- 1Univ. Lille, CNRS, Centrale Lille, Univ. Polytechnique Hauts-de-France, UMR 8520—IEMN, Lille, France
- 2Institute of Analytical Chemistry, Chemo- and Biosensors, University Regensburg, Regensburg, Germany
- 3Laboratory for Life Sciences and Technology (LiST), Danube Private University, Krems, Austria
- 4FZU-Institute of Physics, Czech Academy of Sciences, Prague, Czechia
- 5Biosensor Technologies, AIT-Austrian Institute of Technology GmbH, Tulln an der Donau, Austria
- 6Département de chimie, Quebec Center for Advanced Materials, Regroupement québécois sur les matériaux de pointe, and Centre Interdisciplinaire de Recherche sur le Cerveau et l’Apprentissage, Université de Montréal, Montréal, QC, Canada
For nearly 40 years, surface plasmon resonance (SPR) analysis has been used to better understand the binding interaction strength between surface immobilized bioreceptors and the analytes of interest. The advantage of surface plasmon resonance, over other affinity sensing approaches such as Western blots and ELISAs approaches, resides in its possibility to reveal binding kinetics in a label-free manner. The concept of surface plasmon resonance has in addition been widely employed for the development of biosensors capitalizing on its direct assay format, short response times, simple sample treatments along with multiplexed sensing possibilities. To this must be added the possibility to reach high sensitivity due to the capability of surface plasmon resonance to detect very small changes in refractive index at the sensing interfaces in particular for analytes of larger size such as cells (e.g., bacteria), proteins, peptides and oligonucleotides. Challenges inherent to all affinity approaches call for further research and include non-specific surface binding events, mass transportation restrictions, steric hindrance, and the risk of data misinterpretation in case of lack of selective analyte binding. This opinion article is devoted to outlining the different approaches proposed to address these challenges by e.g., coupling with fluorescence read out, electrochemical sensing, mass spectroscopy analysis and more recently to integrate lateral flow concepts into surface plasmon resonance. Other plasmonic methods such as localized surface plasmon resonance (LSPR), surface enhanced Raman spectroscopy (SERS) will not be considered in detail, as such techniques have nowadays their own standing.
1 Introduction
Surface plasmon resonance (SPR) biosensor technologies have undertaken a dramatic growth in the past decasdes not only at the level of number of performed studies but also in terms of the variety of ways they have been applied. This optical technique serves since several decades to monitor affinity reactions through the measurement of changes in the refractive index occurring upon the binding of target molecules on receptor-modified surfaces. SPR is conventionally utilized by using the Kretschmann configuration of attenuated total reflection method, where incident light falls on the inner interface of a thin gold film in order to achieve phase-matching with surface plasmon waves travelling along the outer gold film interface. Upon contacting analyzed liquid sample with this outer interface that is modified with bioreceptors, target analyte accumulates on the sensor surface due to its affinity interaction with attached bioreceptors. Such accumulation is associated with an increase in the refractive index near to the sensor surface, which subsequently leads to changes in the SPR phase-matching condition providing a direct optical readout of binding efficiency and surface reaction kinetics (rates of association and dissociation rates). As this approach requires minimal amounts of sample volume, it is widely used for screening of individual or whole libraries of antibodies, aptamers, engineered bioreceptors and other ligands determining their binding affinity to a specify target. Once optimum binding affinity have been reached and analyte specific binding established, these different bioreceptors can then be integrated on various types of SPR - based biosensors and serve for bioanalytical purposes. There are many aspects that must be considered including the choice of the recognition element, the surface coupling chemistry, assay format reflecting the size of the analyte as well as the composition of analyzed sample. The major drawback of this label-free method stems from that fact that many biologically relevant processes are inherently linked to mass and charge variation. For a better understanding of the surface reactions taking place on a SPR chip, as well as to render SPR more sensitive from an analytical point of view, combining with other sensing approaches into one multifunctional instrument has been persuade in different manners (Figure 1).
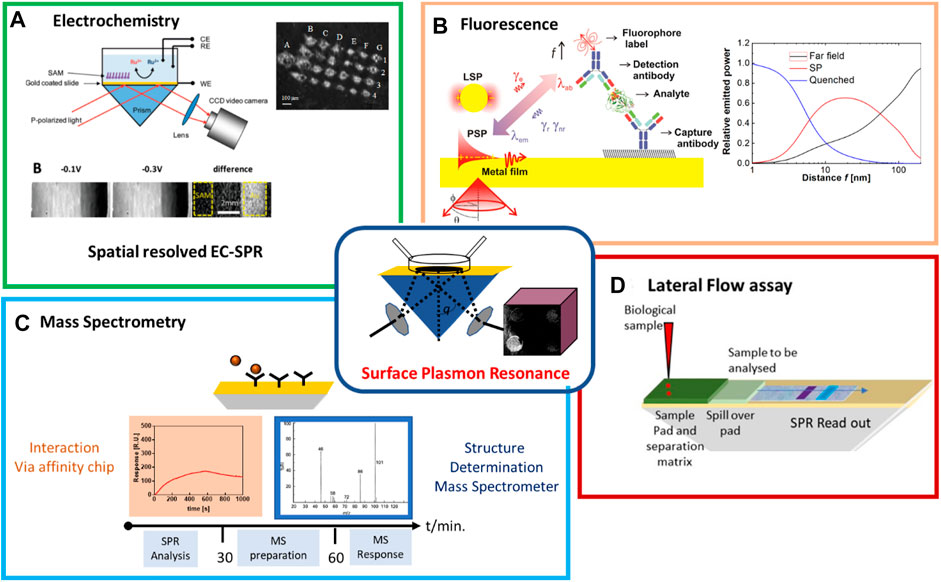
FIGURE 1. Overview of state of the art of multiplexed SPR strategies: (A) Schematic illustration of EC-SPR set up together with SPR images of Ru(NH3)63+ (11.78 mM) in PB (0.5 M, pH = 7) at −0.1 and −0.3 V vs. Ag/AgCl and differences of both images [reprint with permission of (Wang et al., 2010)]. (B) Schematics of the surface plasmon-enhanced fluorescence readout of a sandwich immunoassay with distance-dependent nearfield coupling of the emitter with surface plasmon waves. (C) Flow diagram of SPR coupled to mass spectrometry (MS) analysis. (D) Integration possibility of lateral flow assay (LFA) with SPR read out using LFA membranes as separation membranes and to move the liquid to the SPR chip via capillary forces.
The combination of SPR with electrochemistry (EC-SPR) is dating back to the beginning of the century with the focus at this time on the possibility of individual studies of surface localized electrochemical reactions originated from bound biomolecules and films (Shan et al., 2010; Liu et al., 2017; Wang et al., 2017). EC-SPR has been broadly applied to gain understanding of the electrochemical properties of interface with real-time monitoring of electron transfer processes on modified gold chips (Andersson et al., 2008; Wang et al., 2010) and in polymerization processes (Szunerits et al., 2004; Fortin et al., 2005), characterization of the effect of redox labeled density on SPR response (Patskovsky et al., 2016), or the characterization of biological redox reactions such as the turnover of cytochrome C (Hou et al., 2016). Also, SPR imaging was used to monitor a microarray patterning process based on electropolymerization of pyrrole-labeled oligonucleotides. While the analytical purposes of EC-SPR were restricted to mostly proof-of-concept works, as discussed later, this field still has room for its growth. For instance, measurements can be performed to separate mass and charge effects with temporal resolution via the combination with SPR and a field effect transistor (FET) (Aspermair et al., 2020).
The coupling of SPR with fluorescence allows to benefit from advances in this established central technology widely employed in bioanalysis. Fluorescence is a dominantly used readout method in enzyme-linked immunosorbent assays (ELISA) and polymerase chain reactions (PCR) enhanced due to gradual development of the optical instrumentation and respective fluorophore labeling chemistry. The possible enhancement of fluorophore brightness by near field coupling with surface plasmons supported by metallic thin films and nanostructures (Sokolov et al., 1998; Geddes et al., 2003; Bauch et al., 2014) has been explored by balancing between quenching, surface plasmon field-enhanced excitation rate, surface plasmon-coupled emission, and mediated fluorescence life-time. Besides pushing forward the sensitivity of fluorescence-based assays, the combination with SPR offers access to multimodal biosensing and investigating complex biointerface phenomena that can be tailored for bioanalytical techniques.
The addition of an analytical method capable of molecular identification is achieved by coupling mass spectrometry (MS) with SPR While comparable costly and limited in measuring real-time biological interactions, MS had great utility in solving complex biological structures such as protein and nucleic acid sequences. Approaches proposed include the post-SPR MS analysis by removing the target from the sensor chip prior to MS analysis and the on-ship MS analysis direct on the SPR gold film (Stigter et al., 2013).
Table 1 summarizes tries to give a more detailed overview how each combined technology improves the classic SPR in various biosensing aspects.
2 SPR and electrochemical coupling (EC-SPR)
EC-SPR remains an important (Chieng et al., 2019), still underexplored approach to take the advantages of both techniques for sensing related applications (Riberio et al., 2020). Thus, in EC-SPR, the thin metal film serves to excite surface plasmons and functions as a working electrode. The resonance angle shift in SPR is governed in EC reactions by several processes, including changes in the surface plasmon frequency from the applied potential, redox reaction-induced bulk refractive index changes near the metal film, dielectric changes of the metal film with molecular binding with redox labels (often ferrocene or methylene blue), or EC-induced deposition of a sensing or polymer layer onto the gold SPR film. When combined with voltammetry, SPR measures changes in the physical properties of an electrode surface or molecules adsorbed on the electrode.
The first EC-SPR study dates back to that of Hanken and Corn in 1997, based on detecting local surface potentials (Hankens and Corn, 1997), with the team of Tao being one of the first developing quantitative formalism for EC-SPR (Wang et al., 2010). EC-SPR has several unique advantages including spatial resolution attractive for studying heterogeneous reactions, high surface sensitivity for surface binding processes and detection of reaction species with the optical properties of the reaction species assisting in reaction mechanism formulations. Figure 1A illustrates the prism-based SPR set up and synchronization with electrochemical measurements with typical SPR images at the oxidation and reduction potentials, and the difference of the images between the two states on self-assembled monolayer (SAM)-coated areas and on gold only (Wang et al., 2010). As a first step to sensing, the conductivity change of a polyaniline film modified with horseradish peroxidase (HRP) in the presence of H2O2 and its translation into a change on the SPR signal has been studied (Kang et al., 2001). An EC-SPR/waveguide glucose biosensor that detects 10 mM glucose via an enzymatic reactions in a conducting polymer/glucose oxidase (GOx) multilayer thin film was proposed later where signal enhancement was obtained by monitoring the doping-dedoping events on the polypyrrole. The real-time optical signal could be distinguished between the change in the dielectric constant of the enzyme layer and other non-enzymatic reaction events such as adsorption of glucose and the change of the refractive index of the solution (Baba et al., 2010). While the limit of detection is not competitive, the new principle demonstrated here is of great interest. While performed separately, Gong et al. reported on a protein immunosensor using EC-SPR. The formation of polypyrrole pyrrolic acid was followed in situ by EC-SPR with antibody-antigen interaction measured directly with SPR and via the use of a secondary antibody labeled with alkaline phosphatase by EC using p-aminophenyl phosphate as enzyme substrate. In both cases comparable detection limits of 602.6 and 676.1 ng/ml were achieved.
Patskovsky and coworkers immobilized a stem-looped DNA probe with a methylene blue (MB) tag on a SPR chip to follow hybridization. In presence of the target, the stem-loop unfolds and changes the distance of MB to the gold. Working at the absorption maximum of MP using a wavelength sensitive SPR, the change of oxidation state of MB could be clearly validated. It was also seen that such a sensing system with no diffusion of mediator corresponds to an “absorption” effect. The use of external labels such a MB or ferrocene with high refractive index in addition enhances the sensitivity of SPR, as often shown by the use of colloidal Au nanoparticles, magnetic nanoparticles, etc. DNA hybridization to PNA probes was shown as early as 2005 by Knoll and co-workers using a ferrocene-streptavidin label to biotin-carrying complementary DNA. Quantification of target DNA down to 10 p.m. could be easily obtained from cyclic voltammetry or chronocoulometric but SPR could also follow the hybridization events due to the large size of the ferrocene-streptavidin label (Liu et al., 2005). The group of Meunier et al. proposed 10 years later a highly sensitive and multiplexed EC-SPR method to overcome current limitations of biosensors (Figure 2A). The use of DNA-based structures with redox reporters at the end resulted in a limit of detection (LoD) of 5 nM. Szunerits and coworkers advanced this strategy further and used an imaging SPR to follow the microarray patterning process aided by electropolymerization of pyrrole-labeled oligonucleotides using scanning electrochemical microscopy followed by hybridization studies (Figure 2B) (Fortin et al., 2005).
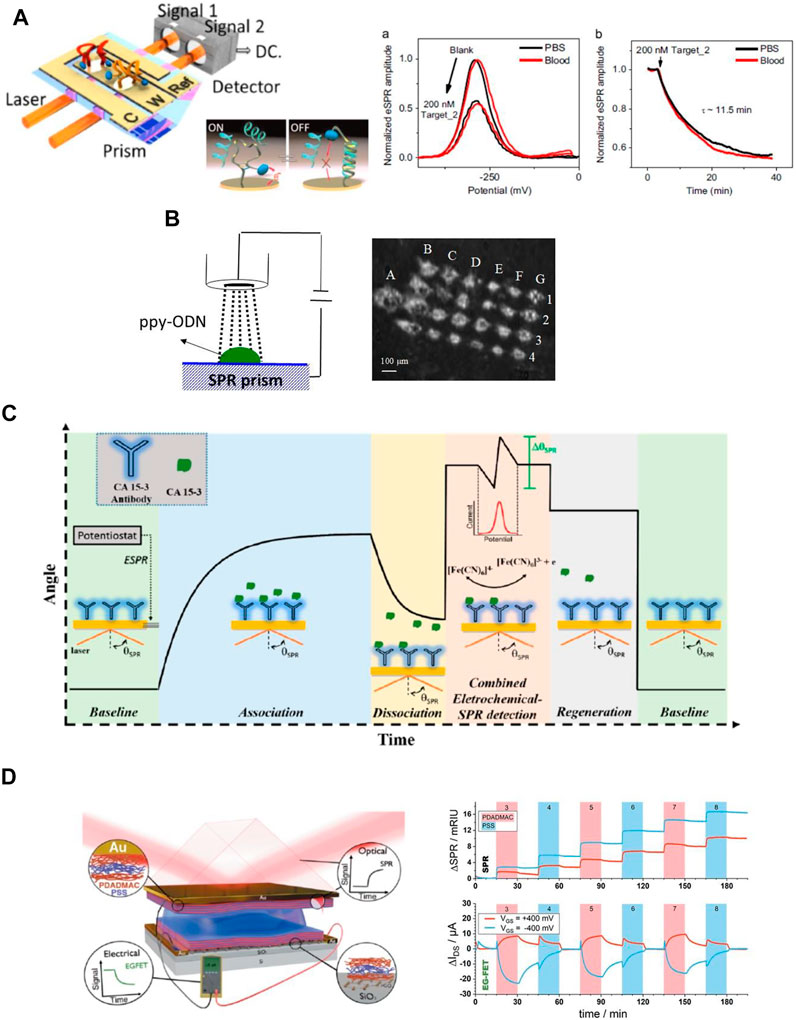
FIGURE 2. Electrochemistry coupled with surface plasmon resonance (EC-SPR): (A) Image of a multiplexed EC-SPR set up. Nano-switch 1 and Nano-switch 2 are immobilized on two separated spots of the same working electrode immersed in the same liquid and imaged with two different lasers. Normalized EC-SPR voltammograms of surface Nano-switch 2 in phosphate buffered saline (PBS) and blood recorded before and after addition of DNA target for 40 min. Real time EC-SPR dynamics of hybridization event of Nano-switch 2 [reprint with permission from (Dallaire et al., 2015)] (B) Use of scanning electrochemical microscopy (SECM) approach for patterning of SPR chip with polypyrrole-DNA strands together with SPR image of modified interface (Fortin et al., 2005). (C) Detection principle of cancer antigen 15-3 (CA-15-3) using EC-SPR [reprint with permission from Ref (Ribereio et al., 2021)]. (D) Illustration of the combined SPR/FET set up and in situ read out of the sequential growth of alternating polydiallyldimethylammonium chloride (PDAMAC) and polystyrene sulfonate (PSS) layers.
Pereira et al. suggested a different strategy for EC-SPR by suggesting a potential-assisted deposition of a redox probe in the SPR surface (Ribereio et al., 2020; Riberio et al., 2020). This concept was then applied to the quantitative analysis of CA 15-3 in biological samples (Figure 2C). First, direct SPR monitoring of interactions between CA 15-3 antigen in solution with surface immobilized antibody was performed until adsorption reached dynamic equilibrium. In a second step, square wave voltammetry measurements were performed in the presence of a redox mediator where a decrease in the redox peak current was observed with increase of CA15-3. The detection limit was 21 U/mL for SPR and 0.0998 U/mL with the SPR-EC approach.
Finally, the dual monitoring of surface reactions in real time by SPR and an electrolyte-gated field-effect transistor were designed (Aspermair et al., 2020) (Figure 2D). This approach allows the simultaneous measurement of surface mass and charge density changes in real time. This platform has the potential to provide new insights into bio adsorption and bio interactions processes but also in the understanding of charge transfer related changes in FET devices upon biomolecule interactions.
3 SPR coupled with fluorescence: Surface plasmon-enhanced and surface plasmon-coupled fluorescence emission
To overcome the intrinsic challenge of SPR biosensors in the analysis of small molecules and very low concentration of target analytes, various means of amplification of affinity binding-induced refractive index changes were reported including those based on enzymatic reactions (Rodríguez-Lorenzo et al., 2012) or using colloidal nanoparticle-enhanced assays (He et al., 2000; Fang et al., 2006) that can be further amplified by nanoparticle aggregation (Calcagno et al., 2022). The first attempt of marrying SPR biosensor and fluorescence readout (Figure 1B) was reported for detection of human chorionic gonadotropin providing a limit of detection (LOD) of 240 p.m. in serum (Attridge et al., 1991). This research direction utilizing the fluorophore labelling was later reintroduced by Lakowicz et al. (2003) as well as the Knoll team (Lieberman and Knoll, 2000; Yu et al., 2004a; Dostalek and Knoll, 2008). The Lakowicz group (Lakowicz et al., 2003) proposed a fluorescence readout of the binding event on the SPR chip by using the highly directional surface plasmon-coupled emission (SPCE) concept. Knoll and co-workers (Lieberman and Knoll, 2000; Yu et al., 2004a; Dostalek and Knoll, 2008) followed a different route by coupling surface plasmons with fluorophore emitters at their excitation wavelength in a method coined as surface plasmon-enhanced fluorescence spectroscopy (SPFS). The use of SPFS enabled to push the LOD for the detection of DNA hybridization to 100 fM level (Dostalek and Knoll, 2008) and for protein analyte such as prostate-specific antigen (PSA) similar LOD of 80 fM was reported (Yu et al., 2004a). Further improvement can be achieved through the employment of SPFS sensor biointerface based on a three-dimensional affinity binding matrix based on carboxylated dextran brush architecture. By this means large protein retention capacity and facile alleviating of the metal-induced fluorescence quenching can advance the SPFS readout (Yu et al., 2004a) and a model immunoassay with directly labeled analyte showed LOD as low as 0.5 fM (Yu et al., 2004b). The SPFS method can be further tailored for optical detection for larger analytes by prolonging the probing depth that is typically limited to about 100 nm. By using more complex layer architectures with a thin metallic films there can be resonantly excited long range surface plasmon (LRSP) modes that travel at the same time along both metal interfaces and probe to micrometer distances (Figure 3A) (Kasry and Knoll, 2006; Dostalek et al., 2007) This approached allowed the detection of the presence of E. coli O157:H7 with the LOD as low as 10 cfu ml−1 (Figure 3A). Besides the quest towards reaching minimum LOD, SPR in combination with SPFS readout provides a platform that allow for multimodal investigations. This approach powered by using SPR imaging was utilized in the investigation of cellular processes in living human epithelial kidney cells associated with cytoskeleton changes (Figure 3B) and other explored directions are discussed below (Chabot et al., 2013).
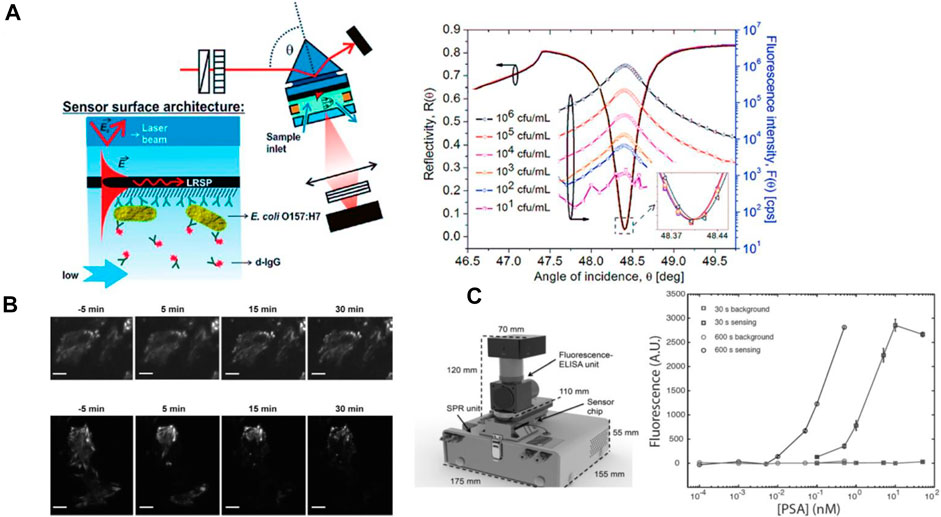
FIGURE 3. SPR coupled with fluorescence: (A) Schematics of long range surface plasmon-enhanced fluorescence immunosensor for detection of bacterial pathogen E. coli O 157:H7. (B) Surface plasmon -enhanced fluorescence images for unstimulated Rat aortic vascular smooth muscle (A7r5) cells (upper image) and in presence of latrunculin (LatA) (lower image) 5 min before stimulation at 5,15; 30 min stimulation [reprint with permission from (Chabot et al., 2013)]. (C) SPR-fluorescence-ELISA instrument together with calibration of the fluorescence output for PSA detection using. [reprint with permission from (Breault-Turcot et al., 2015a)].
To further align SPR with life science techniques, Masson et al. have reported lately on a SPR-fluorescence-ELISA instrument (Breault-Turcot et al., 2015a). While ELISA assays are robust and widely employed, they suffer from long analysis times and a multi-step analysis that lengthen the analysis process. PSA could be successfully detected at clinical concentrations from 10 pM to 50 nM with this integrated system in an assay time of 12 min with a dynamic range of nearly four orders of magnitude, largely outperforming ELISA and SPR alone with a general linear dynamic range of around two orders of magnitude (Figure 3C). Using a similar sensing scheme, the analysis of clinical samples for anti-asparginase revealed excellent co-linearity of SPR and fluorescence ELISA on a single instrument (Charbonneau et al., 2017), and its suitability for clinical sample analysis. Building on this multiplexed instrument concept, the combination of SPR with luminescence techniques was extended to electrochemiluminescence (Dinel et al., 2019). In this version of SPR, the electrochemical response (cyclic voltammetry), luminescence and SPR sensorgram were simultaneously acquired to investigate the different steps involved in the electrochemiluminescence of Ru(bpy)32+. As shown in this paper, SPR provides access to the different interfacial adsorption processes occurring at the working electrode in an electrochemical experiment, a major advantage of coupling SPR to electrochemistry.
The multimodal SPR—fluorescence biosensing was further elaborated for the investigation of thin hydrogel layers by taking advantage of layer architectures supporting LRSP and dielectric optical waveguide modes. LRSP-enhanced fluorescence was employed for the measurement of density gradients in affinity bound molecules inside a hydrogel layer serving as three-dimensional affinity binding matrix (Huang et al., 2010). Similar platform served for the investigation of hydrogel thin film thermo-responsive properties in conjunction with rapid integrated microheater (Toma et al., 2013). Another type of studies exploiting the combination of SPR and SPFS served in the context of enzymatic assays developed for the analysis of nucleic acids. Stengel and Knoll used this method for studying elongation of double stranded DNA tethered to a solid gold surface by the DNA polymerase enzyme and distinguishing of the binding kinetics of enzyme and assembly of the DNA duplex was possible (Stengel and Knoll, 2005). Similar instrument extended with optical waveguide spectroscopy was lately used for the observation of the elongation speed and conformation changes of the single stranded DNA generated on a solid sensor surface by the rolling circle amplification (Figure 4A) (Lechner et al., 2021).
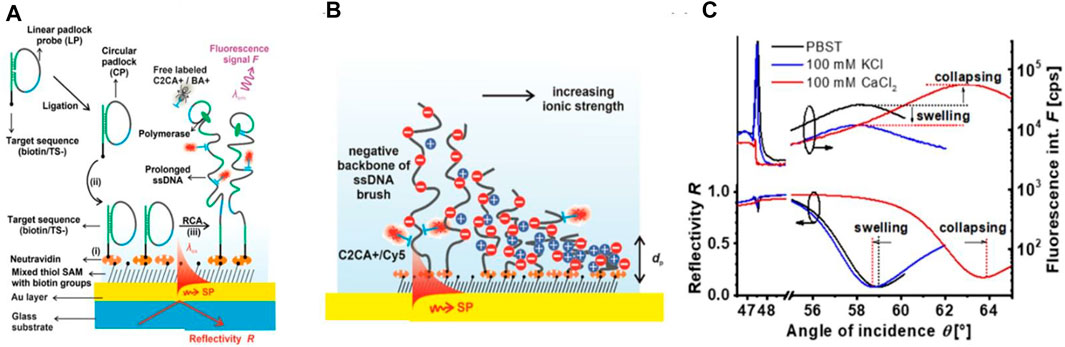
FIGURE 4. Surface plasmon resonance (SPR) and optical waveguide spectroscopy (OWS) with fluorescence labeled DNA: (A) Schematics of the growth of long single stranded DNA polyelectrolyte brush by rolling circle amplification and (B) the optical probing of its collapse by the confined field of surface plasmons with (C) respective changes in the fluorescence and reflectivity angular scans [reprint with permission from Ref (Lechner et al., 2021)].
As illustrated in Figure 4B, these strands formed a dense polyelectrolyte brush architecture that can be collapsed by incorporating calcium ions. Changes in thickness that can reach >10 μm were determined by the analysis of angular reflectivity and fluorescence spectra as shown in Figure 4C. Besides the ATR-based configurations, also grating-coupled SPR geometry was combined with epi-illumination fluorescence spectroscopy in a study on specific detection of exosomes carrying CD81 moieties by the use of magnetic nanoparticle advanced assays (Reiner et al., 2018). This approach allow for accessing the sensor surface through the glass sensor chip which is not possible when optically matched to a bulky prism.
4 SPR and mass spectrometry
Whilst SPR is widely used to study molecular interactions, mass spectrometry (MS) is capable of providing qualitative and quantitative analytical data on mass, structure and composition of an analyte. Integrating surface plasmon resonance analysis with mass spectrometry enables the detection and characterization of molecular interactions to be complemented with identification of interaction partners (Nedelkov and Nelson, 2003; Stigter et al., 2013; Xue et al., 2019). Thus, combining SPR with MS has created a unique approach to protein investigations with an additional deeper insight about molecular interactions.
SPR-MS has been pioneered successfully in the late 1990s by Krone et al. (1997) With the use of arrayed SPR chips, the analytical throughput could be largely increased (Nedelkov et al., 2006). The array format was further improved by a combination of SPR imaging (SPRi) and matrix-assisted laser desorption/ionization (MALDI) mass spectrometry measurements on the same surface, so that kinetic curves of interaction and MALDI mass spectra of the same recorded spots could be obtained (Bellon et al., 2009). Musso et al. demonstrated the potential of SPR-MS for protein biomarker analysis in saliva (Figure 5A). SPRi monitoring of the interactions on a functionalized biochip via immune capture, followed by on-chip MALDI-MS structural identification identified two salivary proteins, α-amylase and lysozyme, present at femtomole levels (Mussi et al., 2015).
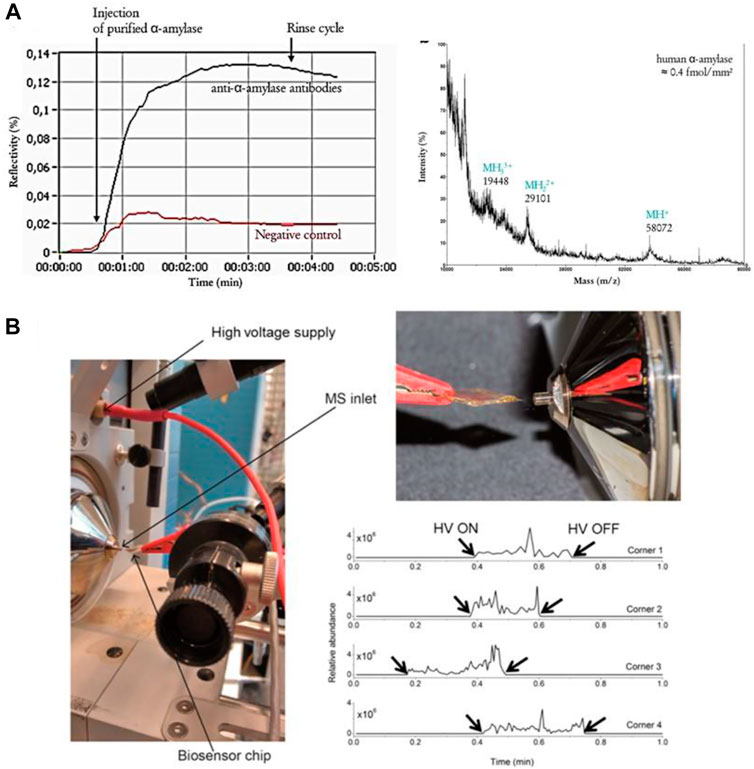
FIGURE 5. SPR-MS analysis. (A) SPRi sensorgram of the binding of α-amylase (10 μg ml−1) to anti-α-amylase antibodies followed by on-chip MALDI-TOF MS analysis [reprint with permission of (Mussi et al., 2015)] (B) (left) Proposed Biochip Spray MS set up using a gold biosensor chip held in front of the MS inlet using an alligator clip and (right) spray obtained after adding 10 μl of methanol and applying a voltage of 5 kV with extracted ion chronogram for m/z 297.1333 ([DON + H]+) [reprint with permission of (Joshi et al., 2017)].
Although, MS is one of the most sensitive and selective analytical techniques, it typically suffers from matrix effects and just like for SPR, the identification of small molecules remains a major challenge. Addressing this challenge, a dielectric barrier charge ionization (DBCI) was used as a new SPR-MS interface, to detect acetaminophen, metronidazole, quinine and hippuric acid in physiological, salt containing solutions (Zhang et al., 2016). Using this interface, samples could first be studied by SPR, then sprayed and ionized, finally analyzed by MS. Competitive assays for deoxynivalenol (DON), an important mycotoxin, and DON-OVA as signal enhancer were developed to detect DON in beer (Joshi et al., 2017). This approach was based on coupling an SPR based immuno-biosensing with ambient ionization MS (Figure 5B). DON was captured on the SPR chip containing an antifouling layer and monoclonal antibodies against the toxin and, after washing, the chip was directly analyzed by spray MS (Joshi et al., 2017). The team of Masson et al. (Forest et al., 2016) demonstrated some years ago the utility of SPRi-MALDI ionization imaging (IMS) MS for the acquisition of multiparametric information, by creating a tissue section imprint on an SPRi sensor surface. Correlated images could be acquired in SPRi and in MALDI IMS for abundant proteins from a single mouse kidney tissue. Quantitative and regioselective SPRi image correlation to MALDI IMS images was obtained for different proteins transferred from a single tissue section.
5 SPR and LFA
Lateral flow assays (LFA) have revolutionized clinical diagnostics. It is the most successful home testing platform as it requires only a few droplets of sample and enables the detection of biomarkers within minutes even when performed by non-trained personnel (Ross et al., 2018). A LFA commonly is based on sandwich immunoassay where the analyte is captured by an antibody and its presence is reported through a labelled reporter antibody. The most common labels are colloidal gold, latex beads or liposomes (Rink et al., 2022) (Figure 6A). The result of an LFA is almost always related to an optical signal generated at the test line which can be read off by the naked eye or a smart phone camera. Chemical signal amplification by metallic nanoparticles enhancement as well by enzymatic initiated color reactions can improve sensitivity in LFAs (Loynachan et al., 2018).
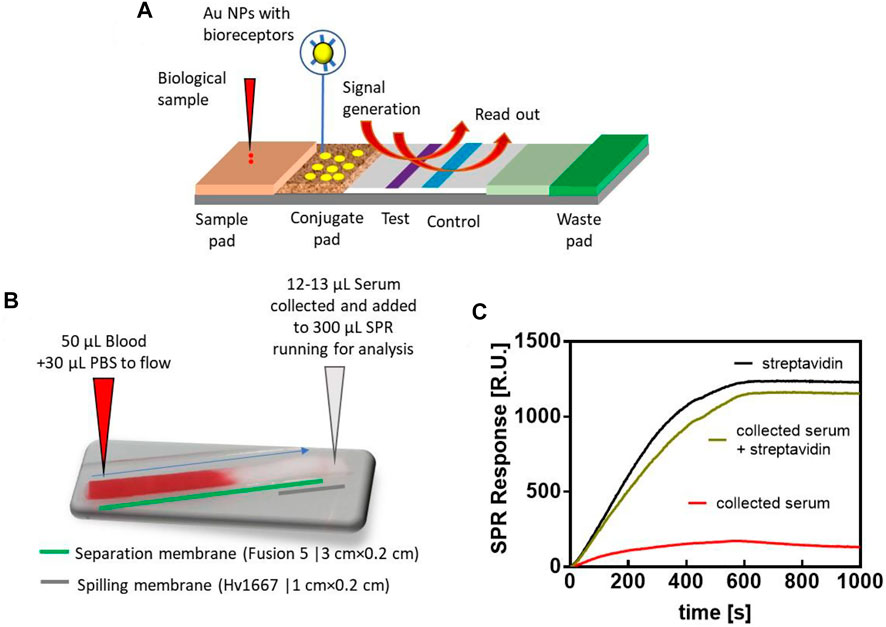
FIGURE 6. Lateral flow concepts coupled to SPR: (A) Concept of LFA with dynamic in the system due to physical analyte transport, chemical reaction and optical read out. (B) Photograph of LFA based blood separation concept together with (C) binding curve of the collected serum (red), collected serum and sample spiked with streptavidin (green) as well as a biding curve of streptavidin (2 μg ml−1) as control. SPR ships used were modified with SH-PEG-Biotin (788 g mol−1) for 3 h at 3.5 mg ml−1. Flow rate: 30 μl min−1 (Measurements were made on a portable P4SPR platform from Affinité Instruments, Canada). All experiments in this study were conducted at 25°C and all sensorgrams were fitted using Prism v7 software.
Electrochemical lateral flow assays (eLFA) capable of detecting nucleic acids have been reported for the quantitative detection of the hepatitis B virus (Srisomwat et al., 2021). A gold metallization strategy was employed for the signal-on electrochemical detection of the target DNA. Under optimal conditions, a broad dynamic range (10 p.m.–2 μM) with an excellent detection limit down to 7.23 p.m. was achieved. Early-stage detection of LipL32, an outer membrane protein present only on pathogenic Leptospira species, has lately been made possible using an integrated lateral flow immunoassay with an electrochemical readout based on a ferrocene trace with LOD of 8.53 pg ml−1 (Deenin et al., 2022).
Interestingly, the LFA concept has not been used in a hyphenated technique to-date, such as SPR-LFA. Albeit, this concept is feasible as preliminary data in our own group demonstrate. Here, we employed the blood-separation Fusion5 membrane with a spilling membrane (Hv10667) to allow the collection of serum samples to be analysed by SPR (Figure 6B). To validate that only the blood cells are separated by the membrane while other proteins will move to the spilling membrane, blood samples were spiked with BSA-streptavidin (50 μg ml−1) and the recovered serum analysed by SPR where the BSA-streptavidin conjugate is bound through HS-PEG-biotin (Figure 6C). This LFA approach could be a significant step forward in the analysis of whole blood in SPR, as prior attempts to analyze whole blood in SPR were plagued with hours-long incubation time through a microdialysis chamber (Breault-Turcot et al., 2015b) or required complex chip design (Terao et al., 2015) with a microslit arrays to filter cells and platelets. Transforming the LFA concept into capillary driven minifluidic systems may further improve the connection of SPR-LFA approaches.
6 Conclusion
SPR is an immensely powerful analytical tool that has been used routinely for affinity-based application approaches. The generation of hyphenated strategies opened up a whole new dimension of possibilities. SPR has been successfully integrated with fluorescent, mass spectrometry, electrochemical and electrical approaches. This resulted in improved sensitivity ranges, real-time analyses of product and the reaction progress, and hence true in-depth analyses even in complex matrices and tissues. The latter is for example achievable when combining imaging SPR and imaging MS and was demonstrated for the quantification and identification of proteins in entire tissue sections, which can become a new tool for histological analysis of cancer or for infectious disease diagnosis. The particular useful feature of SPR based on real-time tracking molecular interactions is not compromised in the different hyphenated strategies. Selective capturing of a target analyte on an SPR biosensor chip containing antibodies before MS analysis insures a cost-effective and time reduced analysis. If no analyte is captured by SPR, follow up qualitative and molecular information by MS analysis is not useful. While the field of SPR-MS has been steadily advancing over the years, this seems to be less true for SPR coupled to electrochemistry.This might question the research significance of SPR technology combination in this domain. Recent work on the combination of SPR with field effect transistor measurements bring however back the interest of coupling SPR to electrical readout. Via a SPR-FET device, a better understanding of intrinsic surface processes was achieved and is primordial for obtaining a better understanding of charge effects occurring in field effect transistors. Knowing that field-effect transistors performing in a liquid environment are highly dependent on the electrolyte ions as well as analyte charges, any additional information helping to understand analyte-bioreceptor interaction and the resulting change in electrical charge transfer characteristic is of high importance. In combination with tuning the optical probing depth in dual SPR and plasmon-enhanced fluorescence studies, complex surface reactions and superimposed effects accompanied with actuating responsive biointerface could be deciphered. Next in this line of hyphenated SPR strategies should be the combination with sample preparation approaches. Native biological samples typically are too complex to allow for the specific and sensitive detection of the desired biomarker. In the laboratory, various sample preparation strategies isolate and pre-concentrate the analyte through centrifugation, filtration, magnetic bead separation etc. Borrowing from the well-known rapid test format of the lateral flow assay (LFA) SPR-LFA may provide a solution to this challenge in which the sample preparation is accomplished via an LFA directing pre-concentrated or pre-isolated biomarkers onto the SPR chip. The use of smartphones as hardware and software capability for read out SPR enables the possibility of economical and accurate on-site portable sensing. The camera, screen, and LED flashlight of the smartphone are employed as components of the sensor. The design of a smartphone SPRP-i system is believed to pave the way towards the development of miniaturized and integrated smartphone iSPR biosensors for emerging point-of-use applications. Improved health management is key to provide better healthcare. Higher standards of healthcare management can be achieved by making a timely decision based on rapid diagnostics, smart data analysis, and informatics analysis. Considering the examples shown in literature of cell phone readout of SPR chips realized in small handheld adaptors plugged into a normal cell phone port, the SPR-LFA approach may become the strategy that advances rapid tests beyond the mere visual readout toward a highly quantifiable and sensitive SPR readout.
Author contributions
DG: Did experiments; DG, RB, SS, AB, WK, JD, and J-FM: Manuscript writing.
Funding
Financial support from the Centre National de la Recherche Scientifique (CNRS), the University of Lille, the Danube Privat University (DPU) and the Natural Science and Engineering Research Council of Canada (NSERC) is acknowledged. DG thanks for an ERASMUS support funding. The support of ANR VDOSAGE (ANR-20-CE19-0022) is acknowledged. Support by the “Fonds France-Canada pour la Recherche” is acknowledged. JD was supported by the Czech Science Fund through the project APLOMA (22-30456J).
Conflict of interest
The author declares that the research was conducted in the absence of any commercial or financial relationships that could be construed as a potential conflict of interest.
Publisher’s note
All claims expressed in this article are solely those of the authors and do not necessarily represent those of their affiliated organizations, or those of the publisher, the editors and the reviewers. Any product that may be evaluated in this article, or claim that may be made by its manufacturer, is not guaranteed or endorsed by the publisher.
References
Andersson, O., Christian, U., Fredrik, B., and Bo, L. (2008). Imaging SPR for detection of local electrochemical processes on patterned surfaces. Sensors Actuators B Chem. 134, 545–550. doi:10.1016/j.snb.2008.05.042
Aspermair, P., Ramach, U., Reiner-Rozman, C., Fossati, S., Lechner, B., Moya, S. E., et al. (2020). Dual monitoring of surface reactions in real time by combined surface-plasmon resonance and field-effect transistor interrogation. J. Am. Chem. Soc. 142, 11709–11716. doi:10.1021/jacs.9b11835
Attridge, J. W., Daniel, P. B., Deacon, J. K., Robinson, G. A., and Davidson, G. P. (1991). Sensitivity enhancement of optical immunosensors by the use of a surface plasmon resonance fluoroimmunoassay. Biosens. Bioelectron. 6, 201–214. doi:10.1016/0956-5663(91)80005-i
Baba, A., Taranekar, P., Ponnapati, R. R., Knoll, W., and Advincula, R. C. (2010). Electrochemical surface plasmon resonance and waveguide-enhanced glucose biosensing with N-alkylaminated polypyrrole/glucose oxidase multilayers. ACS Appl. Mat. Interfaces 2, 2347–2354. doi:10.1021/am100373v
Bauch, M., Toma, K., Toma, M., Zhang, Q., and Dostalek, J. (2014). Plasmon-enhanced fluorescence biosensors: A review. Plasmonics 9, 781–799. doi:10.1007/s11468-013-9660-5
Bellon, S., Buchmann, W., Gonnet, F., Jarroux, N., Anger-Leroy, M., Guillonneau, F., et al. (2009). Hyphenation of surface plasmon resonance imaging to matrix-assisted laser desorption ionization mass spectrometry by on-chip mass spectrometry and tandem mass spectrometry analysis. Anal. Chem. 81, 7695–7702. doi:10.1021/ac901140m
Breault-Turcot, J., Masson, J. F., and Microdialysis, S. P. R. (2015). Microdialysis SPR: Diffusion-gated sensing in blood. Chem. Sci. 6, 4247–4254. doi:10.1039/c5sc00716j
Breault-Turcot, J., Poirier-Richard, H.-P., Couture, M., Pelechacz, D., and Masson, J.-F. (2015). Single chip SPR and fluorescent ELISA assay of prostate specific antigen. Lab. Chip 15, 4433–4440. doi:10.1039/c5lc01045d
Calcagno, M., D’Agata, R., Breveglieri, G., Borgatti, M., Bellassai, N., Gambari, R., et al. (2022). Nanoparticle-enhanced surface plasmon resonance imaging enables the ultrasensitive detection of non-amplified cell-free fetal DNA for non-invasive prenatal testing. Anal. Chem. 94, 1118–1125. doi:10.1021/acs.analchem.1c04196
Chabot, V., Miron, Y., Charette, P. G., and Grandbois, M. (2013). Identification of the molecular mechanisms in cellular processes that elicit a surface plasmon resonance (SPR) response using simultaneous surface plasmon-enhanced fluorescence (SPEF) microscopy. Biosens. Bioelectron. 50, 125–131. doi:10.1016/j.bios.2013.06.018
Charbonneau, D. M., reault-Turcot, J., Sinnett, D., Krajinovic, M., Leclerc, J. M., Masson, J. F., et al. (2017). Tracking silent hypersensitivity reactions to asparaginase during leukemia therapy using single-chip indirect plasmonic and fluorescence immunosensing. ACS Sens. 2, 1761–1766. doi:10.1021/acssensors.7b00584
Chieng, A., Chiang, M., Triloges, K., Chang, M., and Wang, Y. (2019). Recent progress in the studies of electrochemical interfaces by surface plasmon resonance spectroscopy and microscopy. Curr. Opin. Electrochem. 13, 94–99. doi:10.1016/j.coelec.2018.11.002
Dallaire, A.-M., Patskovsky, S., Vallée-Bélisle, A., and Meunier, M. (2015). Electrochemical plasmonic sensing system for highly selective multiplexed detection of biomolecules based on redox nanoswitches. Biosens. Bioelectron. 71, 75–81. doi:10.1016/j.bios.2015.04.011
Deenin, W., Yakoh, A., Kreangkaiwal, C., Chailapakul, O., Patarakul, K., and Chaiyo, S. (2022). Integrated lateral flow electrochemical strip for leptospirosis diagnosis. Anal. Chem. 94, 2554–2560. doi:10.1021/acs.analchem.1c04440
Dinel, M. P., Tartaggia, S., Wallace, G. Q., Boudreau, D., Masson, J. F., and Polo, F. (2019). The fundamentals of real-time surface plasmon resonance/electrogenerated chemiluminescence. Angew. Chem. Int. Ed. Engl. 58, 18202–18206. doi:10.1002/anie.201909806
Dostalek, J., Kasry, A., and Knoll, W. (2007). Long range surface plasmons for observation of biomolecular binding events at metallic surfaces. Plasmonics 2, 97–106. doi:10.1007/s11468-007-9037-8
Dostalek, J., and Knoll, W. (2008). Biosensors based on surface plasmon-enhanced fluorescence spectroscopy. Biointerphases 3, FD12–FD22. doi:10.1116/1.2994688
Fang, S., Lee, H. J., Wark, A. W., and Corn, R. M. (2006). Attomole microarray detection of microRNAs by nanoparticle-amplified SPR imaging measurements of surface polyadenylation reactions. J. Am. Chem. Soc. 128, 14044–14046. doi:10.1021/ja065223p
Forest, S., Breault-Turcot, J., Chaurand, P., and Masson, J.-F. (2016). Surface plasmon resonance imaging-MALDI-TOF imaging mass spectrometry of thin tissue sections. Anal. Chem. 88, 2072–2079. doi:10.1021/acs.analchem.5b03309
Fortin, E., Defontaine, Y., Mailley, P., Livache, T., and Szunerits, S. (2005). Micro-imprinting of oligonucleotides and oligonucleotide gradients on gold surfaces: A new approach based on the combination of scanning electrochemical microscopy and surface plasmon resonance imaging (SECM/SPR-i). Electroanalysis 17, 495–503. doi:10.1002/elan.200403187
Geddes, C. D., Cao, H., Gryczynski, I., Gryczynski, Z., and Fang, J. Y. L. (2003). Metal-enhanced fluorescence (MEF) due to silver colloids on a planar surface: Potential applications of indocyanine green to in vivo imaging. J. Phys. Chem. A 107, 3443–3449. doi:10.1021/jp022040q
Hankens, D. G., and Corn, R. M. (1997). Electric fields and interference effects inside noncentrosymmetric multilayer films at electrode surfaces from electrochemically modulated surface plasmon resonance experiments. Anal. Chem. 69, 3665–3673. doi:10.1021/ac970208b
He, L., Musick, M. D., Nicewarner, S. R., Salinas, F. G., Benkovic, S. J., Natan, M. J., et al. (2000). Colloidal Au-enhanced surface plasmon resonance for ultrasensitive detection of DNA hybridization. J. Am. Chem. Soc. 122, 9071–9077. doi:10.1021/ja001215b
Hou, Y., An, J., Deng, C., Chen, S., and Xiang, J. (2016). Redox-dependent interactions between reduced/oxidized cytochrome c and cytochrome c oxidase evaluated by in-situ electrochemical surface plasmon resonance. Anal. Bioanal. Chem. 408, 4935–4941. doi:10.1007/s00216-016-9586-9
Huang, C. J., Dostalek, J., and Knoll, W. (2010). Long range surface plasmon and hydrogel optical waveguide field-enhanced fluorescence biosensor with 3D hydrogel binding matrix: On the role of diffusion mass transfer. Biosens. Bioelectron. 26, 1425–1431. doi:10.1016/j.bios.2010.07.072
Joshi, S., Zuilhof, H., van Beek, T. A., and Nielen, M. W. F. B. (2017). Biochip spray: Simplified coupling of surface plasmon resonance biosensing and mass spectrometry. Anal. Chem. 89, 1427–1432. doi:10.1021/acs.analchem.6b04012
Kang, X., Cheng, G., and Dong, S. (2001). A novel electrochemical SPR biosensor. Electrochem. Commun. 3, 489–493. doi:10.1016/s1388-2481(01)00170-9
Kasry, A., and Knoll, W. (2006). Long range surface plasmon fluorescence spectroscopy. Appl. Phys. Lett. 89, 101106. doi:10.1063/1.2345594
Krone, J. R., Nelson, R. W., Dogruel, D., Williams, P., and Granzow, R. (1997). BIA/MS: Interfacing biomolecular interaction analysis with mass spectrometry. Anal. Biochem. 244, 124–132. doi:10.1006/abio.1996.9871
Lakowicz, J. R., Malicka, J., Gryczynski, I., and Gryczynski, Z. (2003). Directional surface plasmon-coupled emission: A new method for high sensitivity detection. Biochem. Biophys. Res. Commun. 307, 435–439. doi:10.1016/s0006-291x(03)01214-2
Lechner, B., Hageneder, S., Schmidt, K., Kreuzer, M. P., Conzemius, R., Ereimhult, E., et al. (2021). In situ monitoring of rolling circle amplification on a solid support by surface plasmon resonance and optical waveguide spectroscopy. ACS Appl. Mat. Interfaces 13, 32352–32362. doi:10.1021/acsami.1c03715
Lieberman, T., and Knoll, W. (2000). Surface-plasmon field-enhanced fluorescence spectroscopy. Colloids Surfaces A Physicochem. Eng. Aspects 171, 115–130. doi:10.1016/s0927-7757(99)00550-6
Liu, J., Tian, S., Tiefenauer, L., Nielsen, P. E., and Knoll, W. (2005). Simultaneously amplified electrochemical and surface plasmon optical detection of DNA hybridization based on ferrocene-streptavidin conjugates. Anal. Chem. 77, 2756–2761. doi:10.1021/ac048088c
Liu, X.-W., Yang, Y., Wang, W., Wang, S., Gao, M., Wu, J., et al. (2017). Plasmonic-based electrochemical impedance imaging of electrical activities in single cells. Angew. Chem. Int. Ed. Engl. 56, 8855–8859. doi:10.1002/anie.201703033
Loynachan, C. N., Thomas, M. R., Gray, E. R., Richards, D. A., Kim, J., Miller, B. S., et al. (2018). Platinum nanocatalyst amplification: Redefining the gold standard for lateral flow immunoassays with ultrabroad dynamic range. ACS Nano 12, 279–288. doi:10.1021/acsnano.7b06229
Mussi, J., Buchmann, W., Gonnet, F., Jarroux, N., Bellon, S., Frydman, C., et al. (2015). Biomarkers probed in saliva by surface plasmon resonance imaging coupled to matrix-assisted laser desorption/ionization mass spectrometry in array format. Anal. Bioanal. Chem. 407, 1285–1294. doi:10.1007/s00216-014-8373-8
Nedelkov, D., and Nelson, R. W. (2003). Surface plasmon resonance mass spectrometry: Recent progress and outlooks. Trends Biotechnol. 21, 301–305. doi:10.1016/S0167-7799(03)00141-0
Nedelkov, D., Tubbs, K. A., and Nelson, R. W. (2006). Surface plasmon resonance-enabled mass spectrometry arrays. Electrophoresis 27, 3671–3675. doi:10.1002/elps.200600065
Patskovsky, S., Dallaire, A.-M., and Meunier, M. (2016). Electrochemical surface plasmon resonance sensing with absorptive redox mediator film. Sensors Actuators B Chem. 222, 71–77. doi:10.1016/j.snb.2015.08.051
Reiner, A. T., Fossati, S., and Dostalek, J. (2018). Biosensor platform for parallel surface plasmon-enhanced epifluorescence and surface plasmon resonance detection. Sensors Actuators B Chem. 257, 594–601. doi:10.1016/j.snb.2017.10.116
Ribereio, J. A., Sales, M. G. F., and Pereira, C. M. (2021). Electrochemistry-assisted surface plasmon resonance biosensor for detection of CA 15–3. Anal. Chem. 98, 7815–7824. doi:10.1021/acs.analchem.0c05367
Ribereio, J. A., Sales, M. G. F., and Pereira, C. M. (2020). Electrochemistry-assisted surface plasmon resonance detection of miRNA-145 at femtomolar level. Sensors Actuators B Chem. 316, 128129. doi:10.1016/j.snb.2020.128129
Riberio, J. A., Sales, M. G. F., and Pereira, C. M. (2020). Electrochemistry combined-surface plasmon resonance biosensors: A review. Trend. Anal. Chem. 2022, 116766.
Rink, S., Kaiser, B., Steiner, M.-S., Duerkop, A., and Baeumner, A. J. (2022). Highly sensitive interleukin 6 detection by employing commercially ready liposomes in an LFA format. Anal. Bioanal. Chem. 414, 3231–3241. doi:10.1007/s00216-021-03750-5
Rodríguez-Lorenzo, L., de la Rica, R., Álvarez-Puebla, R. A., Liz-Marzán, L. M., and Stevens, M. M. (2012). Plasmonic nanosensors with inverse sensitivity by means of enzyme-guided crystal growth. Nat. Mat. 11, 604–607. doi:10.1038/nmat3337
Ross, G. M. S., Bremer, M. G. E. G., Wichers, H., an Amerongen, A., and Nielen, W. F. (2018). Rapid antibody selection using surface plasmon resonance for high-speed and sensitive hazelnut lateral flow prototypes. Biosensors 8, 130. doi:10.3390/bios8040130
Shan, X., Patel, U., Wang, S., Iglesias, R., and Tao, N. (2010). Imaging local electrochemical current via surface plasmon resonance. Science 327, 1363–1366. doi:10.1126/science.1186476
Sokolov, K., Chumanov, G., and Cotton, T. M. (1998). Enhancement of molecular fluorescence near the surface of colloidal metal films. Anal. Chem. 70, 3898–3905. doi:10.1021/ac9712310
Srisomwat, C., Yakoh, A., Chuaypen, N., Tangkijvanich, P., Vilaivan, T., and Chailapakul, O. (2021). Amplification-free DNA sensor for the one-step detection of the hepatitis B virus using an automated paper-based lateral flow electrochemical device. Anal. Chem. 93, 2879–2887. doi:10.1021/acs.analchem.0c04283
Stengel, G., and Knoll, W. (2005). Surface plasmon field-enhanced fluorescence spectroscopy studies of primer extension reactions. Nucleic Acids Res. 33, e69. doi:10.1093/nar/gni067
Stigter, E. C. A., de Jong, G. J., and van Bennekom, W. P. (2013). Coupling surface-plasmon resonance and mass spectrometry to quantify and to identify ligands. TrAC Trends Anal. Chem. 45, 107–120. doi:10.1016/j.trac.2012.09.004
Szunerits, S., Knorr, N. C., and Livache, T. (2004). New approach to writing and simultaneous reading of micropatterns: Combining surface plasmon resonance imaing with scanning electrochemical microscopy (SECM). Langmuir 20, 9236–9241. doi:10.1021/la0492557
Terao, K., Hiramatsu, S., Suzuki, T., Takao, H., Shimokawa, F., and Oohira, F. (2015). Fast protein detection in raw blood by size-exclusion SPR sensing. Anal. Methods 7, 6483–6488. doi:10.1039/c5ay01045d
Toma, M., Jonas, U., Mateescu, A., Knoll, W., and Dostalek, J. (2013). Active control of SPR by thermoresponsive hydrogels for biosensor applications. J. Phys. Chem. C Nanomater. Interfaces 117, 11705–11712. doi:10.1021/jp400255u
Wang, S., Huang, X., Shan, X., Foley, K. J., and Tao, N. (2010). Electrochemical surface plasmon resonance: Basic formalism and experimental validation. Anal. Chem. 82, 935–941. doi:10.1021/ac902178f
Wang, Y., Shan, X., Wang, H., Wang, S., and Tao, N. (2017). Plasmonic imaging of surface electrochemical reactions of single gold nanowires. J. Am. Chem. Soc. 139, 1376–1379. doi:10.1021/jacs.6b10693
Xue, J., Bai, Y., and Liu, H. (2019). Hybrid methods of surface plasmon resonance coupled to mass spectrometry for biomolecular interaction analysis. Anal. Bioanal. Chem. 411, 3721–3729. doi:10.1007/s00216-019-01906-y
Yu, F., Persson, B., Löfås, S., and Knoll, W. (2004). Attomolar sensitivity in bioassays based on surface plasmon fluorescence spectroscopy. J. Am. Chem. Soc. 126, 8902–8903. doi:10.1021/ja048583q
Yu, F., Persson, B., Löfås, S., and Knoll, W. (2004). Surface plasmon fluorescence immunoassay of free prostate-specific antigen in human plasma at the femtomolar level. Anal. Chem. 76, 6765–6770. doi:10.1021/ac048937w
Keywords: analytical science, surface plasmon resonace (SPR), mass spectroscopic, electrochemistry, lateral flow assay (LFA)
Citation: Geilfuss D, Boukherroub R, Dostalek J, Knoll W, Masson J-F, Baeumner AJ and Szunerits S (2022) Can classical surface plasmon resonance advance via the coupling to other analytical approaches?. Front. Anal. Sci. 2:1091869. doi: 10.3389/frans.2022.1091869
Received: 11 November 2022; Accepted: 07 December 2022;
Published: 16 December 2022.
Edited by:
Jason Cheng, University of California, Riverside, United StatesCopyright © 2022 Geilfuss, Boukherroub, Dostalek, Knoll, Masson, Baeumner and Szunerits. This is an open-access article distributed under the terms of the Creative Commons Attribution License (CC BY). The use, distribution or reproduction in other forums is permitted, provided the original author(s) and the copyright owner(s) are credited and that the original publication in this journal is cited, in accordance with accepted academic practice. No use, distribution or reproduction is permitted which does not comply with these terms.
*Correspondence: Sabine Szunerits, c2FiaW5lLnN6dW5lcml0c0B1bml2LWxpbGxlLmZy