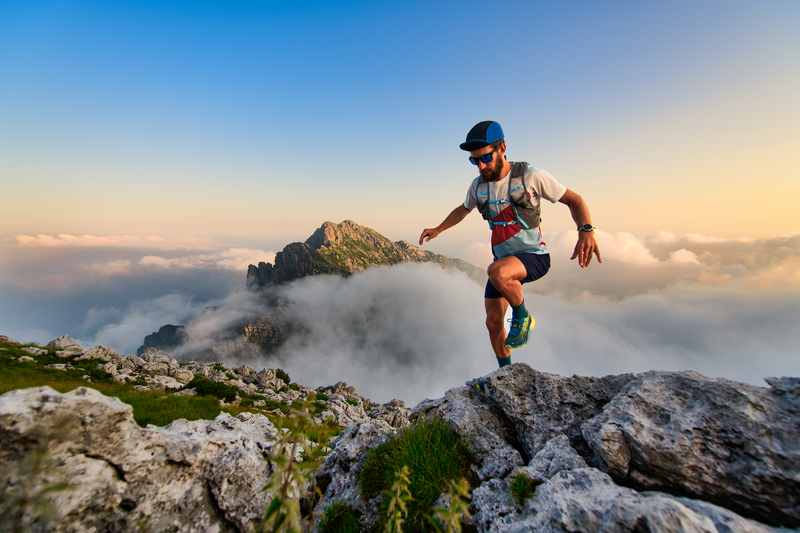
95% of researchers rate our articles as excellent or good
Learn more about the work of our research integrity team to safeguard the quality of each article we publish.
Find out more
SPECIALTY GRAND CHALLENGE article
Front. Carbon , 03 October 2022
Sec. Graphite-ene
Volume 1 - 2022 | https://doi.org/10.3389/frcrb.2022.1034557
Graphite is known and used by humankind since ancient times. It is composed of sp2-bonded, two-dimensional, atomically flat, carbon layers called graphene, weakly joined together by van der Waals forces. Through the ages, graphite found many applications, from a refractory material to electrode for batteries, from pencils to lubricants, among others.
In a pioneering calculation, Wallace obtained the electronic structure of graphite and graphene (Wallace, 1947) that revealed the linear dispersion when the bands cross the Fermi level at the vertices K and K’ of the hexagonal Brillouin zone, a feature that, many years later, became widely known as the perfect emulation of a two-dimensional massless Dirac fermion (Novoselov et al., 2005). For almost 60 years, this result appeared ellusive to experimental investigation, as the isolation of a single layer of graphene—the ultimate 2D system - was thought to be forbidden by the Mermin-Wagner theorem (Mermin, 1968).
But Nature has it own ways, and graphene research took a dramatic turn when Geim & Novoselov isolated graphene for the first time using the mechanical exfoliation (“scotch tape”) method (Novoselov et al., 2004). In 2010, they received the Physics Nobel Prize for this discovery and subsequent studies on this material, which led to a plethora of observations of the truly exquisite properties of 2D massless Dirac fermions to be accessible in tabletop experiments (Geim and Novoselov, 2007).
The discovery of graphene ignited the broader field of 2D materials (Novoselov et al., 2016). Novel layered materials are discovered or predicted in a daily basis, with diverse properties (metals, insulators, semiconductors, magnetic, topological, etc). In addition, the possibility of combining these materials in stacked structures called “van der Waals heterostructures” offers endless possibilities of design engineering of novel structures to meet target functionalities.
These already infinite possibilities can be yet infinitely multiplied by turning the knob that controls the twist angle between neighboring layers. From early theoretical predictions that the electronic structure of bilayer graphene could be tuned by the twist angle (Lopes dos Santos et al., 2007), this possibility evolved to a truly new subfield of graphene and 2D materials research called “twistronics”. A turning point was the discovery of superconductivity in twisted bilayer graphene near the so-called “magical angle” (Cao et al., 2018; Lee et al., 2019; Yankowitz et al., 2019), where bands near the Fermi level become flat and give rise to a variety of strongly-correlated phenomena. This discovery sparkled verifications of such effects in similar and promising systems, such as trilayer graphene (Park et al., 2021; Hao et al., 2021). Today, twistronics is a hot topic not only in graphene but in 2D materials research in general. Although the superconductivity in twisted graphene systems appears to be of unconventional type, the underlying theoretical description is still ellusive, representing an important challenge in graphene physics. The possibility to explore the twist degree of freedom has been extended to other 2D materials and combinations thereof (Devakul et al., 2021). The immense number of possibilities of different structures—combining different materials and twist angles - offers yet another challenge: to predict and design structures for target electronic or optical properties, a daunting task that certainly must be dealt with using artificial intelligence methods (Tritsaris et al., 2021).
The discovery of new phenomena and the use of single and multilayer graphene for selected applications require steady advances in graphene synthesis. From the early works on mechanically exfoliated graphene (Novoselov et al., 2004), synthesis has evolved considerably. Several methods currently exist to synthesize graphene, each having its own advantages and difficulties (Shams et al., 2015; Zhu et al., 2010). Graphene can be synthesized by top-down approaches such as graphite intercalation, pyrolysis, reduction of graphene oxide, electrochemical exfoliation, sonication, etc., as well as bottom-up approaches such as chemical vapor deposition (CVD), epitaxial growth on SiC, and others. Considerable advances have been achieved in CVD growth of large-area monolayer and bilayer graphene (Wang et al., 2021). Novel bottom-up approaches involving precursor molecules were very sucessful in the bottom-up synthesis of atomically-precise graphene nanoribbons and related structures (Cai et al., 2010; Ruffieux et al., 2016), as well as large area graphene sheets synthesized at liquid-liquid interfaces (Lopes et al., 2018). For industrial-scale graphene synthesis, challenges include improve quality control, uniformity and reproducibility of graphene flakes and, in the case of CVD graphene, increasing production rate and scalability. In general, inconsistent quality over different producers is also an issue, and the need to improve an unified standardization or grading scheme is critical (Lin et al., 2019).
Raman spectroscopy holds a special place among graphite and graphene characterization techniques (Jorio et al., 2011). It can be used to determine the number of layers in a multilayer sample (Silva et al., 2020), it is quantitatively sensitive to the presence of both linear and point defects (Cançado et al., 2017), doping (Ferrari, 2007) and pressure (Machon et al., 2018). Recent developments on tip-enhanced Raman spectroscopy (TERS) of graphene (Gadelha et al., 2021) have extended the spatial resolution of Raman measurements to unprecedented regimes, alllowing for measurements of localized phonon modes in twisted bilayer graphene. One challenge in Raman spectroscopy of graphene is to improve even more spatial resolution to measure Raman signals of single defects in graphene in the near future.
The unusual electronic dispersion of graphene gives rise to very attractive optical and plasmonic properties (Grigorenko et al., 2012; Cui et al., 2021), with many possible applications in optoelectronic devices. Plasmons-polaritons in graphene—coupled excitations of photons and electrons—inherit the behavior of 2D massless fermions and can be gate-controlled and imaged (Fei et al., 2012; Chen et al., 2012), enabling the possibility of novel and compact optical devices operating from terahertz to visible frequencies, such as metamaterial and transformation optics devices (Zhao et al., 2016; Vakil and Engheta, 2011; Ju et al., 2011; Lee et al., 2012), photodetectors (Liu et al., 2011); photonic crystals (Xiong et al., 2019), lasers (Chakraborty et al., 2016) and X-ray sources (Wong et al., 2015). Challenges in plasmonic and optical properties of graphene involve improving the quality and reproducibility of patterned nanostructures, efficient coupling light in and out of graphene, and extending plasmon tunability to the vis-NIR range (García de Abajo, 2014).
From the early days of graphene research, prospects applications of graphene in electronics and spintronics were conceived. Graphene itself does not have a band gap, limiting the possibilities of applications in digital electronics. However, several other applications have been explored, from flexible displays (Ahn and Hong, 2014) to radio-frequency devices (Palacios et al., 2010). In addition, the discovery of graphene opened the door to other 2D materials, many of them semiconductors and holding a greater potential for applications in electronics. Furthermore, from the understanding and control of spin injection, transport and relaxation in graphene, several spintronics applications have been conceived (Han et al., 2014). Challenges in graphene electronic and spintronic applications rely in devising controlled fabrication protocols that lead to stable and reproducible devices. Scalabality and wafer-scale integration are of course important issues as well.
The number of graphene applications is growing steadily every year. Graphene’s planar geometry and its sensitivity to the surrounding molecular enviroment make it an ideal material for electrochemical sensors and biosensors (Shao et al., 2010). Graphene and graphene oxide are promising materials for biomedical applications such as drug delivery, biosensing, bioimaging, cancer therapy and theranostics [Shi and Fang, 2018; Song et al., 2020). Applications related to graphene’s outstanding mechanical and conductive properties are also promising, such as anti-corrosive coatings (Cui et al., 2019) and various types of composites (with polymers, metals, oxides, and others) (Huang et al., 2012). Graphene and its composites can be used in a variety of applications, such as fuel cells (Liu et al., 2014), Li-ion batteries (Cai et al., 2017), supercapacitors (Velasco et al., 2021), photocatalysis (Li et al., 2016) and photovoltaic devices (Liu Z. et al., 2015). Graphene-based membranes appear to be a great platform for molecular separation and filtration as well (Liu G. et al., 2015). Challenges in the field of graphene applications involve overcoming the bottlenecks of production cost and volume, in comparison to competing technologies. In addition, to substantially boost graphene penetration in industry, graphene needs to find its “killer” applications, those in which the contributions of graphene are irreplaceable and unique (Lin et al., 2019).
All these applications require careful studies of possible health and environmental impacts of graphene and its derivatives. Whenever possible, such studies must be incorporated in the very early stages of product and process development (“safety-by-design”). Fortunately, from the whole plethora of nanomaterials, graphene-related materials are one of the most well studied in this aspect (Ding et al., 2022; Fadeel et al., 2018; Bortolozzo et al., 2021). Challenges in this field are further understanding the structure-activity relationships of graphene materials in health and environment and devising novel methods of mitigation of possible hazardous effects.
In summary, almost 20 years of after its discovery, the study of graphene and graphene-related materials (including graphite, of course) remains a hot topic from both fundamental and applied science. As such, the Graphite-ene Section of Frontiers in Carbon will be devoted to bring to their readers the latest discoveries in all aspects involving this superlative material.
All authors listed have made a substantial, direct, and intellectual contribution to the work and approved it for publication.
I thank financial support from Brazilian agencies CNPq, FAPERJ, FAPESP, and INCT—Carbon Nanomaterials.
The author declares that the research was conducted in the absence of any commercial or financial relationships that could be construed as a potential conflict of interest.
All claims expressed in this article are solely those of the authors and do not necessarily represent those of their affiliated organizations, or those of the publisher, the editors and the reviewers. Any product that may be evaluated in this article, or claim that may be made by its manufacturer, is not guaranteed or endorsed by the publisher.
Ahn, J.-H., and Hong, B. H. (2014). Graphene for displays that bend. Nat. Nanotechnol. 9, 737–738. doi:10.1038/nnano.2014.226
Bortolozzo, L. S., Côa, F., Khan, L. U., Medeiros, A. M. Z., da Silva, G. H., Delite, F. S., et al. (2021). Mitigation of graphene oxide toxicity in C. elegans after chemical degradation with sodium hypochlorite. Chemosphere 278, 130421. doi:10.1016/j.chemosphere.2021.130421
Cai, J., Ruffieux, P., Jaafar, R., Bieri, M., Braun, T., Blankenburg, S., et al. (2010). Atomically precise bottom-up fabrication of graphene nanoribbons. Nature 466, 470–473. doi:10.1038/nature09211
Cai, X., Lai, L., Shen, Z., and Lin, J. (2017). Graphene and graphene-based composites as Li-ion battery electrode materials and their application in full cells. J. Mat. Chem. A 5, 15423–15446. doi:10.1039/C7TA04354F
Cao, Y., Fatemi, V., Fang, S., Watanabe, K., Taniguchi, T., Kaxiras, E., et al. (2018). Unconventional superconductivity in magic-angle graphene superlattices. Nature 556, 43–50. doi:10.1038/nature26160
Chakraborty, S., Marshall, O. P., Folland, T. G., Kim, Y. J., Grigorenko, A. N., and Novoselov, K. S. (2016). Gain modulation by graphene plasmons in aperiodic lattice lasers. Science 351, 246–248. doi:10.1126/science.aad2930
Chen, J., Badioli, M., Alonso-González, P., Thongrattanasiri, S., Huth, F., Osmond, J., et al. (2012). Optical nano-imaging of gate-tunable graphene plasmons. Nature 487, 77–81. doi:10.1038/nature11254
Cui, G., Bi, Z., Zhang, R., Liu, J., Yu, X., and Li, Z. (2019). A comprehensive review on graphene-based anti-corrosive coatings. Chem. Eng. J. 373, 104–121. doi:10.1016/j.cej.2019.05.034
Cui, L., Wang, J., and Sun, M. (2021). Graphene plasmon for optoelectronics. Rev. Phys. 6, 100054. doi:10.1016/j.revip.2021.100054
Devakul, T., Crépel, V., Zhang, Y., and Fu, L. (2021). Magic in twisted transition metal dichalcogenide bilayers. Nat. Commun. 12, 6730. doi:10.1038/s41467-021-27042-9
Ding, X., Pu, Y., Tang, M., and Zhang, T. (2022). Environmental and health effects of graphene-family nanomaterials: Potential release pathways, transformation, environmental fate and health risks. Nano Today 42, 101379. doi:10.1016/j.nantod.2022.101379
Fadeel, B., Bussy, C., Merino, S., Vázquez, E., Flahaut, E., Mouchet, F., et al. (2018). Safety assessment of graphene-based materials: Focus on human health and the environment. ACS Nano 12, 10582–10620. doi:10.1021/acsnano.8b04758
Fei, Z., Rodin, A. S., Andreev, G. O., Bao, W., McLeod, A. S., Wagner, M., et al. (2012). Gate-tuning of graphene plasmons revealed by infrared nano-imaging. Nature 487, 82–85. doi:10.1038/nature11253
Ferrari, A. C. (2007). Raman spectroscopy of graphene and graphite: Disorder, electron–phonon coupling, doping and nonadiabatic effects. Solid State Commun. 143, 47–57. doi:10.1016/j.ssc.2007.03.052
Gadelha, A. C., Ohlberg, D. A. A., Rabelo, C., Neto, E. G. S., Vasconcelos, T. L., Campos, J. L., et al. (2021). Localization of lattice dynamics in low-angle twisted bilayer graphene. Nature 590, 405–409. doi:10.1038/s41586-021-03252-5
García de Abajo, F. J. (2014). Graphene plasmonics: Challenges and opportunities. ACS Photonics 1, 135–152. doi:10.1021/ph400147y
Geim, A. K., and Novoselov, K. S. (2007). The rise of graphene. Nat. Mater. 6, 183–191. doi:10.1038/nmat1849
Grigorenko, A. N., Polini, M., and Novoselov, K. S. (2012). Graphene plasmonics. Nat. Photonics 6, 749–758. doi:10.1038/nphoton.2012.262
Gustavo Cançado, L., Gomes da Silva, M., Martins Ferreira, E. H., Hof, F., Kampioti, K., Huang, K., et al. (2017). Disentangling contributions of point and line defects in the Raman spectra of graphene-related materials. 2D Mat. 4, 025039. doi:10.1088/2053-1583/aa5e77
Han, W., Kawakami, R. K., Gmitra, M., and Fabian, J. (2014). Graphene spintronics. Nat. Nanotechnol. 9, 794–807. doi:10.1038/nnano.2014.214
Hao, Z., Zimmerman, A. M., Ledwith, P., Khalaf, E., Najafabadi, D. H., Watanabe, K., et al. (2021). Electric field–tunable superconductivity in alternating-twist magic-angle trilayer graphene. Science 371, 1133–1138. doi:10.1126/science.abg0399
Huang, X., Qi, X., Boey, F., and Zhang, H. (2012). Graphene-based composites. Chem. Soc. Rev. 41, 666–686. doi:10.1039/C1CS15078B
Jorio, A., Dresselhaus, M. S., Saito, R., and Dresselhaus, G. (2011). Raman spectroscopy in graphene related systems. Weinheim, Germany: Wiley VCH.
Ju, L., Geng, B., Horng, J., Girit, C., Martin, M., Hao, Z., et al. (2011). Graphene plasmonics for tunable terahertz metamaterials. Nat. Nanotechnol. 6, 630–634. doi:10.1038/nnano.2011.146
Lee, J.-Y., Khalaf, E., Liu, S., Liu, X., Hao, Z., Kim, P., et al. (2019). Theory of correlated insulating behaviour and spin-triplet superconductivity in twisted double bilayer graphene. Nat. Commun. 10, 5333. doi:10.1038/s41467-019-12981-1
Lee, S. H., Choi, M., Kim, T. T., Lee, S., Liu, M., Yin, X., et al. (2012). Switching terahertz waves with gate-controlled active graphene metamaterials. Nat. Mater. 11, 936–941. doi:10.1038/nmat3433
Li, X., Yu, J., Wageh, S., Al-Ghamdi, A. A., and Xie, J. (2016). Graphene in photocatalysis: A review. Small 12, 6640–6696. doi:10.1002/smll.201600382
Lin, L., Peng, H., and Liu, Z. (2019). Synthesis challenges for graphene industry. Nat. Mater. 18, 520–524. doi:10.1038/s41563-019-0341-4
Liu, G., Jin, W., and Xu, N. (2015). Graphene-based membranes. Chem. Soc. Rev. 44, 5016–5030. doi:10.1039/C4CS00423J
Liu, M., Zhang, R., and Chen, W. (2014). Graphene-supported nanoelectrocatalysts for fuel cells: Synthesis, properties, and applications. Chem. Rev. 114, 5117–5160. doi:10.1021/cr400523y
Liu, Y., Cheng, R., Liao, L., Zhou, H., Bai, J., Liu, G., et al. (2011). Plasmon resonance enhanced multicolour photodetection by graphene. Nat. Commun. 2, 579. doi:10.1038/ncomms1589
Liu, Z., Lau, S.-P., and Yan, F. (2015). Functionalized graphene and other two-dimensional materials for photovoltaic devices: Device design and processing. Chem. Soc. Rev. 44, 5638–5679. doi:10.1039/C4CS00455H
Lopes dos Santos, J. M. B., Peres, N. M. R., and Castro Neto, A. H. (2007). Graphene bilayer with a twist: Electronic structure. Phys. Rev. Lett. 99, 256802. doi:10.1103/PhysRevLett.99.256802
Lopes, L. C., da Silva, L. C., Vaz, B. G., Oliveira, A. R. M., Oliveira, M. M., Rocco, M. L. M., et al. (2018). Facile room temperature synthesis of large graphene sheets from simple molecules. Chem. Sci. 9, 7297–7303. doi:10.1039/C8SC02818D
Machon, D., Bousige, C., Alencar, R., Torres-Dias, A., Balima, F., Nicolle, J., et al. (2018). Raman scattering studies of graphene under high pressure. J. Raman Spectrosc. 49, 121–129. doi:10.1002/jrs.5284
Mermin, N. D. (1968). Crystalline order in two dimensions. Phys. Rev. 176, 250–254. doi:10.1103/PhysRev.176.250
Novoselov, K. S., Geim, A. K., Morozov, S. V., Jiang, D., Katsnelson, M. I., Grigorieva, I. V., et al. (2005). Two-dimensional gas of massless Dirac fermions in graphene. Nature 438, 197–200. doi:10.1038/nature04233
Novoselov, K. S., Geim, A. K., Morozov, S. V., Jiang, D., Zhang, Y., Dubonos, S. V., et al. (2004). Electric field effect in atomically thin carbon films. Science 306, 666–669. doi:10.1126/science.1102896
Novoselov, K. S., Mishchenko, A., Carvalho, A., Castro Neto, A. H., Mishchenko, A., and CarvAlho, A. (2016). 2D materials and van der Waals heterostructures. Science 353, aac9439. doi:10.1126/science.aac9439
Palacios, T., Hsu, A., and Wang, H. (2010). Applications of graphene devices in RF communications. IEEE Commun. Mag. 48, 122–128. doi:10.1109/MCOM.2010.5473873
Park, J. M., Cao, Y., Watanabe, K., Taniguchi, T., and Jarillo-Herrero, P. (2021). Tunable strongly coupled superconductivity in magic-angle twisted trilayer graphene. Nature 590, 249–255. doi:10.1038/s41586-021-03192-0
Ruffieux, P., Wang, S., Yang, B., Sánchez-Sánchez, C., Liu, J., Dienel, T., et al. (2016). On-surface synthesis of graphene nanoribbons with zigzag edge topology. Nature 531, 489–492. doi:10.1038/nature17151
Shams, S. S., Zhang, R., and Zhu, J. (2015). Graphene synthesis: A review. Mater. Pol. 33, 566–578. doi:10.1515/msp-2015-0079
Shao, Y., Wang, J., Wu, H., Liu, J., Aksay, I. A., and Lin, Y. (2010). Graphene based electrochemical sensors and biosensors: A review. Electroanalysis 22, 1027–1036. doi:10.1002/elan.200900571
Shi, J., and Fang, Y. (2018). “Biomedical applications of graphene,” in Graphene. Editors H. Zhu, Z. Xu, D. Xie, and Y. Fang (Academic Press), 215–232. doi:10.1016/B978-0-12-812651-6.00009-4
Silva, D. L., Campos, J. L. E., Fernandes, T. F. D., Rocha, J. N., Machado, L. R. P., Soares, E. M., et al. (2020). Raman spectroscopy analysis of number of layers in mass-produced graphene flakes. Carbon 161, 181–189. doi:10.1016/j.carbon.2020.01.050
Song, S., Shen, H., Wang, Y., Chu, X., Xie, J., Zhou, N., et al. (2020). Biomedical application of graphene: From drug delivery, tumor therapy, to theranostics. Colloids Surf. B: Biointerfaces 185, 110596. doi:10.1016/j.colsurfb.2019.110596
Tritsaris, G. A., Carr, S., and Schleder, G. R. (2021). Computational design of moiré assemblies aided by artificial intelligence. Appl. Phys. Rev. 8, 031401. doi:10.1063/5.0044511
Vakil, A., and Engheta, N. (2011). Transformation optics using graphene. Science 332, 1291–1294. doi:10.1126/science.1202691
Velasco, A., Ryu, Y. K., Boscá, A., Ladrón-de-Guevara, A., Hunt, E., Zuo, J., et al. (2021). Recent trends in graphene supercapacitors: From large area to microsupercapacitors. Sustain. Energy Fuels 5, 1235–1254. doi:10.1039/d0se01849j
Wallace, P. R. (1947). The band theory of graphite. Phys. Rev. 71, 622–634. doi:10.1103/PhysRev.71.622
Wang, M., Luo, D., Wang, B., and Ruoff, R. S. (2021). Synthesis of large-area single-crystal graphene. Trends Chem. 3, 15–33. doi:10.1016/j.trechm.2020.10.009
Wong, L. J., Kaminer, I., Ilic, O., Joannopoulos, J. D., and Soljačić, Marin (2015). Towards graphene plasmon-based free-electron infrared to X-ray sources. Nat. Photonics 10, 46–52. doi:10.1038/nphoton.2015.223
Xiong, L., Forsythe, C., Jung, M., McLeod, A. S., Sunku, S. S., Shao, Y. M., et al. (2019). Photonic crystal for graphene plasmons. Nat. Commun. 10, 4780. doi:10.1038/s41467-019-12778-2
Yankowitz, M., Chen, S., Polshyn, H., Zhang, Y., Watanabe, K., Taniguchi, T., et al. (2019). Tuning superconductivity in twisted bilayer graphene. Science 363, 1059–1064. doi:10.1126/science.aav1910
Zhao, X., Yuan, C., Zhu, L., and Yao, J. (2016). Graphene-based tunable terahertz plasmon-induced transparency metamaterial. Nanoscale 8, 15273–15280. doi:10.1039/c5nr07114c
Keywords: graphene, graphite, 2D material, twistronics, plasmons
Citation: Capaz RB (2022) Grand challenges in graphene and graphite research. Front. Carbon 1:1034557. doi: 10.3389/frcrb.2022.1034557
Received: 01 September 2022; Accepted: 13 September 2022;
Published: 03 October 2022.
Edited and reviewed by:
Antonio G. Souza Filho, Universidade Federal do Ceará, BrazilCopyright © 2022 Capaz. This is an open-access article distributed under the terms of the Creative Commons Attribution License (CC BY). The use, distribution or reproduction in other forums is permitted, provided the original author(s) and the copyright owner(s) are credited and that the original publication in this journal is cited, in accordance with accepted academic practice. No use, distribution or reproduction is permitted which does not comply with these terms.
*Correspondence: Rodrigo B. Capaz, cm9kcmlnby5jYXBhekBsbm5hbm8uY25wZW0uYnI=
Disclaimer: All claims expressed in this article are solely those of the authors and do not necessarily represent those of their affiliated organizations, or those of the publisher, the editors and the reviewers. Any product that may be evaluated in this article or claim that may be made by its manufacturer is not guaranteed or endorsed by the publisher.
Research integrity at Frontiers
Learn more about the work of our research integrity team to safeguard the quality of each article we publish.