- 1Department of Biology, Wake Forest University, Winston-Salem, NC, United States
- 2Department of Biological Sciences, University of Manitoba, Winnipeg, MB, Canada
The pesticide fipronil is a GABA receptor antagonist that induces hyperexcitability in the insect nervous system. Its use is controversial because unintentional fipronil exposure may contribute to the ongoing global decline of pollinator populations. Sublethal doses of fipronil have been tentatively linked to reduced colony fitness and impaired learning in the western honey bee, Apis mellifera. The mushroom bodies, insect brain centers important for association learning and memory, contain fipronil-sensitive receptors and may therefore be directly affected by this pesticide. We investigated the synaptic organization of the mushroom bodies in worker honey bees exposed to fipronil using immunolabeling for a pre-synaptic marker and laser scanning confocal microscopy. Exposure of honey bee foragers to fipronil at a feasible field-realistic (1 ppb) concentration decreased the estimated density of immunolabeled microglomerular synaptic complexes in the lip and collar neuropil regions of the mushroom bodies. Effects were also evident after lower (0.1 ppb) and higher (4 ppb) exposures. Other indicators of synaptic organization (bouton number, surface area) were altered by exposure to fipronil in a dose-dependent fashion. These results indicate that sublethal doses of fipronil can result in atypical synaptic organization in the mushroom bodies of honey bees and suggest a mechanism by which fipronil, through perturbation of mushroom body-dependent functions, might diminish honey bee colony survival.
1 Introduction
Fipronil is a phenylpyrazole gamma-aminobutyric acid (GABA) receptor antagonist that causes hyperactivity, paralysis, and death in insects (Tingle et al., 2003). Fipronil also blocks insect glutamate-activated chloride (GluCl) channels (Zhao et al., 2004). Fipronil has been widely used since the 1990s to control insect pests; it is also widely used to control fleas on household pets (Tingle et al., 2003; Casida and Durkin, 2013). The popularity of fipronil arises from the higher potency of the compound at insect GABA receptors relative to vertebrates (Cole et al., 1993; Simon-Delso et al., 2015). This minimizes harms to pesticide applicators and consumers and reduces off-target risks to vertebrate wildlife.
Fipronil is absorbed by plant tissues, allowing insects that feed on any part of a plant to be targeted. Because fipronil is found in nectar, pollen, dust, and water droplets on soil and plant surfaces, non-target pollinators can be exposed as they forage (Desneux et al., 2007; Mahler et al., 2009; Bonmatin et al., 2015; Pisa et al., 2015). It is difficult to assess the magnitude of these exposures because levels of fipronil vary greatly in treated plants. Honey bees (Apis mellifera) risk exposure through visits to contaminated plants, ingestion of contaminated pollen and nectar, and contact with contaminated pollen and wax in their hives (e.g., Chauzat et al., 2006; Mullin et al., 2010; Wilmart et al., 2021). Honey bee exposures may be acute or repeated; their impact potentially varies by duration of exposure and life stage at which the exposure occurred. Often, exposures are significantly lower than both the contact and oral LD50s. For example, an analysis of pesticides in pollen in the northeastern United States reported that fipronil recovered from pollen was 0.56% of the contact LD50 and 0.80% of the oral LD50 for Apis mellifera (Stoner and Eitzer, 2013). Synergistic interactions of fipronil with other pesticides and xenobiotics such as fungicides are also possible (e.g., Zaluski et al., 2017) adding to the difficulty of determining risk under field conditions.
Researchers evaluating the sublethal impacts of fipronil on the nervous system and behavior of honey bees have relied primarily upon controlled exposures in the laboratory. Table 1 compiles results of published studies. This literature survey reveals that sublethal effects of fipronil are subtle, depend upon mode of exposure (ingestion or contact) and dose, and wane post-exposure (Decourtye et al., 2005; El Hassani et al., 2005; Aliouane et al., 2009; Bernadou et al., 2009; El Hassani et al., 2009; Decourtye et al., 2011; Nahar and Ohtani, 2015; Bovi et al., 2018).
Fipronil may also injure the insect brain and perturb behavior through pathways unrelated to antagonism of GABA receptors and glutamate chloride channels. Histological studies of the impact of fipronil on the brains of honey bees and stingless bees have revealed cause for concern. Persisting changes in brain cytochrome oxidase histochemistry were reported as a consequence of exposure of African-derived honey bees of various ages to fipronil (Roat et al., 2013). Histological analysis of the Kenyon cell populations of the mushroom bodies of the stingless bee Scaptotrigona postica revealed dose- and exposure-time dependent increases in pyknotic profiles in the brains of foragers treated either topically or orally with fipronil (Jacob et al., 2014). These results potentially reflect the action of fipronil as an uncoupler of oxidative phosphorylation in mitochondria, as demonstrated in vitro in a human neuronal cell line (Vidau et al., 2011).
The mushroom bodies of the insect brain are sensory integration centers required for performance of specific learning and memory tasks (Menzel, 2014; Devaud et al., 2015). Despite the centrality of the mushroom bodies to insect behavior, few studies have examined the effects of neuroactive pesticides on synaptic organization in this brain structure (Fahrbach and Van Nest, 2016). A hallmark of the mushroom bodies in honey bees (also demonstrated in other hymenopteran species) is age-based and experience-dependent neuroanatomical plasticity (Fahrbach, 2006), assessed by measurements of neuropil volume, dendritic branching, and/or density and number of synapses within the mushroom body neuropils (e.g., Withers et al., 1993; Durst et al., 1994; Farris et al., 2001; Kühn-Bühlmann and Wehner, 2006; Jones et al., 2009; Groh et al., 2012; Stieb et al., 2012; Muenz et al., 2015). Synapses in the mushroom body calyces (input neuropils) into microglomerular synaptic complexes (MCG) can be identified at the level of light microscopy using an antibody to synapsin, a vesicle-associated presynaptic protein (Fahrbach and Van Nest, 2016). Each MCG synaptic complex consists of a presynaptic bouton encircled by dendrites of the Kenyon cells, the intrinsic neurons of the mushroom bodies. The boutons are afferents from the antennal and optic lobes (Gronenberg, 2001). Formation of a long-term memory of an odor-reward pairing was associated with an increase in MCG density in the mushroom body lip neuropil of honey bees (Hourcade et al., 2010), while a negative correlation between MCG number and performance on an olfactory reversal learning task was reported in the lip neuropil of honey bees reared in an impoverished environment (Cabirol et al., 2017). It is therefore plausible that the synaptic organization of the mushroom bodies is reflected in the ability to perform specific learning and memory tasks.
In the present study, honey bee foragers were exposed to fipronil in the laboratory, and the mushroom body calyces were immunolabeled with anti-synapsin (also called anti-SYNORF1) to compare MCG in treated and untreated honey bees. We predicted that antagonism of GABA-signaling by fipronil – essentially, inhibition of inhibitory signaling – would mimic increased sensory input to the mushroom bodies associated with behavioral development in adult worker honey bees, a period of several weeks that spans the transition from hive work to foraging (Seeley, 1982). Because previous studies of the transition to foraging in the experience-rich environment of a field colony have been correlated with changes in density and MCG surface area (Groh et al., 2012; Muenz et al., 2015), we predicted comparable changes (decrease in density, increase in surface area) in young foragers exposed to fipronil. Given the demonstrated importance of GABA in olfactory association and reversal learning in the honey bee (Raccuglia and Mueller, 2013; Boitard et al., 2015), we predicted that effects would be evident in the lip (olfactory) region of the calyces. Despite a lack of pharmacological studies implicating GABA directly in visual learning in honey bees, we also examined the collar (visual) region of the calyces because both lip and collar are innervated by axons projecting from the GABAergic A3 mushroom body extrinsic neurons (Zwaka et al., 2018).
2 Materials and methods
2.1 Animals
Honey bees (Apis mellifera) from a hive with a naturally mated Italian stock queen were collected on the campus of Wake Forest University (Winston-Salem, NC, USA). All subjects were adult workers (non-reproductive females) of known age at time of treatment.
2.2 Fipronil
A stock solution of 1,000,000 ppb was created by dissolving 15 mg of fipronil (Sigma-Aldrich, St. Louis, MO, USA) in dimethyl sulfoxide (DMSO; MP Biomedicals, Solon, OH, USA). This initial stock solution was diluted with deionized water (diH2O) to generate a second stock solution at 1000 ppb. The second stock solution was incorporated into diH2O, pollen paste (4:1:4 ratio of pollen, honey, and water), and 1.0 M sucrose (30% w/w) to produce working concentrations of 0.1, 1, and 4 ppb fipronil (1 ppb = 1 µg/L). These treatments were designed to produce levels of contamination that were impactful but below an estimated acute LD50 of 4.2 ng fipronil/bee (Pisa et al., 2015). Previous studies assessing behavioral changes in honey bees exposed to fipronil treated individuals with a single dose of 0.01 to 1 ng/bee or with chronic feeding of fipronil in sucrose solution containing 2.2, 4.5, or 9 µg/L fipronil (see Table 1).
2.3 Exposure of foragers to fipronil
Combs of worker pupae were transferred from the hive into frame boxes and held overnight in a dark incubator (33°C). Age cohorts were defined by marking newly emerged bees with paint (Testors, Rockford, Illinois, USA) on the dorsal thorax prior to returning them to the field colony. Marked 15-day old workers were captured as young foragers returning to the hive entrance and placed into one of four treatment cages (10 cm x 8 cm x 10 cm), 40 bees per cage. Cages were maintained in an incubator (28°C, 40% relative humidity) in constant darkness. Mortality was checked and dead bees were removed daily. Feeding and cleaning tasks were performed at the same time each day under 650-nm red light (HQRP, Harrison, NJ, USA), which is not detected by honey bees (Peitsch et al., 1992). Three cages received the pollen paste, water, and sucrose containing 0.1, 1, and 4 ppb of fipronil, respectively. The fourth cage served as the control and received a 0-ppb concentration of the DMSO/water vehicle, sucrose, and pollen paste (no fipronil). Pollen paste and solutions were replaced daily. A sample of honey bees from each cage were collected for brain dissection after 4 and 12 days of treatment. The length of treatment was similar to durations used in previous studies that demonstrated impaired learning and memory as a result of exposure to imidacloprid and other pesticides (Decourtye et al., 2005; Zhang and Nieh, 2015). Because the honey bees spent 15 days in the hive prior to caging, ages at time of sampling were either 19 or 27 days, typical of young and experienced foragers, respectively.
2.4 Tissue processing
Bees were immobilized on ice and decapitated. Windows of approximately 3 mm per side were cut into the front of the head capsule. The exposed brains were immediately covered with chilled 4% formaldehyde (Thermo Fisher Scientific) diluted in 0.01 M phosphate-buffered saline (PBS), pH = 7.4. After 18-24 h in fixative (4°C), brains were removed from their head capsules, washed in PBS, embedded in low melting point agarose II (Amresco LLC, Solon, OH, USA), and stored in PBS at 4°C prior to sectioning.
To track sides of the brain, the right optic lobe of each brain was removed prior to embedding. This created a physical marker to ensure consistent analysis of the same side of the brain in each honey bee. Brains were sectioned in their entirety at 100-µm section thickness on a Compresstome VF-200 (Precisionary Instruments Incorporated, Greenville, NC, USA). Sections were immunolabeled with a monoclonal antibody raised in mouse, anti-SYNORF1 [DSHB Cat# 3C11 (anti-SYNORF1), RRID: AB_528479] by the following procedure. Sections were washed in PBS containing 0.2% Triton detergent (Triton X-100, Sigma-Aldrich, St. Louis, MO) and 2% normal goat serum (Jackson ImmunoResearch Laboratories, West Grove, PA, USA) prior to incubation with undiluted anti-SYNORF1 for 48 h at 4°C. After rinsing, sections were incubated at 4°C for 24 h in goat anti-mouse IgG Alexa Fluor 555 (Thermo Fisher Scientific Cat# A-21422, RRID: AB_2535844) diluted 1:500 in PBS with 1% normal goat serum, followed by washing and storage in PBS at 4°C. The binding specificity of anti-SYNORF-1 was initially characterized in Drosophila melanogaster (Klagges et al., 1996), and later demonstrated to recognize a motif conserved across Arthropoda (Hofbauer et al., 2009).
We aimed to analyze 8 brains/group, but in some cases tissue condition after processing did not permit a full neuroanatomical analysis. Final sample sizes for 4 days of treatment were as follows: 0 ppb, 6; 0.1 ppb, 8; 1 ppb, 8; 4 ppb, 3. Final sample sizes for 12 days of treatment were: 0 ppb, 8; 0.1 ppb, 8; 1 ppb, 7; 4 ppb, 8.
2.5 Confocal microscopy
Sections were mounted between No. 1.5 coverslips using a SecureSeal adhesive spacer (Electron Microscopy Sciences, Hatfield, PA, USA) and VECTASHIELD Hard Set mounting medium with DAPI (Vector Laboratories, Inc., Newark, CA, USA) and sealed with clear nail polish (Electron Microscopy Sciences, Hatfield, PA, USA) prior to imaging. All images were collected with a Zeiss LSM 710 confocal laser scanning microscope (Zeiss Microscopy, Oberkochen, Germany) with a Plan-Apochromat 20x 0.80 NA objective lens and Plan-Apochromat 63x 1.4 NA oil-immersion objective lens. Fluorescence was excited with the 561 nm laser line with a pinhole size slightly larger than 1 Airy unit to increase available light emission. Image acquisition was controlled using Zen Black software, Version 8.0 (ZEN Digital Imaging for Light Microscopy, RRID: SCR_013672). Using plane scan mode, 20x images of the left medial and left lateral calyces were captured with a resolution of 1024 (x) × 1024 (y) pixels with line averaging of 4 in every section in which these structures were visible. Scans at 63x within the lip and collar neuropils were captured with a focal depth of 1.2 µm, digital zoom of 1.6 and a resolution of 1024 (x) × 1024 (y) pixels with line averaging of 4. Images were acquired blind to the experimental treatment of the section being imaged; subsequent image analysis was also conducted blind to treatment.
2.6 Estimation of lip and dense collar volumes
Image files acquired with the 20x objective were opened in Fiji 5.1 (Fiji, RRID: SCR_002285). The boundaries of the lip and dense collar subregions were traced to determine cross-sectional areas of the enclosed spaces (Figure 1A). The area (µm2) was then multiplied by the brain section thickness (100 µm) to estimate section volume (µm3). Serial section volumes were summed to estimate total lip and dense collar volumes for the entire brain.
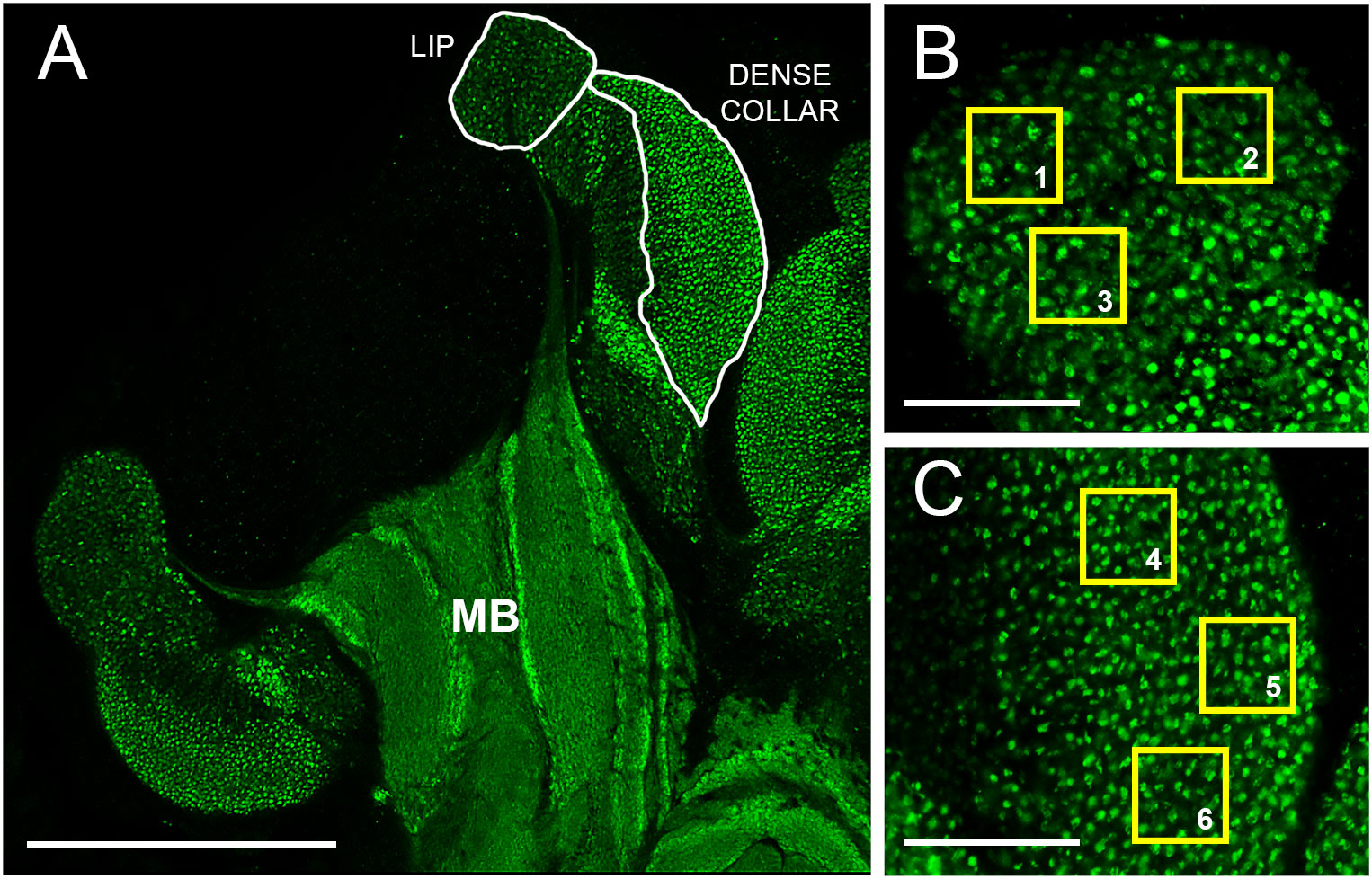
Figure 1 Overview of mushroom bodies and image analysis scheme. (A) Planar section of the left lateral calyx of an adult worker honey bee mushroom body (MB) immunolabeled with an antibody that recognizes synapsin, a synaptic vesicle-associated protein. Examples of lip and dense collar neuropils sampled for MCG density estimates as shown in Panels B and C are indicated (white borders) Scale bar represents 150 µm. (B) Higher magnification view of the lip region. Yellow squares (1-3) illustrate 20 x 20 µm non-overlapping regions of interest (ROIs) used for MCG density estimates. Green dots indicate MCG. Scale bar, 30 µm. (C) Higher magnification view of the dense collar region. Yellow squares (4-6) as in 1B. Scale bar, 30 µm.
2.7 Analysis of MCG density and surface area
To analyze MCG density and surface area in the mushroom bodies, we selected one central section per brain in which the medial and lateral mushroom body neuropils were fully defined (pedunculus and lobes visible in addition to calyces), sampling a consistent subset of the calyces visible in these sections (e.g., Muenz et al., 2015; Sommerlandt et al., 2016). In the present study, the left medial and lateral calyces were sampled. Image processing was performed with Imaris 7.7.2 (Imaris, RRID: SCR_007370). The 63x planar scans were used to produce an index of MCG density within the collected images by counting synapsin-positive profiles in regions of interest (ROIs) 20 µm × 20 µm (as in Stieb et al., 2010). ROIs were applied by the same observer to all images to lip and dense collar subregions within the left medial and lateral calyces: three per lip image (Figure 1B, ROI 1–3) and three per dense collar image (Figure 1C, ROI 4–6). Any ROIs that overlapped with previously acquired ROIs or extended beyond the borders of the lip or dense collar neuropils were rejected. Object surfaces were rendered with a smoothing factor of 0.165 µm and background subtraction of 0.6 µm. Because average MCG profiles are reported to range from ~1-4 µm2 (Groh et al., 2004), thresholding was applied uniformly across all images to reduce background noise and filter objects smaller than 0.35 µm2. After these adjustments, all objects within the ROIs were counted. Synaptic density measurements within the lip and collar were averaged separately within each subject. This procedure was repeated for individual MCG surface area measurements. To validate the Imaris-based analysis method, we performed manual counting of lip and collar MCG in optical sections from three brains and found manual counts to be within ±5% of the automated analyses (data not shown).
2.8 Estimation of MCG number
Total volume was multiplied by average MCG density to estimate the total number of MCG for the lip and dense collar on the left side of each brain analyzed (left lateral and medial calyces summed).
2.9 Statistical analysis
Two-way analysis of variance (treatment x duration of treatment) was used to examine the effect of fipronil exposure on the mushroom body calyces using log (1 + x) transformed data. The lip and the dense collar neuropil regions were analyzed separately. Data structure was assessed using the Shapiro-Wilk normality test and by examination of Q-Q plots. Post hoc contrasts for treatments were performed using Tukey’s multiple comparisons test with adjusted P values. Analyses were carried out with GraphPad Prism 9.5.1 (RRID : SCR_002798). Significance was set at α = 0.05. For ease of visualization, figures display untransformed data.
3 Results
3.1 Density of MCG in the lip neuropil
A two-way ANOVA was performed to analyze the effect of exposure to different concentrations of fipronil and different durations of exposure on the estimated density of MCG in the lip neuropil. Relative to age-matched, untreated controls, caged honey bee foragers exposed to fipronil for 4 and 12 days had altered MCG density in the lip neuropil (Figure 2A). There was a significant effect for concentration of fipronil, F(3, 48) = 23.9, P < 0.0001, accounting for 53.6% of the total variation, a significant effect for duration of exposure, F(1, 48) = 4.5, P = 0.04, and a significant interaction, F(3, 48) = 3.85, P = 0.015. Post hoc pairwise comparisons indicated that all concentrations of fipronil resulted in significant decreases in MCG density at 4 days relative to controls not exposed to fipronil (Table 2). A similar result was obtained for 12 days of exposure, with the exception of the highest concentration of fipronil (0 ppb vs. 4 ppb, Tukey’s multiple comparisons test adjusted P = 0.054).
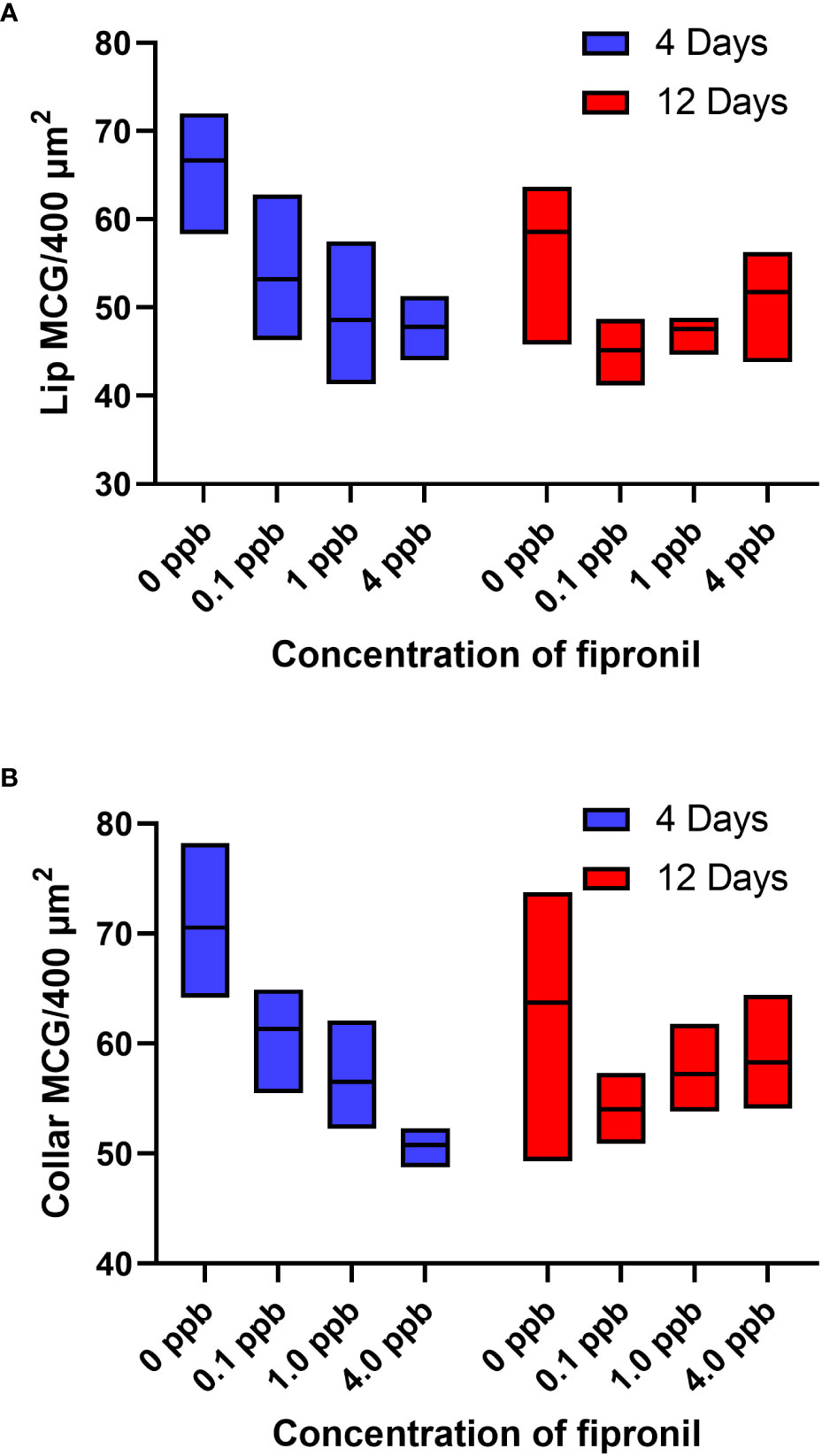
Figure 2 Estimated density of MCG in the lip and dense collar neuropils is altered in a dose and exposure duration-dependent manner by exposure to fipronil. Maximum and minimum scores indicated; line at mean. (A) Lip, MCG per 400 µm2. (B) Dense collar, MCG per 400 µm2. See Table 2 for post hoc comparisons that indicate that the longer exposure (12 days) were potentially less impactful in terms of MCG density in both lip and collar than a shorter exposure (4 days).
3.2 Density of MCG in the dense collar neuropil
There was a significant effect for concentration of fipronil, F(3, 48) = 15.4, P < 0.0001, accounting for 42.3% of the total variation, and a significant interaction effect, F(3, 48) = 6.5, P = < 0.0001. Duration of exposure to fipronil (4 days vs. 12 days) had no effect on density of MCG in the dense collar neuropil, F(1,48) = 0.93, P = 0.3. Post hoc pairwise comparisons indicated that all concentrations of fipronil resulted in significant decreases in MCG density in the collar at 4 days relative to controls not exposed to fipronil (Table 2; Figure 2B). Only the lowest concentration of fipronil produced a significant decrease in MCG density at 12 days (0 ppb vs 0.1 ppb, Tukey’s multiple comparisons test adjusted P = 0.0015).
3.3 Surface area of MCG in the lip neuropil
There was a significant effect for concentration of fipronil, F(3, 48) = 13.9, P <0.0001, accounting for 44.1% of the total variation. Duration of exposure to fipronil (4 days vs. 12 days) had no effect on surface area of MCG in the lip neuropil, F(1,48) = 1.5, P = 0.2, and there was no interaction effect, F(3,48) = 0.9, P = 0.4. Post hoc pairwise comparisons indicated that only the fipronil treatment of 1 ppb produced a significant increase in MCG surface area in the lip at 4 days (0 ppb vs 1 ppb, Tukey’s multiple comparisons test adjusted P < 0.01). All concentrations of fipronil resulted in significant increases in MCG surface area in the lip at 12 days relative to controls not exposed to fipronil (Table 3; Figure 3A).
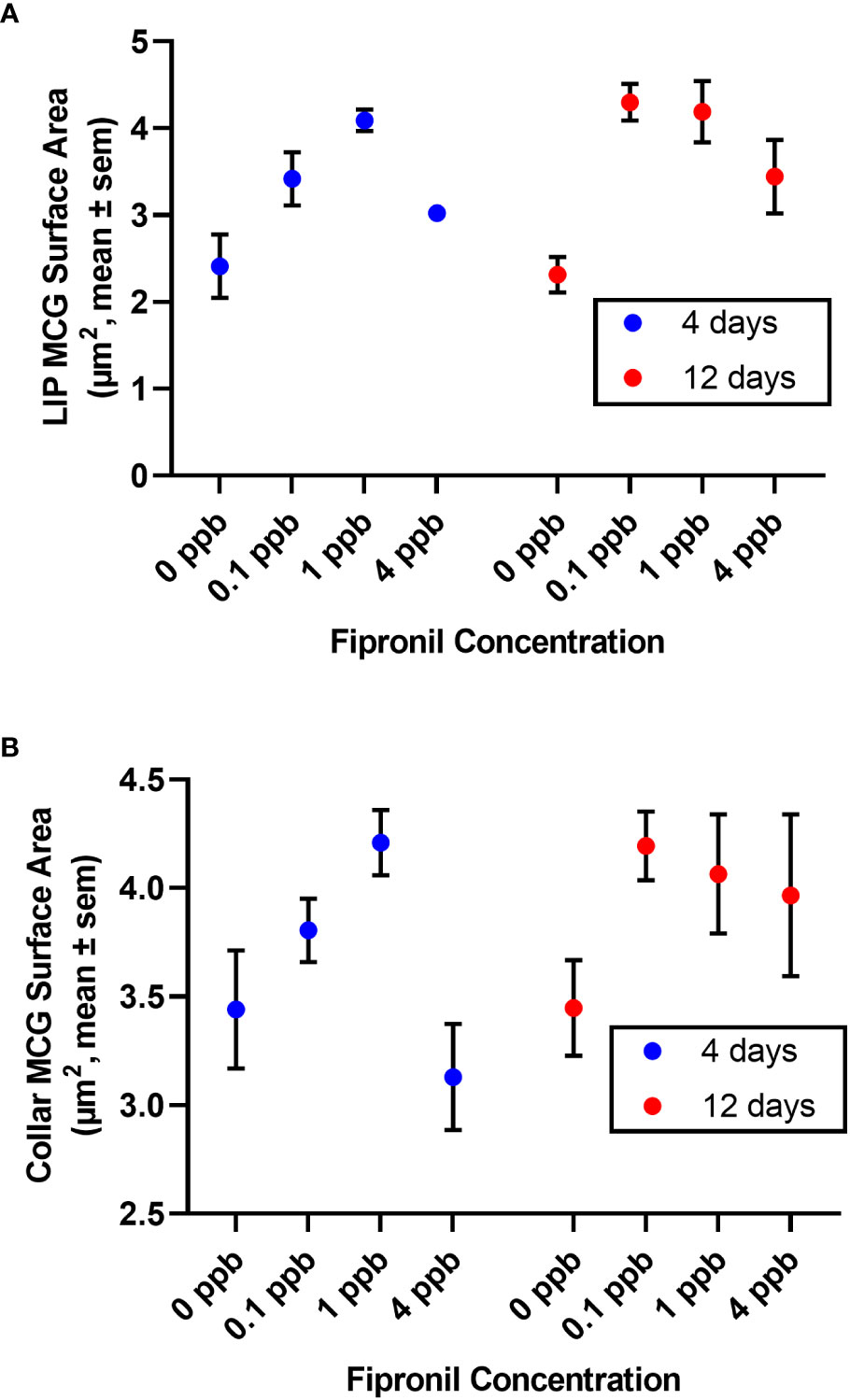
Figure 3 Surface area of terminal boutons in the mushroom body calyces was reduced in a dose and exposure duration-dependent manner by exposure to fipronil. Mean ± standard error of the mean. (A) Lip. (B) Dense collar. See Table 3 for post hoc comparisons indicating a greater sensitivity in the lip than the collar region of the mushroom body neuropil.
3.4 Surface area of MCG in the dense collar neuropil
There was a significant effect for concentration of fipronil, F(3, 48) = 3.8, P <0.02, accounting for 18.3% of the total variation. The duration of exposure to fipronil (4 days vs. 12 days) had no effect on surface area of MCG in the collar neuropil, F(1,48) = 1.8, P = 0.2, and there was no interaction effect, F(3,48) = 1.1, P = 0.35. Post hoc pairwise comparisons indicated that no concentration was more impactful at either 4 days or 12 days (Table 3; Figure 3B).
3.5 Volume of the lip and dense collar neuropils
A significant interaction effect accounted for 16.4% of the total variation in lip volume, F(3,48) = 3.3, P = 0.03, but this result is difficult to interpret in light of the lack of effects for fipronil concentration, F(3,48) = 1.3, P = 0.3, and duration of exposure, F(1,48) = 0.002, P = 0.96. No pairwise comparisons achieved statistical significance. No differences in dense collar volume were found for concentration of fipronil, F(3,48) = 1.6, P = 0.2 or for duration of exposure to fipronil, F(1,48) = 1.6, P = 0.2. There was no interaction effect of fipronil concentration and duration of exposure on dense collar volume, F(3,48) = 0.9825, P = 0.4.
3.6 Estimated total number of MCG in the lip and dense collar neuropils
For the lip, there was a significant effect for concentration of fipronil, F(3,48) = 8.3, P <0.0001, accounting for 30.4% of the total variation. The duration of exposure had no independent effect on estimated total lip MCG, F(1,48) = 0.6, P = 0.5. There was a significant interaction effect, F(3,48) = 3.6, P = 0.02, accounting for 13% of the total variance. Post hoc pairwise comparisons indicated that all concentrations of fipronil resulted in significant decreases in lip MCG number at 4 days relative to controls not exposed to fipronil (Figure 4A). At 12 days, only the 1 ppb dose had a significant effect on total lip MCG (0 ppb vs 1 ppb, Tukey’s multiple comparisons test adjusted P = 0.005). For the collar, there was a significant effect for duration of exposure, F(1,48) = 4.5, P = 0.04, accounting for 7.3% of the total variation. The concentration of fipronil had no independent effect on estimated total collar MCG, F(3,48) = 0.7, P = 0.6. There was a significant interaction effect, F(3,48) = 2.96, P = 0.04, accounting for 14.5% of the total variation. Post hoc pairwise comparisons did not yield any significant effects for total collar MCG (Figure 4B).
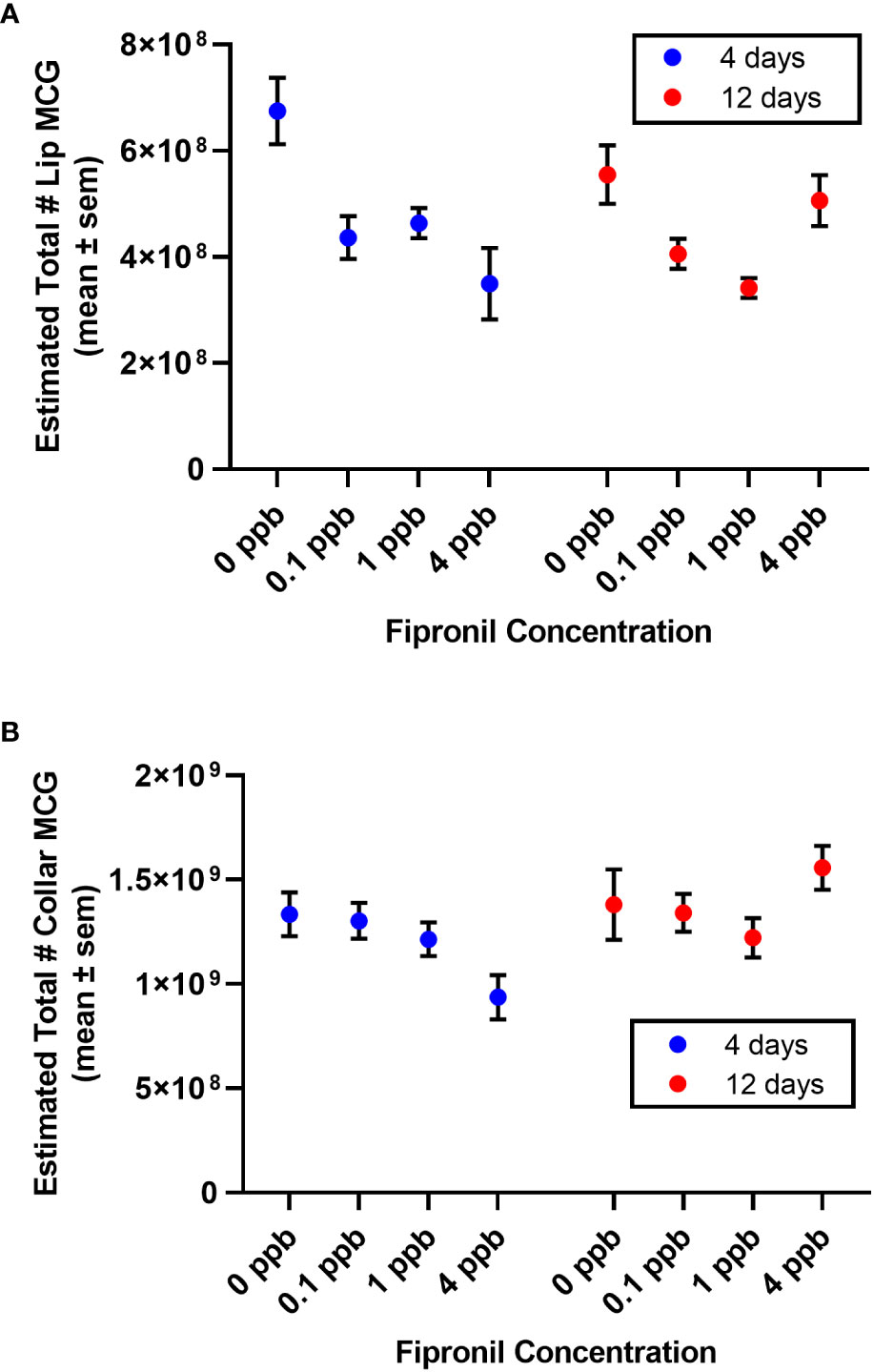
Figure 4 Estimated total number of MCG in the compartments of the mushroom body calyces. Mean ± standard error of the mean. (A) Lip. (B) Dense collar. Note an apparent trend for the 4 ppb concentration to be more impactful after a short exposure than a longer exposure (4 days vs. 12 days).
3.7 Mortality
Mortality of 3% was recorded within 0 ppb control cages after 4 days, and 6% at day 12. Cages receiving 0.1 ppb fipronil treatments experienced 0% mortality after 4 days, and 8% at day 12. Ten per cent mortality was recorded after 4 days of 1 ppb fipronil treatments, and 26% by day 12 treatments. The highest dose, 4 ppb fipronil, produced a mortality of 36% after 4 days of exposure and an 86% mortality at day 12.
4 Discussion
4.1 MCGs as a tool to detect subtle sublethal effects of pesticides on the central nervous system
MCG are plastic structures that change in the honey bee mushroom bodies in density and number with age (Muenz et al., 2015), experience (Krofczik et al., 2008; Groh et al., 2012), and olfactory association training (Hourcade et al., 2010), although the functional significance of these changes is currently poorly understood (Fahrbach and Van Nest, 2016; Cabirol et al., 2017). In this report, we demonstrated changes in an index of MCG studied in planar scans of the mushroom body calyces following exposure to sublethal doses of the pesticide fipronil. Our goal was not an in-depth analysis of synaptic structure in the mushroom body calyces but rather an attempt to develop a tool to assess the sensitivity of presynaptic elements to environmental contaminants as part of an effort to link impaired behavior with cellular mechanisms (Rigosi et al., 2022).
4.2 MCG density and surface area
In honey bees undergoing behavioral development in a field colony, density of MCG starts to decrease in both the lip and collar neuropils during the second week of adult life, resulting in a reduction of MCG density that continues through the course of maturation (Muenz et al., 2015). Here we report that, with fipronil exposure, MCG became sparser in the lip and collar regions relative to controls not exposed to fipronil.
The decreases in density were associated with increased MCG surface area in fipronil-treated bees. One interpretation of this observation is that individual boutons became larger as a result of exposure to fipronil. A similar correlation of density and size of MCG has been previously described for honey bees undergoing typical adult behavioral development (Groh et al., 2012). The observed changes in MCG density and surface area suggest that sublethal exposure to fipronil may accelerate trends evident during age-based development of the adult insect brain, possibly reflecting changes in the balance between synaptogenesis and pruning. Although it may seem counterintuitive that a pesticide would produce accelerated or precocious development, this effect is consistent with the model that experience-dependent development of the mushroom bodies in adult worker honey bees (and other hymenopteran insects) is dependent upon excitatory synaptic signaling in the calyces (Ismail et al., 2006; Dobrin et al., 2011; Cabirol et al., 2017). Blockade of GABA receptors and glutamate-gated chloride channels by fipronil is predicted to increase excitatory synaptic signaling in GABA-innervated structures.
4.3 Calycal volume and estimated number of MCG
Maturation in adult honey bees is coupled with a continuous experience-independent, age-related volume increase of the MB neuropils that plateaus around 15 days of age (e.g., Withers et al., 1993; Farris et al., 2001; Groh et al., 2012; Muenz et al., 2015), followed by a further period of growth linked with foraging experience (Farris et al., 2001; Ismail et al., 2006). In accord with earlier studies, our results showed no significant volume changes of the MB calyx between 19 and 27-day-old honey bees deprived of normal foraging experience during the period of fipronil exposure in laboratory cages. Despite this volume stability, the estimated total number of MCG decreased in the lip in response to 4 days of exposure to 0.1, 1, and 4 ppb fipronil and after 12 days of treatment with 1 ppb fipronil. Similar trends were observed at 4 and 12 days in the dense collar neuropil but post hoc pairwise comparisons did not reveal any significant effects, suggesting a greater sensitivity to fipronil in the lip versus the dense collar.
4.4 Responses to the highest dose of fipronil
Higher mortality characterized honey bees exposed to the highest dose (4 ppb) of fipronil, reducing the number of individuals available to sample for analysis, and impacting somewhat our confidence in results obtained from the high exposure cages. Survival in cages without food or water for more than two days is not possible for adult honey bees (Lorenz et al., 2001), eliminating the possibility that caged bees did not ingest fipronil. In contrast to the 0.1 and 1 ppb treatment cages, abnormal behaviors were observed in the 4 ppb treatment cages, including increased locomotor activity, attempts to fly, inability to climb cage walls, and, occasionally, failed attempts to right the body after falling. These observations are similar to a previously described ‘malaise-like’ behavior seen after exposure of honey bees to toxins such as quinine and lithium chloride (Hurst et al., 2014). These observations also match reports from previous studies of caged honey bees chronically ingesting fipronil (Aliouane et al., 2009) and foragers captured at the hive entrance and injected with fipronil (Nahar and Ohtani, 2015). We are intrigued by the data obtained from honey bees sampled from the 4 ppb cages. Although it may be possible that the high dose subjects were less impacted – or able to compensate – in terms of our brain measures than bees exposed to the lower doses (U-shaped relationship or inverted U-shaped relationship, depending on the measure), these results should be regarded as provisional until replicated. We note that a previously published report showed non-linear effects of fipronil exposure on olfactory learning, with performance most impacted by an intermediate dose compared with higher and lower doses (El Hassani et al., 2005). An alternative explanation is the possibility that bees feeding on the highest concentration of fipronil fed less consistently than their lower concentration counterparts.
4.5 Mechanisms of changes in the mushroom body neuropils
Fipronil-induced changes in the mushroom bodies potentially reflect synaptic pruning. As noted previously, however, fipronil has been shown to induce apoptosis in a variety of cell types, including human neuroblastoma SH-SY5Y cells (Vidau et al., 2011). Mitochondrial collapses and increases in cytochrome oxidase activity have also been described in honey bee tissues after fipronil exposure (Roat et al., 2013; Nicodemo et al., 2014). Acute sublethal doses of fipronil increased intensity of anti-synapsin immunofluorescence in the mushroom bodies of leaf cutting ants (Atta sexdens rubropilosa), suggesting a possible increased release of neurotransmitter (Cintra-Socolowski et al., 2015), which may be linked to neurotoxicity. Because hyperexcitability can perturb calcium homeostasis, cell death can be considered as an alternative explanation for the observed decreases in density and number of MCG in our fipronil treatment groups. We did not observe noticeable pyknotic profiles in our samples but acknowledge that our method was not optimized for detection of such profiles. Although we cannot rule out the impact of changes in oxidative phosphorylation and calcium homeostasis, the increases we observed in bouton size suggest that a shift in the balance of synaptogenesis and synaptic pruning is the likelier reason for the observed changes.
4.6 GABA receptors and GluCl channels
Fast, inhibitory neurotransmission is important for neural processing, learning, and memory (Liu et al., 2007; Okada et al., 2007). In insects, inhibitory transmission relies on GABA and GluCl channels (Cole et al., 1993; Barbara et al., 2005; Buckingham et al., 2005). Functional studies have linked inhibitory neurotransmission with olfactory reversal learning in Drosophila melanogaster (Wu et al., 2012) and, in the honey bee mushroom bodies, with odor-evoked oscillation patterns (Stopfer et al., 1997) and tonic inhibition (Palmer and Harvey, 2014).
Fipronil was initially characterized as targeting GABA receptors but has been shown to block GluCl channels under conditions of acute exposure (El Hassani et al., 2009). Both GABA receptors and GluCl channels are present in pupal and adult honey bee Kenyon cells (Grünewald and Wersing, 2008; Démares et al., 2013). Impacts of fipronil in the mushroom bodies can therefore reflect binding to one or both of these neurotransmitter-gated chloride channels. Precise localization with immunolabeling revealed that GluCl channel variants are most abundant in the lip region, present at lower density in the basal ring, and at lower density still in the collar (Démares et al., 2013). In learning assays, acute exposure to sublethal doses of fipronil reduced the proportion of responders but did not block olfactory learning and memory in honey bees (El Hassani et al., 2005). This effect can be rescued with an L-trans-PDC pre-injection, which blocks chloride currents mediated through GluCl channels, demonstrating that GluCl channels are necessary for olfactory learning (El Hassani et al., 2009). Given that we observed more striking effects in the lip than in the collar, it is tempting to speculate that binding of fipronil to GluCl channels may be responsible for the observed changes, but the present study was not designed to distinguish the two receptors.
Our data suggest a pathway by which sublethal exposure to a pesticide may alter foraging behavior. Initially, exposure to fipronil, even at low concentrations, antagonizes GluCl channels and GABA receptors in the mushroom bodies and/or regions afferent to the calyces such as the antennal lobe, which blocks fast, inhibitory current, resulting in enhanced activity in the lip neuropil. This incites synaptic pruning, generating larger boutons, leading to an overall reduction in synaptic density and number of MCG in the mushroom body lip. A temporal shift in the pattern of adult brain development might then be the neural basis by which fipronil subtly degrades performance in olfactory memory tasks and foraging behavior. Given that deviations from the typical age polyethism schedule have been linked with colony failure in stressed honey bee colonies (Perry et al., 2015), it is possible that even a subtle pesticide-induced acceleration of adult brain development might diminish colony fitness.
4.7 Conclusions
Exposure of caged honey bee foragers to sublethal doses of fipronil altered MCG density and number in a dose- and exposure duration-dependent fashion. MCG in the lip and dense collar of adult foragers become larger and less dense with increasing fipronil exposure, and the total number of MCG in the lip decreased. A tentative mechanism is proposed linking fipronil-induced activity increases in mushroom body circuits with changes in MCG like those produced by foraging but unsynchronized with actual foraging experience.
Despite fipronil’s known actions on ion channels in the nervous system, it is exceedingly difficult to assess the risk posed to honey bees and other pollinators by sublethal exposure to fipronil. Studies vary in terms of mode of exposure (topical or ingestion), duration of exposure (acute or chronic), developmental time of exposure, and timing of the assay relative to the time of exposure (Table 1). Fipronil is a popular pesticide but even in areas where field applications are documented, fipronil contamination of pollen, wax, and honey bees is variable and often low, and linkages of pesticides with colony failure are hard to establish (e.g., Chauzat et al., 2009). Consequently, it is difficult to define what levels of contamination should be regarded as field realistic. In the present study, exposure of foragers to fipronil was continuous and cumulative. The extent to which this exposure mimics living in a contaminated hive is unknown. Data are lacking on the central nervous system effects of interactions of exposure to fipronil with exposure to other xenobiotics. The sublethal impacts of fipronil on the nervous system may – or may not – interact with sublethal impacts of fipronil on other aspects of bee physiology including the gut microbiome (Rouzé et al., 2019), drone fertility (Kairo et al., 2017), and immune gene expression (Aufauvre et al., 2012) to yield suboptimal outcomes at the colony level. Developing a rational framework for assessing risks of sublethal exposure to fipronil and other neuroactive pesticides is a major challenge for 21st century insect neurobiology and sustainable agriculture.
Data availability statement
The datasets presented in this study can be found in online repositories. The names of the repository/repositories and accession number(s) can be found below: The dataset for this study can be found in the Figshare Data Repository at: https://doi.org/10.6084/m9.figshare.22709698.v1.
Ethics statement
Ethical review and approval was not required for the study on animals in accordance with the local legislation and institutional requirements.
Author contributions
JP, BVN, and SF contributed to conception and design of the study. JP performed the experiments, analyzed the images, compiled the data, and performed the initial statistical analysis. BVN completed the statistical analysis. JP and SF wrote the first draft of the manuscript. BVN wrote sections of the manuscript. All authors contributed to manuscript revision, read, and approved the submitted version.
Funding
This work was supported by a grant from the Wake Forest University Center for Energy, Environment and Sustainability (CEES) to JP and SF.
Acknowledgments
S. Hathaway, B. Morrow, and E. Schuppe provided technical assistance. The authors thank G. Marrs of the Wake Forest University Microscopic Imaging Core (RRID : SCR_02975) for assistance with microscopy and image analysis.
Conflict of interest
The authors declare that the research was conducted in the absence of any commercial or financial relationships that could be construed as a potential conflict of interest.
The author SF declared that they were an editorial board member of Frontiers, at the time of submission. This had no impact on the peer review process and the final decision.
Publisher’s note
All claims expressed in this article are solely those of the authors and do not necessarily represent those of their affiliated organizations, or those of the publisher, the editors and the reviewers. Any product that may be evaluated in this article, or claim that may be made by its manufacturer, is not guaranteed or endorsed by the publisher.
References
Aliouane Y., El Hassani A. K., Gary V., Armengaud C., Lambin M., Gauthier M. (2009). Subchronic exposure of honeybees to sublethal doses of pesticides: effects on behavior. Environ. Toxicol. Chem. 28, 113–122. doi: 10.1897/08-110.1
Aufauvre J., Biron D. G., Vidau C., Fontbonne R., Roudel M., Diogon M., et al. (2012). Parasite-insecticide interactions: a case study of Nosema ceranae and fipronil synergy on honeybee. Sci. Rep. 2, 326. doi: 10.1038/srep00326
Barbara G. S., Zube C., Rybak J., Gauthier M., Grünewald B. (2005). Acetylcholine, GABA and glutamate induce ionic currents in cultured antennal lobe neurons of the honeybee, Apis mellifera. J. Comp. Physiol. A. 191, 823–836. doi: 10.1007/s00359-005-0007-3
Bernadou A., Démares F., Couret-Fauvel T., Sandoz J. C., Gauthier M. (2009). Effect of fipronil on side-specific antennal tactile learning in the honeybee. J. Insect Physiol. 55, 1099–1106. doi: 10.1016/j.jinsphys.2009.08.019
Boitard C., Devaud J. M., Isabel G., Giurfa M. (2015). GABAergic feedback signaling into the calyces of the mushroom bodies enables olfactory reversal learning in honey bees. Front. Behav. Neurosci. 9. doi: 10.3389/fnbeh.2015.00198
Bonmatin J. M., Giorio C., Girolami V., Goulson D., Kreutzweiser D. P., Krupke C., et al. (2015). Environmental fate and exposure; neonicotinoids and fipronil. Environ. Sci. Pollut. Res. 22, 35–67. doi: 10.1007/s11356-014-3332-7
Bovi T. S., Zaluski R., Orsi R. O. (2018). Toxicity and motor changes in Africanized honey bees (Apis mellifera L.) exposed to fipronil and imidacloprid. An. Acad. Bras. Cienc. 90, 239–245. doi: 10.1590/0001-3765201820150191
Buckingham S. D., Biggin P. C., Sattelle B. M., Brown L. A., Sattelle D. B. (2005). Insect GABA receptors: splicing, editing, and targeting by antiparasitics and insecticides. Mol. Pharmacol. 68, 942–951. doi: 10.1124/mol.105.015313
Cabirol A., Brooks R., Groh C., Barron A. B., Devaud J. M. (2017). Experience during early adulthood shapes the learning capacities and the number of synaptic boutons in the mushroom bodies of honey bees (Apis mellifera). Learn. Mem. 24, 557–562. doi: 10.1101/lm.045492.117
Casida J. E., Durkin K. A. (2013). Neuroactive insecticides: targets, selectivity, resistance, and secondary effects. Annu. Rev. Entomol. 58, 99–117. doi: 10.1146/annurev-ento-120811-153645
Chauzat M. P., Carpentier P., Martel A. C., Bougeard S., Cougoule N., Porta P., et al. (2009). Influence of pesticide residues on honey bee (Hymenoptera: Apidae) colony health in France. Environ. Entomol. 38, 514–523. doi: 10.1603/022.038.0302
Chauzat M. P., Faucon J. P., Martel A. C., Lachaize J., Cougoule N., Aubert M. (2006). A survey of pesticide residues in pollen loads collected by honey bees in France. J. Econ. Entomol. 99, 253–262. doi: 10.1603/0022-0493-99.2.253
Cintra-Socolowski P., Roat T. C., Nocelli R. C., Nunes P. H., Ferreira R. A., Malaspina O., et al. (2015). Sublethal doses of fipronil intensify synapsin immunostaining in Atta sexdens rubropilosa (Hymenoptera: Formicidae) brains. Pest. Manage. Sci. 72, 907–912. doi: 10.1002/ps.4065
Cole L. M., Nicholson R. A., Casida J. E. (1993). Action of phenylpyrazole insecticides at the GABA-gated chloride channel. Pestic. Biochem. Physiol. 46, 47–54. doi: 10.1006/pest.1993.1035
Decourtye A., Devillers J., Aupinel P., Brun F., Bagnis C., Fourrier J., et al. (2011). Honeybee tracking with microchips: a new methodology to measure the effects of pesticides. Ecotoxicol. 20, 429–437. doi: 10.1007/s10646-011-0594-4
Decourtye A., Devillers J., Genecque E., Le Menach K., Budzinski H., Cluzeau S., et al. (2005). Comparative sublethal toxicity of nine pesticides on olfactory learning performances of the honeybee Apis mellifera. Arch. Environ. Contam. Toxicol. 48, 242–250. doi: 10.1007/s00244-003-0262-7
Démares F., Raymond V., Armengaud C. (2013). Expression and localization of glutamate-gated chloride channel variants in honeybee brain (Apis mellifera). Insect Biochem. Mol. Biol. 43, 115–124. doi: 10.1016/j.ibmb.2012.10.003
Desneux N., Decourtye A., Delpuech J. M. (2007). The sublethal effects of pesticides on beneficial arthropods. Annu. Rev. Entomol. 52, 81–106. doi: 10.1146/annurev.ento.52.110405.091440
Devaud J. M., Papouin T., Carcaud J., Sandoz J. C., Grünewald B., Giurfa M. (2015). Neural substrate for higher-order learning in an insect: Mushroom bodies are necessary for configural discriminations. Proc. Natl. Acad. Sci. U.SA. 112 (43), E5854–E5862. doi: 10.1073/pnas.1508422112
Dobrin S. E., Herlihy J. D., Robinson G. E., Fahrbach S. E. (2011). Muscarinic regulation of Kenyon cell dendritic arborizations in adult worker honey bees. Arthropod Struct. Dev. 40, 409–419. doi: 10.1016/j.asd.2011.01.003
Durst C., Eichmüller S., Menzel R. (1994). Development and experience lead to increased volume of subcompartments of the honeybee mushroom body. Behav. Neural Biol. 62, 259–263. doi: 10.1016/s0163-1047(05)80025-1
El Hassani A. K., Dacher M., Gauthier M., Armengaud C. (2005). Effects of sublethal doses of fipronil on the behavior of the honeybee (Apis mellifera). Pharmacol. Biochem. Behav. 82, 30–39. doi: 10.1016/j.pbb.2005.07.008
El Hassani A. K., Dupuis J. P., Gauthier M., Armengaud C. (2009). Glutamatergic and GABAergic effects of fipronil on olfactory learning and memory in the honeybee. Invert. Neurosci. 9, 91–100. doi: 10.1007/s10158-009-0092-z
Fahrbach S. E. (2006). Structure of the mushroom bodies of the insect brain. Annu. Rev. Entomol. 51, 209–232. doi: 10.1146/annurev.ento.51.110104.150954
Fahrbach S. E., Van Nest B. N. (2016). Synapsin-based approaches to brain plasticity in adult social insects. Curr. Opin. Insect Sci. 18, 27–34. doi: 10.1016/j.cois.2016.08.009
Farris S. M., Robinson G. E., Fahrbach S. E. (2001). Experience- and age-related outgrowth of intrinsic neurons in the mushroom bodies of the adult worker honeybee. J. Neurosci. 21, 6395–6404. doi: 10.1523/JNEUROSCI.21-16-06395.2001
Groh C., Lu Z., Meinertzhagen I. A., Rössler W. (2012). Age-related plasticity in the synaptic ultrastructure of neurons in the mushroom body calyx of the adult honeybee Apis mellifera. J. Comp. Neurol. 520, 3509–3527. doi: 10.1002/cne.23102
Groh C., Tautz J., Rössler W. (2004). Synaptic organization in the adult honey bee brain is influenced by brood-temperature control during pupal development. Proc. Natl. Acad. Sci. U.S.A. 101, 4268–4273. doi: 10.1073/pnas.0400773101
Gronenberg W. (2001). Subdivisions of hymenopteran mushroom body calyces by their afferent supply. J. Comp. Neurol. 435, 474–489. doi: 10.1002/cne.1045
Grünewald B., Wersing A. (2008). An ionotropic GABA receptor in cultured mushroom body Kenyon cells of the honeybee and its modulation by intracellular calcium. J. Comp. Physiol. A. 194, 329–340. doi: 10.1007/s00359-007-0308-9
Hofbauer A., Ebel T., Waltenspiel B., Oswald P., Chen Y. C., Halder P., et al. (2009). The Wuerzburg hybridoma library against Drosophila brain. J. Neurogenet. 23, 78–91. doi: 10.1080/01677060802471627
Hourcade B., Muenz T. S., Sandoz J. C., Rössler W., Devaud J. M. (2010). Long-term memory leads to synaptic reorganization in the mushroom bodies: a memory trace in the insect brain? J. Neurosci. 30, 6461–64655. doi: 10.1523/JNEUROSCI.0841-10.2010
Hurst V., Stevenson P. C., Wright G. A. (2014). Toxins induce ‘malaise’ behaviour in the honeybee (Apis mellifera). J. Comp. Physiol. A. 200, 881–890. doi: 10.1007/s00359-014-0932-0
Ismail N., Robinson G. E., Fahrbach S. E. (2006). Stimulation of muscarinic receptors mimics experience-dependent plasticity in the honey bee brain. Proc. Natl. Acad. Sci. U.S.A. 103, 207–211. doi: 10.1073/pnas.0508318102
Jacob C. R., Soares H. M., Nocelli R. C., Malaspina O. (2014). Impact of fipronil on the mushroom bodies of the stingless bee Scaptotrigona postica. Pest. Manage. Sci. 71, 114–122. doi: 10.1002/ps.3776
Jones T. A., Donlan N. A., O’Donnell S. (2009). Growth and pruning of mushroom body Kenyon cell dendrites during worker behavioral development in the paper wasp, Polybia aequatorialis (Hymenoptera: Vespidae). Neurobiol. Learn. Mem. 92, 485–495. doi: 10.1016/j.nlm.2009.06.007
Kairo G., Poquet Y., Haji H., Tchamitchian S., Cousin M., Bonnet M., et al. (2017). Assessment of the toxic effect of pesticides on honey bee drone fertility using laboratory and semifield approaches: A case study of fipronil. Environ. Toxicol. Chem. 36, 2345–2351. doi: 10.1002/etc.3773
Klagges B. R., Heimbeck G., Godenschwege T. A., Hofbauer A., Pflugfelder G. O., Reifegerste R., et al. (1996). Invertebrate synapsins: a single gene codes for several isoforms in Drosophila. J. Neurosci. 16, 3154–3165. doi: 10.1523/JNEUROSCI.16-10-03154.1996
Krofczik S., Khojasteh U., de Ibarra N. H., Menzel R. (2008). Adaptation of microglomerular complexes in the honeybee mushroom body lip to manipulations of behavioral maturation and sensory experience. Dev. Neurobiol. 68, 1007–1017. doi: 10.1002/dneu.20640
Kühn-Bühlmann S., Wehner R. (2006). Age-dependent and task-related volume changes in the mushroom bodies of visually guided desert ants, Cataglyphis bicolor. J. Neurobiol. 66, 511–521. doi: 10.1002/neu.20235
Liu X. B., Coble J., van Luijtelaar G., Jones E. G. (2007). Reticular nucleus-specific changes in alpha3 subunit protein at GABA synapses in genetically epilepsy-prone rats. Proc. Natl. Acad. Sci. U.S.A. 104, 12512–12517. doi: 10.1073/pnas.0705320104
Lorenz M. W., Kellner R., Völkl W., Hoffmann K. H., Woodring J. (2001). A comparative study on hypertrehalosaemic hormones in the Hymenoptera: sequence determination, physiological actions and biological significance. J. Insect Physiol. 47, 563–571. doi: 10.1016/s0022-1910(00)00133-5
Mahler B. J., Van Metre P. C., Wilson J. T., Musgrove M., Zaugg S. D., Burkhardt M. R. (2009). Fipronil and its degradates in indoor and outdoor dust. Environ. Sci. Technol. 43, 5665–5670. doi: 10.1021/es901292a
Menzel R. (2014). The insect mushroom body, an experience-dependent recoding device. J. Physiol. Paris. 108, 84–95. doi: 10.1016/j.jphysparis.2014.07.004
Muenz T. S., Groh C., Maisonnasse A., Le Conte Y., Plettner E., Rössler W. (2015). Neuronal plasticity in the mushroom body calyx during adult maturation in the honeybee and possible pheromonal influences. Dev. Neurobiol. 75, 1368–1384. doi: 10.1002/dneu.22290
Mullin C. A., Frazier M., Frazier J. L., Ashcraft S., Simonds R., Vanengelsdorp D., et al. (2010). High levels of miticides and agrochemicals in North American apiaries: implications for honey bee health. PloS One 5, e9754. doi: 10.1371/journal.pone.0009754
Nahar N., Ohtani T. (2015). Imidacloprid and fipronil induced abnormal behavior and disturbed homing of forager honey bees Apis mellifera. J. Entomol. Zool. Stud. 3, 65–72.
Nicodemo D., Maioli M. A., Medeiros H. C., Guelfi M., Balieira K. V., De Jong D., et al. (2014). Fipronil and imidacloprid reduce honeybee mitochondrial activity. Environ. Toxicol. Chem. 33, 2070–2075. doi: 10.1002/etc.2655
Okada R., Rybak J., Manz G., Menzel R. (2007). Learning-related plasticity in PE1 and other mushroom body-extrinsic neurons in the honeybee brain. J. Neurosci. 27, 11736–11747. doi: 10.1523/JNEUROSCI.2216-07.2007
Palmer M. J., Harvey J. (2014). Honeybee Kenyon cells are regulated by a tonic GABA receptor conductance. J. Neurophysiol. 112, 2026–2035. doi: 10.1152/jn.00180
Peitsch D., Fietz A., Hertel H., de Souza J., Ventura D. F., Menzel R. (1992). The spectral input systems of hymenopteran insects and their receptor-based colour vision. J. Comp. Physiol. A. 170, 23–40. doi: 10.1007/BF00190398
Perry C. J., Søvik E., Myerscough M. R., Barron A. B. (2015). Rapid behavioral maturation accelerates failure of stressed honey bee colonies. Proc. Natl. Acad. Sci. U.S.A. 112, 3427–3432. doi: 10.1073/pnas.1422089112
Pisa L. W., Amaral-Rogers V., Belzunces L. P., Bonmatin J. M., Downs C. A., Goulson D., et al. (2015). Effects of neonicotinoids and fipronil on non-target invertebrates. Environ. Sci. Pollut. Res. 22 (1), 68–102. doi: 10.1007/s11356-014-3471-x
Raccuglia D., Mueller U. (2013). Focal uncaging of GABA reveals a temporally defined role for GABAergic inhibition during appetitive associative olfactory conditioning in honeybees. Learn. Mem. 20, 410–416. doi: 10.1101/lm.030205.112
Rigosi E., Tison L., Haase A. (2022). Editorial: Effects of pesticides on the brain of pollinating insects. Front. Insect Sci. 2. doi: 10.3389/finsc.2022.1113610
Roat T. C., Carvalho S. M., Nocelli R. C., Silva-Zacarin E. C., Palma M. S., Malaspina O. (2013). Effects of sublethal dose of fipronil on neuron metabolic activity of Africanized honeybees. Arch. Environ. Contam. Toxicol. 64, 456–466. doi: 10.1007/s00244-012-9849-1
Rouzé R., Moné A., Delbac F., Belzunces L., Blot N. (2019). The honeybee gut microbiota is altered after chronic exposure to different families of insecticides and infection by Nosema ceranae. Microbes Environ. 34, 226–233. doi: 10.1264/jsme2.ME18169
Seeley T. (1982). The adaptive significance of age polyethism schedule in honeybee colonies. Behav. Ecol. Sociobiol. 11, 287–293. doi: 10.1007/BF00299306
Simon-Delso N., Amaral-Rogers V., Belzunces L. P., Bonmatin J. M., Chagnon M., Downs C., et al. (2015). Systemic insecticides (neonicotinoids and fipronil): trends, uses, mode of action and metabolites. Environ. Sci. Pollut. Res. 22, 5–34. doi: 10.1007/s11356-014-3470-y
Sommerlandt F. M., Spaethe J., Rössler W., Dyer A. G. (2016). Does fine color discrimination learning in free-flying honeybees change mushroom-body calyx neuroarchitecture? PloS One 11 (10), e0164386. doi: 10.1371/journal.pone.0164386
Stieb S. M., Hellwig A., Wehner R., Rössler W. (2012). Visual experience affects both behavioral and neuronal aspects in the individual life history of the desert ant Cataglyphis fortis. Dev. Neurobiol. 72, 729–742. doi: 10.1002/dneu.20982
Stieb S. M., Muenz T. S., Wehner R., Rössler W. (2010). Visual experience and age affect synaptic organization in the mushroom bodies of the desert ant Cataglyphis fortis. Dev. Neurobiol. 70 (6), 408–423. doi: 10.1002/dneu.20785
Stoner K. A., Eitzer B. D. (2013). Using a hazard quotient to evaluate pesticide residues detected in pollen trapped from honey bees (Apis mellifera) in Connecticut. PloS One 8, e77550. doi: 10.1371/journal.pone.0077550
Stopfer M., Bhagavan S., Smith B. H., Laurent G. (1997). Impaired odour discrimination on desynchronization of odour-encoding neural assemblies. Nature 390, 70–74. doi: 10.1038/36335
Tingle C. C., Rother J. A., Dewhurst C. F., Lauer S., King W. J. (2003). Fipronil: environmental fate, ecotoxicology, and human health concerns. Rev. Environ. Contam. Toxicol. 176, 1–66. doi: 10.1007/978-1-4899-7283-5_1
Vidau C., González-Polo R. A., Niso-Santano M., Gómez-Sánchez R., Bravo-San Pedro J. M., Pizarro-Estrella E., et al. (2011). Fipronil is a powerful uncoupler of oxidative phosphorylation that triggers apoptosis in human neuronal cell line SHSY5Y. Neurotoxicol. 32, 935–943. doi: 10.1016/j.neuro.2011.04.006
Wilmart O., Legrève A., Scippo M. L., Reybroeck W., Urbain B., de Graaf D. C., et al. (2021). Honey bee exposure scenarios to selected residues through contaminated beeswax. Sci. Total Environ. 772, 145533. doi: 10.1016/j.scitotenv.2021.145533
Withers G. S., Fahrbach S. E., Robinson G. E. (1993). Selective neuroanatomical plasticity and division of labour in the honeybee. Nature 364, 238–240. doi: 10.1038/364238a0
Wu Y., Ren Q., Li H., Guo A. (2012). The GABAergic anterior paired lateral neurons facilitate olfactory reversal learning in Drosophila. Learn. Mem. 19, 478–486. doi: 10.1101/lm.025726.112
Zaluski R., Justulin L. A. Jr., Orsi R. O. (2017). Field-relevant doses of the systemic insecticide fipronil and fungicide pyraclostrobin impair mandibular and hypopharyngeal glands in nurse honeybees (Apis mellifera). Sci. Rep. 7, 15217. doi: 10.1038/s41598-017-15581-5
Zhang E., Nieh J. C. (2015). The neonicotinoid imidacloprid impairs honey bee aversive learning of simulated predation. J. Exp. Biol. 218, 3199–3205. doi: 10.1242/jeb.127472
Zhao X., Yeh J. Z., Salgado V. L., Narahashi T. (2004). Fipronil is a potent open channel blocker of glutamate-activated chloride channels in cockroach neurons. J. Pharmacol. Exp. Ther. 310, 192–201. doi: 10.1124/jpet.104.065516
Keywords: Apis mellifera, fipronil, GABA, GluCl receptors, microglomeruli, mushroom bodies, synapsin
Citation: Privitt JJ, Van Nest BN and Fahrbach SE (2023) Altered synaptic organization in the mushroom bodies of honey bees exposed as foragers to the pesticide fipronil. Front. Bee Sci. 1:1219991. doi: 10.3389/frbee.2023.1219991
Received: 09 May 2023; Accepted: 27 July 2023;
Published: 11 August 2023.
Edited by:
Xesús Feás, Academy of Veterinary Sciences of Galicia, SpainReviewed by:
Randolf Menzel, Freie Universität Berlin, GermanyBrian H. Smith, Arizona State University, United States
Copyright © 2023 Privitt, Van Nest and Fahrbach. This is an open-access article distributed under the terms of the Creative Commons Attribution License (CC BY). The use, distribution or reproduction in other forums is permitted, provided the original author(s) and the copyright owner(s) are credited and that the original publication in this journal is cited, in accordance with accepted academic practice. No use, distribution or reproduction is permitted which does not comply with these terms.
*Correspondence: Susan E. Fahrbach, fahrbach@wfu.edu