-Syk, potentially explaining the reduced level of p-Syk by rFVIIIFc, suggesting net inhibitory signaling was induced by rFVIIIFc.
rFVIIIFc treatment of blood monocytes inhibits the formation of osteoclasts after in vitro differentiation
As blood monocytes are precursor cells of osteoclasts, we next sought to evaluate whether rFVIIIFc could alter monocyte differentiation into osteoclasts in vitro. Therefore, CD14+ monocytes were treated with either vehicle, hIgG1, rFVIII, or rFVIIIFc, and then cultured for 7 days with M-CSF and RANKL for formation of monocyte-derived osteoclasts. In the vehicle control group, 2 morphologically distinct cell types were observed: large multinucleated cells and small mononuclear cells, which are consistent with osteoclast morphology and the morphology of M-CSF–differentiated macrophages as intermediate precursors of osteoclasts, respectively (Figure 2A). The cells derived from monocytes exposed to hIgG1 or rFVIII were similar to the vehicle control, displaying both morphologies after differentiation. However, the cells derived from rFVIIIFc-treated monocytes showed a homogenous round cell morphology, distinct from either the giant osteoclast or typical macrophage morphology (Figure 2A).
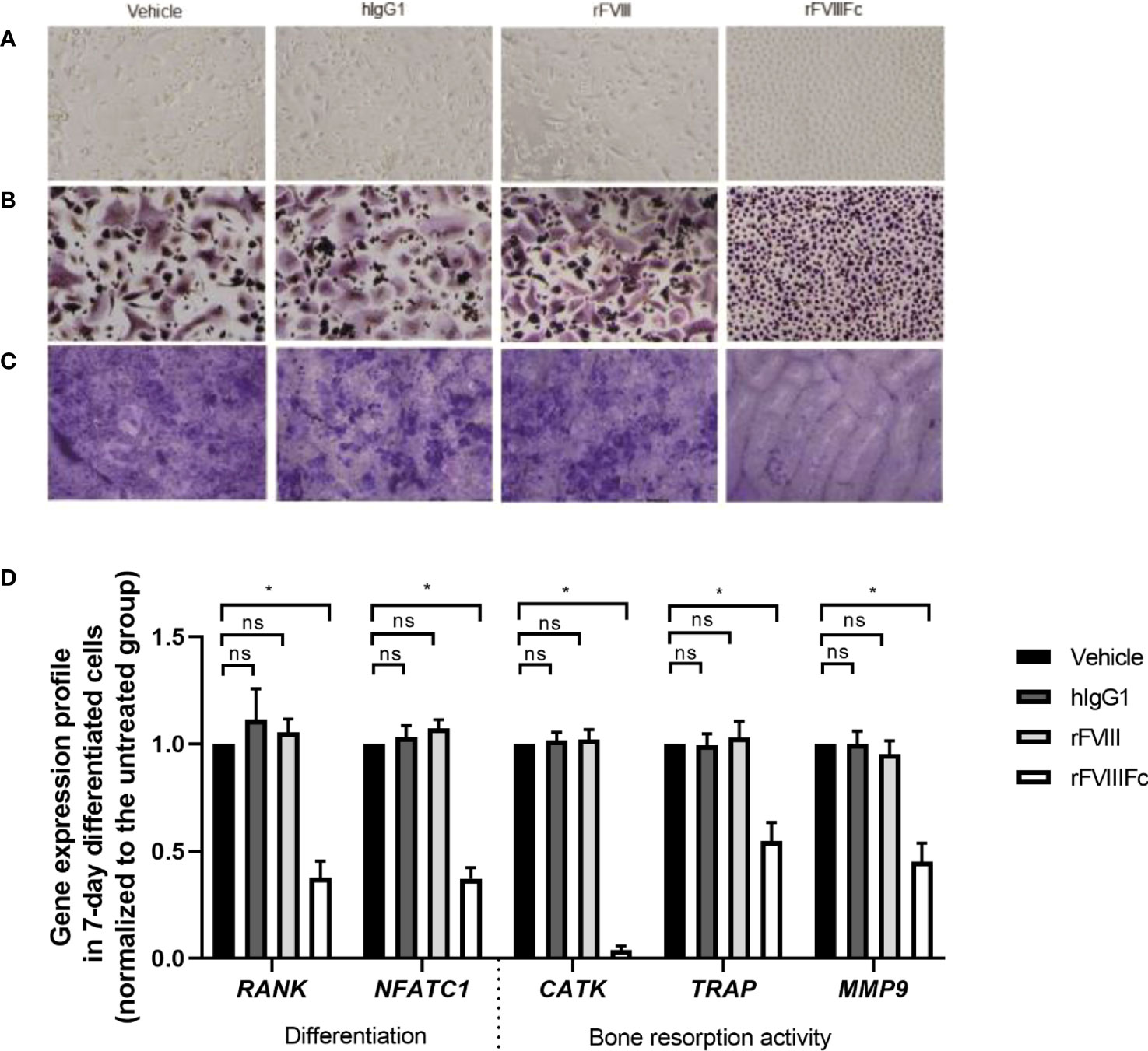
Figure 2 rFVIIIFc treatment of CD14+ blood monocytes inhibits the formation of monocyte-derived osteoclasts in vitro. Representative images of (A) bright field and (B) TRAP activity staining of monocyte-derived osteoclasts treated with vehicle, hIgG1, rFVIII, or rFVIIIFc at 25 nM and cultured for 7 days with M-CSF and RANKL. (C) Representative bone slices stained with toluidine blue after treated monocytes were cultured on top over 7 days for osteoclast differentiation. All images are taken at 10x magnification. (D) Relative levels of gene expression in the differentiated monocytes. For each gene, expression level in each treatment group is normalized to its respective vehicle control cells as the percent relative gene expression. Data are represented as mean ± SEM, n=5. Statistical testing performed using paired t-test between the indicated groups: *P < 0.05; non-significant (ns) P>0.05.
To further distinguish the cell type derived from rFVIIIFc-treated monocytes, we examined TRAP activity staining, a marker for identifying osteoclasts and their precursors. The multinucleated cells derived from the vehicle-, hIgG1-, and rFVIII-treated cells were stained with a diffuse granular pattern as typical TRAP+ osteoclast (Figure 2B); the remaining mononuclear cells were densely stained. The cells derived from rFVIIIFc-treated monocytes were uniformly and densely stained in a distinctive granular pattern and remained as mononuclear cells (Figure 2B), suggesting they were different from the mononuclear macrophages or multinucleated osteoclasts in the other 3 groups.
We sought to determine if these morphologically altered cells derived from rFVIIIFc-treated monocytes still possess bone resorption activity, osteoclasts’ hallmark activity, which can generate lacunae on a bone surface in vitro as a result of bone mineral mobilization and organic matrix degradation (29). Bone slices were co-cultured with cells during osteoclast differentiation. As expected, the differentiated cells derived from vehicle-, rFVIII-, and hIgG1-treated monocytes generated numerous lacunae on the bone surface (Figure 2C). However, the cells derived from rFVIIIFc-treated monocytes generated few lacunae, leaving large notably clean and smooth areas (Figure 2C).
To further characterize these cells, we examined the expression levels of genes associated with osteoclast differentiation (RANK and NFATC1) and bone resorption activity (CATK, TRAP, MMP9) (24, 29, 39). The levels of gene expression in cells derived from rFVIII- or hIgG1-treated monocytes were similar to vehicle-treated monocytes. However, the expression of all 5 genes in the cells derived from rFVIIIFc-treated monocytes were significantly (P<0.05) lower than those in the vehicle group (Figure 2D).
rFVIIIFc treatment of blood monocytes inhibits in vitro monocyte-derived osteoclast formation in a concentration-dependent manner
We next sought to evaluate the potency of rFVIIIFc inhibition of monocyte differentiation into osteoclasts. Monocyte- and osteoclast-related phenotypic markers CD14 and CD51/61 (40, 41) were assessed using flow cytometry to provide a quantitative measure of monocyte-derived osteoclast formation after 7 days of culture. In the vehicle group, there were 2 distinct populations of cells, a CD14+CD51/61− population of M-CSF–induced macrophages, and another characterized by CD14−CD51/61+ as osteoclasts (Figure 3A). Similar results were observed in cells exposed to rFVIII+hIgG1. However, the cells derived from rFVIIIFc-treated monocytes had a reduced number of CD14−CD51/61+ osteoclasts and a notable accumulation of CD14−CD51/61− cells in addition to the conventional CD14+CD51/61- macrophages (Figure 3A).
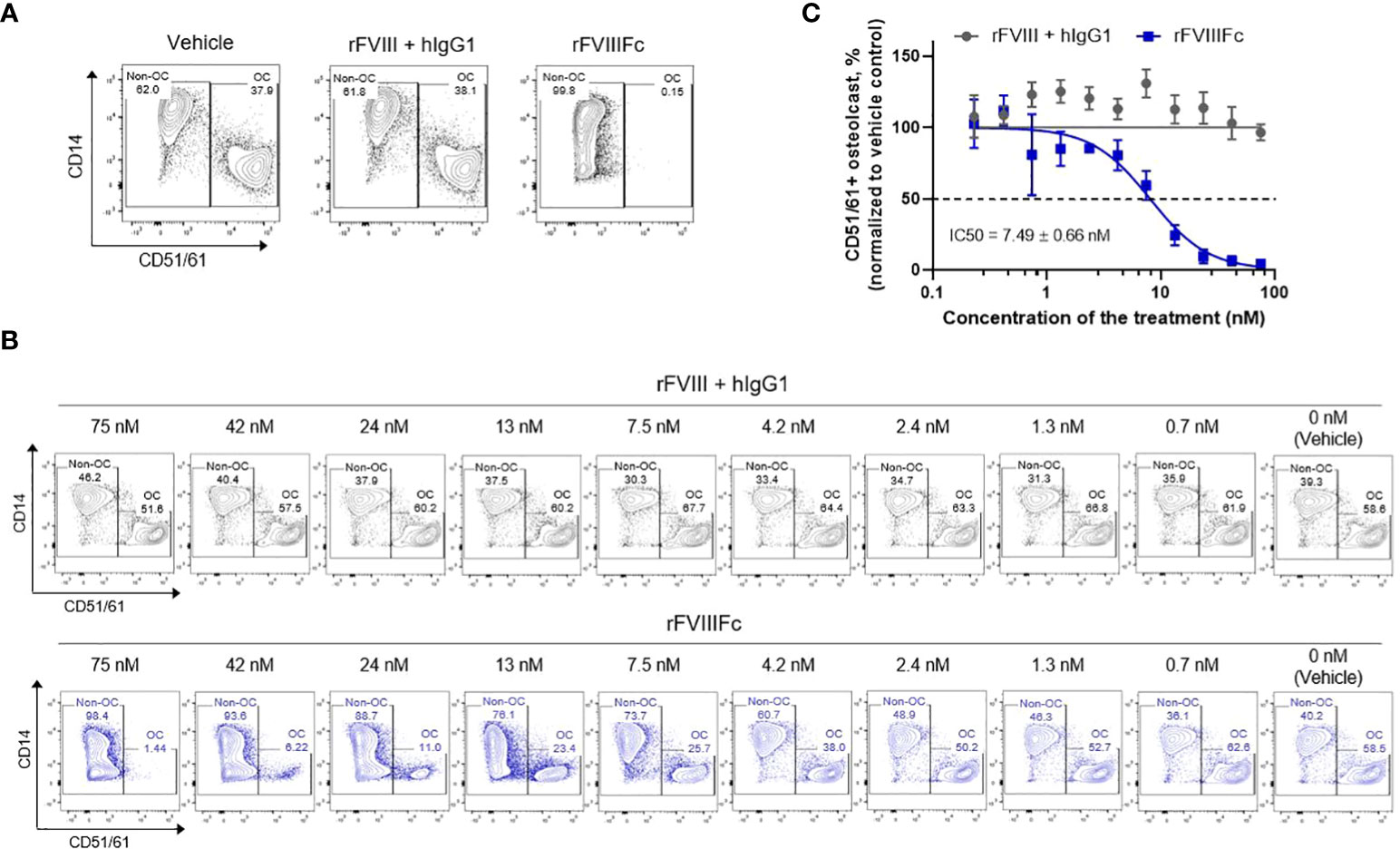
Figure 3 rFVIIIFc treatment of CD14+ blood monocytes inhibits in vitro monocyte-derived osteoclast formation in a dose-dependent manner. (A) Representative flow cytometry plots defining the frequency of osteoclast (CD14−CD51/61+, OC) and non-osteoclast (CD51/61−, Non-OC) cells derived from monocytes treated with vehicle, rFVIII + hIgG1, or rFVIIIFc at 25 nM. (B) Representative flow cytometry plots of osteoclast formation from monocytes treated with serial diluted concentrations of rFVIII + hIgG1 or rFVIIIFc as indicated. (C) Concentration response curves generated from these results. Osteoclast frequency at each dose of the treatment is normalized as a percent of osteoclasts in the respective vehicle control group (100%). The IC50 is represented as mean ± SEM from independent experiments, n=3.
Using this phenotypical analysis, we next measured the extent of CD14−CD51/61+ osteoclast formation, from monocytes treated with a series of concentrations of rFVIII+hIgG1 or rFVIIIFc. In comparison with vehicle treatment, various concentrations of rFVIII+hIgG1 did not change the frequency of osteoclast formation. However, the treatment of monocytes with rFVIIIFc reduced the frequency of osteoclast formation in a concentration-dependent manner (Figure 3B). The mean half maximal inhibitory concentration (IC50) of rFVIIIFc inhibiting the osteoclasts was 7.49 nM (Figure 3C).
rFVIIIFc inhibition of monocyte differentiation into osteoclasts depends on interactions between the Fc domain and FcγRs on the monocyte cell surface
As only rFVIIIFc, but not rFVIII, inhibited monocyte-derived osteoclast formation, we investigated the role of Fc domain interactions with FcγRs in mediating rFVIIIFc inhibitory effects. We utilized rFVIIIFc with an N297A mutation at the Fc domain, which significantly reduces Fc binding to all FcγRs (8, 9, 42). Cells derived from rFVIIIFc-N297A–treated monocytes had significantly higher expression levels of the 4 genes involved in osteoclast differentiation and activity compared with cells derived from rFVIIIFc-treated monocytes, to a level similar to cells derived from the vehicle-, hIgG1- or rFVIII-treated monocytes (Figure 4A). The frequency of CD14−CD51/61+ osteoclasts differentiated from rFVIIIFc-N297A–treated monocytes was approximately 3 times lower than rFVIIIFc-treated monocytes (Figure 4B), although the N297A mutation did not completely reverse the phenotype.
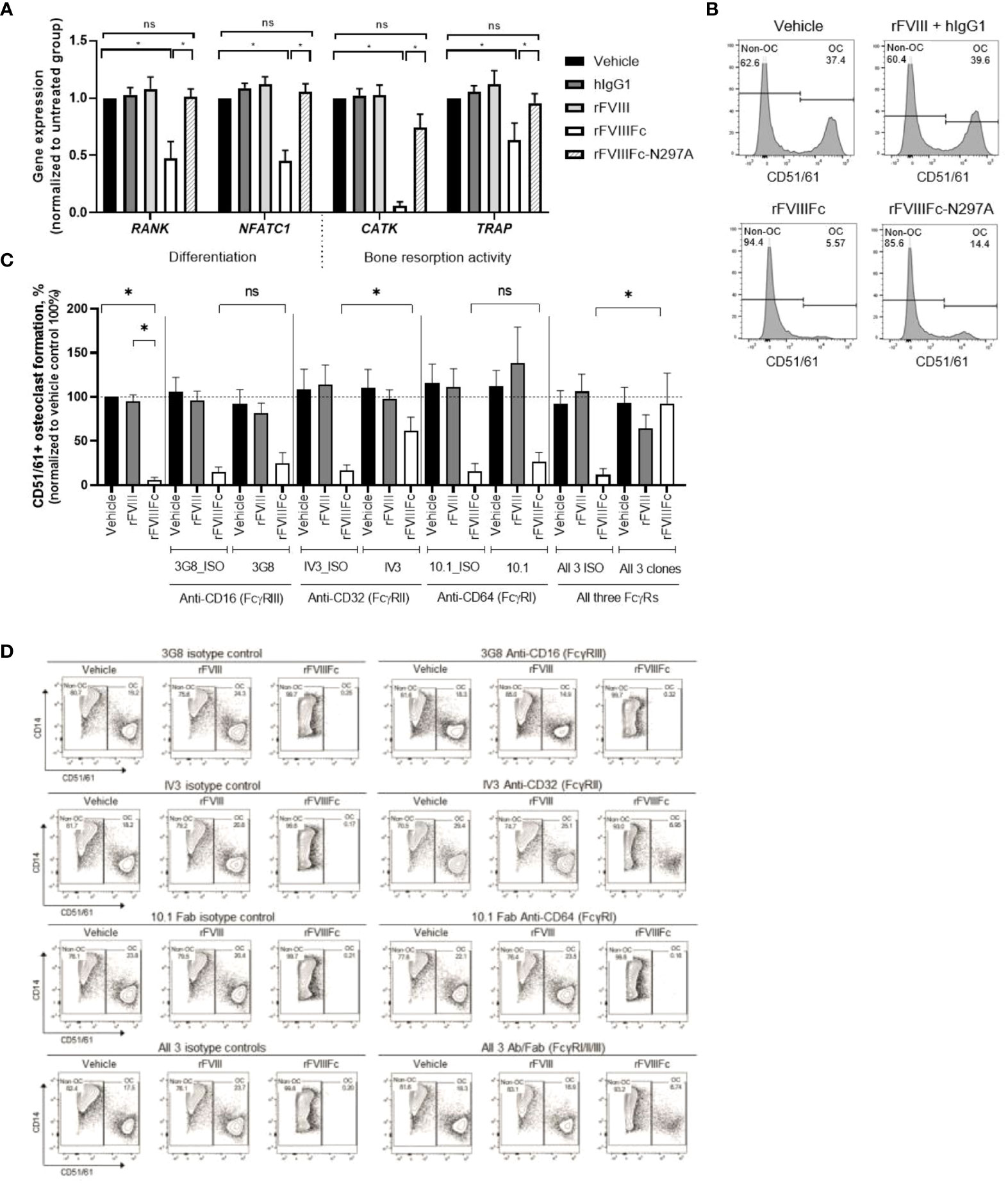
Figure 4 rFVIIIFc inhibition of monocyte differentiation into osteoclasts depends on interactions between the Fc domain and FcγR on the monocyte cell surface. (A) Relative levels of gene expression in differentiated cells from monocytes treated with vehicle, rFVIII + hIgG1, rFVIIIFc, or rFVIIIFc-N297A. For each gene, the expression level in each treatment group is normalized to respective vehicle control cells as percent relative gene expression. Data are represented as mean ± SEM, n=5. Statistical testing performed with paired t-test between indicated groups: *P<0.05. (B) Representative flow cytometry plots of osteoclast frequency derived from the monocytes treated with vehicle, rFVIII + hIgG1, rFVIIIFc, or rFVIIIFc-N297A (25 nM). (C) Summary results of osteoclast frequency and (D) representative flow cytometry plots of differentiated cells from the monocytes with the indicated FcγRs blocked using monoclonal antibodies (67 nM, right panels) or without blocking using isotype controls (67 nM, left panels) after vehicle, rFVIII, or rFVIIIFc treatment (25 nM). Osteoclast frequency in the treatment groups is normalized as a percent of osteoclasts in the respective vehicle control group (100%). Data are represented as mean ± SEM, n=6. Statistical testing performed using the paired t-test between indicated groups: *P < 0.05. Ab, antibody; CD51/61, cluster of differentiation 51/61 (integrin alpha V/integrin beta-3). ns, non-significant.
We next sought to identify which of the 3 types of FcγRs mediate interaction with the Fc domain of rFVIIIFc and account for the inhibition of osteoclast formation. Therefore, before monocytes were treated with rFVIII or rFVIIIFc, each FcγR was blocked using their respective monoclonal antibody (31–35) or a matched isotype antibody as control. Following rFVIIIFc treatment, osteoclast frequency derived from monocytes with a blocked FcγRII was significantly (P<0.05) higher than from monocytes without a blocked FcγRII (Figures 4C, D). In contrast, osteoclast frequency from monocytes with blocked FcγRI or FcγRIII remained at a low level, similar to those cells without a blocked FcγR (Figures 4C, D). In addition, blocking all 3 receptors simultaneously led to a significant (P<0.05) increase in osteoclast frequency compared with cells without blocked receptors, which was slightly but not significantly higher than when FcγRII alone was blocked. These results suggest that the interaction of rFVIIIFc with FcγRII predominantly accounts for its inhibitory effects on osteoclast formation. rFVIIIFc interacting with FcγRI showed no significant contribution to rFVIIIFc inhibitory effects, consistent with its low or absent expression level on CD14+ monocytes (Figure 1E). Although both FcγRI and FcγRII on CD14+ monocytes were engaged by rFVIIIFc (Figure 1E), the significant contribution of FcγRII, but not the FcγRI, in mediating rFVIIIFc’s inhibition of osteoclast formation may be explained by their different downstream signaling pathways (37).
rFVIIIFc inhibition of monocyte differentiation into osteoclasts depends on additional domains of FVIII
We also investigated whether the FVIII portion of rFVIIIFc plays a role in inhibiting monocyte-derived osteoclast formation. The FVIII portion comprises 5 domains (A1, A2, A3, C1, C2), which are structurally well organized and have different orientation (43). In rFVIIIFc, the C-terminal Fc domain is flexibly tethered to the C2 domain as an extended orientation with high mobility (44). Fc and FVIII domains exhibit structural and functional independence (7, 44), suggesting that each domain could be perturbed relatively independently. To this end, we blocked each domain of the FVIII portion of rFVIIIFc using domain-specific FVIII-binding monoclonal antibodies before they were added into monocyte culture, and then assessed monocyte differentiation into osteoclasts. The murine IgG2a or IgG2b subclass of these anti-FVIII antibodies has substantially reduced or abolished binding to human FcγRs (45), which suggests they are unlikely to bind and signal through human FcγRs in monomeric form, although it is a caveat to be noted. Blocking of the A1 (GMA8004), A2 (GMA8017), and A3 (GMA8010) domains of rFVIIIFc did not significantly change the inhibitory effect of rFVIIIFc on monocyte differentiation (Figures 5A, B). Blocking of the C1 (GMA8020) or C2 domain (GMA8014) of rFVIIIFc resulted in 4–5 times higher (P<0.05) osteoclast frequency than from cells treated with rFVIIIFc alone, although it did not fully restore the osteoclast formation to that observed in vehicle group (Figures 5A, B). Overall, these results suggest that C1 or C2 domain of rFVIIIFc partially but significantly contributes to the inhibitory activity of rFVIIIFc on osteoclast formation.
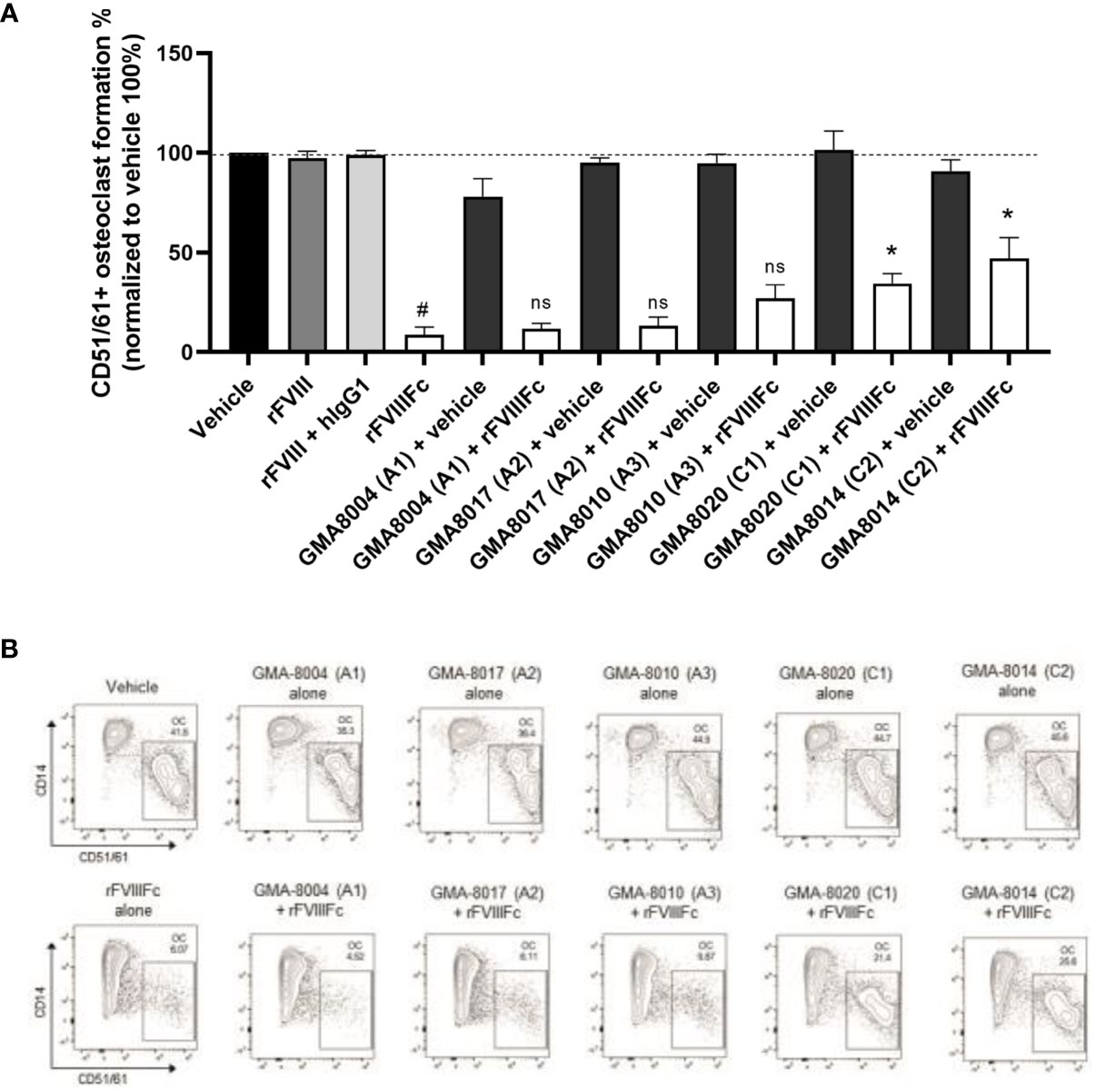
Figure 5 rFVIIIFc inhibition of monocyte differentiation into osteoclasts depends on additional domains of FVIII. (A) Summary results and (B) representative flow cytometry plots and of osteoclast frequency (OC) from monocytes treated with vehicle, rFVIII, rFVIII + hIgG1, or rFVIIIFc, or with rFVIIIFc being bound to a domain specific anti-FVIII antibody as indicated (25 nM), or each individual anti-FVIII antibody. The clone number of the antibodies and target FVIII domain is indicated within parentheses. Osteoclast frequency in the treatment groups was normalized as a percent of osteoclasts in the respective vehicle control group (100%). Data are represented as mean ± SEM, n=7. Statistical testing performed using paired t-test between rFVIIIFc group and indicated group: *P < 0.05, ns, P>0.05; and paired t-test between vehicle and rFVIIIFc group: # P < 0.05.
rFVIIIFc treatment inhibits monocyte-derived osteoclasts and induces a unique pattern of the immune phenotype markers on the cells
We noted that the CD51/61− cells derived from rFVIIIFc-treated monocytes comprise a CD14+ subset (macrophage-like), and a unique CD14− subset (non–macrophage-like), not observed in the other treatment groups (Figure 6A). To further understand this CD14− subset, we examined several myeloid surface markers. The CD14+ macrophage-like cells derived from rFVIIIFc-treated monocytes largely resembled the CD14+ macrophages derived from rFVIII-treated monocytes (Figure 6B). However, the non–macrophage-like CD14- cells had a different expression pattern (Figure 6B), compared with the CD14+ macrophages.
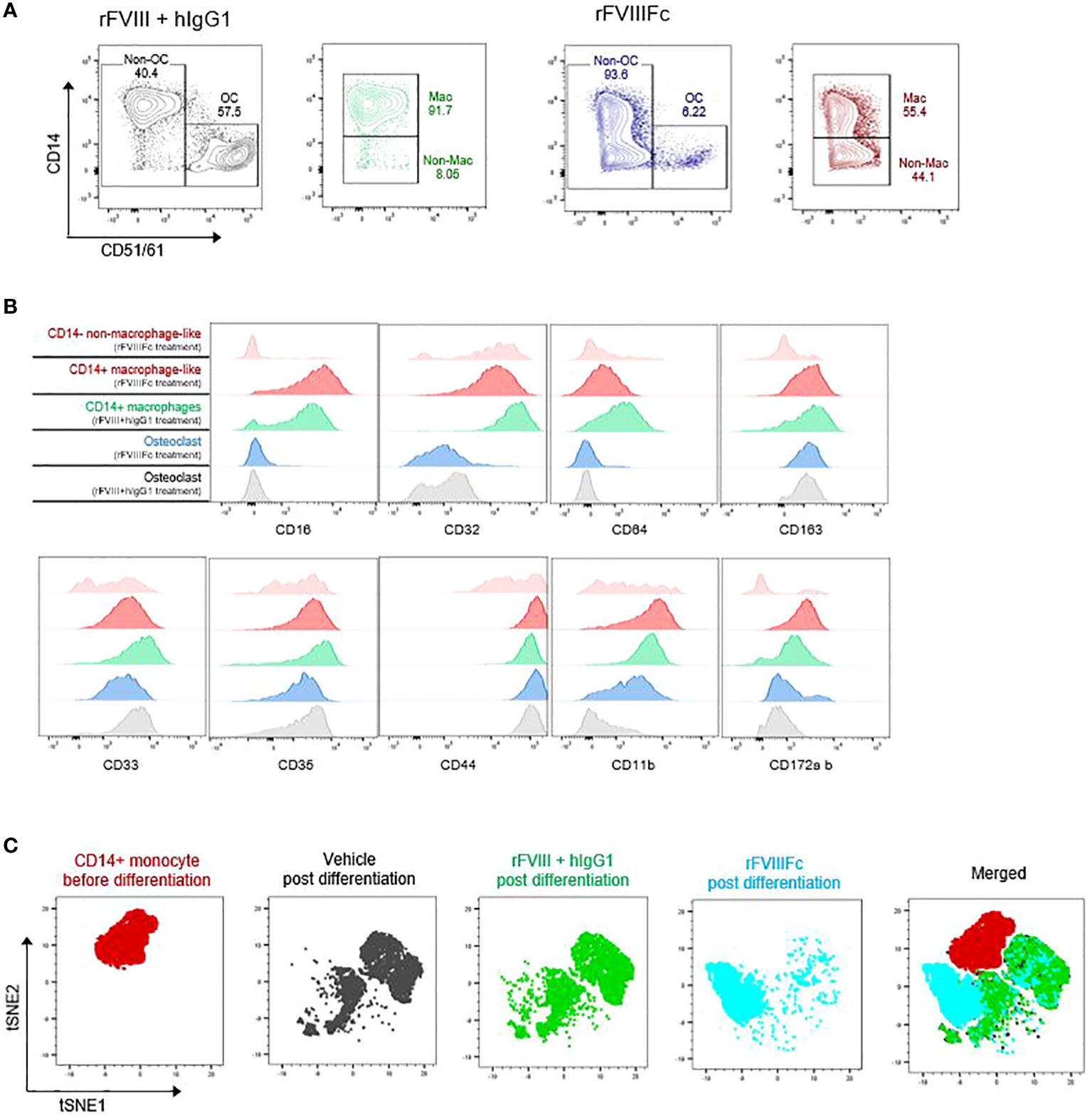
Figure 6 rFVIIIFc induces a unique pattern of immune phenotypical markers on the monocyte after differentiation. (A) Representative flow cytometry plots of osteoclast formation from monocytes treated with rFVIII + hIgG1 or rFVIIIFc (25 nM). Non-osteoclast (Non-OC) cells are further broken down into macrophage-like (Mac) and non–macrophage-like (Non–mac) subsets based on CD14 expression level. (B) Surface expression levels of other monocyte/macrophage markers from each macrophage-like and non–macrophage-like subset in the cells derived from each treatment group. (C) tSNE plots of purified blood monocytes and the differentiated cells derived from the monocytes with each treatment.
To further understand how the cells differentiated from each group differ phenotypically, we clustered the cells based on the expression level of 11 phenotypic markers using tSNE dimension reduction (Figure 6C). The cells derived from rFVIII+hIgG1-treated monocytes closely overlapped with those from vehicle-treated monocytes, with 2 large clusters corresponding to osteoclasts and remaining macrophages. In addition, the cells derived from rFVIIIFc-treated monocytes did not substantially overlap with the cells derived from vehicle- or rFVIII+hIgG1-treated monocytes and appear to be a separate cluster. These results indicate that rFVIIIFc treatment of monocytes inhibits their differentiation into macrophages and osteoclasts, leading to a unique type of differentiated monocytes.
Discussion
The findings presented in this study reveal the unique immune-regulatory effect of rFVIIIFc on monocyte differentiation in vitro. We observed that the Fc, C1, and C2 domains of rFVIIIFc simultaneously interact with the monocyte surface and subsequently induce FcγR inhibitory signaling pathways. Monocytes exposed to rFVIIIFc lose their differentiation potential into osteoclasts, and the cells derived from rFVIIIFc-exposed monocytes are characterized by a lack of typical osteoclast gene expression, functional activity, phenotypic markers, and morphology. Therefore, we propose a “double touchpoint” model for rFVIIIFc interaction with monocytes, skewing to their differentiation from osteoclast to a unique myeloid cell phenotype (Figure 7).
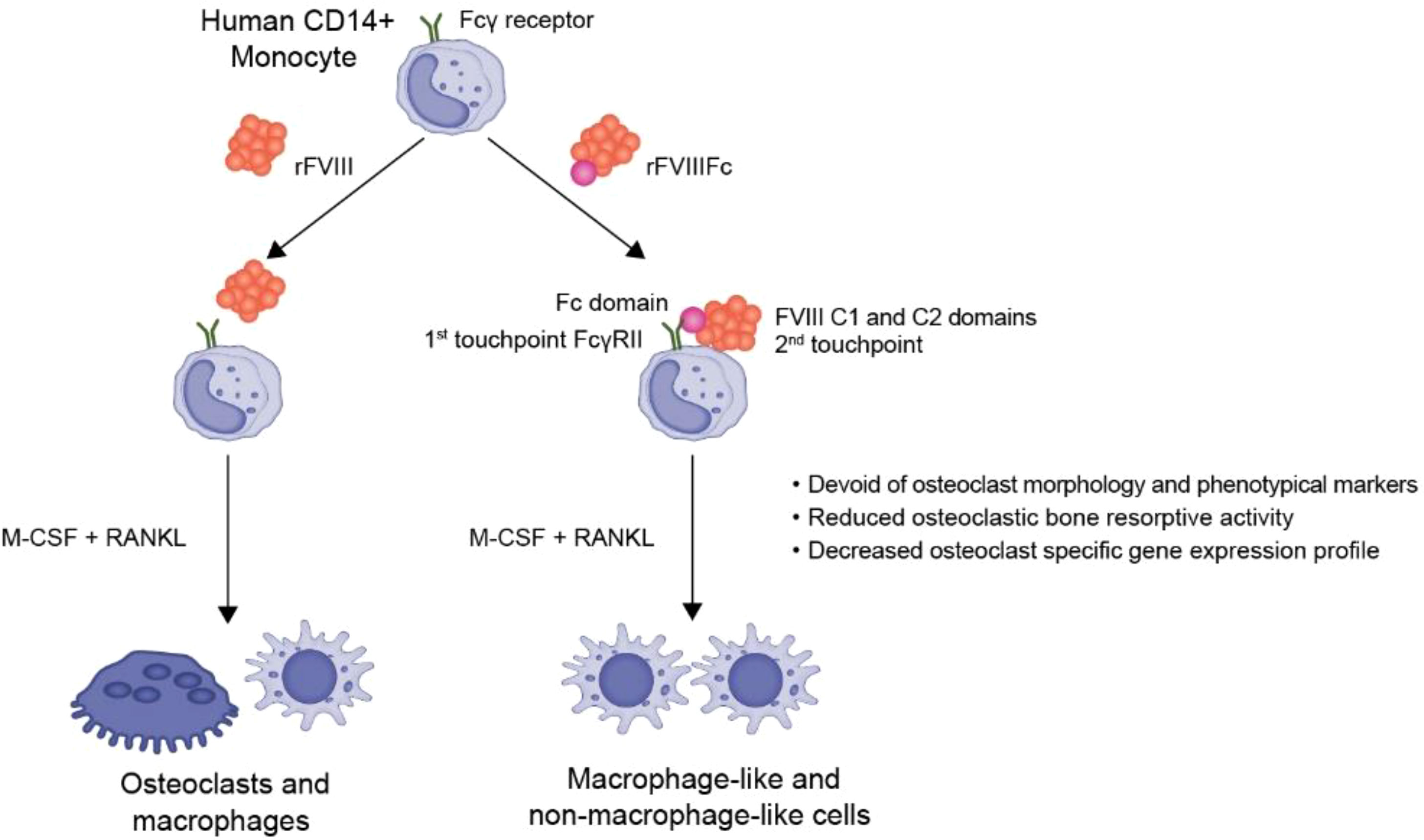
Figure 7 Summary of the engagement of rFVIIIFc with monocyte surface, leading to immunoregulatory effects on monocyte differentiation. rFVIIIFc interacts with monocyte cell surface via the Fc, C1, and C2 domains in a double touchpoint model. This interaction leads to increasing inhibitory signaling in monocytes and subsequent inhibition of osteoclast formation.
In this study, we observed that rFVIIIFc engages with FcγRI and FcγRII on purified CD14+ monocytes but induces a net inhibitory signaling with reduced level of p-Syk. Both FcγRI and FcγRIIA bear an intracellular immunoreceptor tyrosine-based activation motif (ITAM) to mediate activatory signaling through increasing phosphorylation of p-Syk. Only FcγRIIB bears an intracellular immunoreceptor tyrosine-based inhibition motif (ITIM) which mediates inhibitory signaling by phosphorylation of SHP1 or SHP2, in turn leading to deactivation of p-Syk. The reduced p-Syk and increased p-SHP1 and p-SHP2 levels in rFVIIIFc-treated cells indicates that concomitant inhibitory signaling overwhelms activatory signaling. In addition, although both are engaged by rFVIIIFc on the monocyte surface, blocking FcγRII but not FcγRI on monocytes negated the rFVIIIFc inhibitory effects on osteoclast formation, suggesting that FcγRII signaling plays a predominant role. A baseline level of Syk signaling in circulating blood monocytes, likely activated through high-affinity receptor FcγRI by high concentrations of IgGs in human blood circulation (46), might explain why FcγRI engagement does not lead to activation. Thus, the additional engagement of FcγRI by rFVIIIFc on monocytes might not further enhance the existing Syk signaling, allowing FcγRIIB-mediated inhibitory signaling to be predominant.
Our previous study has shown that rFVIIIFc treatment of in vitro cultured monocyte-derived macrophages in the absence of cytokines and growth factors leads to increased levels of Syk phosphorylation, suggesting activatory signaling events as a net outcome of FcγR signaling in the macrophage (8). A recent study showed that rFVIIIFc activates FcγRIIA activatory signaling in monocyte-derived dendritic cells (MoDc). Both of these studies used in vitro cultured monocyte-derived cells, which may have lost the basal levels of FcγR signaling observed in circulating monocytes isolated from blood and, thus, might be sensitive to additional new FcγRI engagement by rFVIIIFc leading to a net outcome of higher levels of activatory signaling, although both activatory and inhibitory signaling might have been stimulated. In addition, the in vitro activation of MoDCs by rFVIIIFc may have limited physiological relevance because MoDCs reside in various tissues (47, 48) and therefore have little chance to be directly exposed to rFVIII or rFVIIIFc in the circulation. Here, we propose that FcγRIIB activation and signaling in undifferentiated, circulating blood monocytes by rFVIIIFc engagement may change the course of monocyte differentiation into osteoclasts.
It is worth noting that FcγRII is a low-affinity Fc receptor with a binding affinity to monovalent Fc at Kd level around 106 M-1 (37, 49). In this study, we determined an IC50 of rFVIIIFc on monocyte differentiation of approximately 7.5 nM, suggesting that monovalent rFVIIIFc could engage FcγRII at a concentration lower than its Kd level. Our study proposes a double touchpoint model with rFVIIIFc interacting with monocytes using the Fc domain and C1 and C2 domains of FVIII for increased binding avidity, which can explain how monovalent rFVIIIFc could engage low-affinity FcγRII. Further studies are needed to identify additional binding partners and their potential signaling roles. A recent study has shown that rFVIIIFc simultaneously binds FcγRIIB and the B cell receptor (BCR) on FVIII-specific B cells, inducing inhibitory signaling through its ITIM motif, demonstrated by downstream SHP phosphorylation and attenuated calcium flux (50). In this case, rFVIIIFc binding to B cells occurs in a similar double touchpoint setting where the FVIII portion binds to the FVIII-specific BCR and Fc engages FcγRIIB simultaneously, thus partly supporting the model we propose for rFVIIIFc interaction with monocytes.
The finding that rFVIIIFc possesses unique activity of inhibiting monocyte-derived osteoclast formation may be relevant to hemophilic arthropathy and overall bone health in patients with hemophilia A. There is limited evidence that endogenous FVIII has an intrinsic role in the regulation of bone mass (51), and thus deficiency of FVIII may directly contribute to lower BMD in patients with hemophilia A. Furthermore, several recent models of needle puncture injury in FVIII-deficient mice provided direct evidence that intra-articular bleeding promotes bone resorption, with bleeding into the joints resulting in synovial inflammation, increased osteoclast activity, and bone loss adjacent to the affected joints (52, 53). In patients receiving rFVIIIFc prophylaxis, rFVIIIFc has the potential to interact with monocytes in the circulation and might reduce the number of monocytes that could differentiate into osteoclasts in the inflammatory joint environment and in the bone remodeling compartment, thus potentially reducing excessive bone resorption.
Conclusion
Here, we have shown that rFVIIIFc interacts with blood monocytes through a double touchpoint model and reduces monocyte differentiation into osteoclast in vitro, thus having the potential to shift the balance between bone resorption and bone remodeling to reduce osteoclastogenesis. Therefore, rFVIIIFc may possess immunoregulatory benefits on affected joints and bone density homeostasis in patients with hemophilia. Data from further studies are needed to determine the dose and effects of rFVIIIFc on monocyte differentiation into osteoclasts, long-term joint health, and overall bone health in the context of patients with hemophilia A.
Data availability statement
The original contributions presented in the study are included in the article. Further inquiries can be directed to the corresponding author.
Author contributions
SD, YD, GR, and KK-T designed aspects of the study, collected, analyzed, and/or interpreted data. JS designed aspects of the study and interpreted data. All authors contributed to the article and approved the submitted version.
Acknowledgments
Medical writing was provided by Sarah Rupprechter, PhD, and Ashleigh Pulkoski-Gross, PhD, CMPP, of Fishawack Ltd., part of Fishawack Health, and this support was funded by Sanofi.
Conflict of interest
This study received funding from Sanofi Cambridge, MA, USA. The funder had the following involvement with the study: Sanofi sponsored the research described in this manuscript and provided funding for medical writing. Sanofi employees were involved in the study design; collection, analysis, and interpretation of data; development of the manuscript; and in the decision to submit the manuscript for publication. SD and YD are current employees of Sanofi and may hold shares and/or stock options in the company. GR, KK-T, and JS were employees of Sanofi and may have held shares and/or stock options in the company at the time of the study. SD, KK-T, GR, and JS are associated with a related patent application.
Publisher’s note
All claims expressed in this article are solely those of the authors and do not necessarily represent those of their affiliated organizations, or those of the publisher, the editors and the reviewers. Any product that may be evaluated in this article, or claim that may be made by its manufacturer, is not guaranteed or endorsed by the publisher.
References
1. Srivastava A, Santagostino E, Dougall A, Kitchen S, Sutherland M, Pipe SW, et al. Wfh guidelines for the management of hemophilia, 3rd edition. Haemophilia (2020) 26 Suppl 6:1–158. doi: 10.1111/hae.14046
2. FDA. Eloctate package insert (2021). FDA. Available at: https://www.fda.gov/media/88746/download (Accessed October 18, 2021).
3. Nolan B, Mahlangu J, Pabinger I, Young G, Konkle BA, Barnes C, et al. Recombinant factor viii fc fusion protein for the treatment of severe haemophilia a: Final results from the aspire extension study. Haemophilia (2020) 26(3):494–502. doi: 10.1111/hae.13953
4. Oldenburg J, Kulkarni R, Srivastava A, Mahlangu JN, Blanchette VS, Tsao E, et al. Improved joint health in subjects with severe haemophilia a treated prophylactically with recombinant factor viii fc fusion protein. Haemophilia (2018) 24(1):77–84. doi: 10.1111/hae.13353
5. Oldenburg J, Pasi J, Pabinger I, Nolan B, Kulkarni R, Blanchette VS, et al. Improvements in joint health during long-term use of recombinant factor viii fc fusion protein prophylaxis in subjects with haemophilia a. In: 12th annual congress of the European association for haemophilia and allied disorders. Prague, Czech Republic (2019) Haemophilia, 25 S1: 114.
6. Dumont JA, Liu T, Low SC, Zhang X, Kamphaus G, Sakorafas P, et al. Prolonged activity of a recombinant factor viii-fc fusion protein in hemophilia a mice and dogs. Blood (2012) 119(13):3024–30. doi: 10.1182/blood-2011-08-367813
7. Peters RT, Toby G, Lu Q, Liu T, Kulman JD, Low SC, et al. Biochemical and functional characterization of a recombinant monomeric factor viii-fc fusion protein. J. Thromb. Haemost. (2013) 11(1):132–41. doi: 10.1111/jth.12076
8. Kis-Toth K, Rajani GM, Simpson A, Henry KL, Dumont J, Peters RT, et al. Recombinant factor viii fc fusion protein drives regulatory macrophage polarization. Blood Adv. (2018) 2(21):2904–16. doi: 10.1182/bloodadvances.2018024497
9. Krishnamoorthy S, Liu T, Drager D, Patarroyo-White S, Chhabra ES, Peters R, et al. Recombinant factor viii fc (Rfviiifc) fusion protein reduces immunogenicity and induces tolerance in hemophilia a mice. Cell Immunol. (2016) 301:30–9. doi: 10.1016/j.cellimm.2015.12.008
10. Blumberg RS, Lillicrap D. Tolerogenic properties of the fc portion of igg and its relevance to the treatment and management of hemophilia. Blood (2018) 131(20):2205–14. doi: 10.1182/blood-2017-12-822908
11. Melchiorre D, Manetti M, Matucci-Cerinic M. Pathophysiology of hemophilic arthropathy. J. Clin. Med. (2017) 6(7):63. doi: 10.3390/jcm6070063
12. Ghosh K, Shetty S. Bone health in persons with haemophilia: A review. Eur. J. Haematol (2012) 89(2):95–102. doi: 10.1111/j.1600-0609.2012.01803.x
13. Cadé M, Muñoz-Garcia J, Babuty A, Fouassier M, Heymann MF, Monahan PE, et al. Fviii at the crossroad of coagulation, bone and immune biology: Emerging evidence of biological activities beyond hemostasis. Drug Discovery Today (2021) 27(1):102–16. doi: 10.1016/j.drudis.2021.07.015
14. Dagli M, Kutlucan A, Abusoglu S, Basturk A, Sozen M, Kutlucan L, et al. Evaluation of bone mineral density (Bmd) and indicators of bone turnover in patients with hemophilia. Bosn J. Basic Med. Sci. (2018) 18(2):206–10. doi: 10.17305/bjbms.2018.2335
15. Barnes C, Wong P, Egan B, Speller T, Cameron F, Jones G, et al. Reduced bone density among children with severe hemophilia. Pediatrics (2004) 114(2):e177–81. doi: 10.1542/peds.114.2.e177
16. Gerstner G, Damiano ML, Tom A, Worman C, Schultz W, Recht M, et al. Prevalence and risk factors associated with decreased bone mineral density in patients with haemophilia. Haemophilia (2009) 15(2):559–65. doi: 10.1111/j.1365-2516.2008.01963.x
17. Paschou SA, Anagnostis P, Karras S, Annweiler C, Vakalopoulou S, Garipidou V, et al. Bone mineral density in men and children with haemophilia a and b: A systematic review and meta-analysis. Osteoporos Int. (2014) 25(10):2399–407. doi: 10.1007/s00198-014-2773-7
18. Nair AP, Jijina F, Ghosh K, Madkaikar M, Shrikhande M, Nema M. Osteoporosis in young haemophiliacs from Western India. Am. J. Hematol. (2007) 82(6):453–7. doi: 10.1002/ajh.20877
19. Gebetsberger J, Schirmer M, Wurzer WJ, Streif W. Low bone mineral density in hemophiliacs. Front. Med. (Lausanne) (2022) 9:794456. doi: 10.3389/fmed.2022.794456
20. Citla-Sridhar D, Sidonio RF Jr., Ahuja SP. Bone health in haemophilia carriers and persons with Von willebrand disease: A Large database analysis. Haemophilia (2022) 28(4):671–8. doi: 10.1111/hae.14565
21. Kempton CL, Antun A, Antoniucci DM, Carpenter W, Ribeiro M, Stein S, et al. Bone density in haemophilia: A single institutional cross-sectional study. Haemophilia (2014) 20(1):121–8. doi: 10.1111/hae.12240
22. Mansouritorghabeh H, Rezaieyazdi Z. Bone density status in bleeding disorders: Where are we and what needs to be done? J. Bone Metab. (2017) 24(4):201–6. doi: 10.11005/jbm.2017.24.4.201
23. Samuelson Bannow B, Recht M, Négrier C, Hermans C, Berntorp E, Eichler H, et al. Factor viii: Long-established role in haemophilia a and emerging evidence beyond haemostasis. Blood Rev. (2019) 35:43–50. doi: 10.1016/j.blre.2019.03.002
24. Delaisse JM, Andersen TL, Kristensen HB, Jensen PR, Andreasen CM, Søe K. Re-thinking the bone remodeling cycle mechanism and the origin of bone loss. Bone (2020) 141:115628. doi: 10.1016/j.bone.2020.115628
25. Kim JM, Lin C, Stavre Z, Greenblatt MB, Shim JH. Osteoblast-osteoclast communication and bone homeostasis. Cells (2020) 9(9):2073. doi: 10.3390/cells9092073
26. Udagawa N, Takahashi N, Akatsu T, Tanaka H, Sasaki T, Nishihara T, et al. Origin of osteoclasts: Mature monocytes and macrophages are capable of differentiating into osteoclasts under a suitable microenvironment prepared by bone marrow-derived stromal cells. Proc. Natl. Acad. Sci. U.S.A. (1990) 87(18):7260–4. doi: 10.1073/pnas.87.18.7260
27. Lorenzo J, Horowitz M, Choi Y. Osteoimmunology: Interactions of the bone and immune system. Endocr. Rev. (2008) 29(4):403–40. doi: 10.1210/er.2007-0038
28. Feng X, Teitelbaum SL. Osteoclasts: New insights. Bone Res. (2013) 1(1):11–26. doi: 10.4248/br201301003
29. Teitelbaum SL. Bone resorption by osteoclasts. Science (2000) 289(5484):1504–8. doi: 10.1126/science.289.5484.1504
30. Sørensen MG, Henriksen K, Schaller S, Henriksen DB, Nielsen FC, Dziegiel MH, et al. Characterization of osteoclasts derived from Cd14+ monocytes isolated from peripheral blood. J. Bone Miner Metab. (2007) 25(1):36–45. doi: 10.1007/s00774-006-0725-9
31. Ben Mkaddem S, Hayem G, Jönsson F, Rossato E, Boedec E, Boussetta T, et al. Shifting fcγriia-itam from activation to inhibitory configuration ameliorates arthritis. J. Clin. Invest. (2014) 124(9):3945–59. doi: 10.1172/jci74572
32. Holl V, Hemmerter S, Burrer R, Schmidt S, Bohbot A, Aubertin AM, et al. Involvement of fc gamma ri (Cd64) in the mechanism of hiv-1 inhibition by polyclonal igg purified from infected patients in cultured monocyte-derived macrophages. J. Immunol. (2004) 173(10):6274–83. doi: 10.4049/jimmunol.173.10.6274
33. Nagelkerke SQ, Dekkers G, Kustiawan I, van de Bovenkamp FS, Geissler J, Plomp R, et al. Inhibition of fcγr-mediated phagocytosis by ivig is independent of igg-fc sialylation and fcγriib in human macrophages. Blood (2014) 124(25):3709–18. doi: 10.1182/blood-2014-05-576835
34. Brown MG, King CA, Sherren C, Marshall JS, Anderson R. A dominant role for fcgammarii in antibody-enhanced dengue virus infection of human mast cells and associated Ccl5 release. J. Leukoc. Biol. (2006) 80(6):1242–50. doi: 10.1189/jlb.0805441
35. Tamm A, Schmidt RE. The binding epitopes of human Cd16 (Fc gamma riii) monoclonal antibodies. implications for ligand binding. J. Immunol. (1996) 157(4):1576–81.
36. Rankin CT, Veri MC, Gorlatov S, Tuaillon N, Burke S, Huang L, et al. Cd32b, the human inhibitory fc-gamma receptor iib, as a target for monoclonal antibody therapy of b-cell lymphoma. Blood (2006) 108(7):2384–91. doi: 10.1182/blood-2006-05-020602
37. Nimmerjahn F, Ravetch JV. Fcgamma receptors as regulators of immune responses. Nat. Rev. Immunol. (2008) 8(1):34–47. doi: 10.1038/nri2206
38. Rosales C. Fcγ receptor heterogeneity in leukocyte functional responses. Front. Immunol. (2017) 8:280. doi: 10.3389/fimmu.2017.00280
39. Takayanagi H, Kim S, Koga T, Nishina H, Isshiki M, Yoshida H, et al. Induction and activation of the transcription factor Nfatc1 (Nfat2) integrate rankl signaling in terminal differentiation of osteoclasts. Dev. Cell (2002) 3(6):889–901. doi: 10.1016/s1534-5807(02)00369-6
40. Maggiani F, Forsyth R, Hogendoorn PC, Krenacs T, Athanasou NA. The immunophenotype of osteoclasts and macrophage polykaryons. J. Clin. Pathol. (2011) 64(8):701–5. doi: 10.1136/jcp.2011.090852
41. Madel MB, Ibáñez L, Rouleau M, Wakkach A, Blin-Wakkach C. A novel reliable and efficient procedure for purification of mature osteoclasts allowing functional assays in mouse cells. Front. Immunol. (2018) 9:2567. doi: 10.3389/fimmu.2018.02567
42. Shields RL, Namenuk AK, Hong K, Meng YG, Rae J, Briggs J, et al. High resolution mapping of the binding site on human Igg1 for fc gamma ri, fc gamma rii, fc gamma riii, and fcrn and design of Igg1 variants with improved binding to the fc gamma r. J. Biol. Chem. (2001) 276(9):6591–604. doi: 10.1074/jbc.M009483200
43. Shen BW, Spiegel PC, Chang CH, Huh JW, Lee JS, Kim J, et al. The tertiary structure and domain organization of coagulation factor viii. Blood (2008) 111(3):1240–7. doi: 10.1182/blood-2007-08-109918
44. Leksa NC, Chiu PL, Bou-Assaf GM, Quan C, Liu Z, Goodman AB, et al. The structural basis for the functional comparability of factor viii and the long-acting variant recombinant factor viii fc fusion protein. J. Thromb. Haemost. (2017) 15(6):1167–79. doi: 10.1111/jth.13700
45. Temming AR, Bentlage AEH, de Taeye SW, Bosman GP, Lissenberg-Thunnissen SN, Derksen NIL, et al. Cross-reactivity of mouse igg subclasses to human fc gamma receptors: Antibody deglycosylation only eliminates Igg2b binding. Mol. Immunol. (2020) 127:79–86. doi: 10.1016/j.molimm.2020.08.015
46. van der Poel CE, Spaapen RM, van de Winkel JG, Leusen JH. Functional characteristics of the high affinity igg receptor, fcγri. J. Immunol. (2011) 186(5):2699–704. doi: 10.4049/jimmunol.1003526
47. Jakubzick CV, Randolph GJ, Henson PM. Monocyte differentiation and antigen-presenting functions. Nat. Rev. Immunol. (2017) 17(6):349–62. doi: 10.1038/nri.2017.28
48. Tang-Huau TL, Segura E. Human in vivo-differentiated monocyte-derived dendritic cells. Semin. Cell Dev. Biol. (2019) 86:44–9. doi: 10.1016/j.semcdb.2018.02.018
49. Bruhns P, Iannascoli B, England P, Mancardi DA, Fernandez N, Jorieux S, et al. Specificity and affinity of human fcgamma receptors and their polymorphic variants for human igg subclasses. Blood (2009) 113(16):3716–25. doi: 10.1182/blood-2008-09-179754
50. Georgescu MT, Moorehead PC, Liu T, Dumont J, Scott DW, Hough C, et al. Recombinant factor viii fc inhibits b cell activation Via engagement of the fcγriib receptor. Front. Immunol. (2020) 11:138. doi: 10.3389/fimmu.2020.00138
51. Weitzmann MN, Roser-Page S, Vikulina T, Weiss D, Hao L, Baldwin WH, et al. Reduced bone formation in males and increased bone resorption in females drive bone loss in hemophilia a mice. Blood Adv. (2019) 3(3):288–300. doi: 10.1182/bloodadvances.2018027557
52. Lau AG, Sun J, Hannah WB, Livingston EW, Heymann D, Bateman TA, et al. Joint bleeding in factor viii deficient mice causes an acute loss of trabecular bone and calcification of joint soft tissues which is prevented with aggressive factor replacement. Haemophilia (2014) 20(5):716–22. doi: 10.1111/hae.12399
Keywords: hemophilia, recombinant factor VIII Fc fusion protein, osteoclasts, monocyte differentiation, FC-gamma receptors
Citation: Duan S, Dang Y, Rajani GM, Kis-Toth K and Salas J (2022) Recombinant factor VIII Fc fusion protein engages monocytes via Fc and FVIII domains to reduce monocyte differentiation into osteoclasts. Front. Hematol. 1:1020852. doi: 10.3389/frhem.2022.1020852
Received: 16 August 2022; Accepted: 05 October 2022;
Published: 03 November 2022.
Edited by:
Michael Uhlin, Karolinska Institutet, SwedenReviewed by:
Seema Patel, Emory University, United StatesDaniel Lagasse, United States Food and Drug Administration, United States
Copyright © 2022 Duan, Dang, Rajani, Kis-Toth and Salas. This is an open-access article distributed under the terms of the Creative Commons Attribution License (CC BY). The use, distribution or reproduction in other forums is permitted, provided the original author(s) and the copyright owner(s) are credited and that the original publication in this journal is cited, in accordance with accepted academic practice. No use, distribution or reproduction is permitted which does not comply with these terms.
*Correspondence: Susu Duan, c3VzdS5kdWFuQHNhbm9maS5jb20=
†Employee at the time of the study. Currently affiliated with Ensoma
‡Employee at the time of the study. Currently affiliated with Jounce Therapeutics