- 1SupreMEtric LLC, Rensselaer, NY, United States
- 2Department of Chemistry, University at Albany, SUNY, Albany, NY, United States
Luminescence spectroscopy is a versatile analytical technique that measures the emitted light resulted from the radiative deactivation of electronically excited states of molecular an atomic species. The field of forensic science has implemented the use of fluorescence spectroscopy for the analysis of bloodstains. Bloodstains discovered at crime scenes can provide crucial information to an investigation. It allows for the identification of the individual providing that there is a match with a known DNA profile. Additionally, determining the time since deposition (TSD) can assist investigators in establishing when the crime occurred or if a bloodstain present is related to the investigated event. However, most techniques that researchers have utilized thus far focus on the analysis of hemoglobin, both for identification and TSD determinations. Unlike other techniques, fluorescence spectroscopy can investigate the endogenous fluorophores within bloodstains. In this brief review, the ability of fluorescence spectroscopy for the analysis of bloodstains will be discussed. Including the ability to identify, determine the time since deposition, and phenotypic characterization of bloodstains.
1 Introduction
Luminescence spectroscopy is a versatile analytical technique that measures the emitted light resulted from the radiative deactivation of electronically excited states of molecular an atomic species. Fluorescence is the most often accounted type of luminescence associated with transitions between states of the same multiplicity. This technique can be used to analyze gas, liquid and solid samples and characterize endogenous fluorophores present. Fluorescence spectroscopy is a rapid, inexpensive, and non-destructive technique, making it ideal for use in forensic investigations. Forensic science research has implemented the use of fluorescence spectroscopy for the analysis of trace evidence and biological samples. In this review, we will cover the capability of fluorescence spectroscopy in the analysis of bloodstains.
Blood is a complex biological sample, that is one of the most common body fluids collected during investigations of violent crimes (Li, 2008). The analysis of bloodstains can provide police detectives with significant amounts of information relevant to the crime in question. Currently, during a criminal investigation, forensic scientists must first identify a stain as blood. This is done through chemical presumptive and confirmatory assays, such as the Phenolphthalein test (presumptive) or RSID™-Blood (confirmatory) (Li, 2008). At the scene, bloodstains can also assist in crime scene event reconstruction using bloodstain pattern analysis (BPA) (Li, 2008). After being identified as blood, DNA testing is done on the stain to link the sample back to a suspect or victim. DNA is currently the primary use for bloodstain analysis during forensic investigations as it provides the most valuable information.
However, with the use of spectroscopy, the usability of bloodstains for forensic purposes increases beyond its current capability. Emerging techniques for bloodstain identification and analysis has been a significant focus of analytical research, with vibrational spectroscopy spearheading the movement by having the most success. Much research has gone into the capabilities of Raman (Doty and Lednev, 2018; Fikiet et al., 2018; Khandasammy et al., 2018) and Infrared (Lu, 2015) spectroscopy for bloodstain analysis. These techniques have shown to be non-destructive, rapid, and confirmatory methods for bloodstain identification, even able to distinguish between peripheral and menstrual blood. Raman spectroscopy has also proven to provide additional information based on blood analysis, including estimating the time since deposition of a bloodstain (Bremmer et al., 2012; Zadora and Menżyk, 2018; Das et al., 2020; Weber and Lednev, 2020), differentiating human and animal blood (Virkler and Lednev, 2009; McLaughlin et al., 2014), medical diagnostics (Ralbovsky and Lednev, 2021a), and discriminating between phenotypic characteristics including age, race and sex of the donors (Mistek et al., 2016; Khandasammy et al., 2018). However, near-infrared (NIR) Raman spectra are dominated by the vibrations of hemoglobin and thus provide limited information about the other components of blood (Virkler and Lednev, 2010).
Additionally, NIR spectroscopy is an emerging technique for bloodstain analysis, which analyzes a different spectral region, 780–2500 nm (Fikiet et al., 2020; Beć et al., 2021). NIR spectroscopy has shown to be a compelling method for analysis of trace samples due to advanced miniaturization endeavors, cost-effectiveness of the sensors, and has the advantage of specificity for the excitation wavelength selection. Huck and coauthors published an excellent overview on miniaturized NIR spectrometers (Beć et al., 2021), discussing principles of the technique, practical applications, and current limitations. Highlighting that there are several uses of NIR spectroscopy for the analysis of bloodstains (Lemler et al., 2014; Pereira et al., 2017; Morillas et al., 2018) including on various substrates (Edelman et al., 2012; Pereira et al., 2017; Fonseca et al., 2022) and common interferences (Pereira et al., 2017; Morillas et al., 2018; Yang et al., 2018). NIR spectroscopy can detect the presence of oxyhemoglobin and amide groups from albumin protein within blood. In comparison with Raman spectroscopy, NIR spectroscopy has not yet shown the ability to detect trace amounts of samples. Analysis of samples with NIR spectrometers require a relatively large amount of sample (∼100 µl), while research grade Raman microscopes can acquire spectra from a single red blood cell (Ralbovsky and Lednev, 2021b) and making the bloodstain identification based on it (Muro and Lednev, 2017).
Unlike other techniques, fluorescence spectroscopy can investigate the endogenous fluorophores within bloodstains at trace deposition amounts. In this brief review, the ability of fluorescence spectroscopy for the analysis of bloodstains will be discussed. Including the ability to identify, determine the time since deposition, and phenotypic characterization of bloodstains.
2 Theory
2.1 Chemical composition of blood
Blood is a significant component of the human body. Blood is composed of red blood cells (erythrocytes), white blood cells (leucocytes), and platelets (thrombocytes), that are suspended in a matrix called plasma. The other biochemical components of blood such as proteins, nucleic acids, lipid, and carbohydrate exist in the plasma (Gaensslen, 1983; Li, 2008).
Red blood cells constitute approximately 50% of whole blood and are responsible for transporting oxygen from the lungs to the rest of the body. Red blood cells do not contain any DNA due to their lack of nuclei, and they are mostly composed of hemoglobin, a protein that is responsible for the transport of oxygen (Gaensslen, 1983; Li, 2008). White blood cells only account for about 1% of whole blood, but they play a large role in the functionality of the human body. There are three main types of white blood cells: monocytes, lymphocytes, and granulocytes (Gaensslen, 1983; Li, 2008). Within the plasma are dissolved proteins, clotting factors, and other metabolic compounds (Gaensslen, 1983; Li, 2008). The primary components capable of being measured by fluorescence spectroscopy exist within the plasma.
Because plasma is mostly made up of water, as bloodstains dry, the water evaporates leaving behind the red blood cells, white blood cells, and proteins. Proteins present in blood contain amino acid residues, some of which are highly fluorescent. Changes to the fluorescent components can be used to estimate the TSD. Within blood there are three main types of fluorophores that have been studied for understanding the biochemical mechanism within blood: tryptophan, reduced nicotinamide adenine dinucleotide (NADH), and flavins (including flavin adenine dinucleotide (FAD)) (Li et al., 2006).
Tryptophan is an aromatic amino acid which is present in numerous blood proteins such as albumin and γ-globulin (Guo et al., 2012; Mc Shine et al., 2017). NADH is an electron transporter that is fundamental to the energy production process within the mitochondria (Chance et al., 1979). There are many forms of flavins within blood, but FAD is the most common. FAD is responsible for electron transportation within human cells (Chance et al., 1979; Galbán et al., 2016). Using fluorescence spectroscopy, characteristic spectral profile can be created during the analysis of bloodstains.
2.2 Fluorescence spectroscopy
Fluorescence spectroscopy was discovered in 1852 by Sir George Stokes, where he studied both organic and inorganic compounds and noted the phenomenon of “dispersive reflection.” This is when the wavelength of the dispersed light is longer than the wavelength of the original light (Valeur and Berberan-Santos, 2011). This phenomenon was labeled as fluorescence. Fluorescence spectroscopy is a type of electromagnetic spectroscopy that measures the emitted light from a sample. Fluorescence is produced after a molecule is excited due to the absorption of radiation. Then as the molecules relax back to the ground state, photons emitted from the lowest excited energy level produce light in the form of fluorescence (Valeur and Berberan-Santos, 2011). Research fluorescence spectrometers allow for the measurements of both emission and excitation spectra. Emission spectra are collected by fixing the wavelength of excitation and measuring over a range of emission wavelengths, while excitation spectra is the opposite. Fluorescence is an ideal method for the analysis of bloodstains because it is sensitive, rapid, and requires limited sample preparation.
There are two main types of fluorescence spectroscopy: steady state fluorescence and that based on lifetime fluorescence measurements. Steady state fluorescence spectroscopy measures the intensity of the fluorescence emitted by the fluorophore after excitation. The intensity of the fluorescence correlates to the concentration of the fluorophore. Therefore, if a sample is measured at a consistent interval, changes to the concentration of the fluorescent molecules can be plotted as a function of time. In contrast, fluorescence lifetime measures the time that a fluorophore spends in the excited state prior to returning to the ground state. Tryptophan is the most common fluorophore that is assessed utilizing lifetime measurements. By tracking the decrease in the fluorescence lifetime of tryptophan, the time since deposition (TSD) can be estimated (Guo et al., 2012; Berezin et al., 2013; Guo et al., 2013; Mc Shine et al., 2017).
The characteristic peaks produced by the fluorophores within bloodstains (tryptophan, NADH, and flavins), enables differentiating blood from other biological samples. By plotting the changes, to either the fluorescence concentration or lifetime, then the TSD of bloodstains can be determined. Finally, the combination of fluorescence spectroscopy with chemometrics, advanced statistical analyses, allows for further analysis of bloodstains, such as phenotypic discrimination between samples.
3 Fluorescence spectroscopy for blood stain analysis
3.1 Identification
After the discovery of fluorescence spectroscopy in the early 19th Century (Valeur and Berberan-Santos, 2011), it was applied to the analysis of bloodstains. In 1985, Wolfbeis and Leiner utilized fluorescence spectroscopy to characterize human blood serum (Wolfbeis and Leiner, 1985). The human sera were collected from human donors, by isolation from the red and white blood cells, and then diluted in a phosphate buffer. Using a range of excitation and emission wavelengths, they were able to create a full topographic profile of human serum. Additionally, they were able to tentatively identify the main fluorophores of serum: tryptophan (287 nm/340 nm), NAD(P)H (345 nm/460 nm), pyridoxic acid lactone (365 nm/425 nm), pyridoxal phosphate Schiff base (410 nm/507 nm), and protein-bound bilirubin (460 nm/515 nm) (Wolfbeis and Leiner, 1985). For each fluorophore, the wavelengths listed are the experimental excitation maximum and emission maximum respectively.
The topographic approach to characterizing the fluorescence profile of serum proved to be a useful technique for the analysis of blood by the identification of the fluorophores. Wolfbeis and Leiner also noted additional uses for this method. As fluorescence spectroscopy is a highly sensitive technique, the location and relative intensity of the peaks are dependent on a donor’s health status. Therefore, they hypothesized that by measuring the total fluorescence spectra of blood serum scientists could distinguish between healthy and non-healthy donors (Wolfbeis and Leiner, 1985). However, as blood serum is a complex matrix, the evaluation of the fluorescence spectra was difficult to discriminate between by the human eye. So, the use of cluster analysis, later classified as a form of chemometrics, was advised.
The analysis of blood is a complex ordeal, due to the large number of components and their variable fluorescent intensities. Unless each component of blood is isolated and analyzed, it is improbable that a ‘complete’ fluorescent profile can be obtained. This is because fluorophores with lower fluorescent intensities can be masked by the stronger components (Wolfbeis and Leiner, 1985). This occurs both when analyzing human serum or whole blood. Therefore, to not be overwhelmed when analyzing blood, was necessary to establish the primary fluorophores to use for further analysis. This was done by Li et al. They measured the excitation and emission spectra of whole human blood and determined that the endogenous fluorophores were tryptophan, NAD(P)H, and FAD (Li et al., 2006). Their maximum wavelengths for the emission and excitation spectra of these components were consistent with those experimentally measured by Wolfbeis and Leiner (Wolfbeis and Leiner, 1985). Using the spectral characteristics of these fluorophores, researchers have been able to perform more in-depth analysis of blood. Primarily, utilizing fluorescence spectroscopy to determine the time since deposition of bloodstains.
3.2 Time since deposition
3.2.1 Steady state fluorescence
As discussed above, steady state fluorescence spectroscopy is a versatile analytical chemistry technique capable of characterizing the components of whole blood. However, during forensic investigations there are a several crucial questions that need to be answered to assist investigators. A primary question being how long a bloodstain has been at a scene. This has been termed the time since deposition (TSD) of a stain. By knowing the age of the bloodstain, investigators can provide a period in which the crime occurred. Additionally, TSD can aid in crime scene reconstruction, corroborate witness statements, and assist investigators to determine which stains are relevant to the crime. Collecting only stains pertinent to the crime under investigation creates more efficient processing of biological evidence and can assist in reducing and eliminating the large backlogs in crime labs.
Using steady state fluorescence spectroscopy, Weber et al. created a fluorescent profile for human peripheral blood up to 24-h post deposition (Weber et al., 2021). Samples were collected fresh for 6 trials to ensure that no contamination or additional chemicals were present and to have control over the time of bleeding. In tandem, Wójtowicz et al. conducted an experiment to measure the fluorescence profiles of menstrual blood up to 9 h post deposition (Wojtowicz et al., 2021). Menstrual blood was collected fresh from volunteers, for 5 trials, to ensure that no artificial anti-coagulants were added to the samples. The bloodstains were not prepared in a solution but analyzed in solid form. Analysis of non-processed samples is ideal for forensic investigations, as this preserves the evidence for further downstream testing such as DNA analysis.
The samples were deposited onto an aluminum foil covered microscope slide and aged under ambient conditions. Data collection occurred at specified hour increments post deposition, and both emission and excitation spectra were obtained. Emission spectra were collected after the excitation at 285, 350, and 465 nm and detection in the range of 295–525, 370–675, and 485–700 nm, respectively. Corresponding excitation spectra were collected for the emission at 340, 460, and 600 nm after excitation in the range of 200–320, 250–445, and 325–580 nm, respectively (Wojtowicz et al., 2021). These excitation and emission wavelength pairs each corresponded to a different endogenous fluorophore: tryptophan (285/340 nm), NADH (350/460 nm), and flavins (465/600 nm). The fluorescent profiles of peripheral and menstrual blood obtained using steady state fluorescence spectroscopy are shown in Figure 1 (Weber et al., 2021; Wojtowicz et al., 2021).
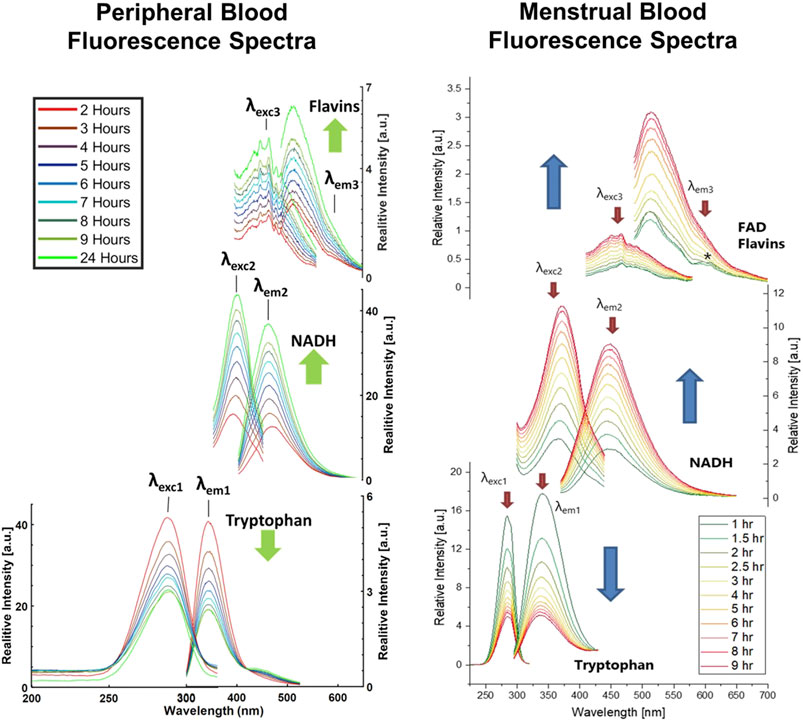
FIGURE 1. Left) Changes in the peripheral bloodstain fluorescence with time since deposition. Excitation and emission spectra collected over first 24 hours after deposition of one peripheral blood sample obtained from Donor 1. Black lines indicate the values of excitation wavelengths applied to measure emission spectra, respectively λexc1 = 285 nm, λexc2 = 350 nm, λexc3 = 465 nm, and emission wavelengths fixed for the collection of excitation spectra, respectively λem1 = 340 nm, λem2 = 460 nm, λem3 = 600 nm. Green arrows indicate the direction of intensity changes. Reprinted from Weber, Alexis, Anna Wójtowicz, and Igor K. Lednev. “Post deposition aging of bloodstains probed by steady-state fluorescence spectroscopy.” Journal of Photochemistry and Photobiology B Biology 221 (2021): 112,251. Copyright 2021, with presmission from Elsevier. Right) Excitation and emission spectra collected over first 9 hours after deposition of the menstrual blood sample obtained from Donor 1. Red arrows indicate the values of excitation wavelengths applied to measure emission spectra, respectively λexc1 = 285 nm, λexc2 = 350 nm, λexc3 = 465 nm, and emission wavelengths fixed for the collection of excitation spectra, respectively λem1 = 340 nm, λem2 = 460 nm, λem3 = 600 nm. Blue arrows indicate the direction of intensity changes. Reprinted from Wójtowicz, A. et al., Probing menstrual bloodstain aging with fluorescence spectroscopy. Spectrochim Acta A Mol Biomol Spectrosc, 2021. 248: p. 119,172. Copyright 2020, with presmission from Elsevier.
It was determined that the fluorescence spectra of each fluorophore in blood showed significant monotonic changes over the first 24-h post deposition. These intensity changes were consistent in both peripheral and menstrual blood and included a decrease of the tryptophan peaks and an increase of the NADH peaks over time (Weber et al., 2021; Wojtowicz et al., 2021). The flavin peaks presented a more complicated trend, a two-stage process that first involved a decrease in the intensity during the first 3 hours, followed by a large increase in the remainder of aging trial. To determine how the fluorescence intensities were changing during aging, the intensity values of the wavelength maxima were plotted as a function of time. This confirmed that there was an exponential decrease concentration of tryptophan during the aging process, while there is an increase in the concentrations of NADH and flavins.
To understand the cause of intensity/concentration changes to the fluorophores, Weber and Wójtowicz explored the ex vivo aerobic aging mechanisms of bloodstains, presented in Figures 2, 3. When aged in an oxygen rich environment, tryptophan will oxidize, however the oxidized form of tryptophan (kynurenine) is not fluorescence (Weber et al., 2021; Wojtowicz et al., 2021). Therefore, there was a decrease in the concentration of tryptophan. NADH and the flavin FAD are part of the cellular respiration process. Once blood exits the body, cellular respiration ceases to occur, leading to the breakdown of the electron transport chain. In consequence, there is a buildup of NADH which causes the increase in the fluorescence intensity overtime (Weber et al., 2021; Wojtowicz et al., 2021). From the electron transport chain, FAD is produced. Once that chain breaks down, there is a halt of FAD production, which was tentatively attributed to initial the decrease in intensity. However, in blood there are many types of flavins including: riboflavin, flavin mononucleotide (FMN), and FAD. All forms of flavins have a similar fluorescence profile as they contain the same main group—pteridine, which is a conjugated compound formed by the tricyclic heterocycle isoalloxazine (Weber et al., 2021; Wojtowicz et al., 2021). Thus, it was hypothesized that the increase in the flavin fluorescence spectra was caused by the oxidation of the many flavins within blood. As fluorescence spectroscopy is not a very selective technique, it was impossible to isolate which flavins are contributing to the spectra. Overall, these studies showed that steady state fluorescence spectroscopy is highly capable of monitoring the changes to both peripheral and menstrual bloodstains over time. As further development of this technique continues, it shows promise to be used in forensic investigations as a rapid, non-destructive, and inexpensive methodology to determine the TSD of bloodstains.
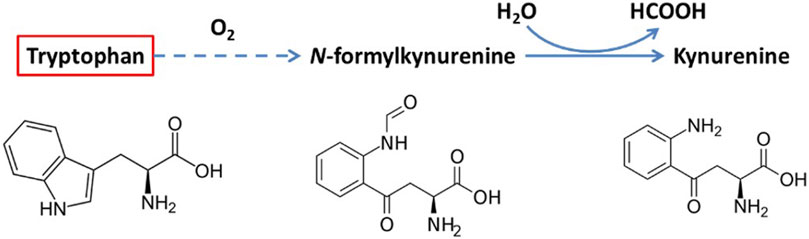
FIGURE 2. Oxidation of tryptophan ex vivo. A fluorescent compound is marked with red. Reprinted from Wójtowicz, A. et al., Probing menstrual bloodstain aging with fluorescence spectroscopy. Spectrochim Acta A Mol Biomol Spectrosc, 2021. 248: p. 119,172. Copyright 2020, with presmission from Elsevier.
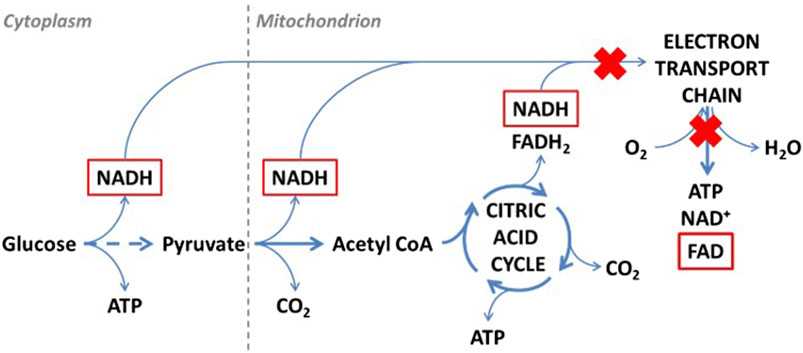
FIGURE 3. Schematic representation of cellular respiration ex vivo. Fluorescent compounds are marked with red. Red crosses indicate processes that are stopped first under aerobic conditions. Reprinted from Wójtowicz, A. et al., Probing menstrual bloodstain aging with fluorescence spectroscopy. Spectrochim Acta A Mol Biomol Spectrosc, 2021. 248: p. 119,172. Copyright 2020, with presmission from Elsevier.
3.2.2 Fluorescence lifetime measurements
Fluorescence lifetime spectroscopy is used to measure the lifetime of the excited states. Within blood, tryptophan is a prominent amino acid that is present in the two proteins albumin and γ-globulin. These proteins account for over 95% of the protein mass within whole blood (Guo et al., 2012). Additionally, the fluorescent lifetime of tryptophan is well documented, and the amino acid is sensitive to changes to the protein conformation. Therefore, when conducting fluorescence lifetime measurements, tryptophan was determined to be ideal for measuring changes to blood overtime. The Berezin research laboratory were the first to utilize fluorescent lifetime measurements to determine the TSD of blood.
Specifically, Guo et al. reported on the capabilities of fluorescence lifetime spectroscopy in 2012 (Guo et al., 2012). Blood samples were collected from different species of dogs and deposited into Petri dishes to age under ambient conditions. Samples were prepared by collecting a portion of the dried blood and dissolving it into a phosphate-buffered saline (PBS) solution. For the spectroscopic measurements, a 295 nm sub nanosecond-pulsed LED in conjunction with the time-resolved single-photon counting (TCSPC) technique was used to measure the lifetime of tryptophan (Guo et al., 2012). They were able to measure the changes to the lifetime of tryptophan in a reproducible manner, depicted in Figure 4. Tryptophan in fresh blood has a fluorescent lifetime of approximately 4 ns, which then declined during the aging process, decreasing down to approximately 2.7 ns after 2 weeks post deposition (Guo et al., 2012). After concluding that this technique showed promise for determining the TSD of bloodstains, Berezin proceeded to patent this methodology (Berezin et al., 2013). This method was found to be rapid, reproducible, and non-dependent on concentration, which urged Guo et al. to further test the capabilities of fluorescence lifetime measurements.
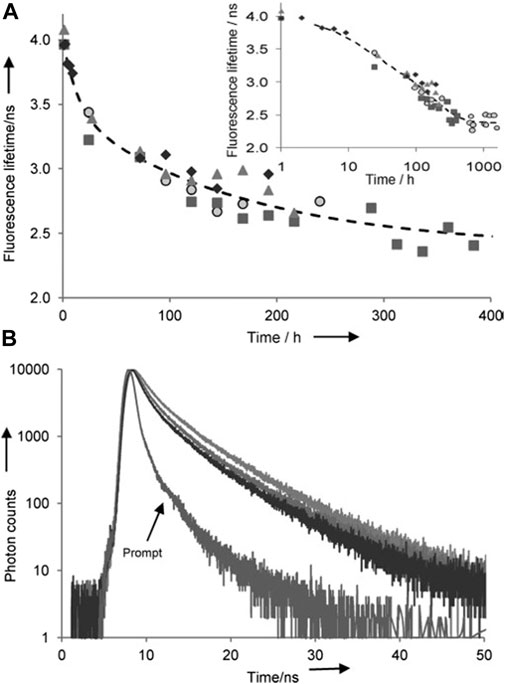
FIGURE 4. (A) Fluorescence lifetime of blood samples in PBS buffer as Fluorescence lifetime of blood samples in PBS buffer as function of aging time (only up to 400 h is shown). Different shapes of the data points represent four different dogs (average from three measurements, error <4%). The fit B was calculated from the two-phase decay modelling. Insert in log scale shows all data up to 1600 h. (B) Selected decays of blood samples from the same bloodstain in PBS buffer. Top line: day 1, middle line: day 4, lowest line: day 7. (ex/em 295/350 nm and prompt ex/em 295/320 nm). Reprinted from Guo, K, Achilefu, S. and Berezin, M.Y. (2012), Dating Bloodstains with Fluorescence Lifetime Measurements. Chem. Eur. J, 18: 1303–1305. Copyright 2012, with permission from John Wiley and Sons.
In 2013, Guo et al. continued their work for the analysis of bloodstains with fluorescence spectroscopy to show that this technique could be utilized for different species (Guo et al., 2013). Isolated human, bovine, rat, mouse, and rabbit albumin were purchased for analysis. Additionally, whole blood and isolated hemoglobin were also acquired for fluorescence lifetime measurements. All solutions were prepared in a PBS solution prior to the fluorescence analysis and the data collection procedure remained the same as their original work (Guo et al., 2012; Guo et al., 2013). In testing samples from several species, they determined all the blood showed comparable optical properties. Which indicated that this method for estimating the TSD of blood can be used not only on human blood, but other species as well. The creation of a single model for TSD would be helpful to forensic scientists and investigators, as it reduces the complexity and time of analysis.
Work done by Guo et al. primarily utilized bloodstains and isolated albumin from non-human species. However, for fluorescence lifetime measurements to be usable for forensic purposes, it was necessary to show that this method will work with human bloodstains. Research by Mc Shine et al. investigated the applicability of fluorescence lifetime measurements to determine the TSD of biological stains, with a focus on blood (Mc Shine et al., 2017). Peripheral blood was collected from 6 human donors, into tubes that were not treated with anti-coagulants. The samples were then deposited onto Petri dished and left to age under ambient conditions. Samples were prepared by placing the blood into tube of PBS to create a blood solution (Mc Shine et al., 2017). In corroboration of previous works, it was determined that the fluorescence lifetime of tryptophan decreases during the aging process. During the first 91 h post deposition the fluoresce lifetime decreased at an exponential rate, after which it plateaued (Mc Shine et al., 2017). This allows for a clear distinction between fresh and old bloodstains, which can be crucial information to know during forensic investigations. Fluorescence lifetime measurements studies show the potential for this technique to determine the TSD of bloodstains for forensic purposes. However, there is more work necessary to fully understand the capabilities of fluorescence spectroscopy so that it can be used for analysis of forensic samples.
3.3 Phenotype research
The capabilities and uses of fluorescence spectroscopy have been greatly expanded upon since its discovery. Beyond the ability to estimate the TSD of blood, fluorescence spectroscopy, when paired with advanced statistical analysis, can discriminate between different species of donors. In 2017, Lu et al. utilized support vector machine discriminate analysis (SVMDA) to differentiate between human and non-human blood (Lu et al., 2017). They collected samples from four species of animals (pigeon, chicken, mouse, and sheep) and using unique features of the spectra, selected by SVMDA, were able to identify human vs. non-human whole blood with 100% accuracy. When analyzing only a solution of isolated red blood cells, they were able to discriminate between species with 94.69%–99.12% accuracy (Lu et al., 2017).
Further species identification work was later conducted by Gan et al., in 2019, where they used a deep belief neural network model (DBN) to classify blood from doves, chickens, mice, and sheep (Gan et al., 2019). DBN is a deep learning method that works on the principle of unique feature extraction of pretreated data to distinguish between classes of samples. They collected whole blood from doves, chickens, mice, and sheep, as blood from these species have numerous similarities, making it difficult to distinguish between them normally. The blood was then diluted in a 0.85% NaCl normal saline solution until the samples possessed a 1% and 3% concentration. Using DBN, Gan et al. were able to properly classify the species samples with 97.5% accuracy (Gan et al., 2019). The ability to discriminate and distinguish between species based on blood is important in many scientific realms including forensic wildlife investigations, import/export inspections, food safety, and medicine.
4 Limitations of current work
Fluorescence spectroscopy is a sensitive technique capable of analyzing minute traces of bloodstains. Steady-state fluorescence spectroscopy has been used to identify the components within blood (Wolfbeis and Leiner, 1985; Li et al., 2006). Of the research done thus far, most of them were proofs-of-concept works. Thus, by increasing the number of samples tested for each form of analysis, the reliability and reproducibility of the methods could be solidified.
By plotting the changes of intensity of the fluorescence peaks over time, a kinetic curve was able to be calculated for the fluorophore’s tryptophan and NADH understand the aging mechanism of peripheral and menstrual blood. While using lifetime fluorescence spectroscopy, the lifetime of tryptophan was able to be measured in the attempt to determine the TSD of peripheral blood. These techniques have shown a strong capacity for analyzing bloodstains. With development, fluorescence spectroscopy will be able to provide probative information about blood traces to investigators, such as the TSD of a stain.
However, there are some limitations to fluorescence spectroscopy relating to TSD analysis, despite the sensitivity of this technique, it is not the most specific. The limited specificity of fluorescence spectroscopy is highlighted in the analysis of flavins, the group of molecules composed of FAD, FMN, and riboflavin. Due to similar base chemical structure, all flavins possess similar fluorescent profiles. Therefore, using this technique, it is not possible to distinguish between the unique flavin molecules. An additional analysis would be required to understand how each flavin is affected by the aging process of blood.
Of the studies conducted thus far, there are some factors that have yet to be investigated. This include testing a longer post deposition period, to create a model capable of determining the TSD of bloodstains. Additionally, increasing the sample size of the donors to incorporate additional races and ages gives researchers the possibility of trying to utilize fluorescence spectroscopy to increase the reliability of any statistical models. Finally, most studies analyzed samples aged under ambient conditions therefore, more research is needed to determine how different external factors affect the analysis of bloodstains.
5 The future of forensic fluorescence
While fluorescence spectroscopy is versatile, the most applicable use of this technique is determining the TSD of bloodstains. The ability to measure consistent changes of the fluorophores within bloodstains overtime make it an ideal technique. Establishing the TSD of a bloodstain will be invaluable for scientists because it will allow them to establish which bloodstains are relevant to event under investigation versus those that are extraneous. This is crucial when there are multiple bloodstains at a scene, which can occur in cases of repeated domestic abuse. Additionally, knowing when a stain is deposited will help with the forensic scene reconstruction and corroborating witness statements. Thus, the future of fluorescent forensic analysis of bloodstains should focus on detailing out the intricacies of the aging mechanism of peripheral and menstrual blood. This technique can be used in combination with other spectroscopic methods that analyze hemoglobin, which is the primary component red blood cells. It is crucial, especially in forensic investigations, to account for and understand how a surrounding environment may affect bloodstain analysis. The primary external factors of interest that should be investigated are the potential impact of substrate the bloodstain is on and the environment during aging, such as temperatures, humidity, and sunlight exposure.
Author contributions
IKL conceived the project, discussed the topic, read and edited the manuscript; AW conducted the literature search, discussed the topic and drafted the manuscript.
Acknowledgments
This project was supported by the STTR Phase I Award No. 2052030 awarded by the National Science Foundation, (AW.). The opinions, findings, and conclusions or recommendations expressed in this publication are those of the authors and do not necessarily reflect those of the National Science Foundation.
Conflict of interest
Authors Alexis Weber and Igor Lednev were employed by SupreMEtric LLC.
Publisher’s note
All claims expressed in this article are solely those of the authors and do not necessarily represent those of their affiliated organizations, or those of the publisher, the editors and the reviewers. Any product that may be evaluated in this article, or claim that may be made by its manufacturer, is not guaranteed or endorsed by the publisher.
References
Beć, K. B., Grabska, J., and Huck, C. W. (2021). Principles and applications of miniaturized near‐infrared (NIR) spectrometers. Chemistry 27 (5), 1514–1532. doi:10.1002/chem.202002838
Berezin, M. Y., Achilefu, S., and Guo, K. (2013). Inventors; Washington University in St Louis WUSTL, assignee. Dating bloodstains and biological fluids with fluorescence lifetime techniques. United States patent application US 13/689, 944.
Bremmer, R. H., de Bruin, K. G., van Gemert, M. J., van Leeuwen, T. G., and Aalders, M. C. (2012). Forensic quest for age determination of bloodstains. Forensic Sci. Int. 216 (1-3), 1–11. doi:10.1016/j.forsciint.2011.07.027
Chance, B., Schoener, B., Oshino, R., Itshak, F., and Nakase, Y. (1979). Oxidation-reduction ratio studies of mitochondria in freeze-trapped samples. NADH and flavoprotein fluorescence signals. J. Biol. Chem. 254 (11), 4764–4771. doi:10.1016/s0021-9258(17)30079-0
Das, T., Harshey, A., Nigam, K., Yadav, V. K., and Srivastava, A. (2020). Analytical approaches for bloodstain aging by vibrational spectroscopy: Current trends and future perspectives. Microchem. J. 158, 105278. doi:10.1016/j.microc.2020.105278
Doty, K. C., and Lednev, I. K. (2018). Raman spectroscopy for forensic purposes: Recent applications for serology and gunshot residue analysis. TrAC Trends Anal. Chem. 103, 215–222. doi:10.1016/j.trac.2017.12.003
Edelman, G., Manti, V., van Ruth, S. M., van Leeuwen, T., and Aalders, M. (2012). Identification and age estimation of blood stains on colored backgrounds by near infrared spectroscopy. Forensic Sci. Int. 220 (1-3), 239–244. doi:10.1016/j.forsciint.2012.03.009
Fikiet, M. A., Khandasammy, S. R., Mistek, E., Ahmed, Y., Halámková, L., Bueno, J., et al. (2018). Surface enhanced Raman spectroscopy: A review of recent applications in forensic science. Spectrochim. Acta. A Mol. Biomol. Spectrosc. 197, 255–260. doi:10.1016/j.saa.2018.02.046
Fikiet, M. A., Tuschel, D., Ermolenkov, V. V., and Lednev, I. K. (2020). Clarifying glass luminescence at near-infrared excitation. Appl. Spectrosc. 74 (2), 187–192. doi:10.1177/0003702819879109
Fonseca, A. C., Pereira, J. F., Honorato, R. S., Bro, R., and Pimentel, M. F. (2022). Hierarchical classification models and Handheld NIR spectrometer to human blood stains identification on different floor tiles. Spectrochim. Acta. A Mol. Biomol. Spectrosc. 267, 120533. doi:10.1016/j.saa.2021.120533
Gaensslen, R. E. (1983). Sourcebook in forensic serology, immunology, and biochemistry. Washington, DC: US Department of Justice, National Institute of Justice.
Galbán, J., Sanz-Vicente, I., Navarro, J., and de Marcos, S. (2016). The intrinsic fluorescence of FAD and its application in analytical chemistry: A review. Methods Appl. Fluoresc. 4 (4), 042005. doi:10.1088/2050-6120/4/4/042005
Gan, J., Zhou, L., Cui, J., Man, B., Jia, X., Shi, S., et al. (2019). Classification of blood species using fluorescence spectroscopy combined with deep learning method. J. Appl. Math. Phys. 7 (10), 2324–2332. doi:10.4236/jamp.2019.710158
Guo, K., Achilefu, S., and Berezin, M. Y. (2012). Dating bloodstains with fluorescence lifetime measurements. Chemistry 18 (5), 1303–1305. doi:10.1002/chem.201102935
Guo, K., Zhegalova, N., Achilefu, S., and Berezin, M. Y. (Editors) (2013). Bloodstain age analysis: Toward solid state fluorescent lifetime measurements (San Francisco, CA: Advanced Biomedical and Clinical Diagnostic Systems XI: SPIE).
Khandasammy, S. R., Fikiet, M. A., Mistek, E., Ahmed, Y., Halámková, L., Bueno, J., et al. (2018). Bloodstains, paintings, and drugs: Raman spectroscopy applications in forensic science. Forensic Chem. 8, 111–133. doi:10.1016/j.forc.2018.02.002
Lemler, P., Premasiri, W. R., DelMonaco, A., and Ziegler, L. D. (2014). NIR Raman spectra of whole human blood: Effects of laser-induced and in vitro hemoglobin denaturation. Anal. Bioanal. Chem. 406 (1), 193–200. doi:10.1007/s00216-013-7427-7
Li, B. H., Zhang, Z. X., Xie, S. S., and Chen, R. (2006). Fluorescence spectral characteristics of human blood and its endogenous fluorophores. Guang Pu Xue Yu Guang Pu Fen Xi 26 (7), 1310–1313.
Lu, P., Fan, Y., Zhou, L., Qian, J., Liu, L., Zhao, S., et al. (2017). Feature extraction and classification of animal blood spectra with support vector machine. Spectrosc. Spectrual analysis 37 (12), 3828–3832.
Lu, Z. (2015). Applications of attenuated total reflectance Fourier transform infrared spectroscopy for forensic analysis. South Carolina: University of South Carolina.
Mc Shine, S., Suhling, K., Beavil, A., Daniel, B., and Frascione, N. (2017). The applicability of fluorescence lifetime to determine the time since the deposition of biological stains. Anal. Methods 9 (13), 2007–2013. doi:10.1039/c6ay03099h
McLaughlin, G., Doty, K. C., and Lednev, I. K. (2014). Raman spectroscopy of blood for species identification. Anal. Chem. 86 (23), 11628–11633. doi:10.1021/ac5026368
Mistek, E., Halámková, L., Doty, K. C., Muro, C. K., and Lednev, I. K. (2016). Race differentiation by Raman spectroscopy of a bloodstain for forensic purposes. Anal. Chem. 88 (15), 7453–7456. doi:10.1021/acs.analchem.6b01173
Morillas, A. V., Gooch, J., and Frascione, N. (2018). Feasibility of a handheld near infrared device for the qualitative analysis of bloodstains. Talanta 184, 1–6. doi:10.1016/j.talanta.2018.02.110
Muro, C. K., and Lednev, I. K. (2017). Identification of individual red blood cells by Raman microspectroscopy for forensic purposes: In search of a limit of detection. Anal. Bioanal. Chem. 409 (1), 287–293. doi:10.1007/s00216-016-0002-2
Pereira, J. F., Silva, C. S., Vieira, M. J. L., Pimentel, M. F., Braz, A., and Honorato, R. S. (2017). Evaluation and identification of blood stains with handheld NIR spectrometer. Microchem. J. 133, 561–566. doi:10.1016/j.microc.2017.04.038
Ralbovsky, N. M., and Lednev, I. K. (2021). Analysis of individual red blood cells for Celiac disease diagnosis. Talanta 221, 121642. doi:10.1016/j.talanta.2020.121642
Ralbovsky, N. M., and Lednev, I. K. (2021). Vibrational spectroscopy for detection of diabetes: A review. Appl. Spectrosc. 75 (8), 929–946. doi:10.1177/00037028211019130
Valeur, B., and Berberan-Santos, M. N. (2011). A brief history of fluorescence and phosphorescence before the emergence of quantum theory. J. Chem. Educ. 88 (6), 731–738. doi:10.1021/ed100182h
Virkler, K., and Lednev, I. K. (2009). Blood species identification for forensic purposes using Raman spectroscopy combined with advanced statistical analysis. Anal. Chem. 81 (18), 7773–7777. doi:10.1021/ac901350a
Virkler, K., and Lednev, I. K. (2010). Raman spectroscopic signature of blood and its potential application to forensic body fluid identification. Anal. Bioanal. Chem. 396 (1), 525–534. doi:10.1007/s00216-009-3207-9
Weber, A., Wójtowicz, A., and Lednev, I. K. (2021). Post deposition aging of bloodstains probed by steady-state fluorescence spectroscopy. J. Photochem. Photobiol. B 221, 112251. doi:10.1016/j.jphotobiol.2021.112251
Weber, A. R., and Lednev, I. K. (2020). Crime clock–analytical studies for approximating time since deposition of bloodstains. Forensic Chem. 19, 100248. doi:10.1016/j.forc.2020.100248
Wojtowicz, A., Weber, A., Wietecha-Posluszny, R., and Lednev, I. K. (2021). Probing menstrual bloodstain aging with fluorescence spectroscopy. Spectrochim. Acta. A Mol. Biomol. Spectrosc. 248, 119172. doi:10.1016/j.saa.2020.119172
Wolfbeis, O. S., and Leiner, M. (1985). Mapping of the total fluorescence of human blood serum as a new method for its characterization. Anal. Chim. Acta 167, 203–215. doi:10.1016/s0003-2670(00)84422-0
Yang, J., Messinger, D. W., and Dube, R. R. (2018). Bloodstain detection and discrimination impacted by spectral shift when using an interference filter-based visible and near-infrared multispectral crime scene imaging system. Opt. Eng. 57 (3), 033101. doi:10.1117/1.oe.57.3.033101
Keywords: fluorescence spectroscopy, bloodstains, forensic science, luminescence, steady-state measurements, lifetime measurements, forensic serology, biological stains
Citation: Weber A and Lednev IK (2022) Brightness of blood: Review of fluorescence spectroscopy analysis of bloodstains. Front. Anal. Sci. 2:906532. doi: 10.3389/frans.2022.906532
Received: 28 March 2022; Accepted: 08 July 2022;
Published: 26 July 2022.
Edited by:
Wendell K. T. Coltro, Universidade Federal de Goiás, BrazilReviewed by:
Justyna Grabska, University of Innsbruck, AustriaMarcel De Puit, Netherlands Forensic Institute, Netherlands
Copyright © 2022 Weber and Lednev. This is an open-access article distributed under the terms of the Creative Commons Attribution License (CC BY). The use, distribution or reproduction in other forums is permitted, provided the original author(s) and the copyright owner(s) are credited and that the original publication in this journal is cited, in accordance with accepted academic practice. No use, distribution or reproduction is permitted which does not comply with these terms.
*Correspondence: Igor K. Lednev, aWxlZG5ldkBhbGJhbnkuZWR1