- 1Sanders-Brown Center on Aging, University of Kentucky, Lexington, KY, United States
- 2Department of Neuroscience, University of Kentucky, Lexington, KY, United States
- 3Department of Physical Medicine and Rehabilitation, University of Kentucky, Lexington, KY, United States
- 4Genomics and Microbiome Core Facility, Rush University, Chicago, IL, United States
- 5Research Informatics Core, University of Illinois Chicago, Chicago, IL, United States
- 6Center for Advanced Stroke Science, Department of Neurology, University of Kentucky, Lexington, KY, United States
- 7Department of Pharmacology and Nutritional Sciences, University of Kentucky, Lexington, KY, United States
- 8Department of Computer Science, University of Kentucky, Lexington, KY, United States
- 9School of Medicine, University of Missouri, Columbia, MO, United States
- 10Spinal Cord and Brain Injury Research Center, University of Kentucky, Lexington, KY, United States
- 11Department of Statistics, University of Kentucky, Lexington, KY, United States
- 12Department of Radiology, University of Missouri, Columbia, MO, United States
- 13Institute for Data Science & Informatics, University of Missouri, Columbia, MO United States
- 14Department of Biological Sciences, University of Missouri, Columbia, MO, United States
Accumulating evidence suggests that gut microbes modulate brain plasticity via the bidirectional gut-brain axis and play a role in stroke rehabilitation. However, the microbial species alterations associated with stroke and their correlation with functional outcome measures following acute stroke remain unknown. Here we measure post-stroke gut dysbiosis and how it correlates with gut permeability and cognitive functions in 12 stroke participants, 18 controls with risk factors for stroke, and 12 controls without risk factors. Stool samples were used to measure the microbiome with whole genome shotgun sequencing and leaky gut markers. We genotyped APOE status and measured diet composition and motor, cognitive, and emotional status using NIH Toolbox. We used linear regression methods to identify gut microbial associations with cognitive and emotional assessments. We did not find significance differences between the two control groups. In contrast, the bacteria populations of the Stroke group were statistically dissimilar from the control groups. Relative abundance analysis revealed notable decreases in butyrate-producing microbial taxa, secondary bile acid-producing taxa, and equol-producing taxa. The Stroke group had higher levels of the leaky gut marker alpha-1-antitrypsin in the stool than either of the groups and several taxa including Roseburia species (a butyrate producer) were negatively correlated with alpha-1-antitrypsin. Stroke participants scored lower on memory testing than those in the two control groups. Stroke participants with more Roseburia performed better on the picture vocabulary task; more Bacteroides uniformis (a butyrate producer) and less Escherichia coli (a pro-inflammatory species) reported higher levels of self-efficacy. Intakes of fiber, fruit and vegetable were lower, but sweetened beverages were higher, in the Stroke group compared with controls. Vegetable consumption was correlated with many bacterial changes among the participants, but only the species Clostridium bolteae, a pro-inflammatory species, was significantly associated with stroke. Our findings indicate that stroke is associated with a higher abundance of proinflammatory species and a lower abundance of butyrate producers and secondary bile acid producers. These altered microbial communities are associated with poorer functional performances. Future studies targeting the gut microbiome should be developed to elucidate whether its manipulation could optimize rehabilitation and boost recovery.
Introduction
Over 795,000 people suffer a stroke every year in the United States alone (1). Recent advances in Stroke therapies have lowered stroke mortality, but survivors are often left severely impaired (2). Rehabilitation therapies such as physical therapy, occupational therapy, and speech therapy are beneficial for inducing neuroplasticity to overcome these impairments (3), but over 40% of stroke survivors are left with moderate to severe disabilities that markedly reduce quality of life (4). Novel multimodal approaches are needed to promote plasticity and restore sensorimotor function through a combination of current rehabilitation therapies with other treatments designed to foster neuroplasticity.
Accumulating evidence from animal studies suggests that gut microbes modulate brain plasticity via the bidirectional gut-brain axis and may play a role in functional recovery after stroke (5). A severely imbalanced microbial community, or dysbiosis, has been shown to occur following stroke, causing a systemic flood of neuro- and immunomodulatory substances due to increased gut permeability and decreased gut motility (6). These substances can impact neuroinflammation as commensal bacteria invade the bloodstream and as intestinal lymphocytes migrate from gut-associated lymphoid tissue to the brain (7). Fecal microbiota transplant has been shown to normalize brain lesion-induced dysbiosis and to improve stroke outcome in mice (7). The microbiome is modifiable as it is influenced by environmental factors such as diet and exercise and could potentially be an additional target in stroke rehabilitation through nutritional and pharmacological interventions (8, 9). Though it is unknown whether the findings from the bidirectional gut-brain axis in animals translate the same way into humans. Human studies thus far have suggested that gut dysbiosis occurs shortly following stroke at one time point and that this dysbiosis is associated with increased blood Apolipoprotein E (10) and IL-6 (11), decreased blood Trimethylamine-N-Oxide (12) and high-density lipoprotein (13), poor early functional outcomes (14), and 180-day mortality (15).
Currently, no human studies have been analyzed changes in the microbiome over the first three-week course of stroke rehabilitation and whether these changes correlate with gut permeability and subsequent recovery as measured by functional outcome measures. Furthermore, no human studies have included control groups with and without risk factors for stroke to delineate how the underlying risk factors contribute to microbiome differences. The goal of the study is to fill the gap by documenting the gut microbiome changes that occur following scute stroke in the first three weeks of rehabilitation and their associations with functional recovery measures. We also included two age-matched control groups with and without stroke risk factors, respectively, which allow us to inquire whether gut-brain axis changes can be attributed to the risk factors underlying stroke.
Materials and methods
Participants
All research activities were approved by the Institutional Review Board at the University of Kentucky. We recruited participants aged 55–85 for this study with consideration the population distribution of Kentucky. We recruited 12 patients in sub-stroke rehabilitation care after first time ischemic stroke Participants with clinically significant (unresolved, requiring on-going medical management or medication) pulmonary, gastrointestinal, dermatologic, hepatic, or renal functional abnormality were excluded. Individuals who had major gastrointestinal surgery within the past five years were also excluded.
Healthy participants (N = 30) were recruited through researchmatch.org and from advertisements posted by the Center for Clinical and Translational Science at the University of Kentucky. Among these participants, 18 did not have a history of stroke but have one or more common cardiovascular risk factors for stroke (Healthy group), and 12 did not have a history of stroke or common cardiovascular risk factors for stroke (At-Risk group) Participants followed the same inclusion/exclusion criteria listed above except for stroke. Table 1 describes the basic demographic characteristics of the participants. Those in the Stroke were slightly older than those in either control group. The Stroke and Healthy group had over 20% APOE ε2 carriers while the At-Risk group contained none. The participants in the Stroke were less educated than those in the Healthy and At-Risk groups. The Healthy group had a lower BMI than the Stroke group and the At-Risk group. The Healthy group by definition had no participants with diabetes, hypertension, or hyperlipidemia. In the stroke group, 66.7% of patients had a stroke in MCA territory, 8.3% in ACA territory, and 25.0% in subcortical territory.
Study design
Each individual received a verbal and written explanation of the purposes, procedures, and potential hazards of the study, and written consent was obtained. The University of California, San Diego Brief Assessment of Capacity to Consent (UBACC) was used to ensure decisional capacity then signed consent was obtained. Subjects were free to withdraw from the study at any time. This research had minimal risk. Following consent, study personnel collected the following information:
• Medical History: We obtained past medical history of the stroke patients from the electronic health record and questionnaires. These data were used solely for research purposes. Variables including age, gender, racial/ethnic background, education level, history of conventional vascular risk factors (hypertension, diabetes mellitus, atrial fibrillation, hyperlipoproteinemia, and smoking habit), and treatment during the acute phase were recorded and used as covariates for our analyses.
• Food Frequency: We assessed diet history using the Dietary Screener Questionnaire in the National Health and Nutrition Examination Survey for the dietary intake over the last month. (https://epi.grants.cancer.gov/nhanes/dietscreen/questionnaires.html). We included the results of the estimated intake of fiber, calcium, whole grains, sugar, dairy, fruits and vegetables, and sugar sweetened beverages.
• Oral Swab Sample: Oral swab was used to determine APOE genotype.
• Self-Care CARE Items: Section GG Self-Care Items are routinely used in the clinical care of inpatient stroke rehab patients to measure functional recovery.
• NIH Toolbox: Cognitive, Motor, Emotional, and Sensation measures from the NIH Toolbox were performed.
• Stool Samples: Stool samples were collected, genotyped, and analyzed using methods obtained from the International Human Microbiome Standards consortium (www.microbiome-standards.org) to determine gut microbial biodiversity.
The medical history, food frequency, NIH Toolbox measures, and stool samples were collected at admission and at a discharge visit for the stroke participants and at a three-month follow-up visit. For Healthy and At-Risk groups, these data were collected at admission and at a three-month follow-up visit. Due to the COVID-19 pandemic, many NIH Toolbox assessments were held via Zoom.
Stool sample collection and analysis
Stool samples were collected in Zymo DNA stabilization solution with sarstedt feces tubes from feces catcher placed on toilet seat. Genomic DNA was extracted from 0.25 grams of stool using ZymoBIOMICS™ DNA Mini Kit and shipped to the Genomics and Microbiome core facility at Rush University for DNA quantification using fluorometer Qubit 3.0. Libraries were constructed and the PCR products purified using 1.0X speed beads and eluted in 15 ul of nuclease-free water and quantified by PicoGreen fluorometric assay (100X final dilution). The libraries were pooled and loaded onto a high sensitivity chip run on the Caliper LabChipGX (Perkin Elmer, Waltham, MA) for size estimation and sequenced using Illumina NextSeq/HiSeq platform. Unassembled sequencing reads were analyzed by the Research Informatics Core at the University of Illinois Chicago for microbiome analysis. Alpha diversity, beta diversity, and relative abundance counts were calculated. For alpha diversity, raw counts were rarefied to 2000 k and the Shannon diversity index was calculated using the vegan R package. For beta diversity, the Bray-Curtis dissimilarity index was used and a Principal Component Analysis (PCA) plot was generated to visualize the diversity. We used MetaPhlAn (Metagenomic Phylogenetic Analysis) to profile the composition of microbial communities using unique clade-specific marker genes identified from ∼17,000 reference genomes (∼13,500 bacterial and archaeal, ∼3,500 viral, and ∼110 eukaryotic) (16).
APOE genotyping
We collected oral swabs from all participants and placed them in Zymo DNA stabilization solution (https://www.zymoresearch.com/collections/swab-collection/products/dna-rna-shield-collection-tube-w-swab). We sent the oral swabs to the Research Informatics Core at the University of Illinois Chicago for DNA extraction and amplification. The Core performed PCR to amplify and measure SNPs rs429358 and rs7412 that define the common allelic variants of Apolipoprotein E.
Functional assessment
Functional analysis was measured using the Self-Care CARE Assessment from Section GG of the standardized patient assessment data elements in the following domains: Eating, Oral Hygiene, Toileting Hygiene, Shower/Bathe Self, Upper Body Dressing, Lower Body Dressing, Putting on/Taking off Footwear (17). Stroke patients were graded on these domains for how independently they were able to perform them on a scale from 1 to 6, with 1 being dependent and 6 being independent.
NIH toolbox
Cognitive, Emotional, and Sensation function were measured using assessments from the NIH Toolbox. The picture vocabulary test was used to measure long-term or crystallized memory (18). The list sorting test was used to measure short-term memory, attention, and executive function (19). The sadness (20), meaning and purpose (21), self-efficacy (22), and support (23) questionnaires were used to measure self-reported values of these emotional domains. The pain intensity scale (24) was used to measure sensation.
Statistical analyses
All statistical analyses were completed using JMP Statistical Software (SAS, Cary, NC, USA) and R Statistical Software (25). Two-sample t-test and 2-way ANOVA were used to determine differences between groups. The MaAsLin2 R package was used to normalize all variables and employ linear regression analysis to correlate various variables with microbiome measures (26). A false discovery rate of q < 0.25 was used in selecting significant variables to correct for multiple comparisons.
Results
Stroke patients had altered microbiome diversity and composition
We determined the beta diversity of the various groups using the Bray-Curtis dissimilarity index. An ANOSIM R test reveals a significant dissimilarity between the Stroke and At-Risk groups (R = 0.405, p = 0.001) and a significant dissimilarity between the Stroke and Healthy groups (R = 0.126, p = 0.039). The dissimilarity between the At-Risk and Healthy groups was not significant (R = 0.0381, p = 0.228). A detailed analysis of the microbial taxa shows significant decreases in butyrate producers [Agathobaculum butyriciproducens, Lawsonibacter asaccharolyticus, and Anaerostipes hadrus (Figures 1A–C)], secondary bile acid producers [Blautia obeum and the genus Ruminococcus (Figures 1D,E)] and equol producers [Adlercreutzia equolifaciens (Figure 1F)]. There was a significant increase in a couple of pro-inflammatory taxa, including Clostridium bolteae and Ruthenibacterium lactatiformans, (Figures 1G,H).
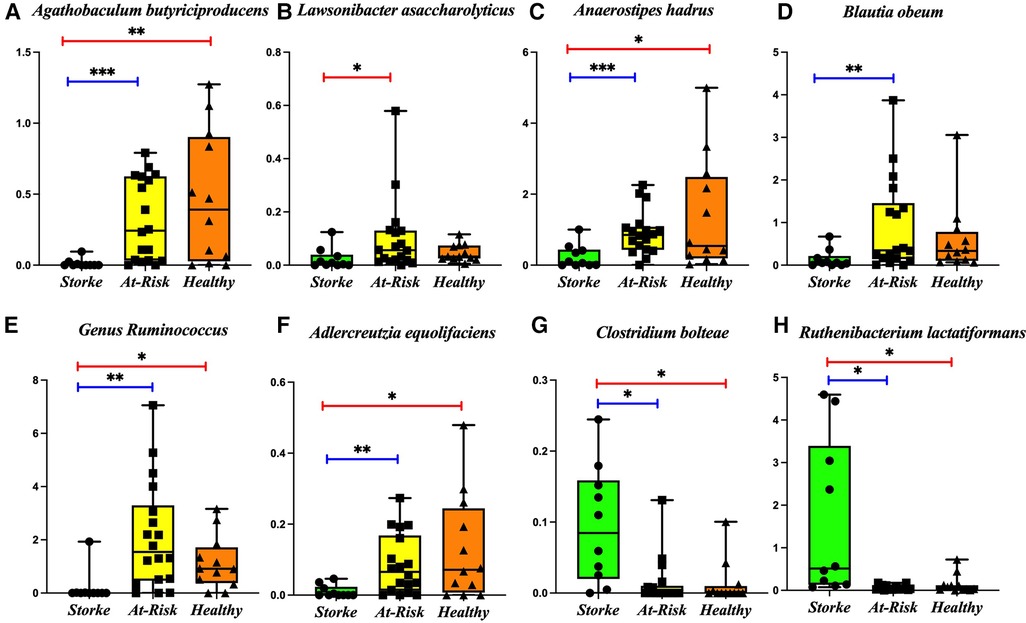
Figure 1. Bacterial taxa significantly changed in the stroke as compared to At-risk and healthy groups. The following taxa are lower in the stroke group: (A) Agathobaculum butyriciproducens, (B) Lawsonibacter asaccharolyticus, (C) Anaerostipes hadrus, (D) Blautia obeum, (E) Genus Ruminococcus, (F) Adlercreutzia equolifaciens, The following taxa are higher in the stroke group: (G) Clostridium bolteae, (H) Ruthenibacterium lactatiformans. *p-value < 0.05; **p-value < 0.01; ***p-value < 0.001.
Stroke dysbiosis is associated with leaky gut markers
Figure 2 shows the average of the leaky gut markers amongst the groups. The calprotectin assay is a marker for intestinal inflammation and did not show differences among the groups (Figure 2A). Alpha-1-antitrypsin is a marker for intestinal permeability and was significantly increased in the Stroke (Figure 2B). Table 2 shows the associations of alpha-1-antitrypsin with various microbial taxa. The presence of alpha-1-antitrypsin was inversely associated with several microbial taxa, including Adlercreutzia equolifaciens, Lawsonibacter asaccharolyticus, the genus Anaerostipes, Blautia obeum, Coprococcus eutactus, Dorea longicatena, Lachnospira pectinoschiza, the genus Roseburia, Agathobaculum butyriciproducens, and the genus Ruminococcus (Figures 3A–J). Alpha-1-antitrypsin was positively associated with Eggerthella lenta, Clostridium bolteae, Anaerotruncus colihominis, and Clostridium leptum (Figures 3K–N).
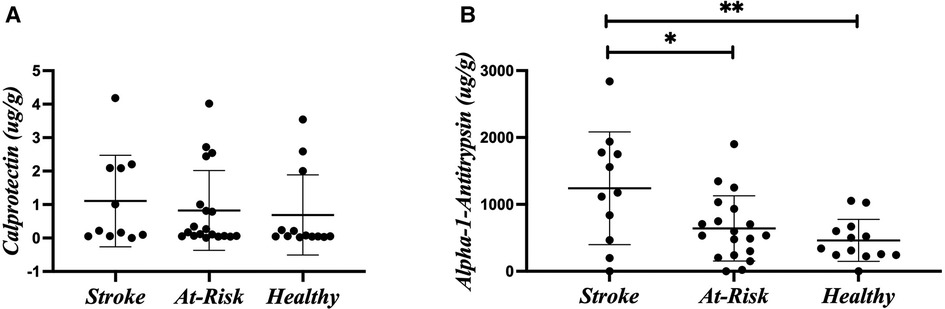
Figure 2. Leaky gut markers. (A) Calprotectin. (B) Alpha-1-antitrypsin. Leaky gut markers were compared amongst the participant groups using Kruskal-Wallis Test. *p-value < 0.05; **p-value < 0.01.
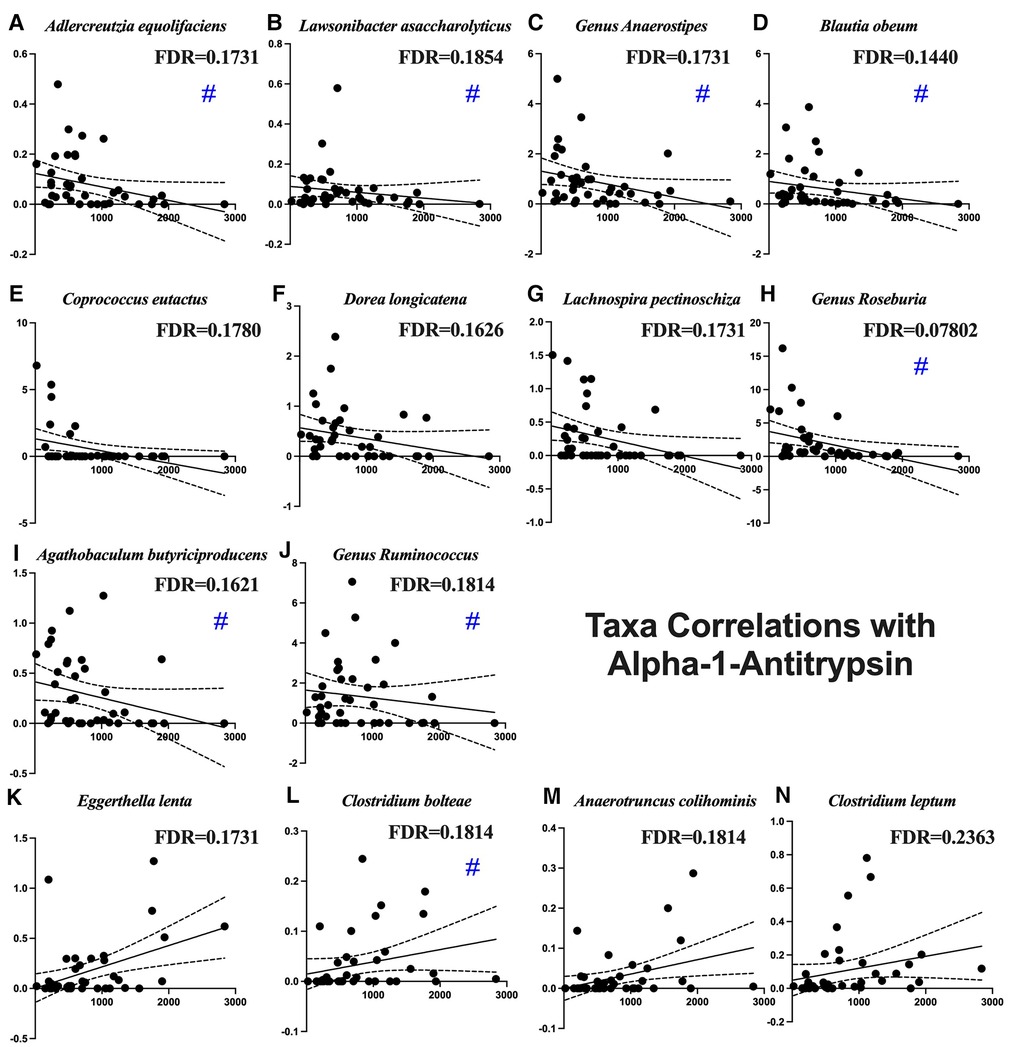
Figure 3. Microbial taxa associated with fecal alpha-1-antitrypsin. Blue pound signs indicate bacteria which were also associated with stroke. Negative associations include (A) Adlercreutzia equolifaciens, (B) Lawsonibacter asaccharolyticus, (C) the genus Anaerostipes, (D) Blautia obeum, (E) Coprococcus eutactus, (F) Dorea longicatena, (G) Lachnospira pectinoschiza, (H) the genus Roseburia, (I) Agathobaculum butyriciproducens, and (J) the genus Ruminococcus. Positive associations include (K) Eggerthella lenta, (L) Clostridium bolteae, (M) Anaerotruncus colihominis, and (N) Clostridium leptum.
Bacteria are associated with dietary intake
Figure 4 shows the diet composition amongst the different participant groups. The Stroke group ate significantly less fiber (Figure 4A) and fruits and vegetables (Figure 4B) and more sugar sweetened beverages (Figure 4C). We correlated diet composition with microbial taxa in the Stroke group using linear correlation (Table 3). We found that an increase of fruits and vegetables was associated with a lower abundance of the genus Bacteroides, Eisenbergiella massiliensis, and Holdemania filiformis (Figures 5A–C) and a higher abundance of Ruminococcus torques and Faecalibacterium prausnitzii (Figures 5D,E). A higher intake of vegetables only was associated with an increase in the relative abundance of Clostridium bolteae (Figure 5F).
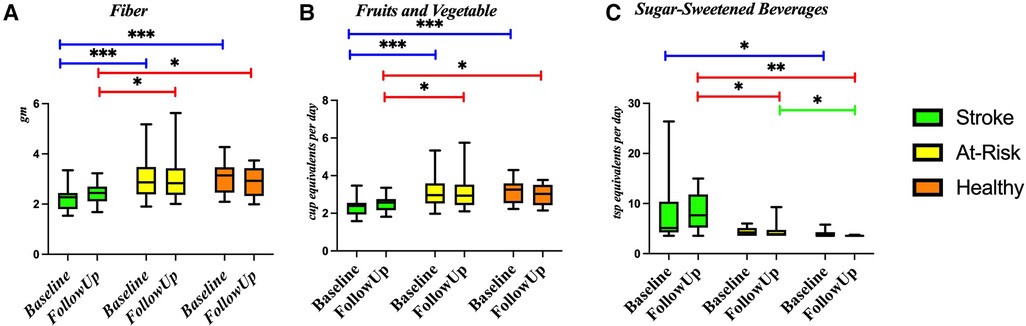
Figure 4. Diet composition amongst the groups. Diet was compared between each of the groups using a Wilcoxon rank sum test in several components: (A) fiber, (B) fruit and vegetables, and (C) sugar-sweetened beverages. *p-value < 0.05; **p-value < 0.01; ***p-value < 0.001.
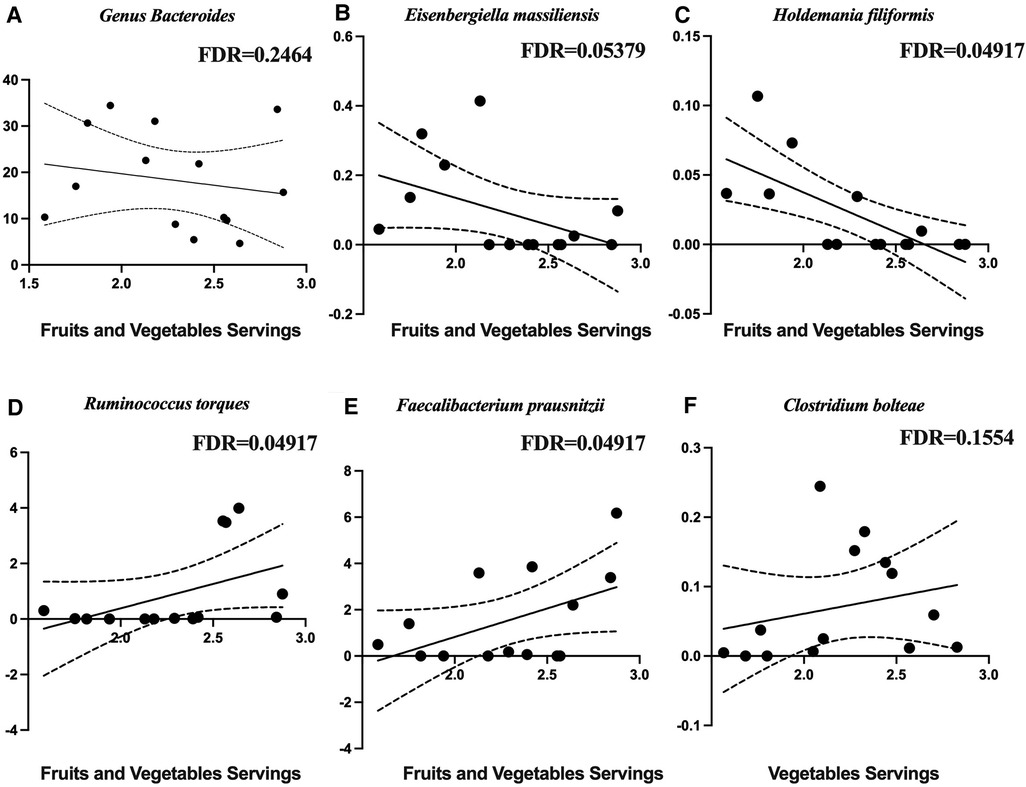
Figure 5. Microbial taxa associated with diet in the stroke participants. Negative associations with fruits and vegetables are with (A) the genus Bacteroides, (B) Eisenbergiella massiliensis, and (C) Holdemania filiformis. Positive associations with fruits and vegetables are with (D) Ruminococcus torques, (E) Faecalibacterium prausnitzii, and (F) clostridium bolteae.
Functional changes induced by stroke
On the Self Care Assessment, the Stroke group reported an average score of 25.4 out of 42 on admission to the hospital and an average score of 36.6 on discharge from the hospital (Figure 6A). On average, the Stroke group scored in the 35th percentile on the picture vocabulary test, the At-Risk group scored in the 52nd percentile, and the Healthy group scored in the 53rd percentile (Figure 6B). On the list sorting test, the Stroke group scored in the 38th percentile, the At-Risk group scored in the 52nd percentile, and the Healthy group scored in the 57th percentile (Figure 6C). On the pain questionnaire, the Stroke group reported a 4.9 out of 10, the At-Risk group reported a 2 out of 10, and the Healthy group reported a 3.25 out of 10 (Figure 6D).
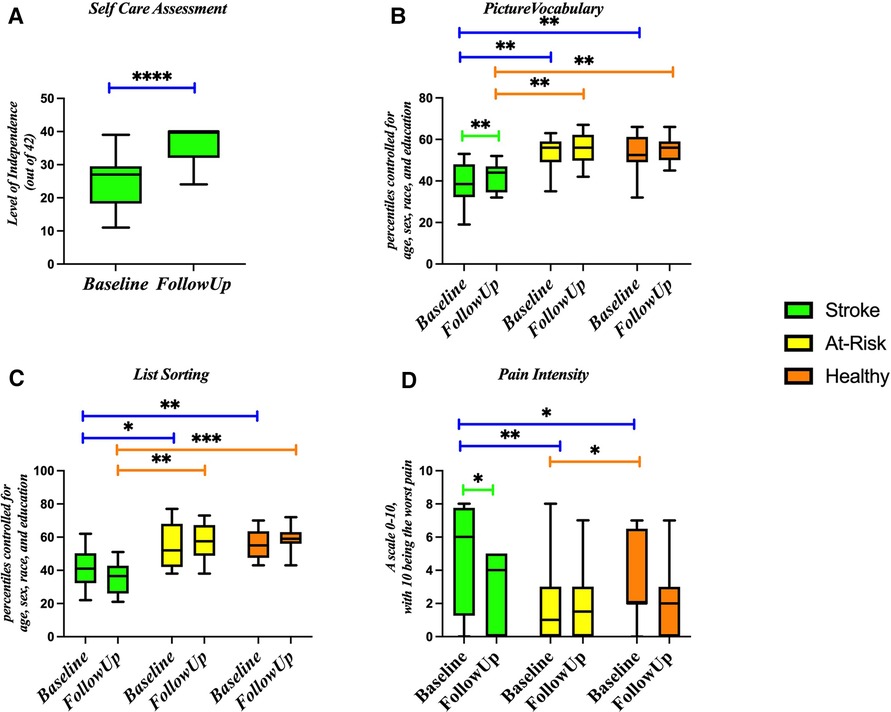
Figure 6. Markers of stroke function at baseline and follow up for stroke, at-risk, and healthy participants. Assessments include (A) self-care assessment, (B) picture vocabulary, (C) list sorting, and (D) pain intensity. *p-value < 0.05; **p-value < 0.01; ***p-value < 0.001.
Microbiota are associated with markers of stroke recovery
Table 4 shows the correlations. Collinsella aerofaciens was positively correlated with self care scores. The genus Roseburia was positively correlated with scores on the picture vocabulary test for the Stroke group. Bacteroides uniformis and Alistipes putredinis were positively correlated with self-efficacy score and Escherichia coli was negatively correlated with self-efficacy. From the Actinobacteria phylum, the class Coriobacteriia is positively correlated with support. From the Bacteroidetes phylum, the family Odoribacteraceae is positively correlated with support. From the Firmicutes phylum, the genus Eubacterium, the family Acidaminococcaceae, Roseburia intestinalis, and Phascolarctobacterium faecium are positively correlated with support. From the Bacteroidetes phylum, Bacteroides ovatus is negatively correlated with support. From the Firmicutes phylum, Erysipelatoclostridium ramosum and Flavonifractor plautii were negatively correlated with support. From the Proteobacteria phylum, the family Veillonellaceae was negatively correlated with support. The family Eubacteriaceae was positively correlated with scores on the meaning and purpose questionnaire. In the Stroke group, Alistipes shahii was positively correlated with pain scores.
Discussion
Here we measured the gut microbiome in the first three weeks of rehabilitation following stroke and its associations with leaky gut markers, dietary intake, and functional recovery measures in 12 stroke participants, 18 control participants with risk factors for stroke, and 12 Healthy participants. We found significantly lower abundances of butyrate producers, secondary bile acid producers, equol producers, and sulfate reducers in the Stroke group and significantly higher abundances of pro-inflammatory taxa. We found no differences between with the At-Risk and Healhty groups, suggesting that the microbiome differences are associated with the stroke itself and not the underlying risk factors.
We found significant dissimilarity between the groups on beta diversity which is consistent with previous human studies that found high dissimilarity between ischemic stroke patients and healthy (27, 28). A detailed analysis of the relative abundance of the microbial taxa revealed several taxa that were lower in the Stroke group compared to either of the control groups. Agathobaculum butyriciproducens is a strictly anaerobic and butyric acid-producing bacteria that has had impressive success in restoring cognition in Alzheimer's disease mouse models (29). Anaerostipes hadrus can produce butyrate from carbohydrates or lactate (30, 31) and is often decreased in diabetes (32). Eubacterium rectale is also a butyrate producer (33) responsible for metabolizing dietary plant polysaccharides (34); it is increased in obesity (35) and reduces inflammatory dendritic cells (33). Lawsonibacter asaccharolyticus is also a butyrate producer (36). Blautia obeum is a natural producer of bile salt hydrolases (37) as well as lantibiotics that inhibit the growth of pathogenic bacteria (38); it has previously been shown to be decreased in acute cerebral infarction (39). Roseburia is a butyrate producer (40) that utilizes acetate (31) and increases serotonin and melatonin and is reduced in ulcerative colitis (41) and hypertension (42); treatment with Roseburia hominis in ulcerative colitis has been shown to strengthen gut barrier function and enhance T regulatory cells (43). Ruminococcus bacteria produce secondary bile acids (44). Adlercreutzia equolifaciens is an equol producer (45) and low abundances have been associated with primary sclerosing cholangitis (46). Desulfovibrionaceae reduces sulfate, a compound causing diarrhea and intestinal pain in overabundance (47).
Several bacterial taxa were significantly higher in the Stroke group as compared to the control groups. Ruthenibacterium lactatiformans is an obligate anaerobe that is a major lactate producer and is also found to be increased in patients with multiple sclerosis (48). Clostridium bolteae is an obligate anaerobe commonly found to be increased in patients with autism (49), neuromyelitis optica spectrum disorders (50), multiple sclerosis (51, 52), and spondyloarthritis (53). Acidaminococcus intestini has been associated with a pro-inflammatory diet (54). Increased abundance of the genus Lachnoclostridium is associated with ulcerative colitis (55) and obesity (56). The genus Anaeromassilibacillus is associated with malnutrition (57). These bacteria are all associated with negative outcomes prevalent in stroke.
Importantly, the Stroke contained a significant percentage of APOE ε2 carriers, which potentially shapes the gut microbiome characteristics for this group with a higher relative abundance of Ruminococcaceae and Gemmiger species and a lower abundance of Prevotellaceae species (58).
Alpha-1-antitrypsin can be a marker of increased intestinal permeability when it is found in the stool. Calprotectin can be a marker of intestinal inflammation. There was a large increase of fecal alpha-1-antitrypsin in our participants with stroke. Gut inflammatory and immune responses following stroke are central to this increased gut permeability. The bacteria that we found are disrupted following stroke that also correlate with alpha-1-antitrypsin include increased Clostridium bolteae and decreased Adlercreutzia equolifaciens, Anaerostipes, Roseburia, Ruminococcus, Blautia obeum, Agathobaculum butyriciproducens, and Lawsonibacter asaccharolyticus. Roseburia has consistently been associated with intestinal permeability both as a microbe that bolsters intestinal permeability (59) and as a microbe that changes in response to changes in the intestinal permeability (60–62). We did not see a significant increase in calprotectin, likely because it is less stable at room temperature (63).
We found that certain dietary features are associated with the abundance of specific bacteria. This is congruent with other groups who have found that dietary modification is associated with gut microbiome composition (64). In our study particularly, we found that most of the bacteria that were affected by stroke were not affected by diet, with the exception of a positive relationship between Clostridium bolteae and vegetable intake. This is interesting since Clostridium bolteae was increased in stroke. There are many studies that describe the effects of a vegetarian diet on the microbiome, noting its association with an increased prevalence of anti-inflammatory species (65, 66). It is possible that dietary elements could promote a microbiome favoring functional recovery following stroke.
Our results indicate that the participants performed functionally better at discharge than at admission to the hospital. This is expected in the rehab setting. The species Collinsella aerofaciens was positively correlated with the Self-Care Assessment. Collinsella aerofaciens is a natural bile salt hydrolase producer (37, 67) that is naturally increased in response to a cow milk supplemented diet (68, 69). While Collinsella aerofaciens is generally considered to be a pro-inflammatory species that increases gut permeability (70), it has been associated with healthy clinical outcomes (71). While bile acids do not normally cross the blood brain barrier, in the context of a leaky blood brain barrier, they can accumulate in the hypothalamus and inhibit the hypothalamic-pituitary-adrenal axis, thereby suppressing the inflammatory response (72, 73).
Participants in the Stroke group perform more poorly on cognitive tests compared to those in the control groups. Cognitive impairment is common following stroke and is often the precursor to dementia and cognitive decline (74). Amongst stroke participants, the genus Roseburia was positively correlated with performance on the picture vocabulary test. Previous groups have seen a correlation of memory performance with Roseburia (75, 76). It is possible that Roseburia enhances memory performance through butyrate production since butyrate has been shown to be a cognitive enhancer of a weak memory (77).
We found that the Stroke group reported lower self-efficacy than the control groups. Bacteroides uniformis and Alistipes putredinis were positively correlated with self-efficacy and Escherichia coli was negatively correlated with self-efficacy. The concept of self-efficacy encapsulates a person's perception of their capability for performance (78). Self-efficacy has been shown to be a strong variable in impacting recovery following stroke (79). While it is not known why these taxa correlate with self-efficacy, it is possible that individuals with a higher self-efficacy are more likely to make healthy choices following stroke which would correlate with butyrate producing bacteria like Bacteroides uniformis as opposed to inflammatory species like Escherichia coli (80). The Enterobacteriaceae family, which E. coli is from, has previously been reported as being associated with bad outcomes in the context of stroke (15, 81, 82).
The class Coriobacteriia was positively correlated with support. Social isolation stress has been shown to alter the gut-brain axis (83). The class Coriobacteriia contains many species which are equol producers. Since equol producers and butyrate producers are associated with social support, it is possible that one mechanism by which people who have strokes and who have more support do better is mediated by the microbiome.
The Stroke group reported experiencing more pain than the control groups. Pain is a very common phenomenon following stroke and can include complex regional pain syndrome, musculoskeletal pain, spasticity-related pain, and post-stroke headache (84). In the Stroke group, Alistipes shahii was positively associated with reported pain. The abundance of Alistipes shahii is highly positively correlated with trimethylamine-N-oxide (TMAO), a marker of poor cardiometabolic health (85). While it is unknown how Alistipes shahii is associated with pain, many other studies have linked the microbiome with pain (86), including other species of Alistipes (87).
While this study provides valuable insights into the associations of the composition of bacterial communities in the gut and various markers of stroke recovery, it has many limitations. As a prospective case control study, it cannot definitively say that the associations are causing stroke recovery to be altered. Future experiments should test the associations found here to determine causation. Further, we did not have access to cognitive status prior to stroke or baseline stroke severity due to the recruitment procedure feasibility. We acknowledge that these are major limitation as the previous cognitive status could influence the microbiome composition and performance on post-stroke cognitive assessments and the baseline stroke severity could impact potential for recovery. Additionally, our sample consists largely of older white adults from Kentucky. Larger studies comprising more diverse populations are needed to see whether these associations are generalizable. Furthermore, we used a false discovery rate of q < 0.25. This means that up to 25% of our found associations may be false positives. More targeted experiments are needed in the future to better characterize these associations.
Altogether, we found that stroke was associated with an increase of pro-inflammatory bacterial taxa and a decrease in taxa that produce butyrate and secondary bile acids necessary for healthy metabolic function. This shift towards inflammation is likely due to the activation of the sympathetic nervous system and hypothalamic-pituitary-adrenal axis in response to the stroke that increases gut permeability and decreases gut motility. While this inflammatory shift can help to mitigate the acute effects of stroke in the brain, the residual inflammation in the weeks and months following the stroke is likely undermining recovery. Previous studies have also found that butyrate-producing bacteria were significantly reduced in cerebral ischemia patients and that this reduction is associated with poor outcomes (27, 88, 89). Future studies should explore treatments targeting the composition of microbial communities following stroke as a way to boost recovery from stroke in combination with other rehabilitation therapies. It is possible that optimizing butyrate producers, secondary bile acid producers, equol producers, and sulfate reducers in the gut through dietary interventions (90–93) could contribute to creating a rehabilitation environment where recovery is boosted.
Data availability statement
The datasets presented in this study can be found in online repositories. The names of the repository/repositories and accession number(s) can be found below: https://www.ncbi.nlm.nih.gov/, Under review.
Ethics statement
The studies involving human participants were reviewed and approved by Institutional Review Board (IRB) of the University of Kentucky. The patients/participants provided their written informed consent to participate in this study.
Author contributions
TCH, LS, and A-LL designed research; TCH, EP, SB, JF, ATY, LMY, Y-HC, XX, KP, LS, and A-LL conducted research; TCH, SG, GC, XX and AS performed statistical analysis; TCH, ATY, LMY, Y-HC, XX, JES, KP, SH, AS, LS, and A-LL wrote paper; TCH, JES, KP, AS, LS, and A-LL had primary responsibility for final content. All authors contributed to the article and approved the submitted version.
Funding
This research was supported by grants from NIH/NIA, NIH/ODS, and American Federation for Aging Research to A-LL (RF1AG062480) and TCH (T32AG057461).
Acknowledgments
The authors would like to thank the generous staff at Cardinal Hill Rehabilitation Hospital for the recruitment of participants and collection and storage of samples. We thank Dr. Frederick A. Schmitt at the University of Kentucky for assisting the NIH Toolbox operation. Bioinformatics analysis in the project described was performed by the UIC Research Informatics Core, supported in part by NCATS through Grant UL1TR002003. This article was prepared while Lumy Sawaki was employed at the University of Kentucky. The opinions expressed in this article are the author's own and do not reflect the view of the National Institutes of Health, the Department of Health and Human Services, or the United States government.
Conflict of interest
The authors declare that the research was conducted in the absence of any commercial or financial relationships that could be construed as a potential conflict of interest.
Publisher's note
All claims expressed in this article are solely those of the authors and do not necessarily represent those of their affiliated organizations, or those of the publisher, the editors and the reviewers. Any product that may be evaluated in this article, or claim that may be made by its manufacturer, is not guaranteed or endorsed by the publisher.
References
1. Benjamin EJ, Blaha MJ, Chiuve SE, Cushman M, Das SR, Deo R, et al. Heart disease and stroke statistics-2017 update: a report from the American heart association. Circulation. (2017) 135(10):e146–603. doi: 10.1161/CIR.0000000000000485
2. Moy E, Garcia MC, Bastian B, Rossen LM, Ingram DD, Faul M, et al. Leading causes of death in nonmetropolitan and metropolitan areas- United States, 1999–2014. MMWR Surveill Summ. (2017) 66(1):1–8. doi: 10.15585/mmwr.mm6601a1
3. Brewer L, Horgan F, Hickey A, Williams D. Stroke rehabilitation: recent advances and future therapies. QJM. (2013) 106(1):11–25. doi: 10.1093/qjmed/hcs174
4. Carandang R, Seshadri S, Beiser A, Kelly-Hayes M, Kase CS, Kannel WB, et al. Trends in incidence, lifetime risk, severity, and 30-day mortality of stroke over the past 50 years. Jama. (2006) 296(24):2939–46. doi: 10.1001/jama.296.24.2939
5. Leung K, Thuret S. Gut microbiota: a modulator of brain plasticity and cognitive function in ageing. Healthcare. (2015) 3(4):898–916. doi: 10.3390/healthcare3040898
6. Stanley D, Moore RJ, Wong CHY. An insight into intestinal mucosal microbiota disruption after stroke. Sci Rep. (2018) 8(1):568. doi: 10.1038/s41598-017-18904-8
7. Singh V, Roth S, Llovera G, Sadler R, Garzetti D, Stecher B, et al. Microbiota dysbiosis controls the neuroinflammatory response after stroke. J Neurosci. (2016) 36(28):7428–40. doi: 10.1523/JNEUROSCI.1114-16.2016
8. Winek K, Meisel A, Dirnagl U. Gut microbiota impact on stroke outcome: fad or fact? J Cereb Blood Flow Metab. (2016) 36(5):891–8. doi: 10.1177/0271678X16636890
9. Mailing LJ, Allen JM, Buford TW, Fields CJ, Woods JA. Exercise and the gut microbiome: a review of the evidence, potential mechanisms, and implications for human health. Exerc Sport Sci Rev. (2019) 47(2):75–85. doi: 10.1249/JES.0000000000000183
10. Wang W, Li X, Yao X, Cheng X, Zhu Y. The characteristics analysis of intestinal microecology on cerebral infarction patients and its correlation with apolipoprotein E. Medicine. (2018) 97(41):e12805. doi: 10.1097/MD.0000000000012805
11. Yamashiro K, Tanaka R, Urabe T, Ueno Y, Yamashiro Y, Nomoto K, et al. Gut dysbiosis is associated with metabolism and systemic inflammation in patients with ischemic stroke. PLoS One. (2017) 12(2):e0171521. doi: 10.1371/journal.pone.0171521
12. Yin J, Liao SX, He Y, Wang S, Xia GH, Liu FT, et al. Dysbiosis of gut Microbiota with reduced trimethylamine-N-oxide level in patients with large-artery atherosclerotic stroke or transient ischemic attack. J Am Heart Assoc. (2015) 4(11):e002699. doi: 10.1161/JAHA
13. Li N, Wang X, Sun C, Wu X, Lu M, Si Y, et al. Change of intestinal microbiota in cerebral ischemic stroke patients. BMC Microbiol. (2019) 19(1):191. doi: 10.1186/s12866-019-1552-1
14. Xia GH, You C, Gao XX, Zeng XL, Zhu JJ, Xu KY, et al. Stroke dysbiosis index (SDI) in gut microbiome are associated with brain injury and prognosis of stroke. Front Neurol. (2019) 10:397. doi: 10.3389/fneur.2019.00397
15. Xu R, Tan C, Zhu J, Zeng X, Gao X, Wu Q, et al. Dysbiosis of the intestinal microbiota in neurocritically ill patients and the risk for death. Crit Care. (2019) 23(1):195. doi: 10.1186/s13054-019-2488-4
16. Beghini F, McIver LJ, Blanco-Miguez A, Dubois L, Asnicar F, Maharjan S, et al. Integrating taxonomic, functional, and strain-level profiling of diverse microbial communities with bioBakery 3. Elife. (2021) 10:e65088. doi: 10.7554/eLife.65088
17. Li CY, Mallinson T, Kim H, Graham J, Kuo YF, Ottenbacher KJ. Characterizing standardized functional data at inpatient rehabilitation facilities. J Am Med Dir Assoc. (2022):S1525-8610(22)00166-9. doi: 10.1016/j.jamda.2022.02.003
18. Parsey CM, Bagger JE, Trittschuh EH, Hanson AJ. Utility of the iPad NIH toolbox cognition battery in a clinical trial of older adults. J Am Geriatr Soc. (2021) 69(12):3519–28. doi: 10.1111/jgs.17382
19. Tulsky DS, Carlozzi N, Chiaravalloti ND, Beaumont JL, Kisala PA, Mungas D, et al. NIH toolbox cognition battery (NIHTB-CB): list sorting test to measure working memory. J Int Neuropsychol Soc. (2014) 20(6):599–610. doi: 10.1017/S135561771400040X
20. Pilkonis PA, Choi SW, Salsman JM, Butt Z, Moore TL, Lawrence SM, et al. Assessment of self-reported negative affect in the NIH toolbox. Psychiatry Res. (2013) 206(1):88–97. doi: 10.1016/j.psychres.2012.09.034
21. Salsman JM, Lai JS, Hendrie HC, Butt Z, Zill N, Pilkonis PA, et al. Assessing psychological well-being: self-report instruments for the NIH toolbox. Qual Life Res. (2014) 23(1):205–15. doi: 10.1007/s11136-013-0452-3
22. Kupst MJ, Butt Z, Stoney CM, Griffith JW, Salsman JM, Folkman S, et al. Assessment of stress and self-efficacy for the NIH toolbox for neurological and behavioral function. Anxiety Stress Coping. (2015) 28(5):531–44. doi: 10.1080/10615806.2014.994204
23. Cyranowski JM, Zill N, Bode R, Butt Z, Kelly MA, Pilkonis PA, et al. Assessing social support, companionship, and distress: National Institute of Health (NIH) toolbox adult social relationship scales. Health Psychol. (2013) 32(3):293–301. doi: 10.1037/a0028586
24. Cook KF, Dunn W, Griffith JW, Morrison MT, Tanquary J, Sabata D, et al. Pain assessment using the NIH toolbox. Neurology. (2013) 80(11 Suppl 3):S49–53. doi: 10.1212/WNL.0b013e3182872e80
25. R Core Team. R: A language and environment for statistical computing. Vienna, Austria: R Foundation for Statistical Computing (2020).
26. Mallick H, Rahnavard A, McIver LJ, Ma S, Zhang Y, Nguyen LH, et al. Multivariable association discovery in population-scale meta-omics studies. PLoS Comput Biol. (2021) 17(11):e1009442. doi: 10.1371/journal.pcbi.1009442
27. Haak BW, Westendorp WF, van Engelen TSR, Brands X, Brouwer MC, Vermeij JD, et al. Disruptions of anaerobic gut bacteria are associated with stroke and post-stroke infection: a prospective case-control study. Transl Stroke Res. (2021) 12(4):581–92. doi: 10.1007/s12975-020-00863-4
28. Dang Y, Zhang X, Zheng Y, Yu B, Pan D, Jiang X, et al. Distinctive gut microbiota alteration is associated with poststroke functional recovery: results from a prospective cohort study. Neural Plast. (2021) 2021:1469339. doi: 10.1155/2021/1469339
29. Go J, Chang DH, Ryu YK, Park HY, Lee IB, Noh JR, et al. Human gut microbiota agathobaculum butyriciproducens improves cognitive impairment in LPS-induced and APP/PS1 mouse models of Alzheimer’s disease. Nutr Res. (2021) 86:96–108. doi: 10.1016/j.nutres.2020.12.010
30. Allen-Vercoe E, Daigneault M, White A, Panaccione R, Duncan SH, Flint HJ, et al. Anaerostipes hadrus comb. nov., a dominant species within the human colonic microbiota; reclassification of eubacterium hadrum Moore et al. 1976. Anaerobe. (2012) 18(5):523–9. doi: 10.1016/j.anaerobe.2012.09.002
31. Louis P, Flint HJ. Formation of propionate and butyrate by the human colonic microbiota. Environ Microbiol. (2017) 19(1):29–41. doi: 10.1111/1462-2920.13589
32. Balvers M, Deschasaux M, van den Born BJ, Zwinderman K, Nieuwdorp M, Levin E. Analyzing type 2 diabetes associations with the gut microbiome in individuals from two ethnic backgrounds living in the same geographic area. Nutrients. (2021) 13(9):3289. doi: 10.3390/nu13093289
33. Islam SMS, Ryu HM, Sayeed HM, Byun HO, Jung JY, Kim HA, et al. Eubacterium rectale attenuates HSV-1 induced systemic inflammation in mice by inhibiting CD83. Front Immunol. (2021) 12:712312. doi: 10.3389/fimmu.2021.712312
34. David LA, Maurice CF, Carmody RN, Gootenberg DB, Button JE, Wolfe BE, et al. Diet rapidly and reproducibly alters the human gut microbiome. Nature. (2014) 505(7484):559–63. doi: 10.1038/nature12820
35. Gomes AC, Hoffmann C, Mota JF. The human gut microbiota: metabolism and perspective in obesity. Gut Microbes. (2018) 9(4):308–25. doi: 10.1080/19490976.2018.1465157
36. Sakamoto M, Iino T, Yuki M, Ohkuma M. Lawsonibacter asaccharolyticus gen. nov., sp. nov., a butyrate-producing bacterium isolated from human faeces. Int J Syst Evol Microbiol. (2018) 68(6):2074–81. doi: 10.1099/ijsem.0.002800
37. Mullish BH, McDonald JAK, Pechlivanis A, Allegretti JR, Kao D, Barker GF, et al. Microbial bile salt hydrolases mediate the efficacy of faecal microbiota transplant in the treatment of recurrent clostridioides difficile infection. Gut. (2019) 68(10):1791–800. doi: 10.1136/gutjnl-2018-317842
38. Hatziioanou D, Gherghisan-Filip C, Saalbach G, Horn N, Wegmann U, Duncan SH, et al. Discovery of a novel lantibiotic nisin O from blautia obeum A2-162, isolated from the human gastrointestinal tract. Microbiology (Reading). (2017) 163(9):1292–305. doi: 10.1099/mic.0.000515
39. Huang L, Wang T, Wu Q, Dong X, Shen F, Liu D, et al. Analysis of microbiota in elderly patients with acute cerebral infarction. PeerJ. (2019) 7:e6928. doi: 10.7717/peerj.6928
40. Song L, He M, Sun Q, Wang Y, Zhang J, Fang Y, et al. Roseburia hominis increases intestinal melatonin level by activating p-CREB-AANAT pathway. Nutrients. (2021) 14(1):117. doi: 10.3390/nu14010117
41. Machiels K, Joossens M, Sabino J, De Preter V, Arijs I, Eeckhaut V, et al. A decrease of the butyrate-producing species Roseburia hominis and Faecalibacterium prausnitzii defines dysbiosis in patients with ulcerative colitis. Gut. (2014) 63(8):1275–83. doi: 10.1136/gutjnl-2013-304833
42. Calderon-Perez L, Gosalbes MJ, Yuste S, Valls RM, Pedret A, Llaurado E, et al. Gut metagenomic and short chain fatty acids signature in hypertension: a cross-sectional study. Sci Rep. (2020) 10(1):6436. doi: 10.1038/s41598-020-63475-w
43. Patterson AM, Mulder IE, Travis AJ, Lan A, Cerf-Bensussan N, Gaboriau-Routhiau V, et al. Human gut symbiont Roseburia hominis promotes and regulates innate immunity. Front Immunol. (2017) 8:1166. doi: 10.3389/fimmu.2017.01166
44. Sinha SR, Haileselassie Y, Nguyen LP, Tropini C, Wang M, Becker LS, et al. Dysbiosis-induced secondary bile acid deficiency promotes intestinal inflammation. Cell Host Microbe. (2020) 27(4):659–70 e5. doi: 10.1016/j.chom.2020.01.021
45. Vazquez L, Florez AB, Rodriguez J, Mayo B. Heterologous expression of equol biosynthesis genes from adlercreutzia equolifaciens. FEMS Microbiol Lett. (2021) 368(13):fnab082. doi: 10.1093/femsle/fnab082
46. Bajer L, Kverka M, Kostovcik M, Macinga P, Dvorak J, Stehlikova Z, et al. Distinct gut microbiota profiles in patients with primary sclerosing cholangitis and ulcerative colitis. World J Gastroenterol. (2017) 23(25):4548–58. doi: 10.3748/wjg.v23.i25.4548
47. Van Hecke T, De Vrieze J, Boon N, De Vos WH, Vossen E, De Smet S. Combined consumption of beef-based cooked mince and sucrose stimulates oxidative stress, cardiac hypertrophy, and colonic outgrowth of Desulfovibrionaceae in rats. Mol Nutr Food Res. (2019) 63(2):e1800962. doi: 10.1002/mnfr.201800962
48. Shkoporov AN, Chaplin AV, Shcherbakova VA, Suzina NE, Kafarskaia LI, Bozhenko VK, et al. Ruthenibacterium lactatiformans gen. nov., sp. nov., an anaerobic, lactate-producing member of the family Ruminococcaceae isolated from human faeces. Int J Syst Evol Microbiol. (2016) 66(8):3041–9. doi: 10.1099/ijsem.0.001143
49. Pequegnat B, Monteiro MA. Carbohydrate scaffolds for the study of the autism-associated bacterium, clostridium bolteae. Curr Med Chem. (2019) 26(35):6341–8. doi: 10.2174/0929867326666190225164527
50. Pandit L, Cox LM, Malli C, D’Cunha A, Rooney T, Lokhande H, et al. Clostridium bolteae is elevated in neuromyelitis optica spectrum disorder in India and shares sequence similarity with AQP4. Neurol Neuroimmunol Neuroinflamm. (2021) 8(1):e907. doi: 10.1212/NXI.0000000000000907
51. Cox LM, Maghzi AH, Liu S, Tankou SK, Dhang FH, Willocq V, et al. Gut microbiome in progressive multiple sclerosis. Ann Neurol. (2021) 89(6):1195–211. doi: 10.1002/ana.26084
52. Song Y, Liu C, Molitoris DR, Tomzynski TJ, Lawson PA, Collins MD, et al. Clostridium bolteae sp. nov., isolated from human sources. Syst Appl Microbiol. (2003) 26(1):84–9. doi: 10.1078/072320203322337353
53. Wang L, Wang Y, Zhang P, Song C, Pan F, Li G, et al. Gut microbiota changes in patients with spondyloarthritis: a systematic review. Semin Arthritis Rheum. (2022) 52:151925. doi: 10.1016/j.semarthrit.2021.11.002
54. Zheng J, Hoffman KL, Chen JS, Shivappa N, Sood A, Browman GJ, et al. Dietary inflammatory potential in relation to the gut microbiome: results from a cross-sectional study. Br J Nutr. (2020) 124(9):931–42. doi: 10.1017/S0007114520001853
55. Wang CS, Li WB, Wang HY, Ma YM, Zhao XH, Yang H, et al. VSL#3 can prevent ulcerative colitis-associated carcinogenesis in mice. World J Gastroenterol. (2018) 24(37):4254–62. doi: 10.3748/wjg.v24.i37.4254
56. Zhou L, Ni Z, Yu J, Cheng W, Cai Z, Yu C. Correlation between fecal metabolomics and gut Microbiota in obesity and polycystic ovary syndrome. Front Endocrinol. (2020) 11:628. doi: 10.3389/fendo.2020.00628
57. Guilhot E, Tidjani Alou M, Diallo A, Raoult D, Khelaifia S. Anaeromassilibacillus senegalensis gen. nov., sp. nov., isolated from the gut of a child with kwashiorkor. New Microbes New Infect. (2016) 12:59–60. doi: 10.1016/j.nmni.2016.04.007
58. Tran TTT, Corsini S, Kellingray L, Hegarty C, Le Gall G, Narbad A, et al. APOE Genotype influences the gut microbiome structure and function in humans and mice: relevance for Alzheimer’s disease pathophysiology. FASEB J. (2019) 33(7):8221–31. doi: 10.1096/fj.201900071R
59. Seo B, Jeon K, Moon S, Lee K, Kim WK, Jeong H, et al. Roseburia spp. Abundance associates with alcohol consumption in humans and its administration ameliorates alcoholic fatty liver in mice. Cell Host Microbe. (2020) 27(1):25–40 e6. doi: 10.1016/j.chom.2019.11.001
60. Plaza-Diaz J, Solis-Urra P, Rodriguez-Rodriguez F, Olivares-Arancibia J, Navarro-Oliveros M, Abadia-Molina F, et al. The gut barrier, intestinal microbiota, and liver disease: molecular mechanisms and strategies to manage. Int J Mol Sci. (2020) 21(21):8351. doi: 10.3390/ijms21218351
61. Khanna S, Bishnoi M, Kondepudi KK, Shukla G. Synbiotic (lactiplantibacillus pentosus GSSK2 and isomalto-oligosaccharides) supplementation modulates pathophysiology and gut dysbiosis in experimental metabolic syndrome. Sci Rep. (2021) 11(1):21397. doi: 10.1038/s41598-021-00601-2
62. Singh DP, Singh J, Boparai RK, Zhu J, Mantri S, Khare P, et al. Isomalto-oligosaccharides, a prebiotic, functionally augment green tea effects against high fat diet-induced metabolic alterations via preventing gut dysbacteriosis in mice. Pharmacol Res. (2017) 123:103–13. doi: 10.1016/j.phrs.2017.06.015
63. Haisma SM, van Rheenen PF, Wagenmakers L, Muller Kobold A. Calprotectin instability may lead to undertreatment in children with IBD. Arch Dis Child. (2020) 105(10):996–8. doi: 10.1136/archdischild-2018-316584
64. Hills RD Jr., Pontefract BA, Mishcon HR, Black CA, Sutton SC, Theberge CR. Gut microbiome: profound implications for diet and disease. Nutrients. (2019) 11(7):1613. doi: 10.3390/nu11071613
65. Sakkas H, Bozidis P, Touzios C, Kolios D, Athanasiou G, Athanasopoulou E, et al. Nutritional Status and the influence of the vegan diet on the gut Microbiota and human health. Medicina. (2020) 56(2):88. doi: 10.3390/medicina56020088
66. Tang ZZ, Chen G, Hong Q, Huang S, Smith HM, Shah RD, et al. Multi-omic analysis of the microbiome and metabolome in healthy subjects reveals microbiome-dependent relationships between diet and metabolites. Front Genet. (2019) 10:454. doi: 10.3389/fgene.2019.00454
67. Wegner K, Just S, Gau L, Mueller H, Gerard P, Lepage P, et al. Rapid analysis of bile acids in different biological matrices using LC-ESI-MS/MS for the investigation of bile acid transformation by mammalian gut bacteria. Anal Bioanal Chem. (2017) 409(5):1231–45. doi: 10.1007/s00216-016-0048-1
68. Rettedal EA, Altermann E, Roy NC, Dalziel JE. The effects of unfermented and fermented cow and sheep milk on the gut Microbiota. Front Microbiol. (2019) 10:458. doi: 10.3389/fmicb.2019.00458
69. Jacobi SK, Yatsunenko T, Li D, Dasgupta S, Yu RK, Berg BM, et al. Dietary isomers of sialyllactose increase ganglioside sialic acid concentrations in the corpus callosum and cerebellum and modulate the colonic microbiota of formula-fed piglets. J Nutr. (2016) 146(2):200–8. doi: 10.3945/jn.115.220152
70. Balakrishnan B, Luckey D, Taneja V. Autoimmunity-associated gut commensals modulate gut permeability and immunity in humanized mice. Mil Med. (2019) 184(Suppl 1):529–36. doi: 10.1093/milmed/usy309
71. Slanzon GS, Ridenhour BJ, Moore DA, Sischo WM, Parrish LM, Trombetta SC, et al. Fecal microbiome profiles of neonatal dairy calves with varying severities of gastrointestinal disease. PLoS One. (2022) 17(1):e0262317. doi: 10.1371/journal.pone.0262317
72. Dhonnabhain RN, Xiao Q, O’Malley D. Aberrant gut-to-brain signaling in irritable bowel syndrome - the role of bile acids. Front Endocrinol. (2021) 12:745190. doi: 10.3389/fendo.2021.745190
73. McMillin M, Frampton G, Quinn M, Divan A, Grant S, Patel N, et al. Suppression of the HPA axis during cholestasis can be attributed to hypothalamic bile acid signaling. Mol Endocrinol. (2015) 29(12):1720–30. doi: 10.1210/me.2015-1087
74. Coco DL, Lopez G, Corrao S. Cognitive impairment and stroke in elderly patients. Vasc Health Risk Manag. (2016) 12:105–16. doi: 10.2147/VHRM.S75306
75. Sadovnikova IS, Gureev AP, Ignatyeva DA, Gryaznova MV, Chernyshova EV, Krutskikh EP, et al. Nrf2/ARE activators improve memory in aged mice via maintaining of mitochondrial quality control of brain and the modulation of gut microbiome. Pharmaceuticals. (2021) 14(7):607. doi: 10.3390/ph14070607
76. Gu C, Zhou W, Wang W, Xiang H, Xu H, Liang L, et al. Zibupiyin recipe improves cognitive decline by regulating gut microbiota in Zucker diabetic fatty rats. Oncotarget. (2017) 8(17):27693–703. doi: 10.18632/oncotarget.14611
77. Vinarskaya AK, Balaban PM, Roshchin MV, Zuzina AB. Sodium butyrate as a selective cognitive enhancer for weak or impaired memory. Neurobiol Learn Mem. (2021) 180:107414. doi: 10.1016/j.nlm.2021.107414
78. Cervone D. Thinking about self-efficacy. Behav Modif. (2000) 24(1):30–56. doi: 10.1177/0145445500241002
79. Nott M, Wiseman L, Seymour T, Pike S, Cuming T, Wall G. Stroke self-management and the role of self-efficacy. Disabil Rehabil. (2021) 43(10):1410–9. doi: 10.1080/09638288.2019.1666431
80. Lieber AC, Hong E, Putrino D, Nistal DA, Pan JS, Nutrition KC, et al. Nutrition, energy expenditure, dysphagia, and self-efficacy in stroke rehabilitation: a review of the literature. Brain Sci. (2018) 8(12):218. doi: 10.3390/brainsci8120218
81. Ling Y, Gong T, Zhang J, Gu Q, Gao X, Weng X, et al. Gut microbiome signatures are biomarkers for cognitive impairment in patients with ischemic stroke. Front Aging Neurosci. (2020) 12:511562. doi: 10.3389/fnagi.2020.511562
82. Ling Y, Gu Q, Zhang J, Gong T, Weng X, Liu J, et al. Structural change of gut Microbiota in patients with post-stroke comorbid cognitive impairment and depression and its correlation with clinical features. J Alzheimers Dis. (2020) 77(4):1595–608. doi: 10.3233/JAD-200315
83. Donovan M, Mackey CS, Platt GN, Rounds J, Brown AN, Trickey DJ, et al. Social isolation alters behavior, the gut-immune-brain axis, and neurochemical circuits in Male and female prairie voles. Neurobiol Stress. (2020) 13:100278. doi: 10.1016/j.ynstr.2020.100278
84. Harrison RA, Field TS. Post stroke pain: identification, assessment, and therapy. Cerebrovasc Dis. (2015) 39(3-4):190–201. doi: 10.1159/000375397
85. Li J, Li Y, Ivey KL, Wang DD, Wilkinson JE, Franke A, et al. Interplay between diet and gut microbiome, and circulating concentrations of trimethylamine N-oxide: findings from a longitudinal cohort of US men. Gut. (2022) 71(4):724–33. doi: 10.1136/gutjnl-2020-322473
86. Freidin MB, Stalteri MA, Wells PM, Lachance G, Baleanu AF, Bowyer RCE, et al. An association between chronic widespread pain and the gut microbiome. Rheumatology. (2021) 60(8):3727–37. doi: 10.1093/rheumatology/keaa847
87. Saulnier DM, Riehle K, Mistretta TA, Diaz MA, Mandal D, Raza S, et al. Gastrointestinal microbiome signatures of pediatric patients with irritable bowel syndrome. Gastroenterology. (2011) 141(5):1782–91. doi: 10.1053/j.gastro.2011.06.072
88. Li H, Zhang X, Pan D, Liu Y, Yan X, Tang Y, et al. Dysbiosis characteristics of gut microbiota in cerebral infarction patients. Transl Neurosci. (2020) 11(1):124–33. doi: 10.1515/tnsci-2020-0117
89. Sun H, Gu M, Li Z, Chen X, Zhou J. Gut Microbiota dysbiosis in acute ischemic stroke associated with 3-month unfavorable outcome. Front Neurol. (2021) 12:799222. doi: 10.3389/fneur.2021.799222
90. Hoffman JD, Yanckello LM, Chlipala G, Hammond TC, McCulloch SD, Parikh I, et al. Dietary inulin alters the gut microbiome, enhances systemic metabolism and reduces neuroinflammation in an APOE4 mouse model. PLoS One. (2019) 14(8):e0221828. doi: 10.1371/journal.pone.0221828
91. Ma D, Wang A, Parikh I, Green SJ, Hoffman JD, Chlipala G, et al. Ketogenic diet enhances neurovascular function with altered gut microbiome in young healthy mice. Sci Rep. (2018) 8:6670. doi: 10.1038/s41598-018-25190-5
92. Yanckello LM, Fanelli B, McCulloch S, Xing X, Sun M, Hammond TC, et al. Inulin supplementation mitigates gut dysbiosis and brain impairment induced by mild traumatic brain injury during chronic phase. J Cell Immunol. (2022) 4(2):50–64. doi: 10.33696/immunology.4.132
93. Yanckello LM, Hoffman JD, Chang YH, Lin P, Nehra G, Chlipala G, et al. Apolipoprotein E genotype-dependent nutrigenetic effects to prebiotic inulin for modulating systemic metabolism and neuroprotection in mice via gut-brain axis. Nutr Neurosci. (2022) 25(8):1669–79. doi: 10.1080/1028415X.2021.1889452
Keywords: stroke, microbiome, functional recovery, NIH toolbox, butyrate, secondary bile acid, leaky gut, dietary questionnaire
Citation: Hammond TC, Powell E, Green SJ, Chlipala G, Frank J, Yackzan AT, Yanckello LM, Chang Y-H, Xing X, Heil S, Springer JE, Pennypacker K, Stromberg A, Sawaki L and Lin A-L (2022) Functional recovery outcomes following acute stroke is associated with abundance of gut microbiota related to inflammation, butyrate and secondary bile acid. Front. Rehabilit. Sci. 3:1017180. doi: 10.3389/fresc.2022.1017180
Received: 11 August 2022; Accepted: 4 October 2022;
Published: 26 October 2022.
Edited by:
Francesco Lena, Mediterranean Neurological Institute Neuromed (IRCCS), ItalyReviewed by:
Simone Cesarano, University of Molise, ItalyMonica Torre, Center for Rehabilitation, San Stef Consortium. Ar. Abruzzo, Italy
© 2022 Hammond, Powell, Green, Chlipala, Frank, Yackzan, Yanckello, Chang, Xing, Heil, Springer, Pennypacker, Stromberg, Sawaki and Lin. This is an open-access article distributed under the terms of the Creative Commons Attribution License (CC BY). The use, distribution or reproduction in other forums is permitted, provided the original author(s) and the copyright owner(s) are credited and that the original publication in this journal is cited, in accordance with accepted academic practice. No use, distribution or reproduction is permitted which does not comply with these terms.
*Correspondence: Ai-Ling Lin YWktbGluZy5saW5AaGVhbHRoLm1pc3NvdXJpLmVkdQ==
Specialty Section: This article was submitted to Rehabilitation in Neurological Conditions, a section of the journal Frontiers in Rehabilitation Sciences