- Department of Chemistry, University of California, Riverside, Riverside, CA, United States
3-Dimensional (3D) printing technology has greatly facilitated the recent advancements in science and engineering that benefit many aspects of scientific investigation, with examples including disease diagnostics, dentistry, aerospace, and fundamental research. For analytical chemistry, many advancements can be directly linked to achievements associated with 3D printing of optics, flow systems, mechanical/structural components, and parts related to detection/measurement, which before the advent of 3D printing were limited by complicated, cumbersome, expensive, and material-limited production. More importantly, the totality of these advances has made the possibility of 3D printing the majority of an analytical system an achievable reality. In this review, we highlight the recent achievements and advancements reported in literature that will facilitate the development of the next-generation analytical instrumentation through the use of 3D printing technology. A great deal of attention is given to those in the context of bioanalytical platforms and novel biosensing strategies. Limited by space, we will explicitly focus the discussion on the following areas: improvement/utilization of new printing materials, methods towards higher resolution, fabrication and production of optical components, novel microfluidic flow systems, and printed structural components for instrumentation.
1 Introduction
3D printing, also known as additive manufacturing, is a rapidly evolving field that has revolutionized the workflow of a number of diverse disciplines (Shahrubudin et al., 2019; Su X. et al., 2021; Dawood et al., 2015; Lambert et al., 2018; Cardoso et al., 2020; Su, 2021; Grajewski et al., 2021). The widespread adoption of 3D printing can be attributed to three key factors. First, the ease of generation and modification of 3D printed objects, which relies on user-friendly computer aided design (CAD) software (Junk and Kuen, 2016). Second, 3D printing is relatively inexpensive and allows access to a large range of printable materials (Shahrubudin et al., 2019), including polymers, organics, glass, ceramics, and metals, the vast majority of which are commercially available (Lee et al., 2017). Third, rapid improvements in print resolution, have facilitated the creation of defined features both on and within the printed object that were previously impossible, enabling new applications (Shahrubudin et al., 2019; Lee et al., 2017). These features have made the process of going from concept to prototype more efficient and reliable. While many 3D printing techniques exist and offer different degrees of power and capability, the most widely used ones, include fused deposition modeling (FDM) (Mohamed et al., 2015), VAT polymerization stereolithography (SLA) (Ng et al., 2020), digital light processing (DLP) (Amini et al., 2023), polyjet printing (PJT) (Layani et al., 2018), selective laser sintering (SLS) (Kruth et al., 2003), and two photon printing (T-PP) (Xing et al., 2015). A summary of the general advantages and disadvantages of each technique is shown in Table 1.
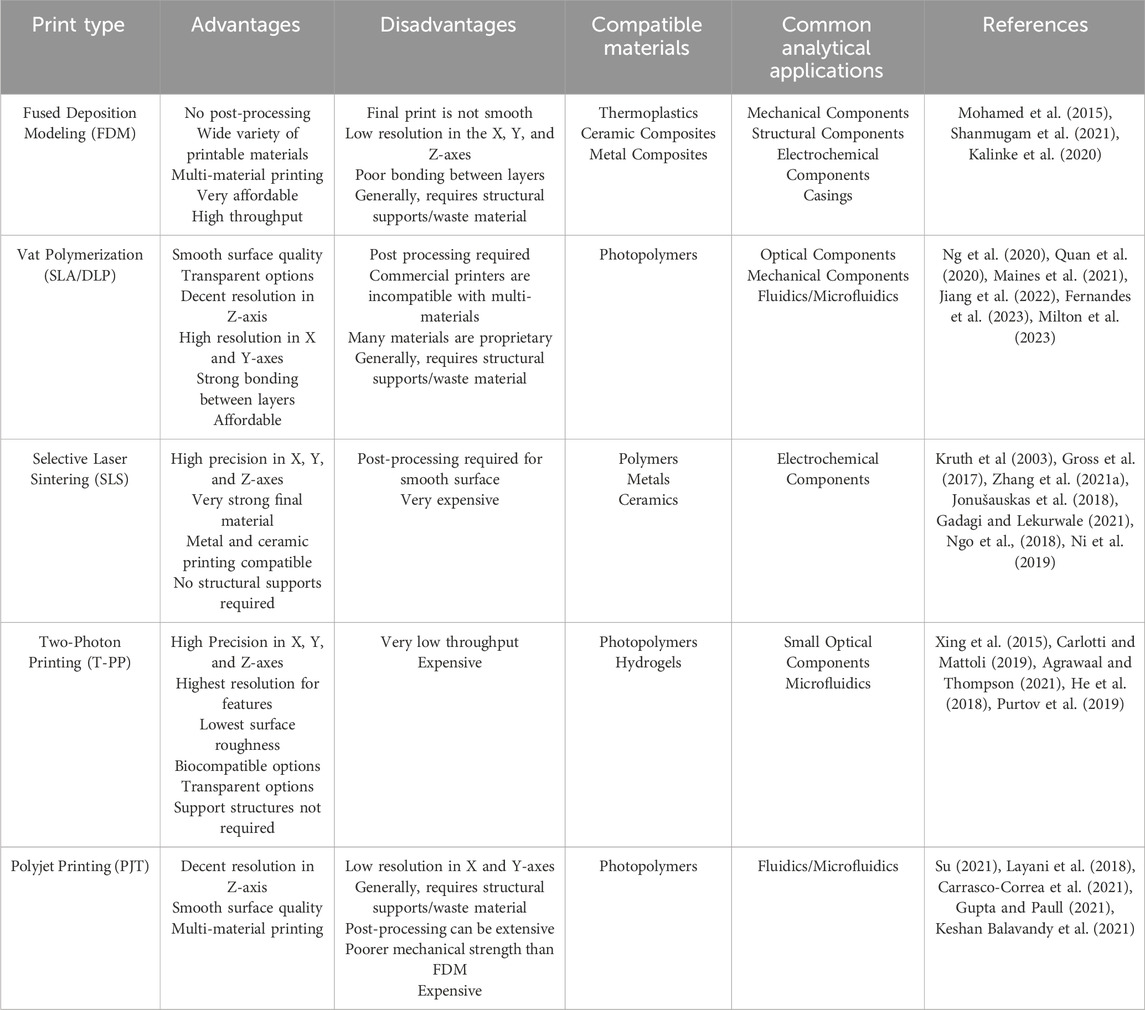
Table 1. Overview of advantages and disadvantages of some of the major 3D printing techniques for development of bioanalytical instrumentation.
FDM involves the layer-by-layer extrusion of semi-liquid material through a heated nozzle (Mohamed et al., 2015). The materials come in the form of long thermoplastic filaments, such as polylactic acid (PLA) and acrylonitrile butadiene styrene (ABS), or biocompatible and highly chemical resistant chemicals such as polyetheretherketone (PEEK), fluoropolymers, polyetherimide (PEI), as well as various composite materials (Shanmugam et al., 2021). FDM based 3D printers tend to be quite affordable and can quickly produce macrostructures, making them an attractive option for applications in which high print precision is not necessary. Additionally, many of these devices are compatible with multi-material printing by exchanging the material being extruded, thereby expanding the applicability of FDM-based 3D printers by allowing for configurations of unique physio-chemical properties (Mohamed et al., 2015). Another key advantage associated with FDM-based 3D printing is that it requires little to no post-processing after deposition, thereby further reducing both expense and production time. However, FDM has some major limitations including low resolution, high surface roughness, and poor mechanical robustness due to lack of strong bonding between layers.
While both SLA and DLP are vat polymerization 3D printing methods (Quan et al., 2020), they have slightly different operating workflows. DLP relies on a conventional stationary light source, such as an arc lamp that triggers the polymerization process of the liquid monomer, usually an epoxy or acrylic-based resin (Quan et al., 2020). The 3D object is built layer-by-layer by hardening the photopolymer on a movable solid platform. SLA uses a UV laser to harden the polymer in both the X and Y coordinates tracing a cross section of the mold spot-to-spot until the layer is completed (Quan et al., 2020). In DLP the operator can control the intensity of the light source thus changing the consistency of the 3D print, which contrasts with the laser used in SLA that cannot be adjusted (Maines et al., 2021). SLA offers better curing (hardening) of the 3D print through the layer-by-layer process, thus potentially improving the surface quality of the print when compared to DLP (Jiang et al., 2022). In terms of printing speed, DLP is generally faster than SLA, as it polymerizes the entire layer at once (Maines et al., 2021). When compared to FDM, both SLA and DLP show significantly reduced surface roughness, lower porosity, and higher resolution at the expense of a higher cost (Quan et al., 2020). While commercial vat polymerization printers currently do not support multi material printing recently Fernandes et al. (2023) have developed a DLP printer that replaced the resin within the vat enabling multi material printing to be achieved.
PJT printers use photocurable liquid resins similar to those employed in VAT polymerization strategies (SLA/DLP), while carrying the added benefit of multi-material printing (Su, 2021). While SLA/DLP rely on the photopolymer as the support for the print, PJT uses a wax filling material as the support during the print (Su, 2021). PJT systems achieve this by employing inkjet heads in conjunction with photopolymers polymerized by a UV light to produce 3D printed structures where different materials can be introduced through a set of inkjet heads. Generally, PJT is used for the production of multi-material parts with complex structures that require smooth surface quality/low porosity such as microfluidics and membranes, though PJT is limited in its ability to produce small, closed channels due to the need to remove support material. The substantially higher expense when compared to vat-polymerization and FDM is also a potential major drawback (Carrasco-Correa et al., 2021). In addition to high cost, another drawback of PJT is the long post-processing of the print, particularly with regards to highly complex designs, which makes this method less attractive for some scientific applications (Carrasco-Correa et al., 2021).
SLS operates by fusing cross sections of a powdered material deposited on a solid support using a high-powered laser (Gross et al., 2017). The solid support is moved after each cross section is completed, more powder is applied, and the process is repeated until the 3D printed structure is finished (Zhang D. et al., 2021). Because the unfused powder remains to support the structure as it is constructed, unique geometries can be facilitated that are not possible with some of the previously discussed methods (Jonušauskas et al., 2018). Using SLS additionally allows for the use of a wider range of materials such as ceramics, metals, organic polymers, and glass. While this process allows for high precision, the complex cleaning process and the use of high-powered laser make SLS expensive and largely inaccessible for hobbyists and researchers alike (Shahrubudin et al., 2019; Vanaei et al., 2021).
The final major 3D printing modality that will be discussed in this review is T-PP (Carlotti and Mattoli, 2019). T-PP operates through a pulsed laser that polymerizes photoactive material at single points within the bulk polymer with high precision (Xing et al., 2015). Due to the polymerization process of T-PP, exceptionally small feature structures can be generated that are well outside the capabilities of other 3D printing techniques (Agrawaal and Thompson, 2021). This quality has made T-PP an extremely valuable tool for applications that require high precision, such as tissue engineering, drug delivery, and optical component development (Carlotti and Mattoli, 2019). However, T-PP comes with a major limitation in print speed due to the point-by-point nature of the printing method. Hence, its usage is generally limited to very small objects.
While many areas of study are using 3D printing in a plethora of unique ways, the presented review will focus on the advancements and utilization of the aforementioned 3D printing methods in the context of bioanalytical sciences, particularly regarding the instrumentation used in those studies. To date, researchers in the field of bioanalytical sciences have used 3D printing for the fabrication of various components for analytical instruments, including but not limited to moving components, casings, optics, flow systems and sensing platforms (Carrasco-Correa et al., 2021; Ambrosi and Bonanni, 2021; Bishop et al., 2016). This has dramatically expanded the capabilities and accessibility of various analytical instruments, as well as reducing the long-term operation cost of the instruments (Carrasco-Correa et al., 2021). With the ever-increasing reliability and technical capabilities of 3D printing, the question of whether a complete analytical system/instrument can be 3D printed and mass produced has arisen (Bishop et al., 2016). From the recent advancements in areas such as new materials, higher resolution methods, and increased print speed, it has become increasingly clear that the answer to this question is a resounding yes. This review will cover some of the recent developments and achievements that have a strong linkage to creating analytical platforms with printing technology and the works that can serve as a resource for implementing 3D printing strategies into the construction of analytical instruments.
While several reviews in the past have covered 3D printing topics in great detail ranging from materials, optical components, microfluidic systems, and more. The presented review attempts to give a new perspective on recent advances in the field of 3D printing related to analytical instrumentation, and to then present an outline on how one could effectively 3D print the bulk of an instrument. For the purposes of this work, the designation of analytical instrument will apply to an instrument that quantitatively measures the presence, properties, interactions, and/or composition of and analyte or solution of interest. While a great deal of publications are widely available related to the 3D printing of various analytical instrumentation components we attempt to outline the process by which one using various materials and methods could generate a 3D printed instrument composed of structural components, moving parts (gears and pumps), optical pieces (prisms and lenses), flow/separation systems (microfluidics and chromatography columns), and/or sensors.
2 3D printing materials
While the 3D printing strategies mentioned above have provided avenues to construct increasingly detailed and functional structures, many of their current capabilities are due to advances in the materials available. These materials are fundamental to the functioning of 3D printing techniques and therefore can enable improvements in a variety of areas, such as print speed (Khosravani and Reinicke, 2020), cost (Carrasco-Correa et al., 2021), resolution (Mao et al., 2017; Wen et al., 2021), surface roughness (Hartcher-O’Brien et al., 2019; Vaidya and Solgaard, 2018a; Al-Dulaijan et al., 2022), biocompatibility (Sta. Agueda et al., 2021; Guttridge et al., 2022), resistance to degradation (Chen et al., 2018; Upadhyay et al., 2020; Khalfa et al., 2021), solvent resistance (Heikkinen et al., 2018; Erokhin et al., 2019), transparency (Nguyen et al., 2017; Odent et al., 2017; Cecil et al., 2020), conductivity (Cardoso et al., 2020; Su, 2021), as well as other diverse properties, such as flexibility (Balakrishnan et al., 2021; Lin et al., 2022). Due to the importance of the material used in additive manufacturing, understanding which materials are available and their applicability is key to the utilization of 3D printing to its fullest potential. For information about the specifics of 3D printing materials, Ranjan et al. (2022) recently provided an in-depth review. As such we will touch only on currently available and recently demonstrated materials that fall under four key categories: polymers, ceramics, metals/conductive materials, and additives to 3D printing materials. Each material possesses its own unique applications to sensor and instrument development, which will be discussed in later sections.
2.1 Polymers
Polymers constitute the most commonly utilized 3D printing materials due to the ability to rapidly and precisely initiate the liquid to solid phase transition. For a more in-depth discussion of the subject Ligon et al. (2017) provide a relatively recent review. Because many polymeric compounds have relatively low melting points and can be heated to extrude small quantities on demand, their use is vital to FDM printing. While pre-polymerized material is used in FDM, monomers can be precisely crosslinked under a UV light source as is done in SLA, DLP, PJT, and T-PP 3D printing. There are many commercially available 3D printing polymers for use in each of these techniques, with PLA, acrylonitrile styrene acrylate, polyethylene terephthalate, polyethylene terephthalate glycol (PETG), polycarbonate (PC), polypropylene, PEEK, polyetherketoneketone, and PEI making up the vast major of commercially available materials with slight variations due to additives (Tümer and Erbil, 2021; Pakkanen et al., 2017; Rett et al., 2021; Dizon et al., 2021; Spoerk et al., 2020; Oladapo et al., 2021; Norani et al., 2021). However, many of the commercial materials often lack integral chemical information due to their proprietary nature (Grajewski et al., 2021). As such, it is required to identify the ideal material for the application before a particular commercial material is chosen. For example, Musgrove et al. (2022) compared three clear polymer resins in the properties of viscosity, heat stability, biocompatibility, and optical clarity. A guide was created on how to systematically test, select, and improve polymer resin printing for optimal integration into a select case. With the extensive list of available polymers for 3D printing it has become a major undertaking to identify the ideal material for each print.
2.2 Ceramic materials
Ceramic materials have become widely used 3D printable materials due to a number of highly desirable properties. Notable features of ceramics include hardness, chemical inertness, resistance to wear/corrosion, and mechanical properties significantly different from other 3D printable materials. Ceramics have found use in sensing applications predominantly through the 3D printing of glass or similar materials for optical components, which will be discussed in more detail later in this review. Notably, analytical use of 3D printed ceramics also includes the fabrication of piezoelectric substrates as recently demonstrated by Liu et al. (2022) The authors investigated three ceramic resins: barium titanate, lead zirconate titanate, and aluminum nitrate, for the fabrication of piezoelectric structures that were then monitored for their applicability to wearable motion sensors. A review by Romanczuk-Ruszuk et al. on 3D printable ceramics Romanczuk-Ruszuk et al. (2023) provides a more complete overview of the field for those interested, but outside of optical components, they see scarce application to analytical instrumentation.
2.3 Metals and conductive materials
3D printing of metals is a niche area due to the added difficulty of controlled melting while maintaining both structural integrity and chemical stability for the print. It relies on either SLS or similar processes known as selective laser melting (SLM) (Gadagi and Lekurwale, 2021). Each of these techniques comes with specific materials they are capable of printing, as well as differing material characteristics. Notably, SLS metal prints are highly porous and brittle (Ngo et al., 2018). SLM has therefore been the popular technique for recent work due to higher fidelity and ability to print metal combinations (Ni et al., 2019). A wide variety of metals are available to be used in these processes, including aluminum, copper, nickel, steel, and titanium, as well as a number of metal alloys (Ni et al., 2019). Alternative methods to deposit metals have also been developed to allow for a wider adoption of metals in additive manufacturing (Ni et al., 2019). For example, 3D printing of copper has been demonstrated with nanometer scale resolution through precise electrochemical reduction of a copper solution onto the substrate. However, this requires a conductive surface be present and the presence of a working electrode (Hengsteler et al., 2021). In addition, several other 3D printing methods can be used for the incorporation of metals into additive manufacturing, such as those demonstrated by Wei et al. (2015) with a graphene electrode and a custom-made resin on FDM.
Conductive 3D printing materials are often produced through addition of inexpensive conductive materials such as carbon black (Stefano et al., 2022) and graphene (Guo et al., 2019). Recently Kalinke et al. (2020) compared the two most popular FDM-PLA conductive 3D printing materials: graphene-PLA by Black Magic and PLA-carbon black by Proto Pasta. They showed that the electrodes made from graphene had superior electrochemical and structural performance relative to those made from carbon black. In contrast, a custom electrode resin developed by Stefano et al. (2022), produced by refluxing PLA and graphite, showed better electrochemical response than commercial graphene or carbon black. Intrinsically conductive polymer mixtures like PEDOT:PSS (Yuk et al., 2020) have also found use in 3D printing. These discoveries lead to the question of what other properties can be achieved via additives and what effects these additives have on printability.
2.4 Material additives
Each of the aforementioned materials is effective alone, but through the combination of the printing materials or addition of other materials, new properties can be achieved. For example, the formation of polymer composites has been a major area of 3D printing material additive research and has been reviewed by Singh et al. (2020) For sensing applications, integration of nanoparticles into 3D printing resins has been demonstrated, and the approach is attractive to many due to the presence of novel functionality. Specifically, conductivity, magnetism, and piezoelectricity can be tuned through the incorporation of nanoparticles (Shafranek et al., 2019). However, these addition can cause major complications, such as loss in structural integrity and/or resolution of the final product (Cardoso et al., 2020). This has been shown to be caused by variable mechanisms dependent on the nanoparticle themselves (Muñoz and Pumera, 2020). For example, plasmonic nanoparticles affect the structure through thermal heating in the process of stereolithography, leading to thermal degradation of the organic polymer. Semiconducting and plasmonic polymers, on the other hand, act as radical quenchers and reduce the amount of radical activated polymerization (Su, 2021).
In a similar fashion to plasmonically active nanoparticles, dyes can be used as additives to facilitate visible light as a cure in SLA resins. Exact control of the optical absorption of the resin is required in all directions during the curing phase to ensure fine resolution is maintained (Quan et al., 2020). As the absorption of the resin increases, the resolution improves as there is less dispersion of the light through the uncured medium (Ahn et al., 2020). In addition to their role as curing catalysts, dyes are also used to introduce functionality to the polymers, such as indicators to changes of pH, physical pressure, light and temperature. The advantages of these additives allow for fine tuning of the polymer to the specific purpose necessary in the application (Gastaldi et al., 2020).
3 Mechanical and structural components
The ability of 3D printers to quickly generate various geometrical designs with sufficient structural stability has made this technology attractive for production of components that enable the sensing apparatus through component alignment, movement, and protection. A great deal of work has been conducted towards 3D printed structural and mechanical parts that are essential to the development and assembly of an analytical instrument. 3D printing of these components has allowed researchers to quickly design and produce unique setups with greatly reduced difficulty. Publications related to formation of gears, cell scaffolds, and outer casings, as well as those demonstrate their application to sensing platforms will be discussed and reviewed in the following section. While not particularly complicated and not directly involved in the sensing, these are components crucial to making 3D printed instruments a reality.
3.1 Moving parts
The use of 3D printed gears and pumps has been reported in various studies including uses for robotics, domestic applications, and in analytical instrumentation (Zhang et al., 2020a; Arena et al., 2023; Vasilescu, 2019; Zatopa et al., 2018; Zolfagharian et al., 2016; Naz et al., 2023). The main benefits of using the parts generated from additive manufacturing include sufficent surface quality, broad availability from both metallic and non-metallic materials, high flexibility in design modifications, and less waste as compared to other strategies (Del Rosario et al., 2022). These features are associated with the material used when printing gears, the most common of which include nylon, ABS, PLA, and PETG (Zolfagharian et al., 2016; Del Rosario et al., 2022; Subramaniyan et al., 2022). One of the major concerns for the printed gears and pumps is limited operational life expectancy, specifically in terms of wear and thermal behavior over prolonged uses. Recent studies have focused on understanding how misalignments, temperature, and heavy use can affect 3D moving parts, and approaches to optimize the system to improve longevity (Zhang et al., 2020a; Subramaniyan et al., 2022; Zhang et al., 2020b; Kotkar et al., 2018; Buj-Corral and Zayas-Figueras, 2023).
The most common types of gears for scientific investigations include spur gears, helical gears, bevel gears, worm gears, and planetary gears (Subramaniyan et al., 2022). Spur gears are defined by having straight protrusions that are parallel to the axis of rotation. Due to their simple design they are very easy to print but are known to be inefficient for work at high speeds (Apparao and Raju, 2021). This weakness has made the selection of material to print a key factor. Helical gears are similar to spur gears, but their edges are angled compared to the axis of rotation (Berger, 2015). Helical gears are ideal for transfer motion in a typical instruments setup, such as moving a stage horizontally or vertically in a microscope. Bevel gears have edges on the conical surface of the gear, which allows for transmission of motion between intersecting or non intersecting shafts for changing the direction or speed of rotation (García-García and González-Palacios, 2018). Worm gears are known for their screw-like shape and transmit motion between non-intersecting shafts that are perpendicular to each other (Berger, 2015). All of these gears can be 3D printed with high quality.
Recently Sharkey et al. (2016) used 3D printing to generate components of an open source microscope including hinges, gears, and the stage. The 3D printed microscope was found to have sub-micron scale motion over a range of 8 × 8 × 4 mm, and a drift of only 20 μm over the course of a week was observed without temperature stabilisation. Sule et al. (2019) created a hand operated, 3D printed centrufuge with two spur gears, two bevel gears, a gear crank and a test tube holder, as shown in Figure 1. Along with a 3D printed centrifuge and microfluidic system to concetrate, separate, and extrude a mixture in sample preperation, the 3D printed microscope forms a realistic analytical paltform that can address some of the point-of-care detection needs in real-world sample analysis. With the diverse types of gears and materials available for their printing, as well as the ability to quickly and inexpensive implement customizable movement, printed gears are a valuable but often overlooked use of 3D printing technology in sensing applications.
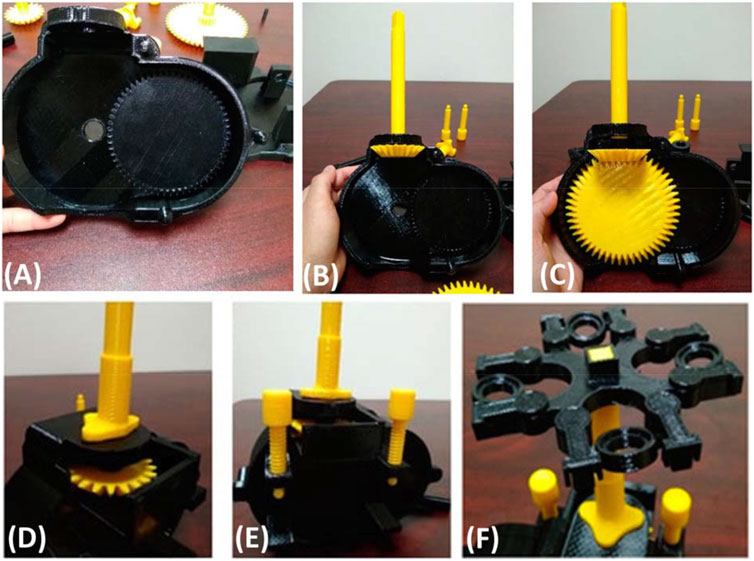
Figure 1. (A) Picture of 3D printed plate and printed spur gear locked in. (B) 3D printed bevel gear inserted into what is shown in (A). (C) Another 3D printed spur gear inserted into the 3D printed apparatus. (D) Aparatus closed with all internal and external 3D printed components. (E) Use of 3D printed bolts to hold the two 3D printed casings covering the 3D printed gears in place. (F) 3D printed testube holders designed to move to allow for centrufigation through a 3D printed hand cranked system not shown. All images are adapted from Sule et al. (2019).
3.2 Structural components for sensing platforms
Perhaps the most popular use of 3D printing in analytical platform construction comes in the making of structural and supporting components of the instrument. These large structures form the basis of sensing platforms when integrated with purchased or fabricated optical and/or electrical components. The required print quality in these applications is generally low, meaning they are easily accessible and rapidly producible. These features leave them particularly amenable to analytical platforms that benefit from low-cost and portability. To further reduce costs, these devices are often designed to accommodate smartphones as the light source and/or detector (Quesada-Gonzalez and Merkoci, 2017). 3D printed spectrophotometers for use with smartphones have been reported for some time, but more recent work has been focused on designing platforms that are easily fabricated and operated, while also being compatible with a range of different smartphones (Bogucki et al., 2019). Works such as these are often aimed at chemical education purposes such as making analytical instrumentation accessible to a broader number of communities, including those with limited resources. Another use of 3D printed smartphone-compatible instruments involves point-of-care medical monitoring (Chan et al., 2017). Some recent examples of this implementation include colorimetric (Zhang et al., 2021b) and electrochemiluminescent (Bhaiyya et al., 2022) monitoring of blood glucose, and nanoprobe-based fluorescent detection of glutathione (Chu et al., 2020). Platforms such as these offer simple, low cost, and portable methods for individuals and healthcare providers to monitor crucial health biomarkers. Another application for this platform is rapid on-site environmental monitoring for regulators and researchers. These sensors are generally colorimetric and have been used to detect environmental contaminants such as heavy metals (Lai et al., 2022), pesticides (Su D. et al., 2021), and halocarbons (Gul et al., 2021).
In addition, a valuable but less elegant usage of 3D printing is for quickly producing housings for home-built analytical tools. Often times these housings amount to simple boxes with cut-outs to align and insert functional components. These housings are valuable components because they can be customized to the envisioned design, improve portability, and limit outside interferences such as light and dust. One example comes from the previously mentioned work by Xiao et al. (2022) which demonstrated the use of 3D printing for the housing of instrumental components for their phone based imaging SPR device (Figure 2). da Silva et al. (2020) used a 3D printed UV chamber with a smartphone detector to achieve high throughput fluorescent analysis of quinine concentration in beverages. Using a biodegradable polymer, this method represents an economically and environmentally friendly example of applications for 3D printed housings. A more complex use for 3D printed housings was demonstrated by Casto et al. (2019) who designed a dual-detector, Taylor dispersion analysis module that could be integrated with a capillary electrophoresis (CE) instrument. In a future publication by the same group, they removed the need for the CE instrument and produced a 3D printed instrument featuring a fully 3D printed eductor that allowed for highly stable pressure through the capillary (Moser et al., 2022). Even though range of potential applications for 3D printed structural components in analytical instrumentation are extremely broad, the examples presented in this section are representative of the bulk of the uses and should provide an introduction to some of their demonstrated uses.
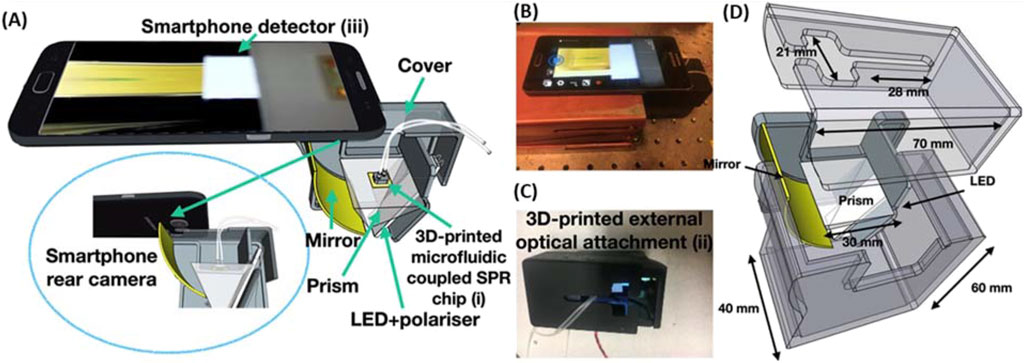
Figure 2. (A) Schematic of the imaging SPR smartphone system integrated with the 3D printed microfluidic system on top of an SPR chip in the Kretschmann configuration. (B) Image of the entire device assembled. (C) Image of the 3D printed structural component to house the optical attachment. (D) The CAD image with appropriate dimensions for the SPR-based smartphone structural attachment. Adapted from Xiao et al. (2022).
4 3D printed optics
There are a number of in-depth reviews that focus on the additive manufacture of optical components. Berglund et al. (2022) provide an excellent overview of recent developments and future outlooks in 3D printed optics as well as practical considerations for selecting proper 3D printing method for a particular component. For information regarding specific materials, Zhang D. et al. (2021) and Blachowicz et al. (2021) have published reviews on 3D printed glass and polymer optical components respectively. To set this section apart from these reviews and a host of other reviews on the subject, we will instead focus on presenting recent examples that are closely related to the integration of 3D printed optical components in analytical applications, as well as recent developments in the materials and methods aimed at platform development. Additionally, in this section we aim to provide a full picture on the field of available 3D printed optical components and some of the key applications to which they can be applied.
Optical components comprise some of the most important and difficult to produce components in analytical instrumentation. As a result, the additive manufacture of optical components for sensing has seen rapid development over the past decade, aided by the burgeoning advancement of 3D printing over the same period (Jiang et al., 2022; Bhuvanesh Kumar and Sathiya, 2021). 3D printing of optical components shares many of the general advantages conferred by additive manufacture such as heightened accessibility, reduced waste, and rapid prototyping (Lambert et al., 2018). Compared to traditional manufacture of glass optics, 3D printing allows for fine control over internal and external structures (Shu et al., 2022; Vogt and Leonhardt, 2016), as well as optical properties through the use of additives inside the deposition material (Destino et al., 2018; Aslani et al., 2023; Ali et al., 2021). In addition, the process can be completed on site in labs with limited resources. However, optical components have specific requirements and considerations when compared to other additively manufactured components. More specifically, they generally require high homogeneity, optical transparency, and refractive indices with an accompanying low surface roughness to reduce losses due to scattering. Achieving satisfactory results over the range of sensing applications to which these optics are applicable has necessitated significant research on both materials and methods.
The materials used in 3D printed optics can be broken down into two major sub-classes: glass and transparent resins/polymers. Glass is an ideal material for optics owing to its high optical transparency and refractive index, as well as its thermal and chemical stability. However, the high melting point of glass has resulted in significant difficulties in producing 3D printed glass with appropriate size, shape, porosity, structural integrity, surface roughness, and/or transparency while maintaining low cost and ease of production. As a result, 3D printing of effective glass optics has largely been restricted to micro-optical components that can reasonably be printed with high resolution methods (Nguyen et al., 2017; Luo et al., 2014; Moore et al., 2020). However, there have been some advances, particularly in regards to glass micro-optics, in the past few years that will be discussed further into this section. Transparent resin and photopolymer-based 3D printing materials, while exhibiting fewer ideal properties for optical applications, are far more compatible with available 3D printing methodologies. Examples of the effective use of these materials in optical components include waveguides (Dingeldein et al., 2013), lenses (He et al., 2018), gratings (Purtov et al., 2019), collimators (Thiele et al., 2016), and refractive elements (Xiao et al., 2016). With the rapid increase in the library of available 3D printing materials and methods, the range and quality of optical components produced may result in printed optics eventually supplanting traditional glass optics as the preferred components for analytical instrumentation. In the proceeding section we will review recent advances in this field that may contribute to this prediction becoming a reality.
4.1 Optical component developments
Due to inherent difficulties associated with 3D printing of glass optical components, the bulk of recent publications in the field remain focused on polymer-based optics. However, there are some notable recent developments to speak of. Toombs et al. (2022) recently demonstrated the high resolution 3D printing of silica glass microfluidic and micro-optical components using a photopolymer-silica nanocomposite material. The use of multi-component materials with direct laser writing (DLW) photopolymerization followed by sintering to remove the photopolymer, leaving behind nonporous glass with high optical transparency, has been in use for some time (Kotz et al., 2017; Gonzalez-Hernandez et al., 2021). However, the unique development presented in Toombs’ work is the integration of a tomographic photopolymerization method, dubbed microscale computed axial lithography (micro-CAL). Instead of photopolymerizing the structure layer-by-layer as with typical SLA/DLP methods, the structures are formed within the bulk solution by projecting 2D images into the resin. Some benefits include smoother surfaces, increased mechanical strength, and more complex geometries due to the lack of need for solid supporting structures. Additionally, compared to T-PP methods, micro-CAL is capable of producing the desired component more quickly. All of these advantages are highly desirable in the design of optical components, and it can be expected that micro-CAL will see future use as a lithographic method not only for glass, but other 3D printing materials as well.
The production of high-quality 3D printed glass optics with polymerization-sintering methods has long been plagued by the issue of shrinkage with accompanying defects (bubbles, warping, etc.) that occur in the process of burning off organic material. In the aim of minimizing this issue, Hong et al. demonstrated the 3D printing single and multi-component glass micro-optics using pre-condensed liquid silica resins (LSRs) (Hong et al., 2021; Hong et al., 2022). These LSRs are synthesized by polymerization of tetramethoxysilane (TMOS) with methacryloxymethyltrimethoxysilane (MMTS), causing a high degree of crosslinking. (Figure 3A). In their first work, the authors were able to produce micro-optics and gratings with surface roughness lower than 6 nm and shrinkage of only 17%. However, it was found that the LSR used in this work containing about 6.5 mol% MMTS had insufficient mechanical stability to support large aspect ratios and more complex multi-component optical components. Increasing MMTS mol% led to a higher degree of cross-linkage resulting in improved mechanical strength, but this was accompanied by increased shrinkage due to the higher organic fraction. Selecting 15% MMTS as their most ideal material composition, Hong and colleagues were able to produce a range of complex micro-optical components boasting surface roughness’s less than 6 nm (Figures 3B–E). To demonstrate a use case for this optimized strategy for glass micro-optic 3D printing, it was used to produce a high-resolution snapshot hyperspectral imaging (HSHI) system (Hong et al., 2023a). While the produced light guide arrays for the HSHI system are too limited in size and structural stability for many applications, with a printer boasting a high range-of-movement stage with appropriate precision, along with material improvements, they could see utility in a range of analytical systems.
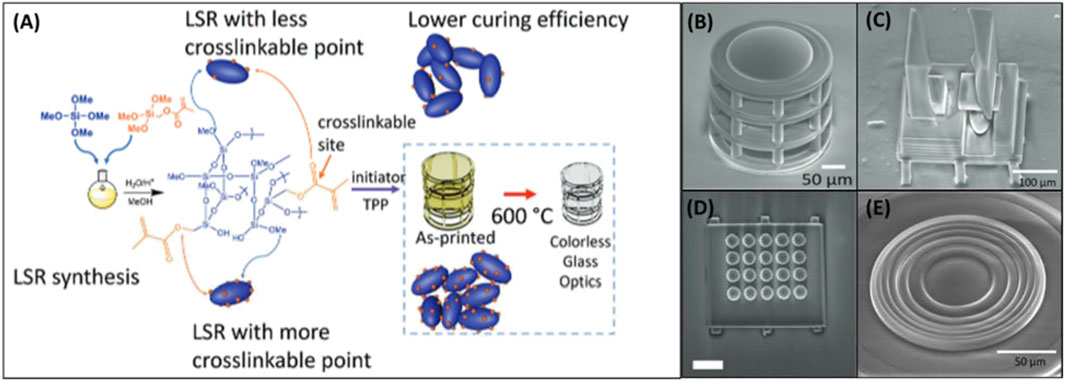
Figure 3. (A) Scheme for LSR synthesis and glass micro-optic fabrication. (B) SEM of printed micro-objective. (C) SEM of 3D printed Alvarez lens. (D) SEM of 3D printed lenslet array. (E) SEM of 3D printed Fresnel surface. Adapted from (Hong et al., 2022).
Recent advances in the materials for 3D printed glass optics are not limited to silica glass. Hong et al. recently reported the production of large (mm-to-cm scale) 3D printed germanate glass optics using a fiber-fed printing method (Hong et al., 2023b). The production of optics-quality glass by 3D printing has primarily been limited to the micrometer regime due to the slow print speeds of high resolution methods. Germanate glass has many positive properties including high mid-IR transparency, refractive indices, chemical stability, and thermal stability. Due to these factors, it has tremendous potential for application to mid-IR sensing applications, but compared to other glass materials, it is comparatively difficult to shape, polish, and grind to appropriate quality for these tasks, thereby limiting its usage (Li et al., 2009). With their optimized printing system, Hong and colleagues produced simple lenses and a multi-lens microscopy system exhibiting surface roughnesses less than 1 nm. This work represents a significant first step in not only the practicality of 3D printing optics-quality germanate glass, but glass optics with other materials as well through their optimized heating and deposition process.
As polymer-based 3D printed optics are the most practical and widely adopted in the field of additive manufacture, the number of publications reporting material and methodological developments extend beyond what can be reasonably encompassed here. Instead, we will present recent examples that are particularly notable and/or impactful and could prove valuable in the development of analytical instrumentation. Publications in this area can be divided into methodological and material developments, or both categories if the new material enables a new printing method or the production of a unique optical component. Additionally, methodological advancements include novel applications of existing 3D printing methods.
The purpose of developing new materials for 3D printing is often to improve the quality of the printed optics. However, an alternative avenue is to expand the range of possible applications through cost, accessibility, or new material properties. One of these properties that is often overlooked is material flexibility, which has particular utility for analytical instrumentation utilizing waveguides. Flexible waveguides have unique potential in analytical platforms by allowing simple and inexpensive routing of light in the system. Nseowo Udofia and Zhou (2020) recently reported the use of ballistic gel materials with a custom micro-extrusion 3D printer to produce highly soft and elastic optical components. The material was used to generate caustic patterns, beam splitters, optical encoders, and decorative waveguides with low cost, low transmission loss, and high optical transparency. Another material development that allows for unique optical application for 3D printing is the addition of fluorescent particles into the printing resin. Tai et al. (2019) integrated perovskite nanocrystals, which have broad applications in optoelectronics (Zhan et al., 2022), into polycaprolactone (PCL) as a protective and workable carrier resin. Using this material, they were able to generate low micron-sized fluorescent fibers and demonstrate tunable down-conversion of light from a blue LED to produce white light, a process that has been the subject of significant research for decades.
As with glass optics, the time and cost efficient 3D printing of high quality polymer-based optical components in the mesoscale without additional (and possibly inconsistent) polishing steps has been a difficult challenge to overcome (Bhaduri et al., 2017; Vaidya and Solgaard, 2018b). One recent attempt to address this comes from Nair et al. (2022) who modified a low-cost and commercially available LCD 3D printer with a fiber-optic taper to improve the resolution of printed components at the cost of reduced print speed (Figure 4A). The fiber-optic taper reduced print speed from 30 mm3 s-1 to 0.25 mm3 s-1, but significantly reduced surface roughness from ∼2 μm to tens of nanometers. It should be noted that while the print speed is significantly reduced, this method is still orders of magnitude faster than most T-PP methods. However, the acquired surface roughness remains significantly higher than what can be acquired with polished glass optics (Chada et al., 2015; Lu et al., 2019). Considering the cost, print speed, and lack of need for additional processing steps, this method may prove attractive for applications where optics of low or medium quality are acceptable. Notably, this work does not represent the technical peak of rapid 3D printing of optics, as another publication reporting a higher printing speed and lower surface roughness of ∼1.35 mm3 s-1 and 13.7 nm respectively (Shao et al., 2019). This was achieved with a custom projection microstereolithography (PμSL) system (Figure 4B) that was able to produce a 3 mm high lens in only 2 min. However, these printing quality improvements do come at the cost of decreased accessibility and significantly increased price of the system. However, once the printing system is in place, the bulk of components for a microscope can be produced in 50 min at a cost of only $4 as was demonstrated in a follow up study (Figure 4A). (Hai et al., 2023) When focusing on the lens specifically (Figure 4B), it was produced in only 3 min at a unit cost of ∼7 cents while boasting improved surface roughness (∼7.63 nm), structural integrity, and imaging contrast when compared to commercially available lenses (Figures 4C, D). Another recent example of mm-scale 3D printed optics comes from Ristok et al. (2020) who produced lenses up to 2 mm across with optical performance and surface roughnesses (∼2.9 nm) comparable to commercial glass lenses. These lenses were produced by a dip-in laser lithography (DILL) T-PP method with an objective that allows for a larger than normal writing field. With a print time of 5 h for the 1 mm lens and 23 h for the 2 mm lens, this method is much slower when compared to the previously presented examples. Nevertheless, these examples clearly demonstrate that there is no ideal method for producing optical components on the mesoscale, and it requires the user to balance quality against the competing factors of cost and print time.
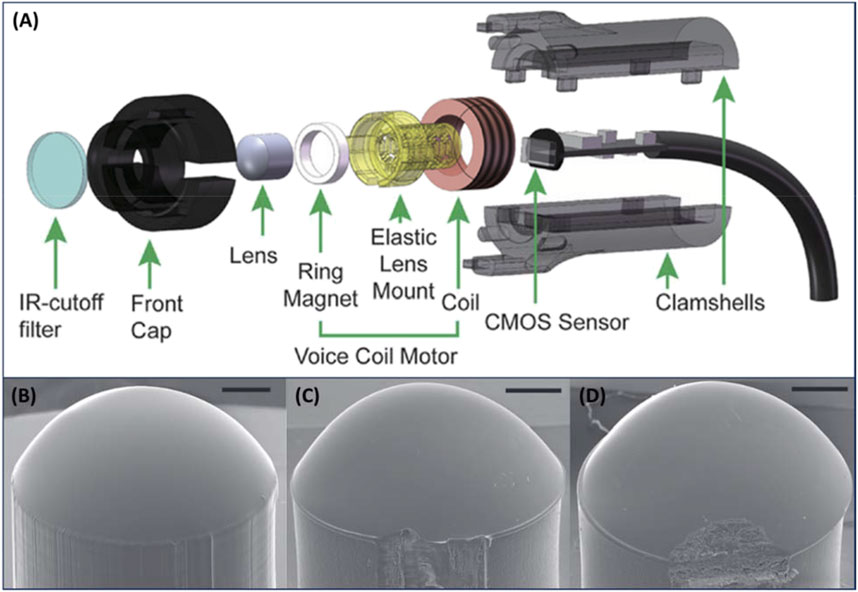
Figure 4. (A) Illustration of design and components used in 3D printed optical microscope. (B) Image of 3D printed lens aspherical lens. (C) Image of lens purchased from Edmund Optics (15-271). (D) Image of lens purchased from Thorlabs, Inc. (APL0303). Scale bars: 500 μm. Adapted from (Vogt and Leonhardt, 2016).
Aside from waveguides and simple UV-Vis or IR lenses, 3D printing has been used for manufacturing other optical components. Sanli et al. (2022) report a custom 3D laser lithography system that allows for the production of high resolution kinoforms. A kinoform is a type of lens that allows for highly efficient focusing of x-rays. These lenses often require complex nanofabrication procedures and are dependent on small feature sizes to perform efficiently. This work demonstrates a relatively low-cost method to produce high quality optics that could be implemented in analytical systems that utilize X-rays. DLW-printed multicomponent achromat and apochromat lenses have been demonstrated that greatly reduce chromatic aberration (Schmid et al., 2021). Optical instruments using multiple wavelength sources can benefit from these lenses that ensure all wavelengths of light are efficiently focused on the same position. One particularly unique implementation of 3D printed lenses comes in the form of customizable optical tweezer arrays with dynamic control of each tweezer using a digital micromirror device (Schaffner et al., 2020). They allow for highly precise trapping and control of individual particles for a wide range of investigations relating to subjects such as biological systems, atmospheric chemistry, and dielectric particles (Gorkowski et al., 2020; Quang et al., 2018; Catala-Castro et al., 2022). The rapid design of 3D-printed optical tweezer arrays that can be customized for individual studies may prove to be an extremely valuable application for additive manufacturing moving forward.
Another advantage to the high level of control inherent to additive manufacture is the ability to produce diffractive optical elements (DOEs) that generate precise optical patterns with utility in a broad range of applications (O'Shea et al., 2003; Tang et al., 2019; Kunwar et al., 2020). Producing these components relies not only on the optimization of printing methodologies, but also the design of the DOEs through modeling. Despite the rapid prototyping ability implicit to additive manufacture, modeling an optimal design prior to production greatly minimizes tedious trial and error. Wang et al. (2020) recently developed a modeling system for the design of “near-perfect” diffractive optical elements, which utilized four key parameters of 3D printing systems–laser power, beam scan speed, hatching distance, and slicing distance–to predict print quality and correct for subwavelength morphological variations before fabrication. Their model, dubbed the lumped 3D printing TPL parametric model, was employed to produce Dammann gratings that generate arrays of light with highly uniform intensity and spacing (Figure 5A). Taking modeling a step further, 3D printed diffractive element networks designed by deep learning (Figure 5B) have recently been demonstrated to control the amplitude and phase of incoming light (Veli et al., 2021). This work proves to be highly impactful to many applications, including spectroscopy, as it lays the groundwork for the rapid design and production of elements for precise control of light. Although the networks demonstrated low power efficiency and the output pulse did not precisely match the computed models (Figure 5C), these issues could be largely remedied in the future by implementation of lower-absorption materials and improved modeling strategies. The combination of high-resolution 3D printing with advanced modelling for prototyping is a new and potentially highly impactful development for optical analytical instruments.
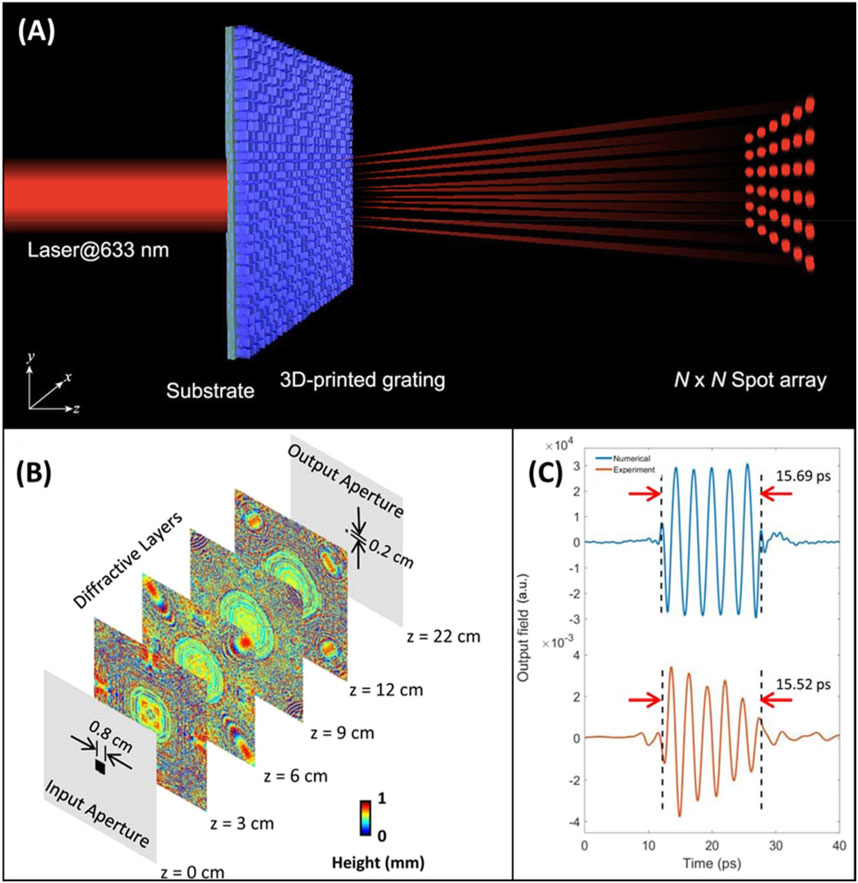
Figure 5. (A) Illustration of 3D printed Dammann grating generating a precise optical array pattern. Adapted from (Wang et al., 2020). (B) Multi-layer pulse shaping network with deep learning designed diffractive layers. (C) Numerical and experimental shaped output pulses from the diffractive network. Adapted from (Veli et al., 2021).
4.2 3D printed optic integration in sensing
3D printed materials have a history of implementation in optical sensing with some examples including Kretschmann configuration surface plasmon resonance (SPR) sensing of cholera toxin (Hinman et al., 2017), surface-enhanced infrared spectroscopy detection of PMMA (Braun and Maier, 2016), and surface layer thickness terahertz sensing (Li et al., 2017). However, as the additive manufacture of optical components is still very much in its infancy, traditionally manufactured components remain largely preferred for actual sensing applications. At this time, the startup cost of obtaining a 3D printer of appropriate quality as well as the materials and training required makes this approach less sensible for researchers who do not have particular interest in making developments in 3D printing itself. In this section, recent examples that demonstrate potential advantages to the use of 3D printed components beyond simple per-unit cost and prototyping considerations will be presented. A focus will be placed on examples where the use of additive manufacture allows for new sensing configurations and improvements instead of simply being used as replacement for traditionally manufactured components.
One of the major practical benefits of the low per-unit price of 3D printed optics is that they can be implemented into sensing units that are disposable. This is particularly beneficial in applications where the optical component is the sensing surface itself and its timely replacement improves performance as the surface becomes fouled or degraded over time. One relevant example is SPR sensing, in which the sensing surface (generally ∼50 nm film of gold) can be directly deposited on the optical coupling element (prism) that can be 3D printed. Hinman et al. (2017) established a method for 3D printing and polishing of transparent prisms for SPR sensing. Lertvachirapaiboon et al. (2021) recently expanded this design by using soft lithography to imprint a grating onto one side of the prism. After depositing gold over the grating, they demonstrated the generation of two different modes of SPR coupling, occurring at visible and NIR wavelengths. The sensitivity of the instrument was also found to correspond to the absorption profile of materials deposited on the surface. In addition, it allowed rapid tuning of SPR wavelength for specific analytes. Disposable 3D printed prisms have also been integrated into single-use cultivation vessels for determining the optical density of microbial culture (Kuhnke et al., 2022). By integrating the prisms into the vessels, light is deflected 90o from the source located under the vessel and detected in the horizontal plane, thereby avoiding perturbations occurring at the liquid-air interface. With this adjustment they were able to achieve roughly 3-times the sensitivity than traditional vessels.
Waveguide based sensors are another emerging use for 3D printing technologies due to the broad range of configurations and materials that are readily accessible. Valued for their simple design and low cost, these sensors typically utilize light transmission change through the waveguide for quantification as the presence of an analyte causes alterations to the resonant modes. Analyte concentration can be determined by measuring the power/intensity change at a set wavelength or the change in the resonant wavelength. While many sensors carry this common motif, the analyte responsive materials and their implementation vary greatly. The waveguide itself can be used to sense the analyte, as reported by Swargiary et al. (2020) The “tower” waveguide was utilized as an isopropanol vapor sensor by measuring output changes as the isopropanol vapor caused swelling. Detection can also be accomplished based on interactions with the cladding material. Darder et al. (2022) reported a 3D printed fiber optic waveguide coated with a cladding composed of Nafion containing Leuco Fuchsin dyes. Formaldehyde vapor was detected based on the intensity change occurring as a result of contact with formaldehyde. The use of a 3D printed optic fiber was necessary to bring the limit of detection within the ranges as removing existing cladding from commercial fiber optics results in high brittleness and chemical instability that negatively affects sensing performance. The use of long-period fiber gratings (LFGs) by 3D printing for various sensing applications has been demonstrated. With LFGs, spaced strips of material that swell in response to an analyte are placed over a fiber optic cable, where they cause a wavelength shift due to changes in refractive index and accompanying resonance changes. This method has been employed for the detection of biologically relevant levels of glucose by DLP-based patterning of ConA and dextran containing hydrogel over a fiber optic cable (Wei et al., 2023). Glucose competes with dextran for binding to ConA, leading to swelling of the polymer, thereby causing the refractive index and resulting wavelength change that enables sensing.
5 3D printed fluidic systems
One major 3D printing application has been the development of flow systems due to the ability to rapidly prototype and adapt new flow geometries at minimal cost. As a result, 3D printed fluidic devices have been extensively reviewed in recent years (Musgrove et al., 2022; Nielsen et al., 2020). 3D printing of microfluidic systems for applications aimed at instrument design and improvement, however, is less explored. In this Review, we will report advancements that expand the capabilities of 3D printed fluidics and recent work that demonstrates the printing of fluidic systems for implementation into analytical instrumentation.
Flow systems are an integral part of many analytical instrumentations by enabling samples to be split, mixed, and separated in various ways to facilitate the sample treatment and processing necessary for detection. While 3D printing has not yet fundamentally changed the capabilities of fluidics, it has drastically improved their accessibility. This has particularly empowered instrumentation development that was previously prohibited by the cost of precise machining. Recent advancements in printing materials and methods with improved resolution, as discussed in previous sections, have permitted 3D printers to achieve smaller channel sizes and more complex geometries that may prove to revolutionize the field of fluidics going forward.
The benefits of increased accessibility are clearly evident in the broad applications of recent 3D printed fluidic systems such as extraction, separation, sensing, and cell growth. Each of these applications relies on advancements in 3D printing technology and materials over the past few decades. These applications will be reviewed in the following sections that focus on extraction and separation techniques, microfluidic sensing applications, cell growth and sorting, as well as reaction vessels and other unique applications. Additionally, recent work with 3D printed fluidics will be discussed, along with their interfacing with 3D printed parts and applications in both benchtop and point-of-care instrumentations.
5.1 Extraction and separation
One major use for 3D printing in the field of microfluidics is to enable rapid extraction and separation of small volumes of analyte solutions. To achieve this, a small 3D printed module is implemented within the analytical platform to automate the commonly laborious steps of extraction/separation. These modules can take many different forms based on their particular use case. A vast range of designs have been reported to achieve maximal capabilities and compatibility with point-of-care sensing and instrumentation.
One area where 3D printed microfluidics have been extensively utilized is solid phase extraction (SPE) through polymerization/packing of monoliths in 3D printed fluidic channels. These SPE materials have been applied in various ways. Gupta and Paull (2021) demonstrated a microfluidic chip capable of splitting flow across 64 channels with highly uniform flow velocities that could be useful for SPE applications (Figure 6). A detachable piston shaped roof was attached within the print that allowed sorbent to be placed in the reservoir. Extraction efficency was greatly improved with the three dimensional bifurcating flow distribution, compared to a single channel or 2D flow distributor due to the substantially higher number of channels. Furthermore, the 3D distributor did not show signs of sorbent saturation as opposed to the 2D distributor, which the authors attirbuted to more uniform distribution of flow across the whole particle bed. Alternatively, Irlam et al. (2020) tested various printing materials and commercial sorbents to develop a 3D printed housing that connects with syringes, allowing detection of various trace explosives through SPE when used in combination with liquid chromatography. 3D printed microfluidics have also been combined with antibody labeled SPE monoliths to enable immunoaffinity specific extraction (Almughamsi et al., 2022). Three antibodies for pre-term birth biomarkers were immobilized to the monolith to enable the extraction and analysis of these markers in serum. It should be noted that the formulation of these monoliths within a microfluidic device can be a difficult task. In an attempt to address this problem, Ren et al. (Belkilani et al., 2022) developed miniature 3D printed solid phase extraction cartridges that simplify the assimilation of SPE materials into the device. The use of integrated frits with porous microstructures between the support and the build materials for polyjet printing (Keshan Balavandy et al., 2021) created a channel that captures SPE materials while maintaining flow. The small tunable SPE cartridges were 3D printed with precise dimensions and connections needed for a select application. SPE materials have also been used in 3D printed centrifugation based separation devices (Zhang et al., 2019; Zhang et al., 2020c) showing excellent promise for all-in-one sample treatment, which could be interfaced with instrumentation for rapid analysis. To this end, 3D printed SPE applications have been interfaced with HPLC (Carrasco-Correa et al., 2020) and ICP-MS (Su and Lin, 2020) to enable direct analysis of extracted components.
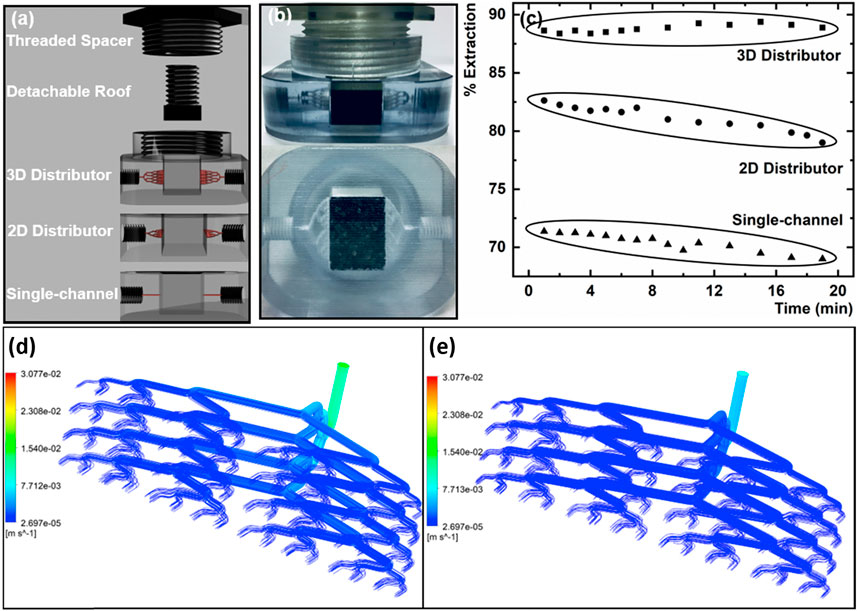
Figure 6. (A) Representation of the multi channeled 3D printed fluidics utilized for solid phase extraction. (B) Image of the 3D bifurcating distributor with activated charcoal packed in the solid phase extraction reservoir. (C) Resulting extraction percent based on different fluidic systems. With fluid dynamic simulations of fluid flow through the 3D flow distributor with flow rates of (D) 0.2 mL min−1 and (E) 0.1 mL min−1. Adapted from (Gupta and Paull, 2021).
Various non-SPE microfluidic devices have been developed for fluid mixing (Duarte et al., 2022), concentration gradient generation (Heuer et al., 2022; Zhang et al., 2021c), cellular lysis (Yang Z. et al., 2022; Nittala et al., 2023), and many other applications (Ogishi et al., 2022; Shrimal et al., 2022; Li et al., 2021; Kulkarni et al., 2023). There are several examples of the integration of these systems with analytical techniques, demonstrating their promise for use in wholly 3D printed system. Habib et al. (2022) demonstrated the combination of microfluidics techniques to enable protein purification. Yang et al. developed a 3D printed microfluidic device to lyse, mix, and separate algal cells for MALDI-MS based lipid profiling, as shown in Figure 7 (Yang Z. et al., 2022). Algal cells were introduced into an array of micropillars that caused droplet breakup and significant mass transfer between the two phases. The lipid rich organic phase was collected from an outlet in the reservoir and spotted on a MALDI-MS chip for lipidomics study. While others have reported 3D printed serpentine microfluidic channels to enable single cell separation for a subsequent analysis with pulsed electric field-induced electrospray ionization MS (Feng et al., 2022). Where cell to cell heterogeneity was analyzed by rapid separation via 3D printed microfluidics. Concentration gradients have also been produced using 3D printed microfluidic devices enabling antibiotic susceptibility testing (Heuer et al., 2022; Zhang et al., 2021c; Winkler et al., 2022), where gradient generating microfluidics formed multiple wells or growth chambers with varying concentrations allowing measurement of bacterial susceptibility to antibiotics.
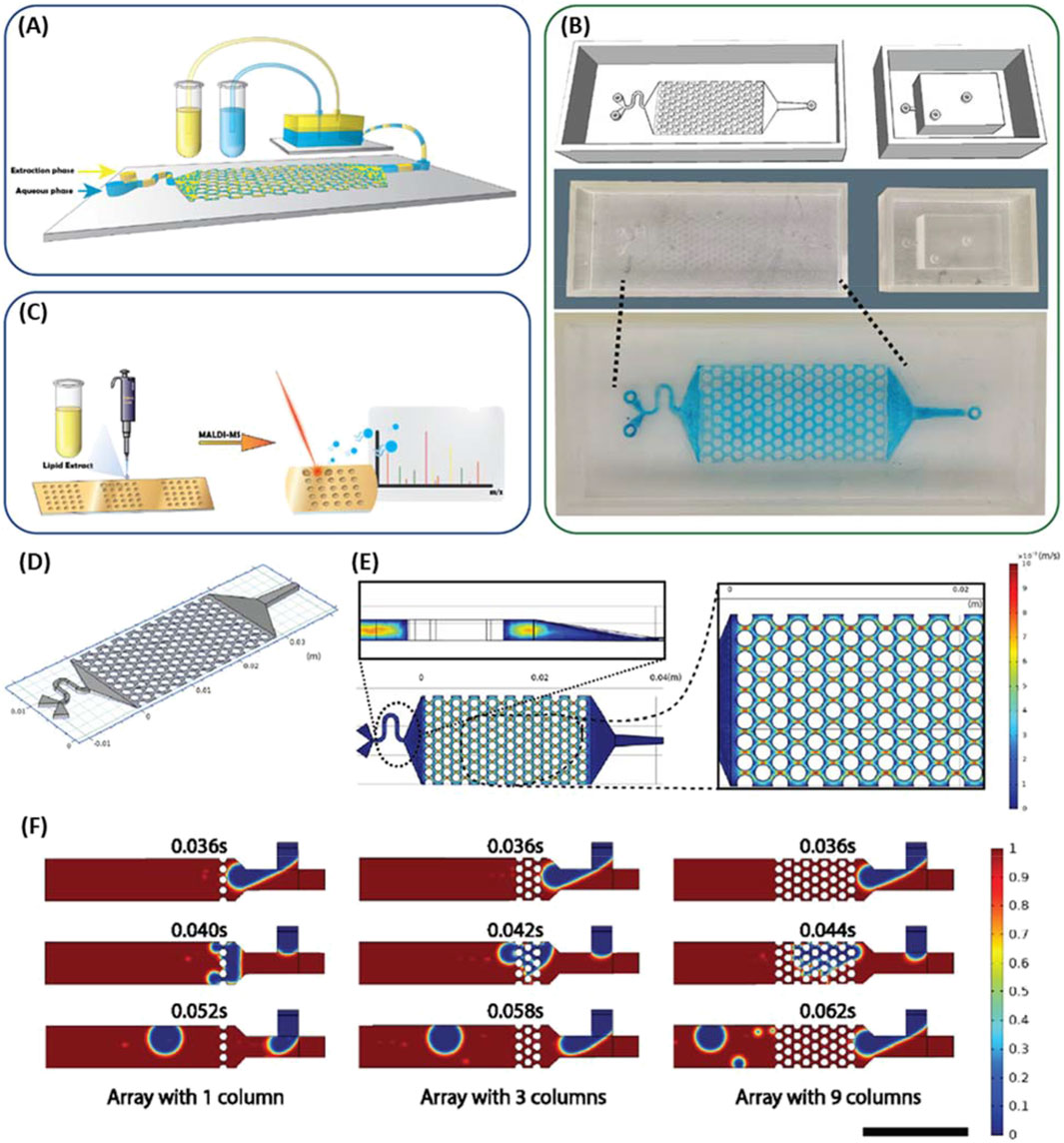
Figure 7. (A) Scheme of 3D printed microfluidic biochip platform for the mixing and separation of the extraction phases. (B) Computer generated print sketches of micromixer and reservoir, printed device with PMMA resin, and the image of the micromixer with filled dye solution in blue. (C) Schematic of workflow for lipid extract loading on the microarray chip for matrix assisted laser desorption ionization time of flight mass spectrometry. (D) Geometry of micromixer for the velocity field simulation. (E) Single phase velocity simulation for water in the micromixer with a focus on the velocity field plot for a sample longitudinal section and cross section. (F) Simulation of the droplet formation in the microfluidics’ T-junction and how the droplets breakup in the microfluidic channels with varying number of columns (Bar = 500 μm). Adapted from (Yang Z. et al., 2022).
Beginning with work by Fee et al. (2014), the past decade has seen significant interest in harnessing the high degree of control conferred by 3D printing to produce highly ordered and efficiently packed chromatographic columns. Approaching the theoretical limits of separation in liquid chromatography columns is not achieved under many of the prevaling industrial fabrication processes that involve randomly packing a slurry of particles into the column (Dolamore et al., 2018). While improvements in separation efficiency and speed have long been driven by reducing particle size, there is a limit that is being quickly approached, leaving packing orientation as an attractive alternative (Salmean and Dimartino, 2019). Theoretical work has long indicated that ordered particle distributions such as in the face centered cubic (FCC) orientation results in lower theoretical plate heights, and by extention, better separation efficiency (Schure et al., 2004). Unfortunately, the often competing factors of print speed, accuracy, and resolution have limited the viability of producing larger 3D printed liquid chromatography columns that can actually outperform commercial columns. To achieve pore/particle sizes of appropriately small size (<2 μm) requires the usage of high resolution methods such as T-PP, which severely limits the column sizes that can be produced. This conflict was recently demonstrated by Matheuse et al. who were able to achieve pore sizes as low as 500 nm using T-PP at the cost of a 470 h print time for a 75 μm × 15 cm column (Matheuse et al., 2022). Beyond the print speed limitations, T-PP lacks compatible resins with ideal chemical and physical properties. An example of recent work attempting to resolve the speed limitation without compromising too heavily on feature size has come from Gritti and Nawata, in which they developed a 3D printing technique that combines stereolithography and photolithography. Dubbed hybrid stereolithography, they were able to achieve resolutions as low as 20 μm with print times not exceeding a few hours (Gritti and Nawada, 2022). Unfortunately, due to the feature size and printing innacuracies, the column was not able to achieve plate heights near those of commercial columns. For a more comprehensive review on progress and challenges in 3D printed chromatography materials, readers are referred to a recent paper by Salmean and Dimartino (2019). Though they still have a long way to go, recent advances in 3D printing technology have shown promise to resolve some of these issues and improve the prospect of rapidly producable and highly customizable liquid chromatography columns.
Electrophoresis has been a highly effective separation technique for decades and the introduction of 3D printing systems provide many of the same opportunities for application to electrophoretic mobility as other separation technologies. This topic has been discussed in depth by Esene et al. (2023) but has seen some major advancements in recent years that warrant discussion. Notably 3D printed microfluidic channels can be interfaced with electrophoresis to enable separation and detection. This has been demonstrated by Selemani and Martin for various neurotransmitters, finding the ability to separate dopamine, catechol, and Dopac using a 3D printed device with integrated microwire electrodes (Selemani and Martin, 2024). A microchip electrophoresis device has also been interfaced with solid-phase extraction to enable the detection of preterm birth biomarkers (PTB) (Esene et al., 2024). They captured PTB biomarkers on an SPE monolith and then eluted them for separation via electrophoresis where four of these biomarkers were detected in the complex matrix of 50% serum. This demonstrates the potential of utilizing 3D printing to develop electrophoretic separation systems and that these systems can be interfaced with other 3D printed systems for extraction or detection.
As demonstrated above, one aspect of extraction that is necessary for analytical instrumentation but is often overlooked is the collection of biologically relevant matrices such as blood/serum (Hoffman et al., 2023), sweat (Yang P. et al., 2022), and saliva (Liao et al., 2023). Advancements in 3D printed microfluidic methodologies for simple extraction of these important biological fluids have been demonstrated in recent years, thereby providing a foundation for 3D printing devices to collect, process, and analyze blood/serum samples. For example, the extraction of blood has been enabled through printing of hollow microneedle structures that can puncture and extract blood through microfluidic channels (Cheng et al., 2023). Microfluidic systems have also been extensively employed for collection of sweat through wearable patches that collect and concentrate sweat into internal microfluidic channels (Chung-Han Wu et al., 2023; Wei et al., 2022). Saliva can be interfaced to microfluidic devices for extraction, separation, or detection through ports that accommodate insertion of commonly utilized oral swabs (Lee et al., 2020). Alternatively, 3D printed mouthguards that contains a paper microfluidic system to collect and test saliva glucose levels have been demonstrated (de Castro et al., 2019). Once collected, these fluids were subjected to further separation or immediately linked to a sensing component for analyte detection.
These examples clearly demonstrate that 3D printed fluidic systems have enabled extraction and separation techniques to be consolidated into easily fabricated devices, which have been further applied to reduce time of operation and simplify complex processes. Similarly, biological fluid extraction has been enabled through printed fluidic systems. The use of 3D printed microfluidics will likely continue to see significant growth in coming years for both separation and separation-facilitated sensing applications.
5.2 3D printed sensors
Clearly sample extraction and separation can be readily achieved within a 3D printed sensing device, and the versatility of 3D printing can further enable microfluidic channels to be modified with sensing applications in mind. This is particularly evident in recent work focused on point-of-care sensors where 3D printing can rapidly fabricate inexpensive fluidic devices for the detection of disease biomarkers. An important aspect of these devices is the introduction of capture and sensing components into the 3D printed fluidic system. Capture systems vary, but commonly rely on affinity interactions to selectively collect the target analyte, whereas the sensing itself is generally achieved through the use of electrochemical or optical methods (Muñoz and Pumera, 2020; Abdalla and Patel, 2021). A popular recent use for 3D printed microfluidics sensors has been the rapid detection of SARS-CoV-2 using both electrochemical (de Matos Morawski et al., 2023; Muhsin et al., 2023; Ali et al., 2022) and optical (Ning et al., 2023; Nguyen et al., 2022) techniques, which have been reviewed by Lin et al. (Lin et al., 2023).
For electrochemical sensing, the electrodes are typically integrated into the microfluidic channels. This can be realized in one of two ways. The first way is through implementation of conductive materials in the printing process (Ryan et al., 2022), as has been addressed in the previous section covering 3D printing materials. The other method is to integrate electrodes into the 3D printed flow channels by either inseting them after printing (Ambrosi et al., 2020; Capel et al., 2018) or by directly printing around the electrode (Li et al., 2019; Su et al., 2022). Both methods have demonstrated promise in enabling electrochemical sensing of a plethora of analytes within 3D printed microfluidic channels. For example, recent work by Chittuam et al. (2023) demonstrated the simultaneous detection of HIV-1 and HCV based on DNA hybridization using a layered microfluidics chip with fully printed electrodes. Ferreira et al. (2021) employed 3D printing to develop an electrochemical cell compatible with various working electrodes. They also employed a 3D pen to fabricate the embedded counter and reference electrodes. Development of portable electrochemical sensors has also become feasible owing to advances in 3D printing. Vinoth et al. demonstrated a point-of-care sensor for saliva biomarkers using passive microfluidic flow, filtration, electrochemical sensors, and a PCB microcontroller, all within a hand-sized device (Vinoth et al., 2023). While electrochemical methods have seen extensive integration with microfluidics systems, other methods can also be employed in conjunction to expand the sensing capability. Sikula et al. (2023) demonstrated a 3D printed spectroelectrochemical sensor that achieves both cyclic voltammetry and UV-Vis spectroscopy. This simultaneous collection of optical and electrochemical data, and the collection of spectroscopic data through optically transparent printed components, is a unique and innovative development.
Optical sensing methodologies have also seen substantial use in the development of microfluidic sensors. This has been accentuated by utilizing smartphone cameras for data acquisition where a 3D printed system is interfaced to measure light intensity change or a colorimetric response. Xiao et al. (2022) fabricated a handheld SPR instrument enabled by smartphone imaging, with 3D printed microfluidics and a casing that holds optical components in alignment. Shang et al. (2022) developed a microfluidic sensor for E. coli that utilizes DNA extraction and amplification with fluorescence detection (Figure 8). The entire system was built onto a single microfluidic chip controlled by finger actuation with detection achieved by smartphone camera. Magnetic nanoparticles were used to separate the bacteria from food samples followed by nucleic acid extraction/purification and finished with recombinase polymerase amplification combined with CRISPR/Cas12a for fluorescence detection. Another reported method utilizing 3D printed finger-powered microfluidic pumps demonstrated detection of myocardial biomarkers using surface enhanced Raman spectroscopy (Liu et al., 2023). Target biomarkers were captured via a sandwich immunoassay between magnetic beads and gold nanoparticles. Magnetic separation within a 3D printed microfluidic system has also been employed to detect okadaic acid via horseradish peroxidase through catalyzed color change detected by a smartphone (Ji et al., 2023). Recently Kumar et al. (2023) developed a portable chemiluminescence-based point-of-care sensor where the fluidic components were 3D printed and interfaced with a temperature controller and smartphone, enabling sample processing and detection of alkaline phosphatase all within a handheld device.
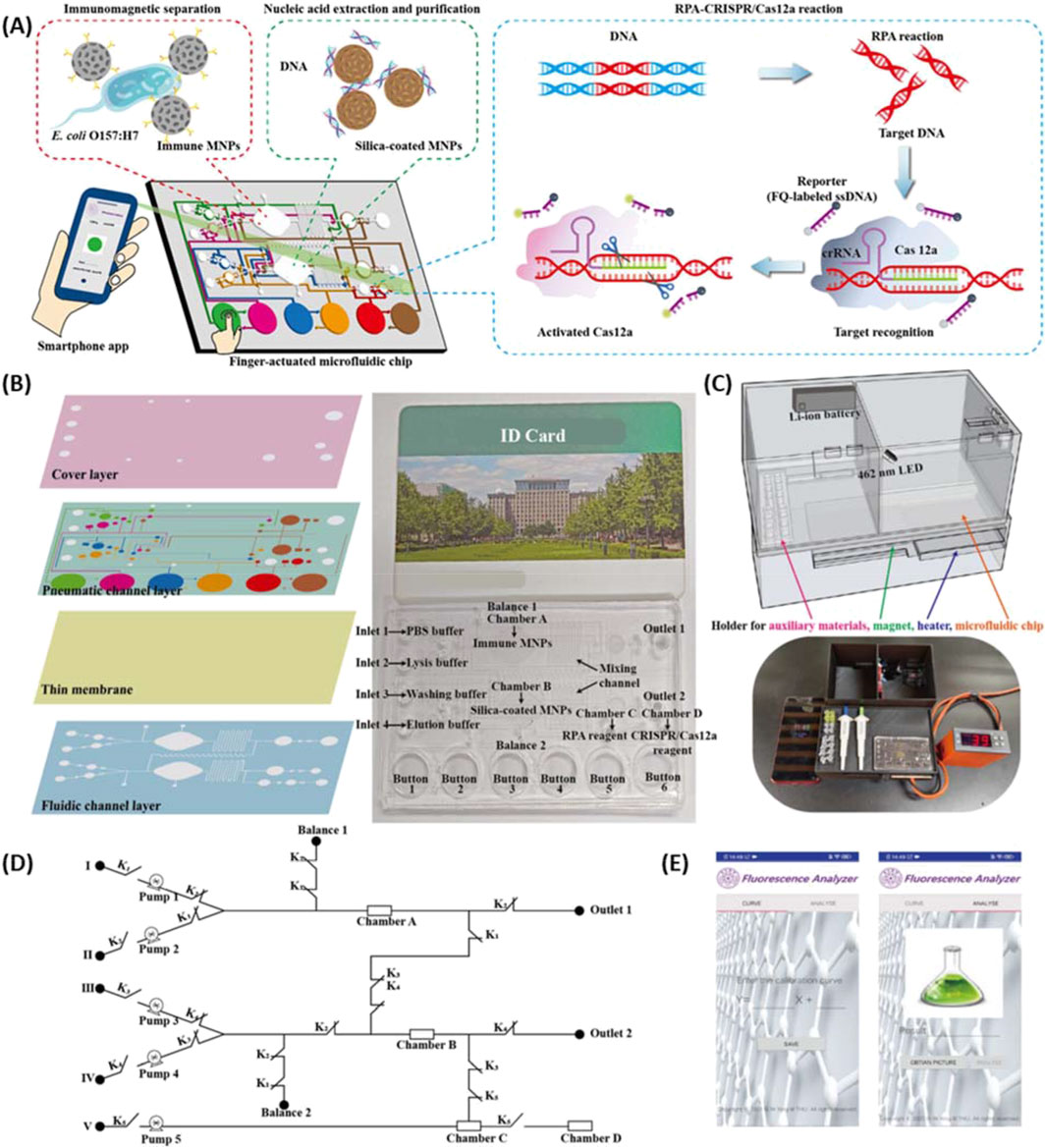
Figure 8. (A) Scheme of the finger actuated microfluidic chip for E. coli detection using RPA-CRISPR/Cas12a fluorescence collected via a smartphone app. (B) Design of the microfluidic chip made up of four layers (left) and image of combined microfluidic chip with id card for scale (right). (C) Model and image of the 3D printed device. (D) Circuit of the fluid control for the microfluidics. (E) Image of the smartphone app developed to interface with the sensor. Adapted from (Shang et al., 2022).
5.3 Cell growth and sorting
Enabling studies involving cell sorting and growth are another popular avenue for the use of 3D printed fluidics. One example is 3D printing of flow chambers that allow 3D growth of cells and organoids (Zheng et al., 2021). Organ-on-a-chip platforms have been fabricated for mimicking and testing cellular systems, and these uses have been previously reviewed (Milton et al., 2023; Saorin et al., 2023). 3D printing has also been expanded to printing with cells, biomaterials, and biomolecules, as discussed in a recent review on 3D bioprinting (Vanaei et al., 2021). Clearly 3D printing technology has been instrumental in enabling rapid development of technqiues for cell-based study and systems.
Recently, 3D printing has been used to faciliate the vascularization of organoids, one of the major limitations that has plagued 3D cell culture (Salmon et al., 2022; Grebenyuk et al., 2023; Homan et al., 2019). Salmon et al. (2022) utilized small microfluidic channels adjacent to the organoid growth chamber for vascular network growth that enabled synchronization of organoids and vasculature (Figure 9). With this system, organoids, endothelial cells, and pericytes from human pluripotent stem cells (hPSC) were cultured. Flow of endothelial cells and pericytes through channels next to the organoid chamber induced vascular growth similar to naturally occurring tumor systems. Grebenyuk et al. (2023) employed a basket like grid of 3D printed microfluidic capilaries to hold and perfuse organoids as they grew. Homan et al. (2019) developed a microfluidic chamber and tested it by allowing for the maturation of organoids, which showed improved vascularization. 3D printing has been utilized to develop a microfluidic chamber for organoid growth that can be interfaced with microscopy instrumentation for imaging (Khan et al., 2021). These systems rely on continual flow from nonprinted pump systems for cell perfussion. Dhwaj et al. (2022) recently demonstrated a 3D printed impedance pump based on repeated tapping on a small portion of tubing for driving flow forward and employed it to maintin liver cell growth. The production of pumping systems for organoid growth is a promising use for 3D printing moving forward.
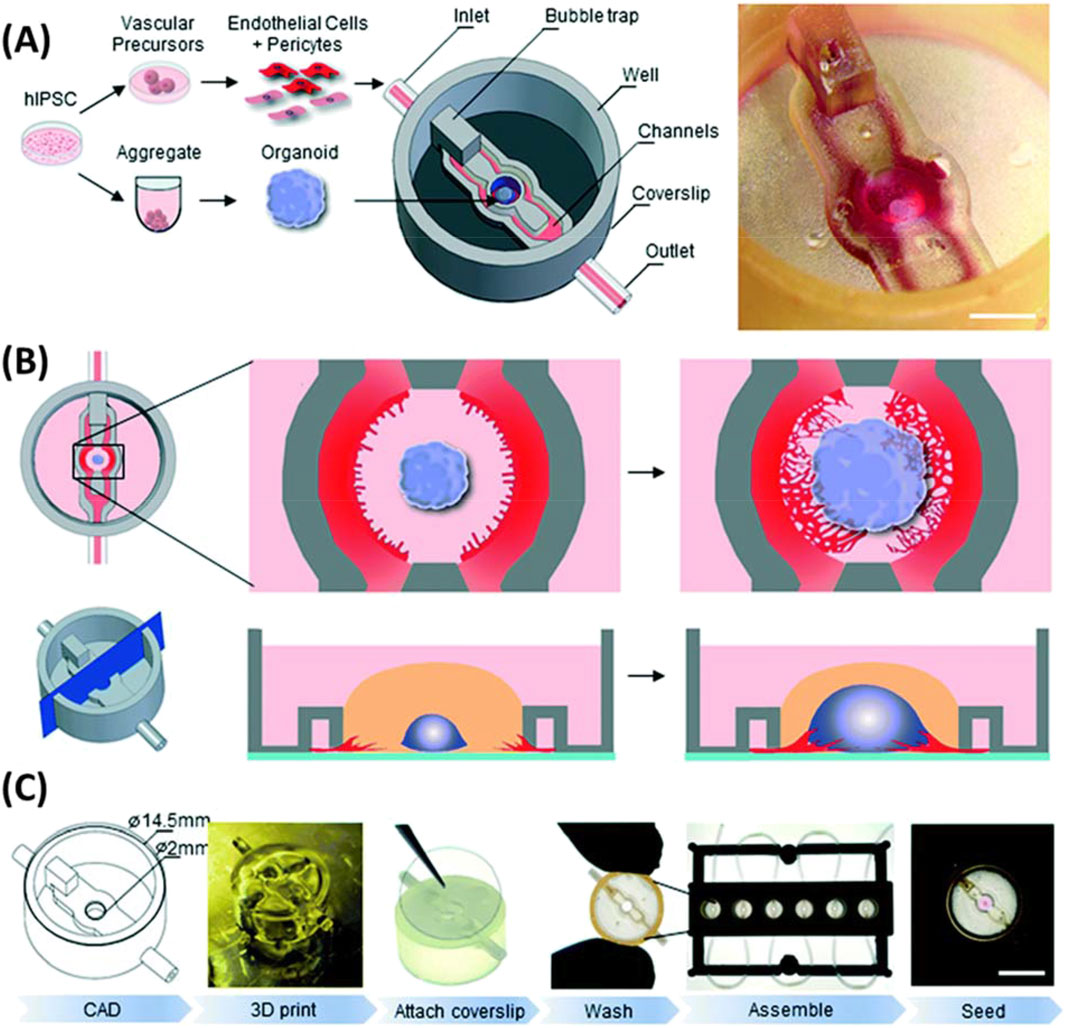
Figure 9. Microfluidics platform utilized for growth of vascularized organoids. (A) With growth of organoids and vascular precursors from the same human induced pluripotent stem cell culture and implemented into a microfluidics chip. (B) Allowing for vascularization to grow into the organoid from adjacent channels with endothelial cells and pericytes. (C) Workflow of chip fabrication and organoid seeding. Adapted from (Salmon et al., 2022).
Another avenue where 3D printed microfluidics have found notable use is as a cheap and customizable method for cell sorting. Ding et al. (2022) developed a system composed of multiple printed units to mix, separate, and concentrate cells. To upscale the system to enable a large scale cell harvesting, Zhu et al. (2020) employed 3D printing to fabricate a system for extraction of white blood cells from whole blood. The system contained modules for blood mixing, lysis of red blood cells, and subsequent extraction of white blood cells. A few studies have also employed 3D printed microfluidics for malignant tumor cell sorting from red and white blood cells (Jiang and Xiang, 2022; Xu et al., 2022). Others have utilized 3D printed fluidics to develop an immunomagnetic platform for cell sorting based on phenotype (Philpott et al., 2022). Wang et al. (2023) developed one of these platforms to separate and analyze circulating tumor-reactive lymphocytes, and printed microfluidics were utilized for cell counting in blood cell analysis, as demonstrated by Yan et al. (2022) In this work, the change in resistance caused by each cell passing through a small hole between the electrodes is measured, thereby allowing cells to be counted.
The adaptability of 3D printing has inspired many applications where microfluidics are integrated or utilized within. The ability to fabricate any conceivable fluidic geometry with ease at low cost has allowed a plethora of different systems to be built. In addition to the applications mentioned above, there are various other topics including reaction catalysis (Pose-Boirazian et al., 2022) and drug encapsulation (Jia et al., 2022) that have been reviewed.
6 Conclusion
This review has summarized the current trends and advancements in the field of 3D printing that are closely related to the construction of analytical instrumentation. The main focus of this work is placed on recent progress in the production of 3D printed components, their implementation in sensing applications, and how 3D printing of an entire analytical instrument could be envisioned, designed, and realized. Highlighted here are the advancements in 3D printing techniques and materials, currently available and being developed, for the creation of optical, microfluidic, mechanical, and structural components. 3D printing has shortened prototyping time and provided cost improvements but is still limited to a small set of feasible materials. However, this material space has significantly expanded in recent years with new printing systems and methods, an area we expect to observe continued growth in the coming years. With the capabilities of these new materials, their extension to sensing applications has become a significant trend that will be fueled by the continual introduction of new materials. The field is progressing rapidly, and it is clear that it has reached a point that one could practically 3D print all of the key components of an analytical platform, except the light or other forms of source and the electronic detector. Based on this, we expect to see 3D printing applications continue to expand. It is clear with time the 3D printers and their source materials will get progressively cheaper, continuously making 3D printing more and more accessible. Furthermore, as new methodologies are developed and implemented the resolution capabilities of 3D printing systems are bound to improve. These advancements are key to the future utilization of 3D printing in analytical instrumentation where small parts or flow channels are currently 3D printing limited. We could envision a 3D printer in nearly every academic lab in the near future due to the incredible versatility it provides in creating diverse devices from the simplest instrument support to complex sample handling devices.
Author contributions
AM: Conceptualization, Formal Analysis, Investigation, Writing–original draft, Writing–review and editing. CE: Conceptualization, Investigation, Writing–original draft, Writing–review and editing. DS: Conceptualization, Investigation, Writing–original draft, Writing–review and editing. SV: Conceptualization, Investigation, Writing–original draft, Writing–review and editing. VH: Writing–original draft, Writing–review and editing. QC: Conceptualization, Formal Analysis, Funding acquisition, Investigation, Project administration, Supervision, Validation, Writing–original draft, Writing–review and editing.
Funding
The author(s) declare that financial support was received for the research, authorship, and/or publication of this article. We would like to acknowledge the financial support from NSF (CHE-2109042).
Conflict of interest
The authors declare that the research was conducted in the absence of any commercial or financial relationships that could be construed as a potential conflict of interest.
The author(s) declared that they were an editorial board member of Frontiers, at the time of submission. This had no impact on the peer review process and the final decision.
Generative AI statement
The author(s) declare that no Generative AI was used in the creation of this manuscript.
Publisher’s note
All claims expressed in this article are solely those of the authors and do not necessarily represent those of their affiliated organizations, or those of the publisher, the editors and the reviewers. Any product that may be evaluated in this article, or claim that may be made by its manufacturer, is not guaranteed or endorsed by the publisher.
References
Abdalla, A., and Patel, B. A. (2021). 3D printed electrochemical sensors. Annu. Rev. Anal. Chem. (Palo Alto Calif) 14 (1), 47–63. doi:10.1146/annurev-anchem-091120-093659
Agrawaal, H., and Thompson, J. (2021). Additive manufacturing (3D printing) for analytical chemistry. Talanta Open 3, 100036. doi:10.1016/j.talo.2021.100036
Ahn, D., Stevens, L. M., Zhou, K., and Page, Z. A. (2020). Rapid high-resolution visible light 3D printing. ACS central Sci. 6 (9), 1555–1563. doi:10.1021/acscentsci.0c00929
Al-Dulaijan, Y. A., Alsulaimi, L., Alotaibi, R., Alboainain, A., Alalawi, H., Alshehri, S., et al. (2022). Comparative evaluation of surface roughness and hardness of 3D printed resins. Materials 15 (19), 6822. doi:10.3390/ma15196822
Ali, M., Alam, F., Ahmed, I., AlQattan, B., Yetisen, A. K., and Butt, H. (2021). 3D printing of Fresnel lenses with wavelength selective tinted materials. Addit. Manuf. 47, 102281. doi:10.1016/j.addma.2021.102281
Ali, M. A., Zhang, G. F., Hu, C., Yuan, B., Jahan, S., Kitsios, G. D., et al. (2022). Ultrarapid and ultrasensitive detection of SARS-CoV-2 antibodies in COVID-19 patients via a 3D-printed nanomaterial-based biosensing platform. J. Med. Virol. 94 (12), 5808–5826. doi:10.1002/jmv.28075
Almughamsi, H. M., Howell, M. K., Parry, S. R., Esene, J. E., Nielsen, J. B., Nordin, G. P., et al. (2022). Immunoaffinity monoliths for multiplexed extraction of preterm birth biomarkers from human blood serum in 3D printed microfluidic devices. Analyst 147 (4), 734–743. doi:10.1039/d1an01365c
Ambrosi, A., and Bonanni, A. (2021). How 3D printing can boost advances in analytical and bioanalytical chemistry. Microchim. Acta 188. doi:10.1007/s00604-021-04901-2
Ambrosi, A., Shi, R. R. S., and Webster, R. D. (2020). 3D-printing for electrolytic processes and electrochemical flow systems. J. Mater. Chem. A 8 (42), 21902–21929. doi:10.1039/d0ta07939a
Amini, A., Guijt, R. M., Themelis, T., De Vos, J., and Eeltink, S. (2023). Recent developments in digital light processing 3D-printing techniques for microfluidic analytical devices. J. Chromatogr. A 1692, 463842. doi:10.1016/j.chroma.2023.463842
Apparao, D., and Raju, M. J. (2021). Design and analysis of spur gear manufactured by DMLS process. Mater. Today Proc. 46, 149–153. doi:10.1016/j.matpr.2020.07.078
Arena, M., Ambrogiani, P., Raiola, V., Bocchetto, F., Tirelli, T., and Castaldo, M. (2023). Design and qualification of an additively manufactured manifold for aircraft landing gears applications. Aerospace 10 (1), 69. doi:10.3390/aerospace10010069
Aslani, V., Toulouse, A., Schmid, M., Giessen, H., Haist, T., and Herkommer, A. (2023). 3D printing of colored micro-optics. Opt. Mater. Express 13 (5), 1372. doi:10.1364/ome.489681
Balakrishnan, H. K., Doeven, E. H., Merenda, A., Dumée, L. F., and Guijt, R. M. (2021). 3D printing for the integration of porous materials into miniaturised fluidic devices: a review. Anal. Chim. acta 1185, 338796. doi:10.1016/j.aca.2021.338796
Belkilani, M., Farre, C., Chevalier, Y., Minot, S., Bessueille, F., Abdelghani, A., et al. (2022). Mechanisms of influenza virus HA2 peptide interaction with liposomes studied by dual-wavelength MP-SPR. ACS Appl. Mater. Interfaces 14 (29), 32970–32981. doi:10.1021/acsami.2c09039
Berger, U. (2015). Aspects of accuracy and precision in the additive manufacturing of plastic gears. Virtual Phys. Prototyp. 10 (2), 49–57. doi:10.1080/17452759.2015.1026127
Berglund, G., Wisniowiecki, A., Gawedzinski, J., Applegate, B., and Tkaczyk, T. S. (2022). Additive manufacturing for the development of optical/photonic systems and components. Optica 9 (6), 623. doi:10.1364/optica.451642
Bhaduri, D., Penchev, P., Batal, A., Dimov, S., Soo, S. L., Sten, S., et al. (2017). Laser polishing of 3D printed mesoscale components. Appl. Surf. Sci. 405, 29–46. doi:10.1016/j.apsusc.2017.01.211
Bhaiyya, M., Pattnaik, P. K., and Goel, S. (2022). Multiplexed and simultaneous biosensing in a 3D-printed portable six-well smartphone operated electrochemiluminescence standalone point-of-care platform. Mikrochim. Acta 189 (2), 79. doi:10.1007/s00604-022-05200-0
Bhuvanesh Kumar, M., and Sathiya, P. (2021). Methods and materials for additive manufacturing: a critical review on advancements and challenges. Thin-Walled Struct. 159, 107228. doi:10.1016/j.tws.2020.107228
Bishop, G. W., Satterwhite-Warden, J. E., Kadimisetty, K., and Rusling, J. F. (2016). 3D-printed bioanalytical devices. Nanotechnology 27 (28), 284002. doi:10.1088/0957-4484/27/28/284002
Blachowicz, T., Ehrmann, G., and Ehrmann, A. (2021). Optical elements from 3D printed polymers. e-Polymers 21 (1), 549–565. doi:10.1515/epoly-2021-0061
Bogucki, R., Greggila, M., Mallory, P., Feng, J., Siman, K., Khakipoor, B., et al. (2019). A 3D-printable dual beam spectrophotometer with multiplatform smartphone adaptor. J. Chem. Educ. 96 (7), 1527–1531. doi:10.1021/acs.jchemed.8b00870
Braun, A., and Maier, S. A. (2016). Versatile direct laser writing lithography technique for surface enhanced infrared spectroscopy sensors. ACS Sensors 1 (9), 1155–1162. doi:10.1021/acssensors.6b00469
Buj-Corral, I., and Zayas-Figueras, E. E. (2023). Comparative study about dimensional accuracy and form errors of FFF printed spur gears using PLA and Nylon. Polym. Test. 117, 107862. doi:10.1016/j.polymertesting.2022.107862
Capel, A. J., Rimington, R. P., Lewis, M. P., and Christie, S. D. R. (2018). 3D printing for chemical, pharmaceutical and biological applications. Nat. Rev. Chem. 2 (12), 422–436. doi:10.1038/s41570-018-0058-y
Cardoso, R. M., Kalinke, C., Rocha, R. G., Dos Santos, P. L., Rocha, D. P., Oliveira, P. R., et al. (2020). Additive-manufactured (3D-printed) electrochemical sensors: a critical review. Anal. Chim. acta 1118, 73–91. doi:10.1016/j.aca.2020.03.028
Carlotti, M., and Mattoli, V. (2019). Functional materials for two-photon polymerization in microfabrication. Small 15 (40), 1902687. doi:10.1002/smll.201902687
Carrasco-Correa, E. J., Cocovi-Solberg, D. J., Herrero-Martínez, J. M., Simó-Alfonso, E. F., and Miró, M. (2020). 3D printed fluidic platform with in-situ covalently immobilized polymer monolithic column for automatic solid-phase extraction. Anal. Chim. Acta 1111, 40–48. doi:10.1016/j.aca.2020.03.033
Carrasco-Correa, E. J., Simó-Alfonso, E. F., Herrero-Martínez, J. M., and Miró, M. (2021). The emerging role of 3D printing in the fabrication of detection systems. TrAC Trends Anal. Chem. 136, 116177. doi:10.1016/j.trac.2020.116177
Casto, L. D., Do, K. B., and Baker, C. A. (2019). A miniature 3D printed LED-induced fluorescence detector for capillary electrophoresis and dual-detector taylor dispersion analysis. Anal. Chem. 91 (15), 9451–9457. doi:10.1021/acs.analchem.8b05824
Catala-Castro, F., Schaffer, E., and Krieg, M. (2022). Exploring cell and tissue mechanics with optical tweezers. J. Cell Sci. 135 (15), jcs259355. doi:10.1242/jcs.259355
Cecil, F., Guijt, R. M., Henderson, A. D., Macka, M., and Breadmore, M. C. (2020). One step multi-material 3D printing for the fabrication of a photometric detector flow cell. Anal. Chim. Acta 1097, 127–134. doi:10.1016/j.aca.2019.10.075
Chada, N., Sigdel, K. P., Gari, R. R. S., Matin, T. R., Randall, L. L., and King, G. M. (2015). Glass is a viable substrate for precision force microscopy of membrane proteins. Sci. Rep. 5, 12550. doi:10.1038/srep12550
Chan, H. N., Tan, M. J. A., and Wu, H. (2017). Point-of-care testing: applications of 3D printing. Lab. Chip 17 (16), 2713–2739. doi:10.1039/c7lc00397h
Chen, J.-Y., Hwang, J. V., Ao-Ieong, W. S., Lin, Y. C., Hsieh, Y. K., Cheng, Y. L., et al. (2018). Study of physical and degradation properties of 3D-printed biodegradable, photocurable copolymers, PGSA-co-PEGDA and PGSA-co-PCLDA. Polymers 10 (11), 1263. doi:10.3390/polym10111263
Cheng, J., Huang, J., Xiang, Q., and Dong, H. (2023). Hollow microneedle microfluidic paper-based chip for biomolecules rapid sampling and detection in interstitial fluid. Anal. Chim. Acta 1255, 341101. doi:10.1016/j.aca.2023.341101
Chittuam, K., Jampasa, S., Vilaivan, T., Tangkijvanich, P., Chuaypen, N., Avihingsanon, A., et al. (2023). Electrochemical capillary-driven microfluidic DNA sensor for HIV-1 and HCV coinfection analysis. Anal. Chim. Acta 1265, 341257. doi:10.1016/j.aca.2023.341257
Chu, S., Wang, H., Du, Y., Yang, F., Yang, L., and Jiang, C. (2020). Portable smartphone platform integrated with a nanoprobe-based fluorescent paper strip: visual monitoring of glutathione in human serum for health prognosis. ACS Sustain. Chem. and Eng. 8 (22), 8175–8183. doi:10.1021/acssuschemeng.0c00690
Chung-Han Wu, H. J. H. M., Paul, B., Balanay, R. K., Ray, T. R., and Ray, T. R. (2023). Skin-interfaced microfluidic systems with spatially engineered 3D fluidics for sweat capture and analysis. Sci. Adv. 9 (18), eadg4272. doi:10.1126/sciadv.adg4272
Darder, M. d.M., Serrano, L. A., Bedoya, M., and Orellana, G. (2022). 3D printing filaments facilitate the development of evanescent wave plastic optical fiber (POF) chemosensors. Chemosensors 10 (2), 61. doi:10.3390/chemosensors10020061
da Silva, E. K. N., dos Santos, V. B., Resque, I. S., Neves, C. A., Moreira, S. G., Franco, M. d. O., et al. (2020). A fluorescence digital image-based method using a 3D-printed platform and a UV-LED chamber made of polyacid lactic for quinine quantification in beverages. Microchem. J. 157, 104986. doi:10.1016/j.microc.2020.104986
Dawood, A., Marti Marti, B., Sauret-Jackson, V., and Darwood, A. (2015). 3D printing in dentistry. Br. Dent. J. 219 (11), 521–529. doi:10.1038/sj.bdj.2015.914
de Castro, L. F., de Freitas, S. V., Duarte, L. C., de Souza, J. A. C., Paixão, T. R. L. C., and Coltro, W. K. T. (2019). Salivary diagnostics on paper microfluidic devices and their use as wearable sensors for glucose monitoring. Anal. Bioanal. Chem. 411 (19), 4919–4928. doi:10.1007/s00216-019-01788-0
Del Rosario, M., Heil, H. S., Mendes, A., Saggiomo, V., and Henriques, R. (2022). The field guide to 3D printing in optical microscopy for life sciences. Adv. Biol. 6 (4), 2100994. doi:10.1002/adbi.202100994
de Matos Morawski, F., Martins, G., Ramos, M. K., Zarbin, A. J. G., Blanes, L., Bergamini, M. F., et al. (2023). A versatile 3D printed multi-electrode cell for determination of three COVID-19 biomarkers. Anal. Chim. Acta 1258, 341169. doi:10.1016/j.aca.2023.341169
Destino, J. F., Dudukovic, N. A., Johnson, M. A., Nguyen, D. T., Yee, T. D., Egan, G. C., et al. (2018). 3D printed optical quality silica and silica–titania glasses from sol–gel feedstocks. Adv. Mater. Technol. 3 (6). doi:10.1002/admt.201700323
Dhwaj, A., Roy, N., Jaiswar, A., Prabhakar, A., and Verma, D. (2022). 3D-Printed impedance micropump for continuous perfusion of the sample and nutrient medium integrated with a liver-on-chip prototype. ACS Omega 7 (45), 40900–40910. doi:10.1021/acsomega.2c03818
Ding, L., Razavi Bazaz, S., Asadniaye Fardjahromi, M., McKinnirey, F., Saputro, B., Banerjee, B., et al. (2022). A modular 3D printed microfluidic system: a potential solution for continuous cell harvesting in large-scale bioprocessing. Bioresour. Bioprocess. 9 (1), 64. doi:10.1186/s40643-022-00550-2
Dingeldein, J. C., Walczak, K. A., Swatowski, B. W., Friedrich, C. R., Middlebrook, C. T., and Roggemann, M. C. (2013). Process characterization for direct dispense fabrication of polymer optical multi-mode waveguides. J. Micromechanics Microengineering 23 (7), 075015. doi:10.1088/0960-1317/23/7/075015
Dizon, J. R. C., Gache, C. C. L., Cascolan, H. M. S., Cancino, L. T., and Advincula, R. C. (2021). Post-processing of 3D-printed polymers. Technologies 9 (3), 61. doi:10.3390/technologies9030061
Dolamore, F., Fee, C., and Dimartino, S. (2018). Modelling ordered packed beds of spheres: the importance of bed orientation and the influence of tortuosity on dispersion. J. Chromatogr. A 1532, 150–160. doi:10.1016/j.chroma.2017.12.004
Duarte, L. C., Pereira, I., Maciel, L. I. L., Vaz, B. G., and Coltro, W. K. T. (2022). 3D printed microfluidic mixer for real-time monitoring of organic reactions by direct infusion mass spectrometry. Anal. Chim. Acta 1190, 339252. doi:10.1016/j.aca.2021.339252
Erokhin, K. S., Gordeev, E. G., and Ananikov, V. P. (2019). Revealing interactions of layered polymeric materials at solid-liquid interface for building solvent compatibility charts for 3D printing applications. Sci. Rep. 9 (1), 20177. doi:10.1038/s41598-019-56350-w
Esene, J. E., Burningham, A. J., Tahir, A., Nordin, G. P., and Woolley, A. T. (2024). 3D printed microfluidic devices for integrated solid-phase extraction and microchip electrophoresis of preterm birth biomarkers. Anal. Chim. Acta 1296, 342338. doi:10.1016/j.aca.2024.342338
Esene, J. E., Nasman, P. R., Akuoko, Y., Tahir, A., and Woolley, A. T. (2023). Past, current, and future roles of 3D printing in the development of capillary electrophoresis systems. TrAC Trends Anal. Chem. 162, 117032. doi:10.1016/j.trac.2023.117032
Fee, C., Nawada, S., and Dimartino, S. (2014). 3D printed porous media columns with fine control of column packing morphology. J. Chromatogr. A 1333, 18–24. doi:10.1016/j.chroma.2014.01.043
Feng, D., Li, H., Xu, T., Zheng, F., Hu, C., Shi, X., et al. (2022). High-throughput single cell metabolomics and cellular heterogeneity exploration by inertial microfluidics coupled with pulsed electric field-induced electrospray ionization-high resolution mass spectrometry. Anal. Chim. Acta 1221, 340116. doi:10.1016/j.aca.2022.340116
Fernandes, Q. R., Jesus, D. P. d., and Fracassi da Silva, J. A. (2023). Simple modification to allow high-efficiency and high-resolution multi-material 3D-printing fabrication of microfluidic devices. Lab a Chip 23 (16), 3694–3703. doi:10.1039/d3lc00356f
Ferreira, P. A., de Oliveira, F. M., de Melo, E. I., de Carvalho, A. E., Lucca, B. G., Ferreira, V. S., et al. (2021). Multi sensor compatible 3D-printed electrochemical cell for voltammetric drug screening. Anal. Chim. Acta 1169, 338568. doi:10.1016/j.aca.2021.338568
Gadagi, B., and Lekurwale, R. (2021). A review on advances in 3D metal printing. Mater. Today Proc. 45, 277–283. doi:10.1016/j.matpr.2020.10.436
García-García, R., and González-Palacios, M. A. (2018). Method for the geometric modeling and rapid prototyping of involute bevel gears. Int. J. Adv. Manuf. Technol. 98 (1-4), 645–656. doi:10.1007/s00170-018-2246-9
Gastaldi, M., Cardano, F., Zanetti, M., Viscardi, G., Barolo, C., Bordiga, S., et al. (2020). Functional dyes in polymeric 3D printing: applications and perspectives. ACS Mater. Lett. 3 (1), 1–17. doi:10.1021/acsmaterialslett.0c00455
Gonzalez-Hernandez, D., Varapnickas, S., Merkininkaitė, G., Čiburys, A., Gailevičius, D., Šakirzanovas, S., et al. (2021). Laser 3D printing of inorganic free-form micro-optics. Photonics 8 (12), 577. doi:10.3390/photonics8120577
Gorkowski, K., Donahue, N. M., and Sullivan, R. C. (2020). Aerosol optical tweezers constrain the morphology evolution of liquid-liquid phase-separated atmospheric particles. Chem 6 (1), 204–220. doi:10.1016/j.chempr.2019.10.018
Grajewski, M., Hermann, M., Oleschuk, R. D., Verpoorte, E., and Salentijn, G. I. (2021). Leveraging 3D printing to enhance mass spectrometry: a review. Anal. Chim. Acta 1166, 338332. doi:10.1016/j.aca.2021.338332
Grebenyuk, S., Abdel Fattah, A. R., Kumar, M., Toprakhisar, B., Rustandi, G., Vananroye, A., et al. (2023). Large-scale perfused tissues via synthetic 3D soft microfluidics. Nat. Commun. 14 (1), 193. doi:10.1038/s41467-022-35619-1
Gritti, F., and Nawada, S. (2022). On the road toward highly efficient and large volume three-dimensional-printed liquid chromatography columns? J. Sep. Sci. 45 (17), 3232–3240. doi:10.1002/jssc.202100962
Gross, B., Lockwood, S. Y., and Spence, D. M. (2017). Recent advances in analytical chemistry by 3D printing. Anal. Chem. 89 (1), 57–70. doi:10.1021/acs.analchem.6b04344
Gul, I., Aer, L., Zhang, M., Jiang, H., Khan, A. A., Bilal, M., et al. (2021). Multifunctional 3D-printed platform integrated with a smartphone ambient light sensor for halocarbon contaminants monitoring. Environ. Technol. and Innovation 24, 101883. doi:10.1016/j.eti.2021.101883
Guo, H., Lv, R., and Bai, S. (2019). Recent advances on 3D printing graphene-based composites. Nano Mater. Sci. 1 (2), 101–115. doi:10.1016/j.nanoms.2019.03.003
Gupta, V., and Paull, B. (2021). PolyJet printed high aspect ratio three-dimensional bifurcating microfluidic flow distributor and its application in solid-phase extraction. Anal. Chim. Acta 1168, 338624. doi:10.1016/j.aca.2021.338624
Guttridge, C., Shannon, A., O'Sullivan, A., O'Sullivan, K. J., and O'Sullivan, L. W. (2022). Biocompatible 3D printing resins for medical applications: a review of marketed intended use, biocompatibility certification, and post-processing guidance. Ann. 3D Print. Med. 5, 100044. doi:10.1016/j.stlm.2021.100044
Habib, T., Brämer, C., Heuer, C., Ebbecke, J., Beutel, S., and Bahnemann, J. (2022). 3D-Printed microfluidic device for protein purification in batch chromatography. Lab. Chip 22 (5), 986–993. doi:10.1039/d1lc01127h
Hai, R., Shao, G., Ware, H. O. T., Jones, E. H., and Sun, C. (2023). 3D printing a low-cost miniature accommodating optical microscope. Adv. Mater 35 (20), e2208365. doi:10.1002/adma.202208365
Hartcher-O’Brien, J., Evers, J., and Tempelman, E. (2019). Surface roughness of 3D printed materials: comparing physical measurements and human perception. Mater. Today Commun. 19, 300–305. doi:10.1016/j.mtcomm.2019.01.008
He, Z., Lee, Y. H., Chanda, D., and Wu, S. T. (2018). Adaptive liquid crystal microlens array enabled by two-photon polymerization. Opt. Express 26 (16), 21184–21193. doi:10.1364/OE.26.021184
Heikkinen, I. T., Kauppinen, C., Liu, Z., Asikainen, S. M., Spoljaric, S., Seppälä, J. V., et al. (2018). Chemical compatibility of fused filament fabrication-based 3-D printed components with solutions commonly used in semiconductor wet processing. Addit. Manuf. 23, 99–107. doi:10.1016/j.addma.2018.07.015
Hengsteler, J., Mandal, B., van Nisselroy, C., Lau, G. P. S., Schlotter, T., Zambelli, T., et al. (2021). Bringing electrochemical three-dimensional printing to the nanoscale. Nano Lett. 21 (21), 9093–9101. doi:10.1021/acs.nanolett.1c02847
Heuer, C., Preuss, J. A., Buttkewitz, M., Scheper, T., Segal, E., and Bahnemann, J. (2022). A 3D-printed microfluidic gradient generator with integrated photonic silicon sensors for rapid antimicrobial susceptibility testing. Lab. Chip 22 (24), 4950–4961. doi:10.1039/d2lc00640e
Hinman, S. S., McKeating, K. S., and Cheng, Q. (2017). Plasmonic sensing with 3D printed optics. Anal. Chem. 89 (23), 12626–12630. doi:10.1021/acs.analchem.7b03967
Hoffman, M. S. F., McKeage, J. W., Xu, J., Ruddy, B. P., Nielsen, P. M. F., and Taberner, A. J. (2023). Minimally invasive capillary blood sampling methods. Expert Rev. Med. Devices 20 (1), 5–16. doi:10.1080/17434440.2023.2170783
Homan, K. A., Gupta, N., Kroll, K. T., Kolesky, D. B., Skylar-Scott, M., Miyoshi, T., et al. (2019). Flow-enhanced vascularization and maturation of kidney organoids in vitro. Nat. Methods 16 (3), 255–262. doi:10.1038/s41592-019-0325-y
Hong, Z., Luo, T., Jiang, S., and Liang, R. (2023b). Fiber-fed 3D printing of germanate glass optics. Photonics 10 (4), 378. doi:10.3390/photonics10040378
Hong, Z., Sun, Y., Ye, P., Loy, D. A., and Liang, R. (2023a). Bio-inspired compact, high-resolution snapshot hyperspectral imaging system with 3D printed glass lightguide array. Adv. Opt. Mater. 11 (9), 2300156. doi:10.1002/adom.202300156
Hong, Z., Ye, P., Loy, D. A., and Liang, R. (2021). Three-dimensional printing of glass micro-optics. Optica 8 (6), 904. doi:10.1364/optica.422955
Hong, Z., Ye, P., Loy, D. A., and Liang, R. (2022). High-precision printing of complex glass imaging optics with precondensed liquid silica resin. Adv. Sci. (Weinh) 9 (18), e2105595. doi:10.1002/advs.202105595
Irlam, R. C., Hughes, C., Parkin, M. C., Beardah, M. S., O'Donnell, M., Brabazon, D., et al. (2020). Trace multi-class organic explosives analysis in complex matrices enabled using LEGO®-inspired clickable 3D-printed solid phase extraction block arrays. J. Chromatogr. A 1629, 461506. doi:10.1016/j.chroma.2020.461506
Ji, Y., Cai, G., Liang, C., Gao, Z., Lin, W., Ming, Z., et al. (2023). A microfluidic immunosensor based on magnetic separation for rapid detection of okadaic acid in marine shellfish. Anal. Chim. Acta 1239, 340737. doi:10.1016/j.aca.2022.340737
Jia, F., Gao, Y., and Wang, H. (2022). Recent advances in drug delivery system fabricated by microfluidics for disease therapy. Bioeng. (Basel) 9 (11), 625. doi:10.3390/bioengineering9110625
Jiang, F., and Xiang, N. (2022). Integrated microfluidic handheld cell sorter for high-throughput label-free malignant tumor cell sorting. Anal. Chem. 94 (3), 1859–1866. doi:10.1021/acs.analchem.1c04819
Jiang, Y., Islam, M. N., He, R., Huang, X., Cao, P., Advincula, R. C., et al. (2022). Recent advances in 3D printed sensors: materials, design, and manufacturing. Adv. Mater. Technol. 8 (2). doi:10.1002/admt.202200492
Jonušauskas, L., Juodkazis, S., and Malinauskas, M. (2018). Optical 3D printing: bridging the gaps in the mesoscale. J. Opt. 20 (5), 053001. doi:10.1088/2040-8986/aab3fe
Junk, S., and Kuen, C. (2016). Review of open source and freeware CAD systems for use with 3D-printing. Procedia CIRP 50, 430–435. doi:10.1016/j.procir.2016.04.174
Kalinke, C., Neumsteir, N. V., Aparecido, G. d. O., Ferraz, T. V. d. B., Dos Santos, P. L., Janegitz, B. C., et al. (2020). Comparison of activation processes for 3D printed PLA-graphene electrodes: electrochemical properties and application for sensing of dopamine. Analyst 145 (4), 1207–1218. doi:10.1039/c9an01926j
Keshan Balavandy, S., Li, F., Macdonald, N. P., Maya, F., Townsend, A. T., Frederick, K., et al. (2021). Scalable 3D printing method for the manufacture of single-material fluidic devices with integrated filter for point of collection colourimetric analysis. Anal. Chim. Acta 1151, 238101. doi:10.1016/j.aca.2020.11.033
Khalfa, A. L., Becker, M. L., and Dove, A. P. (2021). Stereochemistry-controlled mechanical properties and degradation in 3D-printable photosets. J. Am. Chem. Soc. 143 (42), 17510–17516. doi:10.1021/jacs.1c06960
Khan, I., Prabhakar, A., Delepine, C., Tsang, H., Pham, V., and Sur, M. (2021). A low-cost 3D printed microfluidic bioreactor and imaging chamber for live-organoid imaging. Biomicrofluidics 15 (2), 024105. doi:10.1063/5.0041027
Khosravani, M. R., and Reinicke, T. (2020). Effects of raster layup and printing speed on strength of 3D-printed structural components. Procedia Struct. Integr. 28, 720–725. doi:10.1016/j.prostr.2020.10.083
Kotkar, T., Masure, P., Modake, P., Lad, C., and Patil, B. (2018). Modelling and testing of spur gear made of different 3D printed materials. Int. JS Res. Sci. Eng. 4, 1389–1394.
Kotz, F., Arnold, K., Bauer, W., Schild, D., Keller, N., Sachsenheimer, K., et al. (2017). Three-dimensional printing of transparent fused silica glass. Nature 544 (7650), 337–339. doi:10.1038/nature22061
Kruth, J.-P., Wang, X., Laoui, T., and Froyen, L. (2003). Lasers and materials in selective laser sintering. Assem. Autom. 23 (4), 357–371. doi:10.1108/01445150310698652
Kuhnke, L. M., Rehfeld, J. S., Ude, C., and Beutel, S. (2022). Study on the development and integration of 3D-printed optics in small-scale productions of single-use cultivation vessels. Eng. Life Sci. 22 (6), 440–452. doi:10.1002/elsc.202100131
Kulkarni, M. B., Velmurugan, K., Nirmal, J., and Goel, S. (2023). Development of dexamethasone loaded nanomicelles using a 3D printed microfluidic device for ocular drug delivery applications. Sensors Actuators A Phys., 357. doi:10.1016/j.sna.2023.114385
Kumar, P. S., Madapusi, S., and Goel, S. (2023). 3D printed microfluidic chemiluminescence PoC device with self-powering and integrated incubating system: Validation via ALP detection on disposable µPADs. Microchem. J. 189, 108518. doi:10.1016/j.microc.2023.108518
Kunwar, P., Jannini, A. V. S., Xiong, Z., Ransbottom, M. J., Perkins, J. S., Henderson, J. H., et al. (2020). High-resolution 3D printing of stretchable hydrogel structures using optical projection lithography. ACS Appl. Mater Interfaces 12 (1), 1640–1649. doi:10.1021/acsami.9b19431
Lai, W. Q., Chang, Y. F., Chou, F. N., and Yang, D. M. (2022). Portable FRET-based biosensor device for on-site lead detection. Biosens. (Basel) 12 (3), 157. doi:10.3390/bios12030157
Lambert, A., Valiulis, S., and Cheng, Q. (2018). Advances in optical sensing and bioanalysis enabled by 3D printing. ACS Sens. 3 (12), 2475–2491. doi:10.1021/acssensors.8b01085
Layani, M., Wang, X., and Magdassi, S. (2018). Novel materials for 3D printing by photopolymerization. Adv. Mater. 30 (41), 1706344. doi:10.1002/adma.201706344
Lee, J.-Y., An, J., and Chua, C. K. (2017). Fundamentals and applications of 3D printing for novel materials. Appl. Mater. today 7, 120–133. doi:10.1016/j.apmt.2017.02.004
Lee, K., Yoon, T., Yang, H., Cha, S., Cheon, Y., Kashefi-Kheyrabadi, L., et al. (2020). All-in-one platform for salivary cotinine detection integrated with a microfluidic channel and an electrochemical biosensor. Lab. Chip 20 (2), 320–331. doi:10.1039/C9LC01024F
Lertvachirapaiboon, C., Baba, A., Shinbo, K., and Kato, K. (2021). Dual-mode surface plasmon resonance sensor chip using a grating 3D-printed prism. Anal. Chim. Acta 1147, 23–29. doi:10.1016/j.aca.2020.12.027
Li, H., Lousteau, J., Suo, R., Jiang, X., MacPherson, W. N., Bookey, H. T., et al. (2009). “Sensing properties of germanate and tellurite glass optical fibres,” in 20th international conference on optical fibre sensors. doi:10.1117/12.835317
Li, F., Macdonald, N. P., Guijt, R. M., and Breadmore, M. C. (2019). Increasing the functionalities of 3D printed microchemical devices by single material, multimaterial, and print-pause-print 3D printing. Lab a Chip 19 (1), 35–49. doi:10.1039/c8lc00826d
Li, J., Nallappan, K., Guerboukha, H., and Skorobogatiy, M. (2017). 3D printed hollow core terahertz Bragg waveguides with defect layers for surface sensing applications. Opt. Express 25 (4), 4126–4144. doi:10.1364/OE.25.004126
Li, X., He, Z., Li, C., and Li, P. (2021). One-step enzyme kinetics measurement in 3D printed microfluidics devices based on a high-performance single vibrating sharp-tip mixer. Anal. Chim. Acta 1172, 338677. doi:10.1016/j.aca.2021.338677
Liao, C., Chen, X., and Fu, Y. (2023). Salivary analysis: an emerging paradigm for non-invasive healthcare diagnosis and monitoring. Interdiscip. Med. 1. doi:10.1002/inmd.20230009
Ligon, S. C., Liska, R., Stampfl, J., Gurr, M., and Mülhaupt, R. (2017). Polymers for 3D printing and customized additive manufacturing. Chem. Rev. 117 (15), 10212–10290. doi:10.1021/acs.chemrev.7b00074
Lin, T., Xu, Y., Zhao, A., He, W., and Xiao, F. (2022). Flexible electrochemical sensors integrated with nanomaterials for in situ determination of small molecules in biological samples: a review. Anal. Chim. Acta 1207, 339461. doi:10.1016/j.aca.2022.339461
Lin, Z., Zou, Z., Pu, Z., Wu, M., and Zhang, Y. (2023). Application of microfluidic technologies on COVID-19 diagnosis and drug discovery. Acta Pharm. Sin. B 13, 2877–2896. doi:10.1016/j.apsb.2023.02.014
Liu, S., Wang, W., Xu, W., Liu, L., Zhang, W., Song, K., et al. Continuous three-dimensional printing of architected piezoelectric sensors in minutes, 2022, 9790307, doi:10.34133/2022/9790307Research, 2022.
Liu, Y., Gao, R., Zhuo, Y., Wang, Y., Jia, H., Chen, X., et al. (2023). Rapid simultaneous SERS detection of dual myocardial biomarkers on single-track finger-pump microfluidic chip. Anal. Chim. Acta 1239, 340673. doi:10.1016/j.aca.2022.340673
Lu, A., Jin, T., Liu, Q., Guo, Z., Qu, M., Luo, H., et al. (2019). Modeling and prediction of surface topography and surface roughness in dual-axis wheel polishing of optical glass. Int. J. Mach. Tools Manuf. 137, 13–29. doi:10.1016/j.ijmachtools.2018.10.001
Luo, J., Pan, H., and Kinzel, E. C. (2014). Additive manufacturing of glass. J. Manuf. Sci. Eng. 136 (6). doi:10.1115/1.4028531
Maines, E. M., Porwal, M. K., Ellison, C. J., and Reineke, T. M. (2021). Sustainable advances in SLA/DLP 3D printing materials and processes. Green Chem. 23 (18), 6863–6897. doi:10.1039/d1gc01489g
Mao, M., He, J., Li, X., Zhang, B., Lei, Q., Liu, Y., et al. (2017). The emerging frontiers and applications of high-resolution 3D printing. Micromachines 8 (4), 113. doi:10.3390/mi8040113
Matheuse, F., Vanmol, K., Van Erps, J., De Malsche, W., Ottevaere, H., and Desmet, G. (2022). On the potential use of two-photon polymerization to 3D print chromatographic packed bed supports. J. Chromatogr. A 1663, 462763. doi:10.1016/j.chroma.2021.462763
Milton, L. A., Viglione, M. S., Ong, L. J. Y., Nordin, G. P., and Toh, Y. C. (2023). Vat Photopolymerization 3D printed microfluidic devices for organ-on-a-chip applications. Lab a Chip 23, 3537–3560. doi:10.1039/d3lc00094j
Mohamed, O. A., Masood, S. H., and Bhowmik, J. L. (2015). Optimization of fused deposition modeling process parameters: a review of current research and future prospects. Adv. Manuf. 3, 42–53. doi:10.1007/s40436-014-0097-7
Moore, D. G., Barbera, L., Masania, K., and Studart, A. R. (2020). Three-dimensional printing of multicomponent glasses using phase-separating resins. Nat. Mater 19 (2), 212–217. doi:10.1038/s41563-019-0525-y
Moser, M. R., Smith, C. M., Gutierrez, G. G., and Baker, C. A. (2022). 3D printed instrument for taylor dispersion analysis with two-point laser-induced fluorescence detection. Anal. Chem. 94 (16), 6089–6096. doi:10.1021/acs.analchem.1c04566
Muhsin, S. A., He, Y., Al-Amidie, M., Sergovia, K., Abdullah, A., Wang, Y., et al. (2023). A microfluidic biosensor architecture for the rapid detection of COVID-19. Anal. Chim. Acta 1275, 341378. doi:10.1016/j.aca.2023.341378
Muñoz, J., and Pumera, M. (2020). 3D-printed biosensors for electrochemical and optical applications. TrAC Trends Anal. Chem. 128, 115933. doi:10.1016/j.trac.2020.115933
Musgrove, H. B., Catterton, M. A., and Pompano, R. R. (2022). Applied tutorial for the design and fabrication of biomicrofluidic devices by resin 3D printing. Anal. Chim. Acta 1209, 339842. doi:10.1016/j.aca.2022.339842
Nair, S., Trisno, J., Ruan, Q., Daqiqeh Rezaei, S., Simpson, R. E., Yang, J. K. W., et al. (2022). 3D printing mesoscale optical components with a low-cost resin printer integrated with a fiber-optic taper. ACS Photonics 9 (6), 2024–2031. doi:10.1021/acsphotonics.2c00125
Naz, S. A., Huynh, V. T., Doeven, E. H., Adams, S., Kouzani, A., and Guijt, R. M. (2023). Closed-loop control systems for pumps used in portable analytical systems. J. Chromatogr. A 1695, 463931. doi:10.1016/j.chroma.2023.463931
Ng, W. L., Lee, J. M., Zhou, M., Chen, Y. W., Lee, K. X. A., Yeong, W. Y., et al. (2020). Vat polymerization-based bioprinting—process, materials, applications and regulatory challenges. Biofabrication 12 (2), 022001. doi:10.1088/1758-5090/ab6034
Ngo, T. D., Kashani, A., Imbalzano, G., Nguyen, K. T., and Hui, D. (2018). Additive manufacturing (3D printing): a review of materials, methods, applications and challenges. Compos. Part B Eng. 143, 172–196. doi:10.1016/j.compositesb.2018.02.012
Nguyen, D. T., Meyers, C., Yee, T. D., Dudukovic, N. A., Destino, J. F., Zhu, C., et al. (2017). 3D-printed transparent glass. Adv. Mater. 29 (26), 1701181. doi:10.1002/adma.201701181
Nguyen, P. Q. M., Wang, M., Ann Maria, N., Li, A. Y., Tan, H. Y., Xiong, G. M., et al. (2022). Modular micro-PCR system for the onsite rapid diagnosis of COVID-19. Microsyst. Nanoeng. 8, 82. doi:10.1038/s41378-022-00400-3
Ni, J., Ling, H., Zhang, S., Wang, Z., Peng, Z., Benyshek, C., et al. (2019). Three-dimensional printing of metals for biomedical applications. Mater. Today Bio 3, 100024. doi:10.1016/j.mtbio.2019.100024
Nielsen, A. V., Beauchamp, M. J., Nordin, G. P., and Woolley, A. T. (2020). 3D printed microfluidics. Annu. Rev. Anal. Chem. (Palo Alto Calif) 13 (1), 45–65. doi:10.1146/annurev-anchem-091619-102649
Ning, S., Chang, H. C., Fan, K. C., Hsiao, P. Y., Feng, C., Shoemaker, D., et al. (2023). A point-of-care biosensor for rapid detection and differentiation of COVID-19 virus (SARS-CoV-2) and influenza virus using subwavelength grating micro-ring resonator. Appl. Phys. Rev. 10 (2), 021410. doi:10.1063/5.0146079
Nittala, P. V. K., Hohreiter, A., Rosas Linhard, E., Dohn, R., Mishra, S., Konda, A., et al. (2023). Integration of silicon chip microstructures for in-line microbial cell lysis in soft microfluidics. Lab. Chip 23 (9), 2327–2340. doi:10.1039/d2lc00896c
Norani, M. N. M., Abdullah, M., Abdollah, M. F. B., Amiruddin, H., Ramli, F. Z., and Tamaldin, N. (2021). Mechanical and tribological properties of FFF 3D-printed polymers: a brief review. J. Tribol. 29, 11–30.
Nseowo Udofia, E., and Zhou, W. (2020). 3D printed optics with a soft and stretchable optical material. Addit. Manuf. 31, 100912. doi:10.1016/j.addma.2019.100912
Odent, J., Wallin, T. J., Pan, W., Kruemplestaedter, K., Shepherd, R. F., and Giannelis, E. P. (2017). Highly elastic, transparent, and conductive 3D-printed ionic composite hydrogels. Adv. Funct. Mater. 27 (33), 1701807. doi:10.1002/adfm.201701807
Ogishi, K., Osaki, T., Morimoto, Y., and Takeuchi, S. (2022). 3D printed microfluidic devices for lipid bilayer recordings. Lab. Chip 22 (5), 890–898. doi:10.1039/d1lc01077h
Oladapo, B. I., Zahedi, S. A., Ismail, S. O., and Omigbodun, F. T. (2021). 3D printing of PEEK and its composite to increase biointerfaces as a biomedical material-A review. Colloids Surfaces B Biointerfaces 203, 111726. doi:10.1016/j.colsurfb.2021.111726
O'Shea, D. C., Suleski, T. J., Kathman, A. D., and Prather, D. W. (2003). Diffractive optics: design, fabrication, and test. Bellingham, WA, USA: SPIE Press, 1–260.
Pakkanen, J., Manfredi, D., Minetola, P., and Iuliano, L. (2017). About the use of recycled or biodegradable filaments for sustainability of 3D printing: state of the art and research opportunities. Sustain. Des. Manuf. Sel. Pap. Sustain. Des. Manuf. 4, 776–785. doi:10.1007/978-3-319-57078-5_73
Philpott, D. N., Chen, K., Atwal, R. S., Li, D., Christie, J., Sargent, E. H., et al. (2022). Ultrathroughput immunomagnetic cell sorting platform. Lab. Chip 22 (24), 4822–4830. doi:10.1039/d2lc00798c
Pose-Boirazian, T., Martinez-Costas, J., and Eibes, G. (2022). 3D printing: an emerging technology for biocatalyst immobilization. Macromol. Biosci. 22 (9), e2200110. doi:10.1002/mabi.202200110
Purtov, J., Rogin, P., Verch, A., Johansen, V. E., and Hensel, R. (2019). Nanopillar diffraction gratings by two-photon lithography. Nanomater. (Basel) 9 (10), 1495. doi:10.3390/nano9101495
Quan, H., Zhang, T., Xu, H., Luo, S., Nie, J., and Zhu, X. (2020). Photo-curing 3D printing technique and its challenges. Bioact. Mater. 5 (1), 110–115. doi:10.1016/j.bioactmat.2019.12.003
Quang, Q. H., Doan, T. T., Quoc, T. D., and Manh, T. N. (2018). Nonlinear optical tweezers for longitudinal control of dielectric particles. Opt. Commun. 421, 94–98. doi:10.1016/j.optcom.2018.03.068
Quesada-Gonzalez, D., and Merkoci, A. (2017). Mobile phone-based biosensing: an emerging “diagnostic and communication” technology. Biosens. Bioelectron. 92, 549–562. doi:10.1016/j.bios.2016.10.062
Ranjan, R., Kumar, D., Kundu, M., and Chandra Moi, S. (2022). A critical review on Classification of materials used in 3D printing process. Mater. today Proc. 61, 43–49. doi:10.1016/j.matpr.2022.03.308
Rett, J. P., Traore, Y. L., and Ho, E. A. (2021). Sustainable materials for fused deposition modeling 3D printing applications. Adv. Eng. Mater. 23 (7), 2001472. doi:10.1002/adem.202001472
Ristok, S., Thiele, S., Toulouse, A., Herkommer, A. M., and Giessen, H. (2020). Stitching-free 3D printing of millimeter-sized highly transparent spherical and aspherical optical components. Opt. Mater. Express 10 (10), 2370. doi:10.1364/ome.401724
Romanczuk-Ruszuk, E., Sztorch, B., Pakuła, D., Gabriel, E., Nowak, K., and Przekop, R. E. (2023). 3D printing ceramics—materials for direct extrusion process. Ceramics 6 (1), 364–385. doi:10.3390/ceramics6010022
Ryan, K. R., Down, M. P., Hurst, N. J., Keefe, E. M., and Banks, C. E. (2022). Additive manufacturing (3D printing) of electrically conductive polymers and polymer nanocomposites and their applications. eScience 2 (4), 365–381. doi:10.1016/j.esci.2022.07.003
Salmean, C., and Dimartino, S. (2019). 3D-printed stationary phases with ordered morphology: state of the art and future development in liquid chromatography. Chromatographia 82 (1), 443–463. doi:10.1007/s10337-018-3671-5
Salmon, I., Grebenyuk, S., Abdel Fattah, A. R., Rustandi, G., Pilkington, T., Verfaillie, C., et al. (2022). Engineering neurovascular organoids with 3D printed microfluidic chips. Lab. Chip 22 (8), 1615–1629. doi:10.1039/d1lc00535a
Sanli, U. T., Messer, T., Weigand, M., Lötgering, L., Schütz, G., Wegener, M., et al. (2022). High-resolution kinoform X-ray optics printed via 405 nm 3D laser lithography. Adv. Mater. Technol. 7 (9). doi:10.1002/admt.202101695
Saorin, G., Caligiuri, I., and Rizzolio, F. (2023). Microfluidic organoids-on-a-chip: the future of human models. Seminars Cell and Dev. Biol. 144, 41–54. doi:10.1016/j.semcdb.2022.10.001
Schaffner, D., Preuschoff, T., Ristok, S., Brozio, L., Schlosser, M., Giessen, H., et al. (2020). Arrays of individually controllable optical tweezers based on 3D-printed microlens arrays. Opt. Express 28 (6), 8640–8645. doi:10.1364/OE.386243
Schmid, M., Sterl, F., Thiele, S., Herkommer, A., and Giessen, H. (2021). 3D printed hybrid refractive/diffractive achromat and apochromat for the visible wavelength range. Opt. Lett. 46 (10), 2485–2488. doi:10.1364/OL.423196
Schure, M. R., Maier, R. S., Kroll, D. M., and Davis, H. T. (2004). Simulation of ordered packed beds in chromatography. J. Chromatogr. A 1031 (1-2), 79–86. doi:10.1016/j.chroma.2003.12.030
Selemani, M. A., and Martin, R. S. (2024). Use of 3D printing to integrate microchip electrophoresis with amperometric detection. Anal. Bioanal. Chem. 416 (21), 4749–4758. doi:10.1007/s00216-024-05260-6
Shafranek, R. T., Millik, S. C., Smith, P. T., Lee, C. U., Boydston, A. J., and Nelson, A. (2019). Stimuli-responsive materials in additive manufacturing. Prog. Polym. Sci. 93, 36–67. doi:10.1016/j.progpolymsci.2019.03.002
Shahrubudin, N., Lee, T. C., and Ramlan, R. (2019). An overview on 3D printing technology: technological, materials, and applications. Procedia Manuf. 35, 1286–1296. doi:10.1016/j.promfg.2019.06.089
Shang, Y., Xing, G., Liu, X., Lin, H., and Lin, J. M. (2022). Fully integrated microfluidic biosensor with finger actuation for the ultrasensitive detection of Escherichia coli O157:H7. Anal. Chem. 94 (48), 16787–16795. doi:10.1021/acs.analchem.2c03686
Shanmugam, V., Pavan, M. V., Babu, K., and Karnan, B. (2021). Fused deposition modeling based polymeric materials and their performance: a review. Polym. Compos. 42 (11), 5656–5677. doi:10.1002/pc.26275
Shao, G., Hai, R., and Sun, C. (2019). 3D printing customized optical lens in minutes. Adv. Opt. Mater. 8 (4). doi:10.1002/adom.201901646
Sharkey, J. P., Foo, D. C. W., Kabla, A., Baumberg, J. J., and Bowman, R. W. (2016). A one-piece 3D printed flexure translation stage for open-source microscopy. Rev. Sci. Instrum. 87 (2), 025104. doi:10.1063/1.4941068
Shrimal, P., Jadeja, G., and Patel, S. (2022). Ultrasonic enhanced emulsification process in 3D printed microfluidic device to encapsulate active pharmaceutical ingredients. Int. J. Pharm. 620, 121754. doi:10.1016/j.ijpharm.2022.121754
Shu, M., Wu, W., Yang, Q., Chen, J., Guo, C., and Zhang, A. (2022). Compact filters based on dual-mode gap waveguide cavities and 3D printing technology. Microw. Opt. Technol. Lett. 65 (3), 727–732. doi:10.1002/mop.33549
Sikula, M., Vaněčková, E., Hromadová, M., and Kolivoška, V. (2023). Spectroelectrochemical sensing of reaction intermediates and products in an affordable fully 3D printed device. Anal. Chim. Acta 1267, 341379. doi:10.1016/j.aca.2023.341379
Singh, S., Ramakrishna, S., and Berto, F. (2020). 3D Printing of polymer composites: a short review. Mater. Des. Process. Commun. 2 (2), e97. doi:10.1002/mdp2.97
Spoerk, M., Holzer, C., and Gonzalez-Gutierrez, J. (2020). Material extrusion-based additive manufacturing of polypropylene: a review on how to improve dimensional inaccuracy and warpage. J. Appl. Polym. Sci. 137 (12), 48545. doi:10.1002/app.48545
Sta. Agueda, J. R. H., Chen, Q., Maalihan, R. D., Ren, J., da Silva, Í. G. M., Dugos, N. P., et al. (2021). 3D printing of biomedically relevant polymer materials and biocompatibility. MRS Commun. 11 (2), 197–212. doi:10.1557/s43579-021-00038-8
Stefano, J. S., Guterres E Silva, L. R., Rocha, R. G., Brazaca, L. C., Richter, E. M., Abarza Muñoz, R. A., et al. (2022). New conductive filament ready-to-use for 3D-printing electrochemical (bio) sensors: towards the detection of SARS-CoV-2. Anal. Chim. Acta 1191, 339372. doi:10.1016/j.aca.2021.339372
Su, C.-K. (2021). Review of 3D-Printed functionalized devices for chemical and biochemical analysis. Anal. Chim. Acta 1158, 338348. doi:10.1016/j.aca.2021.338348
Su, C. K., and Lin, J. Y. (2020). 3D-Printed column with porous monolithic packing for online solid-phase extraction of multiple trace metals in environmental water samples. Anal. Chem. 92 (14), 9640–9648. doi:10.1021/acs.analchem.0c00863
Su, D., Zhao, X., Yan, X., Han, X., Zhu, Z., Wang, C., et al. (2021b). Background-free sensing platform for on-site detection of carbamate pesticide through upconversion nanoparticles-based hydrogel suit. Biosens. Bioelectron. 194, 113598. doi:10.1016/j.bios.2021.113598
Su, R., Park, S. H., Ouyang, X., Ahn, S. I., and McAlpine, M. C. (2022). 3D-printed flexible organic light-emitting diode displays. Sci. Adv. 8 (1), eabl8798. doi:10.1126/sciadv.abl8798
Su, X., Wang, T., and Guo, S. (2021a). Applications of 3D printed bone tissue engineering scaffolds in the stem cell field. Regen. Ther. 16, 63–72. doi:10.1016/j.reth.2021.01.007
Subramaniyan, M., Karuppan, S., Radhakrishnan, K., Rajesh Kumar, R., and Saravana Kumar, K. (2022). Investigation of wear properties of 3D-printed PLA components using sandwich structure–a review. Mater. Today Proc. 66, 1112–1119. doi:10.1016/j.matpr.2022.04.913
Sule, S. S., Petsiuk, A. L., and Pearce, J. M. (2019). Open source completely 3-D printable centrifuge. Instruments 3 (2), 30. doi:10.3390/instruments3020030
Swargiary, K., Jarutatsanangkoon, P., Suwanich, P., Jolivot, R., and Mohammed, W. S. (2020). Single-step 3D-printed integrated optical system and its implementation for a sensing application using digital light processing technology. Appl. Opt. 59 (1), 122–128. doi:10.1364/AO.59.000122
Tai, C. L., Hong, W., Kuo, Y., Chang, C., Niu, M., Ochathevar, M. K. P., et al. (2019). Stretchable luminescent organic-inorganic perovskite nanocrystal-polymer composites for 3D printing and white light-emitting diodes. ACS Appl. Mater Interfaces 11 (33), 30176–30184. doi:10.1021/acsami.9b06248
Tang, J., Ren, J., and Han, K. Y. (2019). Fluorescence imaging with tailored light. Nanophotonics 8 (12), 2111–2128. doi:10.1515/nanoph-2019-0227
Thiele, S., Gissibl, T., Giessen, H., and Herkommer, A. M. (2016). Ultra-compact on-chip LED collimation optics by 3D femtosecond direct laser writing. Opt. Lett. 41 (13), 3029–3032. doi:10.1364/OL.41.003029
Toombs, J. T., Luitz, M., Cook, C. C., Jenne, S., Rapp, B. E., Kotz-Helmer, F., et al. (2022). Volumetric additive manufacturing of silica glass with microscale computed axial lithography. Science 376 (6590), 308–312. doi:10.1126/science.abm6459
Tümer, E. H., and Erbil, H. Y. (2021). Extrusion-based 3D printing applications of PLA composites: a review. Coatings 11 (4), 390. doi:10.3390/coatings11040390
Upadhyay, R. K., Mishra, A. K., and Kumar, A. (2020). Mechanical degradation of 3D printed PLA in simulated marine environment. Surfaces Interfaces 21, 100778. doi:10.1016/j.surfin.2020.100778
Vaidya, N., and Solgaard, O. (2018a). 3D printed optics with nanometer scale surface roughness. Microsystems Nanoeng. 4 (1), 18. doi:10.1038/s41378-018-0015-4
Vaidya, N., and Solgaard, O. (2018b). 3D printed optics with nanometer scale surface roughness. Microsyst. Nanoeng. 4, 18. doi:10.1038/s41378-018-0015-4
Vanaei, S., Parizi, M., Salemizadehparizi, F., and Vanaei, H. (2021). An overview on materials and techniques in 3D bioprinting toward biomedical application. Eng. Regen. 2, 1–18. doi:10.1016/j.engreg.2020.12.001
Vasilescu, M. D. (2019). Constructiv and technological consideration on the generation of gear made by the DLP 3D-printed methode. Mat. Plast. 56, 440–443. doi:10.37358/mp.19.2.5203
Veli, M., Mengu, D., Yardimci, N. T., Luo, Y., Li, J., Rivenson, Y., et al. (2021). Terahertz pulse shaping using diffractive surfaces. Nat. Commun. 12 (1), 37. doi:10.1038/s41467-020-20268-z
Vinoth, R., Sangavi, P., Nakagawa, T., Jayaraman, M., and Vinu Mohan, A. M. (2023). All-in-one microfluidic device with an integrated porous filtration membrane for on-site detection of multiple salivary biomarkers. Sensors Actuators B Chem., 379. doi:10.1016/j.snb.2022.133214
Vogt, D. W., and Leonhardt, R. (2016). 3D-Printed broadband dielectric tube terahertz waveguide with anti-reflection structure. J. Infrared, Millim. Terahertz Waves 37 (11), 1086–1095. doi:10.1007/s10762-016-0296-3
Wang, H., Zhang, W., and Yang, J. K. W. (2020). Toward near-perfect diffractive optical elements via nanoscale 3D printing. ACS Nano 14 (8), 10452–10461. doi:10.1021/acsnano.0c04313
Wang, Z., Ahmed, S., Labib, M., Wang, H., Wu, L., Bavaghar-Zaeimi, F., et al. (2023). Isolation of tumour-reactive lymphocytes from peripheral blood via microfluidic immunomagnetic cell sorting. Nat. Biomed. Eng. 7, 1188–1203. doi:10.1038/s41551-023-01023-3
Wei, H., Han, L., Yin, R., Yang, T., Liu, Y., Mou, C., et al. (2023). Micro-3D printed Concanavalin A hydrogel based photonic devices for high-sensitivity glucose sensing. Sensors Actuators B Chem., 386. doi:10.1016/j.snb.2023.133707
Wei, L., Fang, G., Kuang, Z., Cheng, L., Wu, H., Guo, D., et al. (2022). 3D-printed low-cost fabrication and facile integration of flexible epidermal microfluidics platform. Sensors Actuators B Chem. 353, 131085. doi:10.1016/j.snb.2021.131085
Wei, X., Li, D., Jiang, W., Gu, Z., Wang, X., Zhang, Z., et al. (2015). 3D printable graphene composite. Sci. Rep. 5 (1), 11181–11187. doi:10.1038/srep11181
Wen, X., Zhang, B., Wang, W., Ye, F., Yue, S., Guo, H., et al. (2021). 3D-printed silica with nanoscale resolution. Nat. Mater. 20 (11), 1506–1511. doi:10.1038/s41563-021-01111-2
Winkler, S., Menke, J., Meyer, K. V., Kortmann, C., and Bahnemann, J. (2022). Automation of cell culture assays using a 3D-printed servomotor-controlled microfluidic valve system. Lab. Chip 22 (23), 4656–4665. doi:10.1039/d2lc00629d
Xiao, C., Eriksson, J., Suska, A., Filippini, D., and Mak, W. C. (2022). Print-and-stick unibody microfluidics coupled surface plasmon resonance (SPR) chip for smartphone imaging SPR (Smart-iSRP). Anal. Chim. Acta 1201, 339606. doi:10.1016/j.aca.2022.339606
Xiao, T. P., Cifci, O. S., Bhargava, S., Chen, H., Gissibl, T., Zhou, W., et al. (2016). Diffractive spectral-splitting optical element designed by adjoint-based electromagnetic optimization and fabricated by femtosecond 3D direct laser writing. ACS Photonics 3 (5), 886–894. doi:10.1021/acsphotonics.6b00066
Xing, J.-F., Zheng, M.-L., and Duan, X.-M. (2015). Two-photon polymerization microfabrication of hydrogels: an advanced 3D printing technology for tissue engineering and drug delivery. Chem. Soc. Rev. 44 (15), 5031–5039. doi:10.1039/c5cs00278h
Xu, X., Huang, X., Sun, J., Chen, J., Wu, G., Yao, Y., et al. (2022). 3D-Stacked multistage inertial microfluidic chip for high-throughput enrichment of circulating tumor cells. Cyborg Bionic Syst. 2022, 2022. doi:10.34133/2022/9829287
Yan, J., Wang, C., Fu, Y., Guo, J., and Guo, J. (2022). 3D printed microfluidic Coulter counter for blood cell analysis. Analyst 147 (14), 3225–3233. doi:10.1039/d2an00633b
Yang, P., Wei, G., Liu, A., Huo, F., and Zhang, Z. (2022b). A review of sampling, energy supply and intelligent monitoring for long-term sweat sensors. npj Flex. Electron. 6 (1), 33. doi:10.1038/s41528-022-00165-9
Yang, Z., Li, B., Stuart, D. D., and Cheng, Q. (2022a). Three-dimensional printed microfluidic mixer/extractor for cell lysis and lipidomic profiling by matrix-assisted laser desorption/ionization mass spectrometry. View 4 (1). doi:10.1002/viw.20220041
Yuk, H., Lu, B., Lin, S., Qu, K., Xu, J., Luo, J., et al. (2020). 3D printing of conducting polymers. Nat. Commun. 11 (1), 1604. doi:10.1038/s41467-020-15316-7
Zatopa, A., Walker, S., and Menguc, Y. (2018). Fully soft 3D-printed electroactive fluidic valve for soft hydraulic robots. Soft Robot. 5 (3), 258–271. doi:10.1089/soro.2017.0019
Zhan, Y., Cheng, Q., Song, Y., and Li, M. (2022). Micro-nano structure functionalized perovskite optoelectronics: from structure functionalities to device applications. Adv. Funct. Mater. 32 (24). doi:10.1002/adfm.202200385
Zhang, D., Liu, X., and Qiu, J. (2021a). 3D printing of glass by additive manufacturing techniques: a review. Front. Optoelectron 14 (3), 263–277. doi:10.1007/s12200-020-1009-z
Zhang, H., Chen, Z., Dai, J., Zhang, W., Jiang, Y., and Zhou, A. (2021b). A low-cost mobile platform for whole blood glucose monitoring using colorimetric method. Microchem. J. 162, 105814. doi:10.1016/j.microc.2020.105814
Zhang, H., Yao, Y., Hui, Y., Zhang, L., Zhou, N., and Ju, F. (2021c). A 3D-printed microfluidic gradient concentration chip for rapid antibiotic-susceptibility testing. Bio-Design Manuf. 5 (1), 210–219. doi:10.1007/s42242-021-00173-0
Zhang, Y., Xiang, J., Wang, Y., Qiao, Z., and Wang, W. (2020c). 3D printing fabrication and test of a centrifugal cartridge with an integrated gravity valve for solid phase extractions. Sensors Actuators A Phys., 315. doi:10.1016/j.sna.2020.112353
Zhang, Y., Mao, K., Leigh, S., Shah, A., Chao, Z., and Ma, G. (2020b). A parametric study of 3D printed polymer gears. Int. J. Adv. Manuf. Technol. 107, 4481–4492. doi:10.1007/s00170-020-05270-5
Zhang, Y., Purssell, C., Mao, K., and Leigh, S. (2020a). A physical investigation of wear and thermal characteristics of 3D printed nylon spur gears. Tribol. Int. 141, 105953. doi:10.1016/j.triboint.2019.105953
Zhang, Y., Xiang, J., Wang, Y., Qiao, Z., and Wang, W. (2019). A 3D printed centrifugal microfluidic platform for spilled oil enrichment and detection based on solid phase extraction (SPE). Sensors Actuators B Chem. 296, 126603. doi:10.1016/j.snb.2019.05.080
Zheng, F., Xiao, Y., Liu, H., Fan, Y., and Dao, M. (2021). Patient-specific organoid and organ-on-a-chip: 3D cell-culture meets 3D printing and numerical simulation. Adv. Biol. (Weinh) 5 (6), e2000024. doi:10.1002/adbi.202000024
Zhu, S., Wu, D., Han, Y., Wang, C., Xiang, N., and Ni, Z. (2020). Inertial microfluidic cube for automatic and fast extraction of white blood cells from whole blood. Lab. Chip 20 (2), 244–252. doi:10.1039/c9lc00942f
Keywords: 3D printing, analytical instrumentation, optics, fluidics, scaffolds
Citation: Malinick AS, Ebel CP, Stuart DD, Valiulis SN, Hanson VA and Cheng Q (2024) Novel and rapid analytical platform development enabled by advances in 3D printing. Front. Anal. Sci. 4:1505510. doi: 10.3389/frans.2024.1505510
Received: 03 October 2024; Accepted: 22 November 2024;
Published: 06 December 2024.
Edited by:
Zhanjun Yang, Yangzhou University, ChinaReviewed by:
Ying Liu, Nanjing University, ChinaJosé Alberto Fracassi da Silva, State University of Campinas, Brazil
Chen Zong, China Pharmaceutical University, China
Copyright © 2024 Malinick, Ebel, Stuart, Valiulis, Hanson and Cheng. This is an open-access article distributed under the terms of the Creative Commons Attribution License (CC BY). The use, distribution or reproduction in other forums is permitted, provided the original author(s) and the copyright owner(s) are credited and that the original publication in this journal is cited, in accordance with accepted academic practice. No use, distribution or reproduction is permitted which does not comply with these terms.
*Correspondence: Quan Cheng, cXVhbi5jaGVuZ0B1Y3IuZWR1
†These authors have contributed equally to this work and share first authorship