- 1Department of Electrical and Electronics Engineering, School of Engineering and Natural Sciences, Istanbul Medipol University, Istanbul, Türkiye
- 2Karel Electronics, Mobile Communication Technologies, Ankara, Türkiye
Public protection and disaster relief (PPDR) agencies rely on wireless communications to respond in the event of emergencies. Public safety networks (PSNs) provide the wireless network used by emergency services. PSN is used to support push-to-talk services with some data transmission by employing land mobile radios. However, PPDR agencies are increasingly relying on additional information such as videos that require higher bandwidths. Therefore, many countries are transitioning or integrating their public safety networks with advanced broadband wireless communication systems such as fourth-generation (4G) long-term evolution (LTE) and planning to evolve to fifth-generation (5G) new radio (NR) in the future. The paper investigates infrastructure sharing mechanisms and deployment strategies in the transition of PSNs to a 4G LTE network, including a roadmap for cost analysis. Additionally, the paper examines LTE-based PSN deployment scenarios in various countries and engages in a discussion of the advantages and disadvantages of different sharing mechanisms and coexistence schemes. Finally, the challenges within the Public Safety Broadband Network (PSBN) are addressed and potential future research directions in this domain are deliberated.
1 Introduction
First responders serve a crucial role in today’s society, especially in times of natural disasters and other emergencies by facilitating public facilities. The advancement of technology signals new threats or challenges to public safety, which eventually necessitates the development of better strategies to combat them. One such critical technology is the development of wireless systems for public protection and disaster relief (PPDR) agencies to ensure better communication (and coordination) between the different entities. Classically, these communication systems have relied on rate-restricted, narrowband services such as voice calling and short message service (SMS). However, with the ubiquity of video surveillance services and their potential in improving public safety, there is a pressing need for the development of broadband public safety networks (PSNs).
The transition of PSNs from narrowband to broadband, however, is extremely challenging and might require complete revamping of nationwide networks. The transition strategy for any country may vary depending on the population distribution, geographical maps, current network deployment status, available budget, commercial operation regulations, etc. (Ferrus and Sallent, 2015). The time and cost associated with this migration calls for the examination/comparison of the different possible approaches that can be utilized for infrastructure sharing and public–private partnerships utilized in various countries.
Within the academic literature, research that focuses on long-term evolution (LTE) for PSNs has been explored in various articles (Doumi et al., 2013; Ferrus et al., 2013b, 2013a; Favraud et al., 2016; Oueis et al., 2017). Doumi et al. (2013) have discussed the transition to broadband technologies to improve public safety, taking into account the existing LTE standards and identifying essential enhancements that are required to replace narrowband technologies. Similarly, Ferrus et al. (2013b) have examined the suitability of LTE and its related technologies to provide mobile broadband services for PPDR. They have suggested that the future scenarios for meeting the data-intensive demands of PPDR agencies will likely involve a combination of dedicated and commercial LTE-based mobile networks. The same authors in Ferrus et al. (2013a) have identified and discussed the primary techno-economic motivators, spanning technology, network, and spectrum aspects. Furthermore, Favraud et al. (2016) conducted a survey of potential public safety use cases along with the resulting network topologies that they bring about. It also addresses the present state of the 3rd Generation Partnership Project (3GPP, 2011) standards in this context and emphasizes the forthcoming challenges. These drivers are expected to serve as the groundwork for making future PPDR communications both efficient and economically viable. Nevertheless, these studies omit the exploration of LTE and PSN infrastructure-sharing mechanisms. Moreover, Oueis et al. (2017) have presented an account of the initial technical aspects of the isolated E-UTRAN operation for public safety (IOPS) feature, which was standardized in Release 13 in conjunction with LTE. Subsequently, based on its limitations, they deliberated on the potential directions for the advancement of IOPS in the future.
Furthermore, some articles have concentrated on conducting analyses related to spectrum sharing (Ferrus et al., 2012; Ferrus et al., 2012; Sohul et al., 2016; Yuksel et al., 2016) that provide a thorough perspective on the five potential spectrum sharing models, all of which contribute to enhancing capacity during emergency situations and improving PPDR communications. Yuksel et al. (2016) examined the potential of public safety (PS) spectrum for PS communication applications and identified the three essential characteristics that contribute to successful PS spectrum implementation: providers being motivated to share, sharing becoming the standard practice, and government authority being effectively employed to encourage increased sharing. Moreover, Sohul et al. (2016) have explored our concept of the adaptable, swiftly deployable, and adjustable PS system that is designed to fulfill immediate and future requirements for a crucial communication infrastructure. The article also delves into the benefits of spectrum sharing to support this overarching vision. Additionally, Chaudhry and Hafez (2019) analyzed land mobile radios (LMRs) and LTE technologies for PPDR networks within the 700 MHz spectrum. This review provides insights into the potential drawbacks of LTE for PSNs.
A comprehensive examination of the public safety LMRs and LTE systems has been provided in the PSN literature (Baldini et al., 2014; Kumbhar et al., 2017; Jarwan et al., 2019). In Kumbhar et al. (2017), a comparative analysis of the old and new technologies for PSN has been made, which also considers the current state of the PSNs in countries such as the United States, the United Kingdom, and Canada. In Baldini et al. (2014), the authors have dealt with the usability and flexibility of wireless communication technologies for PSNs in terms of technology, regulations, research activities, and standardization studies. In Jarwan et al. (2019), LTE-based PSNs have been examined from three aspects: first, legacy technologies, architecture, and spectrum allocation have been discussed. Second, the challenges and research problems of LTE-based PSNs have been identified. Finally, all of the studies so far have been comprehensively included.
Some studies have focused on the general case of infrastructure sharing without considering PSNs. Cano et al. (2020) have presented a comprehensive overview regarding performance assessment and resource management. Meddour et al. (2011) have examined network sharing and analyzed estimated savings regarding the techno-economic dimension, available technical solutions, feasibility, and constraints. Moreover, some technical papers have proposed methods for infrastructure sharing (Bousia et al., 2016; Cano et al., 2017). Cano et al. (2017) have presented optimal sharing strategies by mathematically considering the radio access network (RAN) infrastructure sharing from technical and economic perspectives. Their proposed method aimed to maximize the quality of service (QoS) and the profits of mobile network operators (MNOs). A roaming-based infrastructure sharing method that can be applied between multiple MNOs during low traffic periods was proposed by Bousia et al. (2016). The proposed method ensured that MNOs can make individually profitable closure decisions for their base stations (BSs).
Some papers have investigated infrastructure sharing for PSNs (Hallahan and Peha, 2011; Fantacci et al., 2016; Marabissi and Fantacci, 2017). Public–private infrastructure sharing was analyzed from two perspectives in Hallahan and Peha (2011). The initial one pertains to a profit-oriented private sector partnership, whereas the second involves a public sector partnership that allocates resources to the initiative contingent. In Fantacci et al. (2016), the methods for transitioning to an LTE-based PSN were proposed as dedicated, over the commercial operator, and hybrid. Moreover, a RAN sharing scenario was proposed that guaranteed PPDR requirements by reducing network deployment costs and time in Marabissi and Fantacci (2017). This method was based on the integration of RAN with a dedicated network. Moreover, Hallahan and Peha (2013) have quantified the benefits concerning capacity to demonstrate that by creating numerous agreements with commercial carriers in each region, public safety can access a substantial amount of capacity projected for severe emergencies without significantly undermining service quality for commercial users with challenges.
Additionally, the application of fifth generation (5G) new radio (NR) for PSNs has been explored in the literature (Pérez et al., 2020; Ali et al., 2021; Chochliouros et al., 2021; Suomalainen et al., 2021; Li et al., 2022). In Chochliouros et al. (2021), the authors have introduced a PS scenario with the primary aim of showcasing the utilization of a shared 5G infrastructure during emergencies that involves both first responders and civilians. Pérez et al. (2020) have assessed the current advancements and highlighted the key challenges in providing PSNs. They examined the integration of new 5G mobile networks for the dynamic deployment of virtualized emergency services within future mobile communications. Furthermore, Suomalainen et al. (2021) have investigated and conducted a survey concerning the security architecture and mechanisms that are intended to enhance prioritized PS communication within 5G networks. Moreover, Li et al. (2022) identified critical technical hurdles and elaborated on the evolution of 5G NR features aimed at fulfilling the emerging safety-critical prerequisites. These developments encompass achieving universal connectivity, facilitating effective group communications, offering precise positioning capabilities for first responders, and prioritizing mission-critical data traffic. Also, Ali et al. (2021) delved into the importance of internet of things (IoT) and 5G networks in advancing sustainability within urban environments, especially within the context of disaster management systems.
The mentioned studies lack a comprehensive tutorial on deploying PSNs and scenarios involving infrastructure sharing with MNOs. Additionally, these papers overlook the costs associated with site and infrastructure installations, which is critical in determining sharing mechanisms. Infrastructure sharing is primarily driven by the aim to reduce costs, and the absence of consideration for these costs leaves the selection of sharing mechanisms uncertain. Moreover, recent strategies adopted by different countries for migrating to PSNs are not considered case studies. As depicted in Table 1, existing PSN surveys and articles fail to provide a roadmap for cost analysis, transition strategies from LTE to 5G, and coexistence with vertical networks such as railways and marine networks. Our current work addresses these gaps by offering a comprehensive tutorial on PSN deployment, specifically emphasizing infrastructure sharing. We delve into all three PSN deployment scenarios, providing detailed insights. We also thoroughly examine infrastructure sharing variations, such as passive sharing, RAN sharing, and core network (CN) sharing. This analysis is coupled with a roadmap incorporating cost analysis derived from network dimensions like coverage and capacity for various strategies. Furthermore, we explore PSN deployment strategies implemented by different countries while investigating spectrum sharing scenarios based on existing LTE-based PSN networks in comparison to various nations.
The remaining paper is organized as follows: Section 2 examines LTE-based PSN deployment options for the dedicated, hybrid, and commercial networks. Section 3 examines infrastructure sharing mechanisms. A roadmap to cost analysis, capacity, and coverage dimensioning approaches are introduced in Section 4. Section 5 discusses the deployment plans of countries for broadband PSNs. Section 6 explains the possible challenges and solutions for the coexistence between different networks. Section 7 outlines the challenges and potential future directions concerning LTE-based PSNs. Finally, Section 8 concludes the paper.
2 Network deployment
This section describes different broadband PSN deployment models and CN deployment options. First, ownership and operation models are explained, followed by spectrum usage (low/high and dedicated/shared). After that, operational expenditures (OPEX) and capital expenditures (CAPEX) are discussed.
Network deployment and operation may be undertaken by a government, any MNO/contractor, or a combination of both. As such, any of the following options might be utilized:
• Government-owned and government-operated (GO-GO),
• Government-owned and contractor-operated (GO-CO),
• Contractor-owned and contractor-operated (CO-CO).
In the GO-GO and GO-CO scenarios, the resources are exclusive to the PSN agencies, and it is considered a dedicated network. In CO-CO scenarios, however, the sharing options are much more diverse, depending on the contracts signed between the PSN customers, government, and contractor. Some European countries are already using these options for deploying a PSN. According to Ferrus and Sallent (2015), Belgium's ASTRID and Finland's VIRVE are examples of GO-GO PSN arrangements; Germany's BDBOS and Austria's BOS are examples of GO-CO PSN arrangements; and the United Kingdom's Airwave and Denmark's SINE are examples of CO-CO PSN arrangements. Some of these examples are discussed in detail in Section 5. To guarantee efficiency and effectiveness, the PSNs depend heavily on the deployment criteria and associated business models (government and public safety organization demands). Therefore, implementation alternatives must be considered in addition to many deployment approaches. Experts divide the potential models into three (Fantacci et al., 2016):
• Dedicated network: New private mobile broadband network for PPDR services.
• Commercial network: Use of PPDR services over the commercial network based on specific contracts.
• Hybrid network: A hybrid solution combining commercial and private network infrastructure.
For deploying a new PPDR network, spectrum availability is one of the main concerns (Doumi et al., 2013). The coverage and network deployment costs depend on operational frequency bands. PSNs generally use lower frequencies as they provide more coverage. Thus, these frequencies require fewer base stations and simplify network planning. By contrast, higher frequencies have much less coverage and require more base stations. Therefore, the expenses can significantly increase on the basis of the determined frequency. In addition to these considerations, the PSN may have its dedicated spectrum, or the operator may provide spectrum resources (no dedicated spectrum) in a partnership scenario. It is also feasible to choose a middle-ground solution. For example, the PSN can allocate some of its spectrum to PPDR while sharing the rest of the spectrum with MNOs. In this way, as seen in the United States, MNOs can provide infrastructure services to PSNs in exchange of spectrum (Kumbhar et al., 2017). Contrary to the United States example, as seen in the United Kingdom, MNOs can allocate some of their spectrum to the PSN for a fee (Ferrus and Sallent, 2015).
2.1 Dedicated network
Dedicated networks refer to privately deployed networks for public safety communication. Private deployment allows the network to be designed and configured for the PSN requirements. The analysis of national historical use-cases reveals that the most common approach used by PPDR agencies in the legacy systems is to own and run their network [such as terrestrial trunked radio (TETRA) and Project 25 (P25)] (PPDR-TC, 2013).
Broadband PSNs require flexibility, reliability, durability, and effective coverage and must also be resistant to malfunctions and outages (Favraud et al., 2016). A dedicated PSN can be a perfect solution for the user as the network can meet all user requirements and is also fully controllable. On the contrary, from the contractor’s point of view, this deployment can be costly and challenging (Fantacci et al., 2016). Table 2 summarizes the key characteristics of the LTE network's design for PPDR users (Ferrus and Sallent, 2015). In addition, one of the main concerns in deploying a dedicated network for PSNs and commercial networks is the determination of frequency bands. PSNs desire frequencies from the 700 MHz band of the operator spectrum for optimum capacity and coverage-related features (Ferrus and Sallent, 2015).
The expenditures for wireless network deployment can be classified into two: CAPEX and OPEX. CAPEX mainly refers to the initial investment in physical infrastructure. OPEX corresponds to the funds required to operate the network properly. Deploying a new broadband-dedicated PSN infrastructure can take time and require excessive investment. According to Ferrus and Sallent (2015), RAN deployment constitutes up to 70% of the total network deployment cost. There are some cost-saving infrastructure-sharing methods to cope with the high CAPEX and OPEX of deploying dedicated networks. These methods are explained in detail in Section 3. As mentioned earlier, PSNs require full coverage across the country. Therefore, additional site acquisition is required in rural areas. As a result, site installation cost reduces by about 60% by sharing existing infrastructure (Seybold, 2010). In case of emergency, the transportable/fast deployable equipment can handle this requirement. Such equipment are called cells on wheels (COWs) and cells on light trucks (COLTs) and are quickly deployable in cells that can be easily transported to PPDR services as required. In addition, with the developing technology, drones can be used as non-terrestrial networks (NTN) for PSNs. This approach has reduced network installation costs by about 30% (Seybold, 2010).
As seen in Figure 1, there are two possible deployment scenarios for network infrastructure dedicated to PSNs. The first is a mobile broadband network planned, constructed, operated, and owned by the authority as a GO-GO model. In the second, mobile broadband service is provided by MNOs through service offerings as a GO-CO model. In government-owned network deployment options, the government owns the equipment and infrastructure and controls the network process. On top of this, when requirements and conditions change, the network can quickly adapt to these new requirements. In dedicated network deployment, operations can be carried out by the government (GO-GO) or more capable and professional organizations (GO-CO). In the contractor-operated network option, the network is operated specifically by MNOs for mission-critical services.
2.2 Commercial networks
Commercial networks refer to PSN clients using their public safety communications over commercial operators’ networks via public safety mobile virtual network operators (MVNOs) (Jarwan et al., 2019). While the option to use the PSN over commercial networks is a fast and low-cost solution, specific issues have to be considered when it comes to QoS and mission-critical applications (Ferrus et al., 2013b).
Mission-critical communications have different requirements from commercial communications, particularly in terms of security, reliability, flexibility, and application. In terms of security, it will be difficult to guarantee security requirements when PSN services are used over commercial networks because the security metrics of commercial networks may not be configured to meet the security requirements of critical applications. Moreover, PSNs require high reliability and flexibility. Commercial networks must provide assurance and maintain a backup so that mission-critical communications can be operational when their network entities are damaged. Therefore, commercial operators must perform site hardening per requirements for this assurance [National Public Safety Telecommunications Council (NPSTC), 2014]. However, a compromise must be achieved between the necessary and desired site hardening. Site hardening and sheltering across all locations and infrastructure will be both financially and timely expensive which may cause delays in initial service launch or limited coverage (NPSTC, 2012b).
PSN users use some applications that are not available in commercial network systems, such as group calls and device-to-device (D2D) communication. Direct communication between mobile devices when network coverage is not available is one of the key features of the TETRA system. Proximity-based services (ProSe) refer to the LTE version of this feature and are standardized by 3GPP as D2D technology (3GPP, Dec. 2022d). In commercial LTE networks, the communication between two user equipment (UE) is established over the Enhanced UMTS Terrestrial Radio Access Network (E-UTRAN) and LTE core. With the ProSe feature, devices can communicate in two additional ways.
• Direct communication: UE can communicate directly among themselves.
• Locally routed communication: UE can only communicate over E-UTRAN without including LTE network in the communication.
This feature is required for LTE to be a public safety system technology. In addition, it can contribute significantly to reducing the load of the network, and also allows communication outside the normal coverage area (Ferrus and Sallent, 2015).
Additionally, in critical and emergency situations, commercial networks can become overloaded and congested, causing some applications to be out of service. For this reason, it is necessary to prioritize and allocate resources when making a service-level agreement (SLA) with commercial operators. Although there are some standardized regulatory rules and services for prioritization by 3GPP, the ability to ensure that critical connections are established consistently is imperative for mission-critical communications. It is possible to enable prioritization for access to LTE network using the multimedia priority service (MPS) application which ensures that high priority sessions are delivered and completed. An access with a range of priority levels is given to the authorized MPS subscribers. The QoS characteristics of the evolved packet system (EPS) carriers that administer the required preferred treatment are then selected using these MPS levels. According to the network operator policy, the policy and charging rules function (PCRF) entity decides which QoS parameters should be configured, such as the guaranteed bitrate and QoS class identifier for the EPS carrier. A logical function entity known as RAN Congestion Awareness Function (RCAF) gathers this information. It then reports to the PCRF as RAN user plane Congestion Status (RCS) (3GPP, Dec. 2014a). In addition to the QoS settings, the MPS priority level is used to provide the MPS subscriber with special access categories used in the evolved packet core (EPC). This method prevents overcrowding of radio interface control channels by limiting the access attempts by certain users (3GPP, Dec. 2021a; Ferrus et al., 2013b). Moreover, an adaptive predetermined dedicated capacity in a shared commercial network gives the required spectrum to PSNs during crises. In this way, it increases the spectrum efficiency by allowing the commercial use of the spectrum when it is not required by the PSN (Borkar et al., 2011).
Table 3 summarizes the objective differences between commercial network models and PPDR network models (Ferrus and Sallent, 2015). When deploying and operating mobile networks, MNOs seek to maximize their profits, hence their coverage is determined by population density. On the contrary, PPDR networks are established to protect the state, life, and property. Therefore, regardless of the income, PPDR networks require full coverage (including indoors, forests, and mountains). Another difference is that the capacity of commercial networks is determined by the busy hour of a regular day, and an outage is possible but undesirable due to the customer and revenue loss. On the contrary, the worst case scenarios (such as earthquake and national threat) determine the capacity of the PSNs and outages are unacceptable as they may cause life loss or threats. Therefore, most of the capacity is often unused, and resources are wasted. It should be noted that the capacity sharing of the private–public safety network approach can provide 15% of reduction of the costs (Seybold, 2010). Network resources can be allocated to other users by giving PSN user prioritization. Also, while commercial network communication is one to one, PPDR communication is dynamic and one to many. Commercial network data traffic is essentially a compromise of internet access corresponding to downloads. On the other hand, the data traffic of PPDR networks remains mainly within the PSN agencies, which means that the upload is more than the download. Therefore, network planning of PPDR networks differs from that of commercial networks. Also, subscriber information is confidential in PSNs, and the agencies have user information. The PSNs store this information, and authentication is done at the user level. In commercial networks, prioritization is minimal and may vary with the subscription level or application. By contrast, this prioritization is critical in PPDR services and changes dynamically based on the role and event level. Also, commercial networks’ preferred charging methods are per minute for voice, per GB for data, and per message for SMS. The PPDR charging methods can be quarterly or annual subscriptions without measuring usage.
From a deployment perspective, there are two possible deployment scenarios for PPDR services over commercial networks. The same mobile broadband services can be offered to PSN users as public customers or according to the specific requirements of PSN users. As mentioned previously, the first option should be avoided as users of PSN have different requirements than commercial users, such as security, and must be prioritized. If the PSN operator chooses the second option, then SLAs should be made on the specified aspects to meet the requirements of the PSN users.
2.3 Hybrid solution
A combination of dedicated and commercial approaches is called a hybrid solution. PPDR clients use dedicated PSNs for mission-critical applications and commercial LTE networks for the rest of the applications. According to the previous sections, deploying a dedicated PSN will be time-consuming and costly, especially in the beginning. On the other hand, commercial networks may struggle to meet PPDR service requirements. Therefore, using PSN services over commercial networks can be hazardous from the points of security, resilience, coverage, and mission-critical service requirements (Fantacci et al., 2016). For these reasons, there is a trend toward implementing a hybrid solution to deploy PPDR networks. Rather than just using dedicated resources, the appropriate hybrid approach based on infrastructure sharing can reduce the disadvantages while preserving the advantages of the other two approaches. Additionally, hybrid systems provide greater flexibility regarding spectrum utilization, service administration, radio access regulations, and area coverage.
As shown in Figure 1, the hybrid approach can be implemented in four different ways. First, MNOs and PPDR authorities may operate their services in different regions. Second, PPDR and public users can share RAN. Third, in addition to the second option, PPDR users have dedicated RAN, where PSN is operated as the MVNO. Fourth, PSN has a dedicated CN in addition to the dedicated RAN so that PSN is operated as an extended MVNO. These options can also be seen as stages from the commercial model to the dedicated PSN model. The example of Finland as a case study for this approach is discussed in Section 5.5.
2.4 Core network deployments
The core network elements of LTE include mobility management entity (MME), serving gateway (S-GW), packet data network gateway (P-GW), home subscriber server (HSS), and PCRF. The MME provides mobility and session management by acting as a control node between the UE and BS. The S-GW provides routing traffic between the LTE RAN and P-GW. The P-GW routes traffic between the S-GW and other networks such as the internet. The HSS stores client data and sends authentication messages to the MME. The PCRF is responsible for measuring mobile data consumption and providing QoS information to the P-GW (Jarwan et al., 2019).
Cellular CNs have a partially distributed and partially centralized architecture. Possibly, the distributed architecture may be sufficient to meet the rapidly increasing traffic requirements and reduce the cost of CN installation (Chan et al., 2011). Because the distributed network architecture avoids redundant routes in the traffic flow. It can more easily scale increasing numbers of users while allowing for dynamic mobility management that directs traffic when a user requests support. Dynamic mobility management increases network scalability and avoids signaling overhead and unnecessary use of resources. For an LTE network, there are four different network distribution schemes investigated in the literature.
• Centralized control plane (MME) and centralized data plane (S-GW and P-GW): In this deployment scenario, the network is simpler to maintain and operate than in the other scenarios. Client systems, users, and servers can be easily added or removed. On the other hand, in network failures, having a single main server causes the entire system to crash (single point of failure). In addition, collecting all user information on a single main server creates a security risk in case of a possible attack, and attackers can access all kinds of data from this attack. In addition, the computing power that can be obtained with a single server is limited. Therefore, using a centralized system in large networks makes scalability difficult.
• Centralized control plane (MME) and distributed data plane (S-GW and P-GW): With the distributed data plane, the signal overhead will be reduced as the destinations will be more specific. In addition, in the distributed architecture, the MME can reduce latency by choosing the most appropriate S-GW. Increasing the number of gateways is ineffective in increasing capacity after a certain number. Therefore, the excessive increase in cost can be prevented by choosing the optimum number of gateways (Chan et al., 2011). Distributing the data plane enables local video services by allowing edge computations. Finally, centralizing the control plane makes MME pooling, scaling, and redundancy impossible.
• Distributed control plane (MME) and centralized data plane (S-GW and P-GW): Distribution of the control plane improves reliability and reduces the latency of mobility management mechanisms such as handover. However, using a central anchor point causes delays in the transmission to reach its destination, whereas the systems with distributed control planes ensure finding the optimal route and reduced encapsulation overhead increases capacity (Chan et al., 2011). In addition, the network is set up to provide mobility support to each user. This results in the unnecessary use of network resources in mobility support.
• Distributed control plane (MME) and distributed data plane (S-GW and P-GW): As a final network distribution scheme, distributed systems are more reliable than centralized systems. Because they can serve multiple regions from independent servers without connecting to a single server, the service can be expanded as required by installing more servers to make scalability easier. Furthermore, this system reduces the risk of bottlenecks by distributing the processing load to different servers. However, distributed systems are more constrained and time consuming to deploy and maintain than centralized systems, and each server must be optimized internally. Lastly, distributed systems require coordination between different servers in case of inter-server interruption or failures that may result in service interruptions.
Summarizing different deployment scenarios, the central systems have a simple structure, but delays are difficult to avoid. They are also very susceptible to errors, and one failure can prevent the entire network from working. In addition, increased overheads reduce capacity, and resources are wasted while providing mobility support. Increasing demand for resources makes it difficult to scale and manage the network. On the other hand, distributed systems increase complexity, although they can reduce latency. Distributed systems are more resistant to failures, so the system can continue to operate even if failures occur. Also, depending on whether the data plane or control plane is deployed, two different overheads can be reduced as mentioned in Chan et al. (2011). Resource usage can be reduced by optimizing mobility support in distributed systems. Finally, network scalability is easier, therefore the network can be easily expanded and managed according to the increasing demand.
The distribution of the control plane is a process of pooling and clustering a certain number of MMEs in a given geographical area and is called MME pooling. MMEs in the same pool work as a single MME, even though they are physically different devices. Pool boundaries are determined, so connection problems do not arise in places where the received signal strength decreases. MME pooling provides geo-redundancy and increases capacity. MME handoff within the same pool is operated by reducing the signaling overhead and latency in the same pool. Additional MMEs can be easily attached to the system in a planned manner and allow maintenance without service interruption. According to Savic (2011), the moderate deployment of MME reduces latency and allows fast control signaling and call setup. It facilities MME pooling/scaling and provides geo-redundancy of the MME. The P-GW and S-GW should be deployed as distributed as possible. In other words, they should be placed on the edge to provide local video services, reduce latency, and distribute user traffic.
2.5 5G deployments
LTE network operators actively research the best 5G deployment and migration options. However, this is not an easy task as it depends on the operator’s current CN and RAN deployments. Thus, there is no single option that is optimal for all operators. Due to this reason, 3GPP has defined several architectures for 5G deployment, as shown in Figure 2. In this figure, defined architectures are standardized to provide backward compatibility with LTE UE, enable utilization of some of the 5G features to the new generation UE, and provide higher data rates via dual connectivity to both LTE and 5G networks.
The options (Opt.) demonstrated in Figure 2 can be classified as standalone (SA) and non-standalone (NSA). Opt. 1, 2, and 5 are the SA architectures. Opt. 1 is the current state of the operator’s LTE network. In a 5G deployment/migration scenario, every LTE network starts from Opt. 1. Opt. 2 is the standalone 5G network which is the end goal of every 5G deployment/migration process. Opt. 5 is a scenario where 5G CN is deployed and used with ng-eNBs. These ng-eNBs are enhanced versions of conventional eNodeB; however, they do not support new physical layer features of 5G. As such, Opt. 5 does not support features like mmWave and multi-numerologies. The remaining options are NSA architectures, defined for backward compatibility while enabling some of the 5G features in the migration process. Opt. 3 allows new 5G physical layer features to be used in the network while serving users from LTE EPC. In this option, eNodeB is the master node that directs the 5G traffic into en-gNBs (en-gNB is a gNB that can communicate with an eNB). In some extensions of Opt. 3, it is possible to direct some of the traffic directly from EPC to the en-gNB. Opt. 4 has an established 5G core and gNB network with ng-eNBs. In this architecture, the master node is the gNB, which steers some of its traffic to the ng-eNB according to the operation scenario. Finally, Opt. 7 uses an established 5G core to serve users mainly via ng-eNBs and uses gNBs to enhance data dates via dual connectivity. In Opt. 7, the existing LTE RAN can utilize 5G core features like network slicing. For more detailed information on each architecture, readers are referred to 3GPP (2017).
Both 5G CN and RAN have drastic changes and improvements over their LTE counterparts. Thus, direct deployment of a 5G network or direct migration from LTE to 5G is infeasible. Therefore, operators can achieve this migration in multiple stages using the architectures defined in the standards. For example, Opt. 1 → Opt. 3 → Opt. 2 is a possible approach for commercial networks to achieve a 5G network. With this approach, the operator can leverage its existing CN and RAN infrastructure with added benefits and smoothly transition into the 5G network by efficiently deploying gNBs. However, for PSNs, Opt. 1 → Opt. 7 → Opt. 2 seems more suitable. With annual reports of the First Responder Network Authority (FirstNet) [USA’s PSN authority—Federal Communications Commission (FCC), 2012], most investments are made on the core side. This makes sense since most public safety features involve the CN. Therefore, public safety operators can establish a standalone 5G core without steering away from their investment plans, which can be used with their existing LTE RAN infrastructure for service. The migration process can be planned in even more stages with a mixture of defined architectures.
3 Infrastructure sharing
Infrastructure, comprising electronic components, physical sites, and towers, is a critical part of any wireless network. In most cases, operators tend to deploy their infrastructure independently. However, infrastructure sharing between different operators has become increasingly popular due to cost (capital and operating) cutting benefits. This strategy is also considered for PSNs; however, in case of which, the following tradeoff should be considered: the reduction in expenses due to sharing comes at the cost of the operator's flexibility and control over the network.
Infrastructure sharing can occur in three different ways depending on the current situation of MNOs. In the first case, one or more operators can deploy a network according to their requirements. Second, operators can choose to merge their existing networks, and this type of network sharing has significant cost advantages. As a last option, one of the operators can agree to receive resources and services from the other operator’s existing network (Meddour et al., 2011). As the PSN migrates to LTE network, in any active infrastructure sharing scenario, a sharing is most likely over the last option since operators’ LTE networks are available and in service.
According to GSMA (2012), Australian operators Telstra and H3G have RAN sharing agreements, and Cyprus operators Vodafone and Areeba have national roaming and site sharing agreements. In Germany, the operator O2 uses the T-mobile network for national roaming. In 2009, Tele2 and Telenor in Sweden created a partnership to build a statewide 4G network and share spectrum. Other operators have shared mobile spectrum and network building costs (Systems, 2015). In addition, the operators Vodafone and Orange in the United Kingdom are preparing to share RAN. We have given these examples for commercial sharing situations. The examples of infrastructure sharing for PSNs will be detailed in Section 5.
A network architecture known as 5G network slicing makes it possible to multiplex virtually separate logical networks on the same physical network infrastructure (Rost et al., 2017). Each network slice is an independent end-to-end network built to satisfy the demands of particular applications (Foukas et al., 2017). The idea of network slicing may be seen as an alternative to network sharing since it creates an end-to-end logical network that utilizes shared infrastructure. According to the network slicing idea, RAN, transport, and CN resources for the network provider are virtualized, and all these resources may subsequently be dynamically or permanently given to various MNOs (Ksentini and Nikaein, 2017; Afolabi et al., 2018).
3.1 Passive sharing
Passive sharing involves sharing of the physical site and any passive elements, such as towers, facilities, power supply devices, air conditioning equipment, and backup systems (Nokia, 2014). In other words, this enables the existence of independent networks that share the physical environment and therefore do not require coordination between the MNOs. The passive sharing of radio and backhaul resources allows operators to control their resources, resulting in reduced site rental and construction costs. The different passive sharing options are described in the following sections.
3.1.1 Site sharing
In a network deployment, the cost of network sites is often unnecessarily expensive to operators. In this scenario, MNOs locate their BSs on the same site and negotiate with each other regarding site location selection. Each MNO uses its network equipment, so sharing is only based on location as shown in Figure 3. The advantages of site sharing are the costs associated with site leasing and sharing energy resources among MNOs. For these reasons, the site-sharing scenario is more likely to be used for the PSN because it is the sharing scenario with the most straightforward configuration.
Ordinarily, MNOs enter directly into a site-sharing arrangement. However, permitting third parties, called tower companies, have recently been included in such agreements to provide towers to communications providers. In a PSN deployment, operators can consider various site-sharing agreements (Frisanco et al., 2008).
1. The commercial operator agrees to grant a PSN operator access to their facility.
2. The commercial operator and PSN operator agree to grant access to each other’s facilities.
3. The PSN operator and multiple commercial operators agree to grant access to each other’s facilities.
In addition, both the MNO and PSN site owners are likely to require the reuse of their current land mobile radio locations (NPSTC, 2012a). Therefore, the PSN administration has an advantage in the site-sharing scenario. The PSNs request to migrate to the LTE network with its legacy TETRA and P25 sites. They may make these sites available to the partner operator by increasing the operator’s interest in making a site-sharing agreement. This makes it possible for the operators to provide service coverage in a wider geographical area, which is especially attractive for operators bound by geographic coverage restrictions.
3.1.2 Mast sharing
Mast sharing is a specific type of site sharing. In addition to site sharing, MNOs share the same tower, rooftop, or antenna frame for remote radio heads (RRUs) deployment as seen in Figure 3. Tower sharing has some challenges. One of which is two or many MNOs network services operating in the same physical place. Therefore, the load-bearing capability of the towers, the azimuth angles of various service providers, antenna tilt, and height should be considered by the operators before making the contract (GSMA, 2012).
Also, according to NPSTC (2014), PSN networks require more secure and reliable sites and towers than do commercial networks. Accordingly, in the case of mast sharing, current masts might not be built to fulfill the overhead requirements of operators who choose to share the mast. However, new masts might be constructed with the necessary final load-bearing capability. While existing masts may support the higher weight, they might not have adequate room for the new equipment. Therefore, they should be evaluated independently (GSMA, 2012).
3.1.3 Backhaul sharing
The transport network that connects the mobile network’s CN and RAN is referred to as mobile backhaul. Backhaul can be established via wireless (mmWave or free space optical) or wired (fiber) deployment. Wireless backhaul deployment is much more profitable than fiber backhaul deployment in terms of the initial deployment cost. However, mmWave backhaul imposes a high OPEX cost, especially in urban areas where the frequency bands become expensive and congested due to the existing spectrum crunch (Feng et al., 2016). In addition, equipment is more expensive at these frequencies. Also, the connection distance and capacity of the infrastructure are more limited than is for fiber (Sharma et al., 2021). Accordingly, once the fiber is installed, its OPEX is lower than is for mmWaves.
According to Systems (2015), 80% of the fiber cost comes from excavation and construction work. Due to the initial cost, it takes 18–20 years for the fiber to catch the mmWave in total cost, and this time is excessive for the operator. With emerging technologies such as micro-trenching, it may take 6–8 years for the fiber cost to catch up with the mmWave cost. As a result, although the mmWave capacity and operational costs are higher, it is more common than is for fiber because of the initial cost being much lower. However, the demand for fiber is increasing in the long term. On the top of these, operators are becoming more interested in pooling backhaul infrastructure as a way to reduce total network expenses. In addition, railways are known to install fiber infrastructure on train routes to fulfill the signaling function of trains. Since fiber infrastructure is strong in countries with many railways, it may be profitable to share infrastructure with railways in these countries. This is explained in Section 6.
3.2 Active sharing
Active sharing means sharing active network elements (such as antennas, radio nodes, node controllers, and switches) that are commonly used by operators. In the locations data, traffic is growing drastically, and active sharing can be risky as adopting the current situation becomes difficult to manage due to operators' conflict of interest. Conversely, active sharing is recommended to reduce new network deployments where data traffic is limited. This section is divided into two parts depending on which part of the network (radio access or core) is shared.
3.2.1 Radio access network sharing
RAN sharing refers to two or more MNOs sharing RAN equipment and network infrastructure. Instead of unfairly using each other’s resources to generate income, MNOs should share fairly in order to serve consumers more effectively according to SLAs. Sharing the RAN infrastructure between two or more operators makes it easy to generate large CAPEX savings in distant and rural locations where coverage is the key design requirement for radio network implementation. On the other hand, sharing physical sites are sometimes the only practical solution for improving capacity in urban regions with the shortages of available sites. However, this often results in more hardware and a larger physical footprint on each location (Nokia, 2014). In this section, we present various distribution possibilities for RAN sharing.
3.2.1.1 Multi-operator radio access network
Figure 3 shows the multi-operator RAN (MORAN) sharing scenarios. In the antenna sharing scenario, the RRU is shared by MNOs. Radio units and the support system can be shared by using baseband units (BBUs) from several MNOs. Operators have their carriers and unique parameter configurations. One of the advantages of using multiple BBUs is that MNOs can individually configure their eNodeB to meet their QoS requirements. Since the RRUs are shared, only BBU investment is required. However, the cost-benefit of this sharing is limited as the costs of the transmission equipment and antennas are relatively small (Meddour et al., 2011). Regarding antenna sharing challenges, RRU sharing requires a spectrum configuration. In addition, the RRU frequency range must complement the spectrum of MNOs. Also, the difficulty of RRU connection management increases if multiple BBUs are connected to a single RRU. Moreover, RAN sharing can hurt QoS levels due to reduced signal strength when antennas are combined. This represents a reduction in output power and affects the network’s coverage, that is, it may not meet that QoS parameters in some areas such as indoors. However, this does not apply to the leading 3GPP RAN sharing techniques (GSMA, 2012). According to Meddour et al. (2011), commercial operators can experience CAPEX and OPEX savings in the range of 20%–30% by sharing sites and antennas.
In the BS sharing scenario, BS is shared by MNOs. On the RAN side, many carriers can be utilized individually by MNOs. However, one common BBU is utilized for transport traffic aggregation, and cells are linked to operator-owned carriers. As the advantages of this scenario, each MNO aggregates mobile traffic on its carrier frequencies and is fully compliant with regulatory authority standards since S1 interfaces are standardized in 3GPP (3GPP, Mar. 2022b). In addition, BBU expenditures are shared by MNOs. When compared to other MORAN options, ensuring QoS requirements will be comparatively/relatively simpler. However, MNOs should have an agreement on QoS requirements as there may be a conflict of interest in power sharing since the ownership of BBU is discussible. Moreover, different MNO carriers operate at the same BBU, therefore management complexity of the BBU increases.
In MME and BS scenarios, two or more MNOs can share BS and MME. Since MME is attached to a BS, the number of signaling control planes (CPs) is reduced when compared to the other MORAN options. From the cost perspective, this option does not require additional investment in MME, BBU, and RRU. This scenario also has some difficulties. BS authentication is conducted in MME (3GPP, Mar. 2022c). Therefore, this can introduce security issues when the MME is shared. In addition, MNOs may experience problems with the authentication of BS because MNOs can use different CP signal parameters.
3.2.1.2 Multi-operator core network
Figure 3 shows the multi-operator core network (MOCN) sharing scenario where the RAN part is completely shared that includes the spectrum. MNOs share one BS at the cell site. Meanwhile, they use different dedicated CNs. As an advantage, this option saves the carriers from extra RAN investment burden since the RAN portion is fully shared. When compared to the MORAN scenarios, this option is easier to configure as the frequency is also shared. Although the MOCN scenario is assumed to be stable and permanently installed, it can also be used dynamically by allowing radio access to be shared between two or more operators only during off-peak hours to save energy. No anticipated changes to 3GPP standards are required for this operation (3GPP, Jun. 2011). Since the ownership of BS is discussible, operation on the RAN side is difficult. Moreover, there are regulatory challenges for BBUs and carriers due to traffic aggregation on the same nodes.
Additionally, MOCN networks must provide ProSe communication between two UE camped on the same radio access network but serviced by separate MOCN public land mobile networks (PLMNs) according to operator regulations (3GPP, Jun. 2013).
3.2.1.3 Gateway core network
Figure 3 shows the gateway core network (GWCN) sharing scenario, where the S-GW and MME are also shared as CN elements in addition to RAN sharing. Since the S-GW, MME, and BS are shared, no additional connections are required between the MNOs. The spectrum is shared between the MNOs or allocated to each MNO separately. Due to the huge number of virtualized terminals, MVNOs should employ this technology instead of MNOs. As a result, the MVNO regulatory permit may be necessary for MNOs. However, the threat of sharing the MMEs responsible for the authentication and authorization of UE complicates the installation of the GWCN on PSNs. It is desirable for PSNs to have their own MMEs (Jarwan et al., 2019) since the information on roaming and access restrictions must always be provided to the eNBs by the MME in the GWCN sharing scenarios (3GPP, Mar. 2022a). In the GWCN scenario, an overload initialization procedure is applied as a solution when there is an MME overload since the MME is shared. This is to notify the relevant BS to reduce the signal load regardless of whether an operator exceeds the limit (3GPP, Jun. 2014b).
It is stated that in all RAN sharing scenarios, the RAN part should be configured according to the different requirements of the operators. Contrary to the use of similar assets, creating a truly fair and value-based share requires an equal exchange of guaranteed performance. Therefore, a performance monitoring system is required that evaluates current throughput and bandwidth usage along with key performance indicators (KPIs) such as latency, latency ripple, and packet loss. Carriers can establish and maintain significant SLAs with such a framework. Both operators benefit from increased network coverage and revenue potential when shared assets are used, and fair performance metrics and reporting are also provided to ensure accountability and equal access (Accedian, 2015). Performance monitoring for RAN sharing provides several benefits. It allows carriers to reduce costs by allocating RAN resources fairly based on usage, enables operators to meet SLAs, and supports capacity planning.
3.2.2 Core network sharing
Servers, core network components, and radio equipment sharing are all included in core network sharing. Hardware such as switching centers with HSS, PCRF billing platforms, and systems also correspond to logical entities of the CN, which include equipment identity register (EIR) functions. EIR functions can be expensive, so operators find it appealing to share these resources (GSMA, 2012). However, the CN carries out several crucial tasks in delivering the operator’s services and holds a wealth of sensitive data about the operator’s operations. As a result, sharing a CN across competing operators might be challenging. However, there are alternative forms of sharing, such as national roaming or the creation of MVNO where operators can utilize the same underlying network to deliver their services (Meddour et al., 2011).
The PSN services that share a CN with commercial operators can reduce the traffic load and cost of the network. On the other hand, the commercial operator has the right to carry out package inspections at the P-GWs (3GPP, Mar. 2021b). Accordingly, the commercial operator can inspect PSN communications. Therefore, a possible sharing scenario will require encryption at the application layer. In addition, the HSS should be separate for security, and each operator should keep their clients’ databases for security measures. Moreover, the commercial operator will be required to make arrangements on the CN, and the workload on commercial gateways will increase according to the non-shared scenario. In the scenario where PSN services are hosted within a private CN, service quality is consistently maintained because workload planning is based on the gateway. Furthermore, security is enhanced due to the PSN’s isolation from external networks.
3.3 Network roaming
Network roaming, within the scope of network sharing, means that an operator provides services to their clients with resources that do not belong to them by using the infrastructure of another operator. Although traffic from one operator’s subscriber is being transported and routed via another operator’s network, network roaming can be seen as a type of infrastructure sharing. However, common network elements are unnecessary for this sharing to occur. As long as the two MNOs have a roaming agreement, roaming is permitted. The following categories can be used to classify roaming, national roaming, international roaming, and inter-system roaming (GSMA, 2012).
• National roaming usually occurs between competing providers within the same nation, providing services in the same or different geographic areas. This is especially useful for a site that operators allocate in low-income regions due to low subscriber density.
• International roaming is similar to national roaming in some ways, except that roaming is done between carriers in different countries. In this way, customers can use their phones as usual while traveling abroad. International roaming is more complicated than national roaming, as various authorities in different countries assign different frequency bands to the same technology. Accordingly, handsets are operable in different bands.
• Inter-system roaming occurs between networks using various standards and different architectures, as in broadband LTE and TETRA roaming. Inter-system roaming provides a way for new platforms to provide the same level of coverage as a network installed before release. In this way, inter-system roaming often facilitates the implementation of new standards and technologies.
Moreover, according to 3GPP (Sep. 2018), each CN operator must be allowed to set the roaming contracts in the event of MOCN shared network deployment.4 Roadmap to cost analysis
In this section, LTE coverage and capacity dimensioning is explained and a roadmap to cost analysis is made. Then, the required number of sites is found according to the dimensioning analysis. Next, the cost model of radio access elements is given, and insights about the cost is made depending on the RAN and passive elements of infrastructure.
The first stage of network planning is dimensioning, which involves determining the number and capacity of the network components necessary to provide sufficient QoS to its users. Dimensioning is predicated on a particular set of input parameters, and the outcomes are only pertinent to that particular set of parameters that include the considered region and anticipated traffic.
4.1 Coverage dimensioning
Coverage dimensioning is achieved using radio link budgeting and propagation models as explained in the following sections.
4.1.1 Radio link budget
Radio link budget specifies the largest region that a base station may cover. The service throughput specified by the cell edge user corresponds to a required signal-to-interference-and-noise ratio (SINR) level at the receiver which is used to compute the maximum allowable path loss (MAPL). By using proper propagation models, the MAPL is transformed to distance. In coverage dimensioning, this distance or radius of the cell is used to calculate the number of sites required to cover the entire region (Huawei, 2010).
It is important to note that the characteristics of the deployment region of the network (dense urban, urban, suburban, rural, etc.) are decisive for the link budget results due to different propagation loss characteristics. For calculating the required number of sites, first, effective isotropic radiated power (EIRP) and minimum signal strength required (MSSR) must be calculated, then MAPL calculation must be made separately for downlink (DL) and uplink (UL) according to these calculations (Chanie, 2020). The maximum allowable attenuation of the radio wave at the air interface, depending on the propagation patterns is expressed as
where margins refer to the power used to mitigate interference and fading. The possible losses in this equation include penetration loss, body loss, and feeder loss. Gains are obtained from diversity, antenna, etc. The MAPL has different values for the transmission medium, as well as for the DL and UL. For LTE-based PSN UE with no external antennas, the EIRP and MSSR are given as
Since transmission in the UL will be from UE to BS, the body loss and feeder loss will be swapped in the EIRP and MSSR formulas because body loss originates from UE and feeder loss originates from external antennas.
We can formulate the receiver sensitivity, which indicates the MSSR to enable decoding by a BS or UE if there is no interference as
Extra path loss is incurred when a signal traverses from the outdoor to an indoor environment, referred to as the penetration loss, caused by the structure/material of the building. It differs on every clutter type, such as approximately 15 dB rural loss, 8 dB urban loss, and 6 dB indoor/in-vehicle loss (Chanie, 2020). Feeder loss is the term used to describe signal loss caused by the devices in the antenna’s path to the receiver. Body loss occurs when a terminal antenna is placed close to the body, blocking and absorbing the signal. The BS that affects the DL budget and terminal side that affects the UL budget should consider the transmitter power.
Antenna gain defines the power density ratio of signals produced from the same point by the actual and theoretical antenna when the input power is the same. The interference margin describes the increase in terminal noise level caused by user interference. There is a trade-off between capacity and coverage in cellular technologies, as the interference margin highly depends on the actual projected capacity. The blockage caused by a structure or natural feature is called slow fading margin or shadow fading. According to statistics, the average signal levels received over a given distance follow the log-normal distribution with time and location.
The SINR is the primary LTE performance metric. Cell edge distance is defined by the required SINR for the minimum throughput for the served users. As a result, the correctness of the radio link budgeting and dimensioning processes enable efficient/accurate/better cell deployment. Exemplary modulation and coding scheme (MCS) and the propagation channel model impact the required SINR. Higher modulation orders require a higher SINR. The Shannon formula C = BW log2(1 + SINR) may be used to get the capacity for the target SINR, where C and BW refer to channel capacity and bandwidth, respectively.
These parameters are the client specifications that guarantee delivery of the service requested by the user. The site radius and the total number of sites are determined using the cell edge performance criterion. There are three ways to improve cell edge performance. These include figuring out the lowest data rate for maximum coverage at the cell edge, adaptive modulation and coding, and establishing larger cell radius.
The LTE can function in the 700, 1,800, and 2,600 MHz bands. Knowing the geographic conditions of the target region, SINR distribution information, and channel types (car, pedestrian) are also necessary.
4.1.2 Propagation models
The link budget heavily relies on the radio propagation model. An appropriate propagation model accounts for the maximum path loss and enables an assessment of the maximum cell coverage area. The number of BSs necessary to cover the desired geographic region is determined by the cell coverage area. The cell’s coverage area will change depending on the chosen carrier frequency. As the carrier frequency decreases, cell coverage area increases.
There are two types of radio propagation patterns: indoor and outdoor. Different elements play a role in these propagation patterns. Obstructions in the propagation path such as structures and trees should be considered for outdoor environments. Different configurations result in different rates of signal loss. The least amount of fading occurs while spreading in open space. In urban or suburban regions, radio waves attenuate more than they do in free space. Moreover, we see the highest fading rates in the dense urban environments. Low RF transmit power, a small coverage area, and dynamic environmental variations are characteristics of the indoor propagation model. Frequency band and deployment region type (transmission medium) affect how signals propagate. The Okumura–Hata and Longley–Rice models are mentioned in (Abebe, 2019), and cell radius formulas are given according to these propagation models.
The formula for cell radius (R) in kilometers for free space, depending on carrier frequency (fc) in MHz and path loss (PL) in dB, is given as (Cerwin, 2019)
The formula of the Okumura–Hata model, which is much more realistic than the free space model for urban areas, is given as (Rappaport et al., 1996)
where ht, hr, and Cf refer to the effective transmitter, receiver heights, and correction factor, respectively. The formula of the Longley–Rice model, which is not only more accurate with accurate terrain elevation data but also more computationally intensive (Kasampalis et al., 2014) for urban areas Line of sight scenario, is given as (Longley, 1968)
where k1, k2, and W() refer to slopes of a smooth curve and Lambert W function. Ae corresponds to A0 − k1d0 − k2 log d0, where d0 is the reference distance and A0 is the computed attenuation at this distance. The site coverage areas can be found on the basis of the computed cell radius.
Site areas are found on the basis of sectorization. If a site coverage is omnidirectional, then the coverage area can be found from
4.2 Capacity dimensioning
The coverage dimensioning procedure is then followed by capacity dimensioning. The capacity analysis provides a prediction of the number of sites that are required to transfer the anticipated traffic over the coverage region. This process determines if the system can support the determined load with the current site density or if additional sites are required.
The total number of eNodeBs placed in the network determines the network’s theoretical capacity. Various phenomena impact cell capacity in LTE, such as interference intensity and packet scheduler implementation. LTE capacity dimensioning aims to determine the network’s packet switch throughput depending on each user’s channel condition and available bandwidth.
Cell average throughput calculations are done together with configuration analysis for capacity analysis. Then, the number of supported subscribers per cell is calculated with the help of traffic model analysis. Then, if the number of eNodeBs found from the coverage analysis meets the total subscriber capacity requirements, the number of eNodeBs will be the same obtained from the coverage analysis. Otherwise, the number of eNodeBs should be adjusted according to the capacity requirements.
Examples for configuration analysis can be given as a selection of frequency reuse mode, bandwidth, carrier configurations, multiple-input and multiple-output (MIMO) configurations, etc. Traffic model analysis specifies customer requirements, such as target user’s number, active user ratio, service bearing rate, overbooking, cell edge access rate, and average data rate. Single site capacity is calculated from system simulation after configuration analysis from eNodeB capacity. Cell average throughput can be calculated as (Syed, 2009)
where Pi is the probability corresponding to the carrier-to-interference-and-noise ratio (CINR) and cell average Throughputi is the throughput calculated on the basis of the CINR. Network throughput can be calculated by multiplying single-user throughput by the number of users. It is then divided by the number of eNodeB found from the coverage analysis. If the result satisfies the cell average throughput, the eNodeB number has been found; otherwise, it is adjusted back to the capacity requirements. CN capacity dimensioning is done in a similar fashion. The number of UE is considered as the capacity when determining the number of MMEs and HSSs required to deploy a network. On the other hand, the operational capacities of the S-GW and P-GW are calculated over throughput.
In order to meet the requirements of PPDR services, EPC units must not exceed a specific load. Both for this reason and to ensure the geographical redundancy of the system, the deployment plans of the units should be made accordingly. For the health of the PSNs, the average loads of the units should be kept at 80% (Checko et al., 2012). In order to keep the network load at this level, the CN capacity dimensioning should be done over these ratios.
When the load capacities determined by the configuration exceed, the EPC units can allow the communication to continue by redirecting traffic over distributed network elements. MME backup is not required in a distributed deployment scenario as the MMEs are easily interchangeable. However, with the use of backup MMEs in centralized distribution, when the MME capacity exceeds 80%, requests to this MME are forwarded to the backup MME. After CN capacity dimensioning, a distributed deployment architecture can be applied to meet the latency requirements of PPDR services. In a way, this can be seen as CN coverage dimensioning.
4.3 Case study for cost model
In this section, we delve into a case study focusing on installing broadband PSN on commercial LTE sites, based on a technical paper (Seybold, 2010). Additionally, we incorporate the cost ratios for establishing independent PSN sites in rural areas from the same study, subjecting them to joint analysis. This reference is the source for underlying equipment and CAPEX assumptions. Nonetheless, recognizing the potential for cost fluctuations over time, our study exclusively offers a case study on cost model ratios. We present preliminary insights into potential installation cost reductions achievable through various sharing scenarios outlined in Section 3 for PSN site deployment. Furthermore, we quantify the incremental cost changes for these configurations as percentages. The calculations and methodologies are briefly summarized in Seybold (2010) for an in-depth understanding. Given the lack of reliable sources about cost components on the internet, our cost analysis in this section is predicated on a singular basis. The primary objective of this section is to furnish an approximate cost recovery projection within infrastructure sharing scenarios rather than offer an exhaustive cost breakdown.
The technical paper encompassing the broadband network cost model contains six distinct PSN installation configurations for LTE cells, alongside two standalone rural PSN site installation configurations. Table 4 provides a comprehensive overview of the cost escalation percentages for varying PSN upgrades or new installations. The following section focuses on the features and essential aspects of these configurations and why there is a price difference between them.
• Configuration-1: Non-Rural Site Upgrade Configuration with Coax and 22.5 m tower height
The cost components of this scenario can be summarized as follows: cables, tower connection material, MIMO antenna, eNodeB, eNodeB termination fiber Ethernet, engineering, and installation. Tower connection materials are employed twice due to the coaxial cable being wound twice per sector, doubling the cost. In the context of three sectors, their respective costs are multiplied by three, as antennas supporting the MIMO technology are a requisite for each sector. Except for engineering, installation, eNodeB, and connection costs, the expenses for this equipment are regarded as the three-sector cost for the subsequent case study.
• Configuration-2: Non-Rural Site Upgrade Configuration with Coax and 45 m tower height
In this configuration, the three-sector cost can increase by 117% due to the necessity of tower-mounted amplifiers. Additionally, engineering and installation costs may experience an 18% rise, as these expenses can be computed to be 40% of the total equipment cost (Seybold, 2010). If we analyze these configuration preferences with regard to the tower height, we can identify scenarios that fall into non-rural categories, such as urban and suburban areas. In urban areas, where the usage density is generally higher, capacity may be emphasized. Low tower height and narrow coverage area might be necessary to serve more capacity efficiently within a confined space. This may also help prevent inter-cell interference.
• Configuration-3: Non-Rural Site Upgrade Configuration with Fiber and 22.5 m tower height
When a fiber cable connects equipment, a remote radio head (RRH) must be added. In addition, because the contents of the cables and tower connection materials change, the cost of the three sectors may increase by 155% when compared to the tower height of 22.5 m and the coaxial cable configuration.
• Configuration-4: Non-Rural Site Upgrade Configuration with Fiber and 45 m tower height
In contrast to the scenario involving the use of the coaxial cable, there is minimal cost variation when doubling the tower height. This is due to the fact that the RRH is not duplicated with the increase in tower height, and it is a component that constitutes the majority of the costs.
• Configuration-5: Rural Site Upgrade Configuration with Coax and 67.5 m tower height
The equipment remains consistent with that of the non-rural scenario. Up to this point, the costs mentioned pertain to the RAN portion, encompassing the three-sector cost and eNodeB expenses. In addition to these costs, two supplementary expenses are associated with upgrading an existing LTE site in rural scenarios: microwave equipment for backhaul and hardening. Regarding tower height, rural areas are expected to have a lower density of users, making coverage a primary concern in contrast to non-rural areas. Employing high tower structures helps prevent unnecessary site installations and provides service over a wider coverage area.
• Configuration-6: Rural Site Upgrade Configuration with Fiber and 67.5 m tower height
The cost can be potentially reduced by 1.6% when compared to the coaxial cable configuration due to the increased cost of the tower-mounted amplifier. It should be noted that upgrading LTE cell sites to the PS network inherently involves mast and site sharing.
• Configuration-7: Rural Site Installation Configuration with Coax and 67.5 m tower height
Additional payment is required for site acquisition and construction for a completely new installation. Site installation costs may be 68% higher than site upgrades for the coax-cable configuration.
• Configuration-8: Rural Site Installation Configuration with Fiber and 67.5 m tower height
Site installation may cost 69% more than does site upgrading for the fiber cable configuration for the same reasons.
As part of the case study, we compare the cost item ratios across the eight scenarios depicted in Figure 4. The comparison reveals that, for non-rural configurations, a substantial portion of the cost is attributed to the eNodeB component. By contrast, rural configuration costs are more diversified, encompassing hardening and microwave equipment expenses. This distinction becomes even more pronounced when considering site acquisition and construction in new PSN site installations.
Fronthaul is characterized as the fiber-optic link within the radio access network (RAN) framework, connecting the baseband unit (BBU) and remote radio head (RRH). Conventional coaxial systems on existing cell towers worldwide are undergoing a comprehensive transformation. The outdated and resource-intensive copper cables, known for their size, cost, and high power consumption, are being removed and substituted with fiber optic cables. This transition to fiber optics provides enhanced capacity and greater reach and introduces heightened scalability. This improved infrastructure ensures sustained expansion of coverage and bandwidth, all while delivering cost-effectiveness well into the future (Macknofsky, 2015). As the utilization of fiber cables has gained popularity, this analysis exclusively focuses on fiber cable configurations (Wake et al., 2004; Systems, 2015). Based on the provided data, we can derive insights concerning cost savings for two of the MORAN scenarios and passive infrastructure sharing in the deployment of PSN sites.
1. Site sharing: In installing the rural site configuration, as depicted in Figure 4, site acquisition constitutes approximately 16% of the total cost. Therefore, by establishing a site-sharing agreement with MNOs, it is possible to mitigate 16% of the cost. Unfortunately, we cannot provide any cost-related information concerning site and mast sharing for new installations in the non-rural scenario due to the absence of available cost data.
2. Mast sharing: In addition to the cost of site acquisition in site sharing, the construction cost is also eliminated in the mast sharing scenario. Therefore, 41% cost savings can be achieved by making a mast sharing agreement with the MNOs.
3. Antenna sharing: Antenna sharing saves the PPDR operator from the cost of the three sectors and passive infrastructure cost. In non-rural scenarios, sharing antennas with MNOs can save 23% of equipment costs in the three sectors. There will also be savings in engineering and installation costs, which account for 40% of the three-sector cost. In other words, a total of 51% savings can be achieved. For the upgraded and newly installed PSN in rural scenarios, sharing antennas can lead to 11% and 7% cost savings, respectively, as shown in Figure 4. This translates to 24% and 56% savings, respectively, covering the costs of engineering and installation, site acquisition, and construction.
4. BS sharing: Base station sharing will save eNodeB costs and costs of the three sectors within the RAN portion. In non-rural scenarios, when the PPDR operators engage in BS sharing, they can achieve savings of 23% for three sectors, 28% for engineering and installation, and 49% for eNodeB costs, as depicted in Figure 4. This signifies that the PPDR operator incurs no expenses beyond the infrastructure sharing contract fee with the MNOs. For scenarios involving the upgrading and installation of new rural sites, all costs except for the hardening cost will be eliminated. This could lead to cost savings of 67% for upgrade configurations and 81% for new installation configurations.
The case study demonstrates that some infrastructure-sharing scenarios can result in substantial cost savings. In other sharing scenarios, the greater the degree of sharing, the more significant the cost savings. However, when negotiating contracts with mobile operators, it is essential to consider the factors specified in Section 3, such as the QoS requirements of PPDR users.
5 Country applications
5.1 United States
The idea of establishing a public safety broadband network (PSBN) comes with the Middle Class Tax Relief and Job Creation Act of 2012 (which will be referred to as the Public Safety Spectrum Act). The Public Safety Spectrum Act establishes the FirstNet to oversee the construction and operation of this network (FCC, 2012). To start off the network construction, the Congress had allocated FirstNet $7 billion and 20 MHz of 700 MHz (758–768/788–798 MHz) broadband spectrum (FirstNet, 2015). The Public Safety Spectrum Act specifies that the PSBN consists of CN and RAN that feature the following:
• The CN for the PSBN consists of national and regional data centers and other elements and functions that may be distributed geographically, all of which shall be based on commercial standards and provide the connectivity between the radio access network and public internet or public switched network or both.
• The RAN for the PSBN consists of all cell site equipment, antennas, and backhaul equipment, based on commercial standards that are required to enable wireless communications with devices, using the public safety broadband spectrum and shall be developed, constructed, managed, maintained, and operated taking into account the plans developed in the state, local, and tribal planning.
Since the Public Safety Spectrum Act had nothing to prevent private partnerships and required PSBN from being based on commercial standards, FirstNet released a request for proposal (RFP) in January 2016 (FirstNet, 2016), and in March 2017, FirstNet awarded AT&T with a 25-year contract (FirstNet, 2017). Figure 5 summarizes the financial model of the partnership between FirstNet and AT&T. In this partnership, FirstNet allows monetization of its 20 MHz spectrum and $6.5 billion over 5 years. Public safety spectrum access has interesting benefits itself. These benefits (Gallagher, 2018) are
1. Public safety spectrum is not limited to a defined geographical area.
2. Permit for the use of higher powered devices that can provide wider coverage for the 700 MHz spectrum licensed for public safety use.
3. For the 700 MHz spectrum, AT&T authorized to monetize does not count against spectrum holding limits set for wireless carriers by the FCC.
In return, AT&T gives access to their network assets which are valued at about $180 billion, brings their expertise for the operation of the PSBN, and allows self-sustainability to FirstNet through annual payments. Partner AT&T has to invest about $40 billion using its revenue, and by legislation, FirstNet has to reinvest any revenue that exceeds its base operating costs into the network. Due to legislation and technical concerns, the PSBN must operate with a standalone core. According to these constraints, AT&T has developed a solution for FirstNet. Within 1 year, the standalone FirstNet core was launched for public safety users, and AT&T’s solution allowed the FirstNet core to operate by accessing AT&T’s all spectrum and RAN (FirstNet, 2018). Through prioritization and preemption, public safety users benefited from the premium service offered by the nationwide PSN in a very short time. Through the revenues that AT&T and FirstNet produced, the network has continued to expand its coverage and network capabilities to serve more users anywhere in the country. Notably, the improvements in coverage affect AT&T’s service quality since deployed RANs can also serve commercial users of AT&T. USA’s approach to PSBN deployment showcases how much a public–private partnership can benefit commercial and PSN operators.
5.2 Korea
The Republic of Korea’s necessity for a dedicated PSBN has shown itself during the sinking of MV Sewol in 2014. Out of 476 passengers and crew, 304 died in the disaster (Park and Hancocks, 2015). During the incident, first responder authorities were criticized for poor handling of the disaster (Jin and Song, 2017). However, the main reason for the ineffectiveness of first responders was due to the outage of communications due to excessive usage at a moment of urgency (Choi and Kim, 2015; Kang, 2015). Following the Sewol incident, mission-critical LTE with 3GPP Release 13 was selected for Korea’s PSBN in July 2014, and 20 MHz from Band 28 (718–728/773–783 MHz) was allocated in December 2014 (Hong and Kim, 2019).
South Korea’s PSBN (Safe-Net) implements the “All Four One” design philosophy to provide nationwide coverage economically. This approach features the usage of the 700 MHz spectrum allocated for public safety that is shared between public safety long-term evolution (PS-LTE), railways (LTE-R), and maritime (LTE-M) as an enormous interconnected network. In this sense, the deployment of Safe-Net involves the deployment of three private networks interconnected with each other. “All Four One” solution involves four different classes of solutions interworking to achieve one unified PSBN. These solutions include fixed and mobile base stations deployed, operated, and maintained by the PS-LTE network, interworking with RAN and CN of commercial networks, and interworking with other networks built for public safety (LTE-R, LTE-M, TETRA). Instead of opting for a complete RAN sharing in FirstNet’s case, Safe-Net decided to have its own RAN in highly populated areas and use commercial networks’ enormous LTE coverage (Telecom, 2022) to give service in underground areas, indoor areas, and rural regions. Finally, other networks would be utilized as sub or main solutions in their respective scenarios. Table 5 summarizes the use-cases of these solutions.
Samsung has collaborated with the CN operator for Safe-Net in Korea KT to build the network (Samsung, 2019). PS-LTE, LTE-R, and LTE-M all have different ownerships and deployment plans. The PS-LTE network is owned by the Ministry of the Interior and Safety. The total budget (deployment and operation) for the PS-LTE project is about 1.48 trillion KRW ($1.08 billion). In total, three core networks, 15,000 base stations, and 240,000 user equipment are estimated to be deployed to the field (Hong and Kim, 2019). PS-LTE deployment started with pilot projects in November 2015 and was completed in March 2021 (Kim, 2021). LTE-R network is owned by the Ministry of Land, Infrastructure, and Transportation. LTE-R network deployment consists of migration from VHF systems for normal train/trunked radio system (TRS) for high-speed trains to LTE-R. The main project track is estimated to be 4,856 km. The LTE-R network is expected to deploy two core networks, 65,000 base stations, and 10,000 user equipment until 2027 (Kim, 2021). Finally, LTE-M that is owned by the Ministry of Oceans and Fisheries started at its development stage in 2016 with pilot projects and was completed in December 2020. Through LTE-M, Korea has provided coverage up to 100 km in its waters and launched its user service in 2021. Overall, two core networks, 700 base stations, and 35 k routers have been employed for the LTE-M network.
The “All Four One” approach is a unique approach that South Korea has come up with to provide nationwide coverage in an economical manner. However, as three different networks share the same spectrum, co-channel interference is one of the significant challenges, especially between PS-LTE and LTE-R networks. Later sections cover the analysis and solutions proposed in the literature for PS-LTE and LTE-R coexistence. Nonetheless, the “All Four One” approach is a very plausible design philosophy for countries with a small land mass that allows very efficient spectrum usage for multiple objectives.
5.3 United Kingdom
Airwave is a TETRA-based narrowband private network that serves emergency users in the United Kingdom. This system has a geographical coverage of 99% and population coverage of 98% (Ferrus and Sallent, 2015). Although the Airwave system still performs well today, it is costly to operate. This reason is the basis for the United Kingdom’s eagerness to replace Airwave with an LTE-based system for public safety users (General, 2016). This LTE-based system is called Emergency Services Network (ESN), and it provides integrated critical voice and broadband data services to its users. The ESN is jointly funded by the Home Office, Department of Health & Social Care, the Scottish and Welsh Governments, and by the emergency services (General, 2019). It is forecast to cost up to £9.3 billion in 2037 as compared to £12 billion for the Airwave system, with a break-even point in July 2029. For providing ESN, the Home Office awarded three main contracts in 2015 to Everything Everywhere (EE) for priority access to its existing networks—Motorola Solutions, United Kingdom—to provide the necessary software and systems, and Kellogg, Brown, and Root (KBR) to be the Home Office’s delivery partner. Apart from these three main contracts, there were also a few contracts with Vodafone in 2016 and Samsung in 2017.
For a better grasp on the ESN solution, it is beneficial to check on the public safety spectrum situation in Europe. The Electronic Communications Committee (ECC) within the European Conference of Postal and Telecommunications Administrations (CEPT) has allocated 5 × 2 MHz (698–703/753–758 MHz) and 3 × 2 MHz (733–736/788–791 MHz) bands for broadband public safety uses. However, according to our investigation, there are several problems that have risen from this allocation:
• There is the problem with supplying chipsets that support 698–703 MHz. Hence, it is difficult to utilize 5 × 2 MHz allocated for public safety.
• Fractured spectrum allocation (3 × 2 MHz, 5 × 2 MHz) that complicates hardware design.
• 5 × 2 MHz and 3 × 2 MHz is only enough to guarantee mission critical voice service.
Every European country that complies with this spectrum allocation has issues migrating from narrowband PSNs into broadband networks. Therefore, we can see unique solutions for each European country on this issue. The United Kingdom commercialized its public safety spectrum to EE and gained access to both LTE spectrum and RAN that EE owns. In this way, public safety users gain prioritized access to EE’s spectrum similar to FirstNet. The ESN solution can be summarized as follows:
• ESN users access the network via EE commercial RAN. Accordingly, EE has to increase the coverage of its mobile network to match the requirements.
• The ESN will have its dedicated core to support mission critical requirements and security for its users. In line with this, EE will deploy its part of the CN with Motorola Solutions for the ESN. Furthermore, Motorola Solutions will develop public safety applications as well.
• The link between the CN and control room will be provided by KBR.
The ESN is a unique solution that utilizes a similar structure to FirstNet without any dedicated spectrum. It is an interesting example that showcases that difference in spectrum allocations in different regions significantly impacts the migration process. The United Kingdom’s case also demonstrates that a dedicated network such as that in Korea is very difficult to realize for European countries. Hence, for PSBN solutions, European countries have to rely on commercial networks in one way or another due to insufficient spectrum.
5.4 Belgium
ASTRID is a government-owned corporation that was founded in 1998 and is responsible for operating the PSN in Belgium. ASTRID first served its users through a TETRA-based network. Later in 2014, ASTRID launched a broadband service called Blue Light Mobile (BLM). BLM allowed its subscribers to be served with a priority from commercial 3G networks. ASTRID has settled with Belgium’s three commercial networks Proximus, Mobistar, and Base. ASTRID has become an MVNO and managed its own subscriber identity module (SIM) cards. These SIM cards have their preferred network, which can be changed manually by the user or automatically when the coverage is lost to maintain continuous service. Later, the 3G service is upgraded to LTE service due to commercial network migration to LTE. Apart from SIM card management, ASTRID also provides a VPN program to its users to guarantee confidentiality, with public safety applications that depend on the requirements of the public safety organization and cloud services. BLM has been a temporary solution for 5–10 years. However, its service is still ongoing, and due to spectrum limitations, it proves itself as a viable final solution.
5.5 Nordic countries
The agreement between the three Nordic nations of Norway, Sweden, and Finland to synchronize their PSNs to develop universal technology solutions across national boundaries indicates that they will go in the same direction. Finland designated the roadmap consisting of five steps in the broadband PSN project called VIRVE 2.0 (Stojkovic, 2016). Norway and Sweden are expected to pursue these steps and develop their PSNs, and consequently, they have an agreement on harmonizing their PSNs. Finnish TETRA operator VIRVE will build and use a hybrid system consisting of a GO-GO model private and commercial LTE network. The private network has CN and RAN parts and cooperates with the commercial LTE network. The roadmap of Finland is determined to achieve maximum efficiency from the legacy TETRA network, which has a high cost and wide coverage, while transitioning to LTE-based PSN.
Nødnett is a private radio network designed only for PPDR users that adheres to the TETRA standard. Before the countrywide establishment of Nødnett in December 2015, there was no private national PSN in Norway and a variety of alternative systems were instead utilized by the PPDR groups (Stojkovic, 2016). The Emergency Communications Directorate (Norwegian: Direktoratet for nødkommunikasjon) manages the government-owned Nødnett network. For the Ministry of Justice, DNK is in charge of building, managing, and deploying the network; in other words, the network is contractor-operated as the GO-CO model. The network deployment took 9 years and required a huge investment (6,560 million Norwegian krone = 690 million USD) (Stojkovic, 2016). Deploying a specialized LTE network is the first option for switching from TETRA Nødnett to LTE Nødnett. In Norway, creating a brand-new national dedicated PSN LTE from scratch when the dedicated TETRA network has just been completed is unlikely since it would require yet another huge expenditure. A more cost-effective way is to execute a gradual transition and eventually convert the existing TETRA network to an LTE network. As a matter of fact, around 70% of any mobile network cost is RAN (Ferrus and Sallent, 2015). Moreover, most of the expenses are RAN expenses of the real estate site (rented or acquired) rather than that of radio and transmission equipment. Thus, the existing TETRA cell sites can be reused to significantly reduce costs. As mentioned previously, the frequencies of CEPT given for the PSN have shortcomings and problems. Therefore, the PSN will use the LTE network spectrum of the agreed operators in Finland. Thus, VIRVE 2.0 has no dedicated spectrum (Holen, 2019).
The migration between different distribution solutions can be smooth. First, mission-critical broadband services only depend on commercial networks (as in MVNO). Having LTE RANs and MMEs that share with commercial networks (as in GWCN) is the second phase. Following that PSNs will move such that they only share a portion of their LTE RAN with business operators (as in MORAN or MOCN). The ultimate goal would be to separate the PSN domain by developing unique LTE-based PSNs that completely fulfill the stringent PS criteria and the operation’s mission-critical standards (Jarwan et al., 2019).
We will examine the roadmap of the transition of Finland PSN to VIRVE 2.0 LTE PSN network similar to approaches in Jarwan et al. (2019). Figure 6A shows the current status of the standard TETRA network which provides PPDR service with dedicated RAN and CN parts. The first step is building a MVNO to meet the increasing daily data requirements, as shown in Figure 6B. The subscriber and service delivery systems will be expanded to support user authorization on a broadband network. Initially, an authority may utilize SIM cards acquired outside the country (similar to the Belgium arrangement). However, eventually, the goal will be to have subscribers in the LTE core and govern them. Currently, the TETRA network and services are still used as usual. The subscribers’ database HSS resides in the commercial operator’s CN. Exemplary, if more than one operator provides services, each will have their individual HSS for the same user/subscriber group. Commercial operators are responsible for performing operational subscriber management. The TETRA network and services are still in operation. The subscribers’ database or HSS is stored in the CN of the commercial operator. Exemplary, if there are many operators offering services, each will have a separate HSS for the same user or subscriber group. The task of operational subscriber management falls to commercial operators. PSN users can establish a connection to the preferred network using unique SIM cards to use commercial LTE network services. Thus, critical users can be distinguished from commercial users with these SIM cards, and they can perform roaming to the different operator networks.
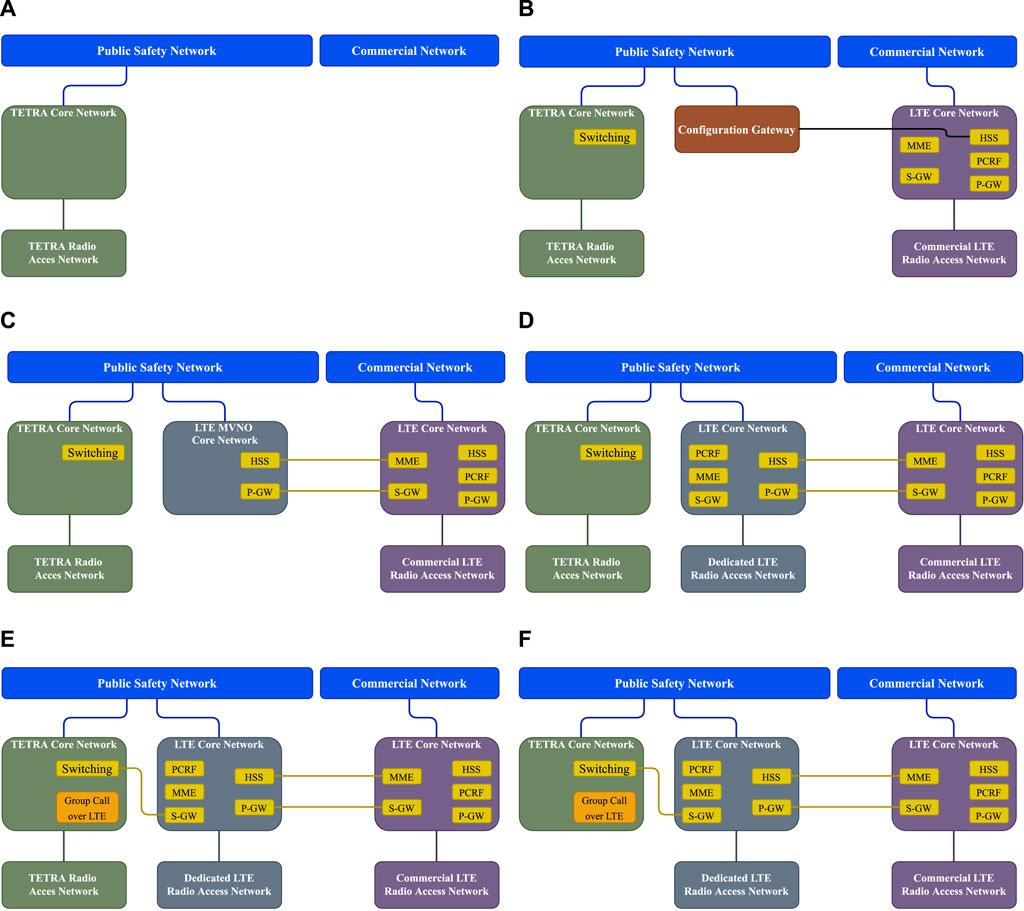
FIGURE 6. Finland roadmap to broadband PSN. (A) Legacy system TETRA. (B) TETRA and non-mission critical commercial broadband data. (C) TETRA and LTE MVNO core. (D) TETRA and dedicated LTE and commercial LTE. (E) TETRA and hybrid LTE. (F) TETRA core and hybrid LTE.
As seen in Figure 6C, the second phase involves running important voice and message traffic on the narrowband TETRA network and high-speed, non-mission critical and secure data traffic on the commercial broadband network. The same services and procedures as in the first phase are used here to give services to users. However, private LTE networks no longer require numerous subscriber databases because they now have a single HSS database at their core. This mini LTE core becomes the user’s home network by roaming the users to the commercial network. On the top of that, the MVNO provides a comfortable environment for migrating from one commercial operator to another, as private and commercial LTE does not require the entire database to be migrated. In this way, it becomes responsible for performing operational subscriber management (Cox, 2012). In this step, a partial core network is generated, and the construction of the dedicated LTE core part begins by switching LTE mini core from “Light MVNO” to “Full MVNO.”
The third step is to expand the individual dedicated LTE core and build a private owned LTE RAN to selected locations to complement the coverage of commercial operators as shown in Figure 6D. In this instance, phone service is still offered through the TETRA network, but mission-critical data services are now delivered over a private fourth generation (4G) network. As already indicated, the end objective of this transition process is to establish a combination of private and commercial 4G networks. The complete 4G core, which includes all the standard 4G core components and the 4G RAN, has replaced the MVNO’s original 4G mini core. As a result, the MVNO is changed into a private MNO that may now function independently. Private 4G radio access is only possible in areas where commercial 4G networks do not offer coverage. As a result, 4G networks will continue to provide standard interconnection and interoperability across private and commercial networks.
The fourth phase, as shown in Figure 6E, demonstrates the viability of crucial voice-over 4G standardization and the manufacturer’s support for group call-over 4G functionality on the TETRA side. The time has come to link the TETRA and 4G networks so that 4G devices may access additional TETRA services across 4G networks. Both broadband and narrowband networks offer the same phone services. Additionally, the private 4G network offers both mission-critical phone and data services, while voice services are still accessible on the TETRA network and may be utilized as a backup when utilizing commercial 4G networks for coverage. These modifications should enable the TETRA network to implement 4G functionalities on the TETRA side, among other potential demands.
After fulfilling the requirements for the PSN as shown in Figure 6F, step five involves removing TETRA radio access by ensuring the broadband service’s availability and dependability. TETRA voice services are no longer required as a backup since 4G mission-critical services are now developed sufficiently to replace TETRA RAN. The commercial 4G radio access is utilized as previously, while the private 4G network offers all services. The inter-systems interface of the TETRA network will allow devices connected to the 4G network to utilize the group calling service it offers. The inter-systems interface allows LTE devices to communicate with TETRA devices.
6 Coexistence
Section 5 explains the South Korean PSBN deployment policy “All Four One”. This policy consists of interworking backward compatible four networks to provide an economical PSBN deployment. The defining feature of this design is the utilization of the same 10 × 2 MHz spectrum to serve all users in the network. However, with the nationwide coverage requirement, this design leads to co-channel interference between users of different networks. Therefore, interference management shows up as the dominant problem of this design. Out of these four networks, the commercial LTE networks are not allowed to use the public safety spectrum. Therefore, the co-channel interference problem relies upon PS-LTE, LTE-R, and LTE-M networks.
The coexistence of PS-LTE, LTE-R, and LTE-M is quite a unique problem in their operational scenarios. The PS-LTE network is required to have nationwide coverage. However, the LTE-R network is required to cover train tracks, and LTE-M is required to cover the seas. These geographical coverage requirements lead up to a unique scenario for each network. Features of these coverage scenarios can be exploited for interference management solutions. Moreover, the interworking of these networks can be extended so that RAN or CN sharing is made possible between them. In this structure, the interference from the PS-LTE structure will be the limiting factor for others due to its geographical coverage features. In line with this, we have categorized the related works into three categories, as seen in Table 6. Table 6 shows the possible coexistence scenarios, and related field test results and solution mechanisms developed so far.
Coexistence between PS-LTE and LTE-R is the first category in Table 6. This coexistence scenario is critical since possible communication outages can cause huge disasters for both networks. In an emergency near train tracks, interference from the LTE-R network can disrupt PS-LTE, or a possible PS-LTE interference to the LTE-R network can cause huge train accidents due to an outage in train signaling. Overall challenges for PS-LTE and LTE-R coexistence have been addressed by Choi et al. (2015). The main problem of this coexistence relies on strict reliability requirements in LTE-R, which has to be satisfied under constant interference from the PS-LTE. Won and Kim (2017) introduced performance changes and a link budget analysis method from the obtained field test results in Korea. As concluded in the previous work, this interference from the PS-LTE must be addressed to provide a stable railroad wireless communication network. In line with this, Ahmad et al. (2017, 2020), Chen et al. (2017), and Um et al. (2020) have analyzed and proposed solutions for this problem. Ahmad et al. (2017) and Chen et al. (2017)proposed inter-cell interference coordination (ICIC) schemes that utilize coordinated scheduling (CS) in coordinated multipoint (CoMP) networks. Ahmad et al. (2020) proposed a mobile personal cell deployment mechanism depending on public safety situations that can provide priority-based load balance and interference management. Finally, a measurement-based spectrum sharing mechanism has been proposed by Um et al. (2020) that is done without a coordinator.
The second scenario covered in Table 6 is PS-LTE and LTE-M coexistence. In this scenario, the interference problem is similar to the PS-LTE and LTE-R coexistence. However, the design of PS-LTE coverage has been limited to 20 km offshore. Due to this, interference decreases as ships sail away (Jo et al., 2018). Moreover, Song et al. (2019) have proposed a RAN-sharing mechanism between PS-LTE and LTE-M networks to reduce interference and run field tests with excellent results. Another problem in this system is the initial access procedures of UE. For this problem, a mission-critical user priority–based random access scheme was proposed by Ahmad and Chang (2019). Finally, Ahmad and Chang (2020) considered a CS CoMP network, which consists of all three PSNs to develop an ICIC solution that allows all three networks to coexist with each other. However, no field tests have been conducted considering all three networks simultaneously.
“All Four One” is a South Korean deployment strategy that can be used in other countries to deploy their private PSBN solutions economically. However, the same design policy can be used to deploy only LTE-R networks that coexist with commercial networks. Coexistence research for LTE-R networks can allow efficient spectrum usage and more feasible national spectrum allocations. The main idea is that networks like LTE-R and LTE-M, which only utilize the spectrum over a specific coverage area, lead to inefficient spectrum usage. Thus, developing mechanisms that allow spectrum sharing between specific and more extensive coverage networks can open up various opportunities.
7 Challenges and future research
PSBN deployment fundamentally has three main challenges. We can summarize these challenges as limited bandwidth, geographical coverage, and economic challenges. As discussed in Section 5, most countries have allocated 2 × 10 MHz of spectrum for PSBN applications. This allocation is insufficient considering the use-cases for PSNs. Section 5 mentions some of the solutions developed by advanced PSN countries. However, these solutions mostly rely on network deployment and partnerships with commercial networks. Limited bandwidth problems can also be solved by developing bandwidth-efficient transmission schemes. From this aspect, even though partnership solutions are effective, there is a huge gap in the literature about PSBN centric physical layer design. PSBNs have unique deployment scenarios as mentioned in Section 6. These scenarios should be exploited to develop smart transmission schemes specific for PSBNs. Some open research areas involving this aspect include, but are not limited to, scenario-specific waveform design (especially for railway systems), multiple access schemes, and physical layer design-based coexistence. Moreover, there is a lot of potential to exploit specific multiple antenna structures for creative solutions. Some great examples from the literature use frequency diverse arrays (FDAs) to provide clever modulation and transmission schemes for specific scenarios (Nusenu and Wang, 2018; Wu et al., 2021). We believe that existing and future MIMO technologies such as FDAs and near-field MIMO have to be explored further to provide PSBNs with scenario centric solutions.
Geographical coverage has been introduced previously in the literature for network deployment. For example, narrowband PSNs have to provide a certain amount of geographical coverage depending on country regulations and network use-case. However, broadband networks generally consider a percentile population coverage instead of geographical coverage by country regulations. As a result, providing country-wise coverage with a broadband network requires operators to invest in areas with low populations and have no return. Due to the concept of population coverage, instead of a geographical coverage analysis, broadband networks usually depend on a traffic and capacity analysis. On the other hand, PSBNs have to provide significant capacity and country-wise coverage. As a result, PSBN deployment faces a dilemma. Geographical coverage requirement for PSBNs presents a trade-off problem in the deployment stage. Planning a deployment with a large capacity requires more site deployments. Thus, a large capacity network deployment with complete geographical coverage results in enormous deployment and operational costs. Moreover, traffic analysis techniques used for capacity prediction are insufficient for PSBN since traffic can be highly volatile depending on the emergency. Thus, future PSBN deployments can benefit from traffic analysis methods that are more suitable for public safety users and possible deployment methods that deal with the mentioned capacity-coverage trade-off problem. South Korea’s “All Four One” deployment scenario is an excellent example of solving the capacity-coverage trade-off problem.
Economic challenges, especially for developing countries, can be investigated under two stages. The deployment stage is the first stage of PSBNs, and the most drastic limiting factor is the coverage-capacity trade-off. Previous passages have already mentioned this problem and possible solutions. However, we can summarize possible solutions such as increasing bandwidth efficiency, better traffic analysis, and new deployment techniques. In the second stage, operating costs become a massive problem for public safety operators after deployment. If a country can afford its PSBN, this is not a huge problem. It is just a matter of planning. However, a better solution is to create a business model that can make public safety operators at least self-sufficient. An example of this is FirstNet’s business model. Possible partnerships between commercial operators and the government turn this problem into an interesting research topic. Commercial operators have a lot of available bandwidth and an enormous network, while the government has concessions to offer to their partners. These concessions, which depend on the partnership scenario, may yield drastic advantages for the operator over their competitors. Thus, research on existing and future government–commercial partnerships is necessary to keep a healthy telecommunication market.
8 Conclusion
PSNs have garnered an increasingly important role in today’s society. While these networks have conventionally been supported with narrowband systems, there has been a push in recent years toward broadband communication systems for PPDR applications. The general trend in this regard is the adaption of LTE technologies; however, the migration decision has innumerable critical factors that include the architecture, spectrum allocation, network deployment/dimensioning, service provisioning, operation, and management. Till date, any tutorial detailing with these issues, along with case studies of different countries that have already had successful migration has been lacking from the literature. In this work, we provide a comprehensive overview of infrastructure-sharing schemes for LTE-based PSN networks. Also, deployment scenarios are examined for PSN-dedicated networks using LTE over commercial operators and hybrid solution methods. Subsequently, we delve into an exploration of infrastructure-sharing mechanisms from the PSN standpoint. Following this, we address coverage and capacity dimensioning, which is pivotal in determining the number of sites that are required for a comprehensive cost analysis. Then, we present a study case for the cost analysis, which serves as a guide in this endeavor. Key countries migrating existing PSNs to LTE-based PSNs are introduced in detail. Finally, the coexistence schemes of PS-LTE, LTE-R, and LTE-M are explained. When deploying broadband PSN, the infrastructure sharing mechanisms can be implemented along with coexistence schemes, taking into account the availability of spectrum, compatibility of spectrum with existing commercial network equipment, and the case study methods.
Author contributions
AS, TY, AA, EM, and HA contributed to the conception and design of the study. AS and TY wrote the first draft of the manuscript. AS, TY, AA, and EM wrote sections of the manuscript. All authors contributed to the article and approved the submitted version.
Acknowledgments
The authors would like to thank Muhammad Sohaib J. Solaija for his valuable comments and suggestions to improve the quality of the paper.
Conflict of interest
Author AA was employed by company Karel Electronics.
The remaining authors declare that the research was conducted in the absence of any commercial or financial relationships that could be construed as a potential conflict of interest.
Publisher’s note
All claims expressed in this article are solely those of the authors and do not necessarily represent those of their affiliated organizations, or those of the publisher, the editors, and the reviewers. Any product that may be evaluated in this article, or claim that may be made by its manufacturer, is not guaranteed or endorsed by the publisher.
References
3GPP (2011). Study on impacts on signalling between User Equipment (UE) and core network from energy saving (Release 11). Tech. Specif. 24, 826. ver 11.0.0.
3GPP (2013). Feasibility study for proximity services (ProSe) (Release 12). Tech. Specif. 22, 803. ver 12.2.0.
3GPP (2014a). Study on system enhancements for user plane congestion management (Release 13). Tech. Specif. 23, 705. ver 13.0.0.
3GPP (2014b). Evolved universal terrestrial radio access network (E-UTRAN); study on RAN sharing enhancements (Release 12). Tech. Specif. 36, 856. ver 12.0.0.
3GPP (2017). Study on new radio access technology: radio access architecture and interfaces. Tech. Specif. 38, 801. ver 14.0.0.
3GPP (2018). Report on technical options and conclusions (Release 8). Tech. Specif. 23 882. ver 8.0.0.
3GPP (2022a). Evolved universal terrestrial radio access (E-UTRA) and evolved universal terrestrial radio access network (E-UTRAN); overall description; stage 2 (Release 17). Tech. Specif. 36 300. ver 17.0.0.
3GPP (2022b). Feasibility study for evolved universal terrestrial radio access (UTRA) and universal terrestrial radio access network (UTRAN) (Release 17). Tech. Specif. 25 912. ver 17.0.0.
3GPP (2022c). Network sharing; architecture and functional description (Release 17). Tech. Specif. 23 251. ver 17.0.0.
3GPP (2022d). Proximity-based services (ProSe); stage 2 (Release 17). Tech. Specif. 22 303. ver 17.0.0.
Abebe, G. (2019). “4G LTE radio network dimensioning in case of adama city,” (ASTU). Master’s thesis.
Afolabi, I., Taleb, T., Samdanis, K., Ksentini, A., and Flinck, H. (2018). Network slicing and softwarization: A survey on principles, enabling technologies, and solutions. IEEE Commun. Surv. Tutorials 20, 2429–2453. doi:10.1109/COMST.2018.2815638
Ahmad, I., and Chang, K. (2019). Mission critical user priority-based random access scheme for collision resolution in coexisting PS-LTE and LTE-M networks. IEEE Access 7, 115505–115517. doi:10.1109/access.2019.2934778
Ahmad, I., and Chang, K. (2020). Mission-critical user priority-based cooperative resource allocation schemes for multi-layer next-generation public safety networks. Phys. Commun. 38, 100926. doi:10.1016/j.phycom.2019.100926
Ahmad, I., Chen, W., and Chang, K. (2017). LTE-railway user priority-based cooperative resource allocation schemes for coexisting public safety and railway networks. IEEE Access 5, 7985–8000. doi:10.1109/access.2017.2698098
Ahmad, I., Jang, J., and Chang, K. (2020). QoS priority-based mobile personal cell deployment with load balancing for interference reduction between users on coexisting public safety and railway LTE networks. Electronics 9, 2136. doi:10.3390/electronics9122136
Ali, K., Nguyen, H. X., Vien, Q.-T., Shah, P., Raza, M., Paranthaman, V. V., et al. (2021). Review and implementation of resilient public safety networks: 5G, IoT, and emerging technologies. IEEE Netw. 35, 18–25. doi:10.1109/mnet.011.2000418
Baldini, G., Karanasios, S., Allen, D., and Vergari, F. (2014). Survey of wireless communication technologies for public safety. IEEE Commun. Surv. Tutorials 16, 619–641. doi:10.1109/SURV.2013.082713.00034
Borkar, S., Roberson, D., and Zdunek, K. (2011). “Priority access for public safety on shared commercial LTE networks,” in 2011 Technical Symposium at ITU Telecom World (ITU WT), Geneva, Switzerland, 24-27 October 2011, 105–110.
Bousia, A., Kartsakli, E., Antonopoulos, A., Alonso, L., and Verikoukis, C. (2016). Game-theoretic infrastructure sharing in multioperator cellular networks. IEEE Trans. Veh. Technol. 65, 3326–3341. doi:10.1109/TVT.2015.2445837
Cano, L., Capone, A., Carello, G., Cesana, M., and Passacantando, M. (2017). On optimal infrastructure sharing strategies in mobile radio networks. IEEE Trans. Wirel. Commun. 16, 3003–3016. doi:10.1109/TWC.2017.2673841
Cano, L., Capone, A., and Sansò, B. (2020). On the evolution of infrastructure sharing in mobile networks: A survey. ITU J. Future Evol. Technol. 1, 21.
Cerwin, S. (2019). Radio Propagation and Antennas - a non-mathematical treatment of radio and antennas (AuthorHouse).
Chan, H. A., Yokota, H., Xie, J., Seite, P., and Liu, D. (2011). Distributed and dynamic mobility management in mobile internet: current approaches and issues. JCM 6, 4–15. doi:10.4304/jcm.6.1.4-15
Chanie, W. (2020). “LTE radio access network dimensioning by particle swarm optimization,” (AAU). Master’s thesis.
Chaudhry, A. U., and Hafez, R. H. (2019). “LMR and LTE for public safety in 700 MHz spectrum,” in Wireless communications and mobile computing 2019, Tangier, Morocco, 24-28 June 2019, 1–17.
Checko, A., Ellegaard, L., and Berger, M. (2012). “Capacity planning for Carrier Ethernet LTE backhaul networks,” in 2012 IEEE Wireless Communications and Networking Conference (WCNC), Paris, France, 1-4 April 2012 (IEEE), 2741–2745.
Chen, W., Ahmad, I., and Chang, K. (2017). Co-channel interference management using eICIC/FeICIC with coordinated scheduling for the coexistence of PS-LTE and LTE-R networks. EURASIP J. Wirel. Commun. Netw. 2017, 34–14. doi:10.1186/s13638-017-0822-6
Chochliouros, I. P., Spiliopoulou, A. S., Lazaridis, P. I., Zaharis, Z. D., Spada, M.-R., Pérez-Romero, J., et al. (2021). 5G for the support of public safety services. Wirel. Personal. Commun. 120, 2321–2348. doi:10.1007/s11277-021-08473-5
Choi, J.-K., Cho, H., Oh, H.-S., Kim, K.-H., Bhang, M.-J., Yu, I.-S., et al. (2015). “Challenges of LTE high-speed railway network to coexist with LTE public safety network,” in 2015 17th International Conference on Advanced Communication Technology (ICACT), Phoenix Park, PyeongChang, 1-3 July 2015 (IEEE), 543–547.
Choi, K., and Kim, M. (2015). Gukminanjeon hwakboreul wihan gingeuptongsinmangsiseutem tonghapguchuk bangan [a plan to establish an integrated emergency communication network system to secure public safety]. Hangukgyeonghogyeongbihakhoeji J. Korean Secur. Guard Soc., 169–198.
Cox, C. (2012). An introduction to LTE: LTE, LTE-advanced, SAE and 4G mobile communications. New Jersey, United States: John Wiley and Sons.
Doumi, T., Dolan, M. F., Tatesh, S., Casati, A., Tsirtsis, G., Anchan, K., et al. (2013). LTE for public safety networks. IEEE Commun. Mag. 51, 106–112. doi:10.1109/mcom.2013.6461193
Fantacci, R., Gei, F., Marabissi, D., and Micciullo, L. (2016). Public safety networks evolution toward broadband: sharing infrastructures and spectrum with commercial systems. IEEE Commun. Mag. 54, 24–30. doi:10.1109/MCOM.2016.7452262
Favraud, R., Apostolaras, A., Nikaein, N., and Korakis, T. (2016). Toward moving public safety networks. IEEE Commun. Mag. 54, 14–20. doi:10.1109/mcom.2016.7432142
Fcc, (2012). Implementing public safety broadband provisions of the middle class tax relief and job creation act of 2012. final rule. Fed. Regist. 77, 62461–62463.
Feng, W., Li, Y., Jin, D., Su, L., and Chen, S. (2016). Millimetre-wave backhaul for 5g networks: challenges and solutions. Sensors 16, 892. doi:10.3390/s16060892
Ferrus, R., Pisz, R., Sallent, O., and Baldini, G. (2013a). Public safety mobile broadband: A techno-economic perspective. IEEE Veh. Technol. Mag. 8, 28–36. doi:10.1109/MVT.2013.2252273
Ferrus, R., Sallent, O., Baldini, G., and Goratti, L. (2013b). Lte: the technology driver for future public safety communications. IEEE Commun. Mag. 51, 154–161. doi:10.1109/mcom.2013.6619579
Ferrus, R., Sallent, O., Baldini, G., and Goratti, L. (2012). Public safety communications: enhancement through cognitive radio and spectrum sharing principles. IEEE Veh. Technol. Mag. 7, 54–61. doi:10.1109/mvt.2012.2190180
Ferrus, R., and Sallent, O. (2015). Mobile broadband communications for public safety: The road ahead through LTE technology. New Jersey, United States: John Wiley and Sons.
Foukas, X., Patounas, G., Elmokashfi, A., and Marina, M. K. (2017). Network slicing in 5G: survey and challenges. IEEE Commun. Mag. 55, 94–100. doi:10.1109/MCOM.2017.1600951
Frisanco, T., Tafertshofer, P., Lurin, P., and Ang, R. (2008). “Infrastructure sharing and shared operations for mobile network operators from a deployment and operations view,” in NOMS 2008 - 2008 IEEE Network Operations and Management Symposium, Salvador, 7-11 April 2008, 129–136.
Gallagher, J. C. (2018). “The first responder network (firstnet) and next-generation communications for public safety: issues for congress,” in CRS report for congress, 1–27.
General, A. (2016). “Upgrading emergency service communications: the emergency services network,”. Tech. rep.
Hallahan, R., and Peha, J. (2013). Enabling public safety priority use of commercial wireless networks. Homel. Secur. Aff. 9.
Hallahan, R., and Peha, J. M. (2011). The business case of a network that serves both public safety and commercial subscribers. Telecommun. Policy 35, 250–268. doi:10.1016/j.telpol.2010.12.006
Holen, S. (2019). “5G vertical user workshop national plans and roadmaps towards broadband,” in Public safety communication europe.
Jarwan, A., Sabbah, A., Ibnkahla, M., and Issa, O. (2019). LTE-based public safety networks: A survey. IEEE Commun. Surv. Tutorials 21, 1165–1187. doi:10.1109/COMST.2019.2895658
Jin, J., and Song, G. (2017). Bureaucratic accountability and disaster response: why did the korea coast guard fail in its rescue mission during the sewol ferry accident? Risk, Hazards Crisis Public Policy 8, 220–243. doi:10.1002/rhc3.12115
Jo, S.-W., Jang, J. H., Yu, S., and Shim, W. (2018). A validation of field test results for LTE-maritime. IFAC-PapersOnLine 51, 153–158. doi:10.1016/j.ifacol.2018.09.485
Kang, H.-J. (2015). A study on the public safety long term evolution disaster communication network. J. Digital Contents Soc. 16, 43–51. doi:10.9728/dcs.2015.16.1.43
Kasampalis, S., Lazaridis, P. I., Zaharis, Z. D., Bizopoulos, A., Zettas, S., and Cosmas, J. (2014). “Comparison of longley-rice, itu-r p. 1546 and hata-davidson propagation models for dvb-t coverage prediction,” in Bmsb, 1–4.
Kim, D. C. (2021). Korea safe-net status update. Available at: https://critcommsnetwork.com/videos/korea-safe-net-status-update.
Ksentini, A., and Nikaein, N. (2017). Toward enforcing network slicing on RAN: flexibility and resources abstraction. IEEE Commun. Mag. 55, 102–108. doi:10.1109/MCOM.2017.1601119
Kumbhar, A., Koohifar, F., Güvenç, , and Mueller, B. (2017). A survey on legacy and emerging technologies for public safety communications. IEEE Commun. Surv. Tutorials 19, 97–124. doi:10.1109/COMST.2016.2612223
Li, J., Nagalapur, K. K., Stare, E., Dwivedi, S., Ashraf, S. A., Eriksson, P.-E., et al. (2022). 5g new radio for public safety mission critical communications. IEEE Commun. Stand. Mag. 6, 48–55. doi:10.1109/mcomstd.0002.2100036
Longley, A. G. (1968). Prediction of tropospheric radio transmission loss over irregular terrain: A computer method-1968, 67. Boulder, Colorado: Institute for Telecommunication Sciences.
Marabissi, D., and Fantacci, R. (2017). Heterogeneous public safety network architecture based on RAN slicing. IEEE Access 5, 24668–24677. doi:10.1109/access.2017.2768800
Meddour, D.-E., Rasheed, T., and Gourhant, Y. (2011). On the role of infrastructure sharing for mobile network operators in emerging markets. Comput. Netw. 55, 1576–1591. Recent Advances in Network Convergence. doi:10.1016/j.comnet.2011.01.023
Nokia (2014). “Network Sharing: delivering mobile broadband more efficiently and at lower cost,”. Technical report.
NPSTC, (2014). “Defining public safety grade systems and facilities,” in A NPSTC public safety communications report.
Npstc, (2012a). “Local control in the nationwide public safety broadband network,” in NPSTC broadband working group local control task group.
Npstc, (2012b). “Public safety broadband high-level launch requirements statement of requirements for firstnet consideration,” in A NPSTC public safety communications report.
Nusenu, S. Y., and Wang, W.-Q. (2018). Range-dependent spatial modulation using frequency diverse array for OFDM wireless communications. IEEE Trans. Veh. Technol. 67, 10886–10895. doi:10.1109/tvt.2018.2870045
Oueis, J., Conan, V., Lavaux, D., Stanica, R., and Valois, F. (2017). Overview of LTE isolated E-UTRAN operation for public safety. IEEE Commun. Stand. Mag. 1, 98–105. doi:10.1109/mcomstd.2017.1600875
Park, M., and Hancocks, P. (2015). Sewol ferry disaster: One year on, grieving families demand answers.
Pérez, M. G., Celdrán, A. H., Clemente, F. J. G., and Pérez, G. M. (2020). “Review and open challenges of public safety networks to manage emergency settings in 5G,” in 2020 17th international conference on electrical engineering/electronics, computer, telecommunications and information technology (ECTI-CON), Phuket, Thailand, 24-27 June 2020 (IEEE), 555–558.
Rappaport, T. S., et al. (1996). Wireless communications: Principles and practice, 2. New Jersey: prentice hall PTR.
Rost, P., Mannweiler, C., Michalopoulos, D. S., Sartori, C., Sciancalepore, V., Sastry, N., et al. (2017). Network slicing to enable scalability and flexibility in 5G mobile networks. IEEE Commun. Mag. 55, 72–79. doi:10.1109/MCOM.2017.1600920
Seybold, A. (2010). A broadband network cost model: A basis for public funding essential to bringing nationwide interoperable communications to America’s first responders. OBI Technical Paper No. 2, FCC.
Sharma, T., Chehri, A., and Fortier, P. (2021). Review of optical and wireless backhaul networks and emerging trends of next generation 5G and 6G technologies. Trans. Emerg. Telecommun. Technol. 32, e4155. doi:10.1002/ett.4155
Sohul, M. M., Yao, M., Ma, X., Imana, E. Y., Marojevic, V., and Reed, J. H. (2016). Next generation public safety networks: A spectrum sharing approach. IEEE Commun. Mag. 54, 30–36. doi:10.1109/mcom.2016.7432144
Song, B., Lim, K., Lee, B., Cho, D., Lee, H., Jang, J., et al. (2019). Smart-navigation over pilot LTE-maritime: deployment and coexistence with ps-lte. IEEE Commun. Mag. 57, 126–131. doi:10.1109/mcom.2019.1800260
Stojkovic, M. (2016). “Public safety networks towards mission critical mobile broadband networks,” (NTNU). Master’s thesis.
Suomalainen, J., Julku, J., Vehkaperä, M., and Posti, H. (2021). Securing public safety communications on commercial and tactical 5G networks: A survey and future research directions. IEEE Open J. Commun. Soc. 2, 1590–1615. doi:10.1109/ojcoms.2021.3093529
Syed, A. B. (2009). “Dimensioning of LTE network:description of models and tool, coverage and capacity estimation of 3GPP long term evolution radio interface,” (TKK). Master’s thesis.
Telecom, K. (2022). KT telecom LTE coverage. Available at: https://nqi.kt.com/KTCVRG/coverage#.
Um, J., Kim, I., and Park, S. (2020). “Measurement based LTE resource block sharing for inter-operators: PS-LTE and LTE-R,” in 2020 International Conference on Information and Communication Technology Convergence (ICTC), Jeju Islan, Korea, October 21-23, 2020 (IEEE), 1092–1094.
Wake, D., Webster, M., Wimpenny, G., Beacham, K., and Crawford, L. (2004). “Radio over fiber for mobile communications,” in 2004 IEEE International Topical Meeting on Microwave Photonics (IEEE Cat. No.04EX859), Ogunquit, ME, USA, 04-06 October 2004, 157–160. doi:10.1109/MWP.2004.1396863
Won, H.-s., and Kim, S.-c. (2017). Lte-r linkbudget analysis for channels interference in the case of sharing the integrated public network frequency. J. Korea Inst. Inf. Commun. Eng. 21, 1833–1839.
Wu, X., Shao, H., Lin, J., Li, Q., and Shi, Q. (2021). High-speed user-centric beampattern synthesis via frequency diverse array. IEEE Trans. Signal Process. 69, 1226–1241. doi:10.1109/tsp.2021.3054988
Keywords: broadband, coexistence, core network, cost analysis, infrastructure sharing, network design, public safety network, radio access network
Citation: Sümer AS, Yılmaz T, Memisoglu E, Akkurt A and Arslan H (2023) Public safety network design for broadband wireless access. Front. Comms. Net 4:1065903. doi: 10.3389/frcmn.2023.1065903
Received: 10 October 2022; Accepted: 29 August 2023;
Published: 22 September 2023.
Edited by:
Pantelis Koutroumpis, University of Oxford, United KingdomReviewed by:
Dimitris Varoutas, National and Kapodistrian University of Athens, GreeceMichel Kadoch, École de technologie supérieure (ÉTS), Canada
Copyright © 2023 Sümer, Yılmaz, Memisoglu, Akkurt and Arslan. This is an open-access article distributed under the terms of the Creative Commons Attribution License (CC BY). The use, distribution or reproduction in other forums is permitted, provided the original author(s) and the copyright owner(s) are credited and that the original publication in this journal is cited, in accordance with accepted academic practice. No use, distribution or reproduction is permitted which does not comply with these terms.
*Correspondence: Ahmet Sacid Sümer, YWhtZXQuc3VtZXIxQG1lZGlwb2wuZWR1LnRy; Talha Yılmaz, dGFsaGF5aWxtYXpAYXNlbHNhbi5jb20udHI=
†These authors have contributed equally to this work and share first authorship