- 1Department of Health Sciences and Technology, ETH Zurich, Zurich, Switzerland
- 2Lewis-Sigler Institute for Integrative Genomics, Princeton University, Princeton, NJ, United States
The function of General Control Nonderepressible 2 (GCN2), an evolutionary-conserved component of the integrated stress response (ISR), has been well-documented across organisms from yeast to mammals. Recently GCN2 has also gained attention for its role in health and disease states. In this review, we provide a brief overview of GCN2, including its structure, activation mechanisms and interacting partners, and explore its potential significance as a therapeutic target in various age-related diseases including neurodegeneration, inflammatory disorders and cancer. Finally, we summarize the barriers to effectively targeting GCN2 for the treatment of disease and to promote a healthier aging process.
Introduction
GCN2 was first identified in yeast as a serine/threonine kinase and later recognized as the homolog of mouse and human eukaryotic translation initiation factor 2 alpha kinase 4 (EIF2AK4). It functions as an amino acid sensor and serves to activate the integrated stress response (ISR) upon amino acid deprivation. Proper ISR activation is essential for cells to make “live or die” decisions and maintain organismal homeostasis under stress, and GCN2 plays a central role in this system.
More than two decades ago, GCN2’s role in nutrient sensing was first reported in a screen for genes functioning in the general amino acid control pathway in budding yeast Saccharomyces cerevisiae (Hinnebusch, 1985). The activation of GCN2 under amino acid starvation has been well studied, particularly in yeast. When levels of a specific amino acid are limiting, deacylated (“uncharged”) levels of the corresponding tRNA increase. Uncharged tRNAs directly bind to GCN2 on its histidyl-tRNA synthetase-like (HisRS) domain and thereby convert GCN2 from an autoinhibited state to a functionally active state by driving homodimerization and autophosphorylation (Diallinas and Thireos, 1994; Wek et al., 1995; Narasimhan et al., 2004; Gallinetti et al., 2013) (Figure 1). Active GCN2 phosphorylates the alpha subunit of the translation initiation factor eIF2 (EIF2A) at serine 51 (Lu et al., 1999; Holcik and Sonenberg, 2005; Ravindran et al., 2014). This leads to a decrease in global protein translation while allowing for preferential translation of specific stress-responsive transcripts, including activating transcription factor 4 (ATF4), which can push a cell towards either survival or regulated cell death depending on the severity of the stress stimulus (Pakos-Zebrucka et al., 2016; Costa-Mattioli and Walter, 2020). The ISR pathway also comprises a negative feedback component, including ATF4-driven expression of machinery to dephosphorylate eIF2α, allowing a return to normal cellular function once the stressor is overcome. The ISR plays a major role in health and disease states and tends to become dysregulated with aging. Additionally, mild activation of GCN2 via dietary protein dilution or individual amino acid restriction has been shown to extend lifespan in preclinical models.
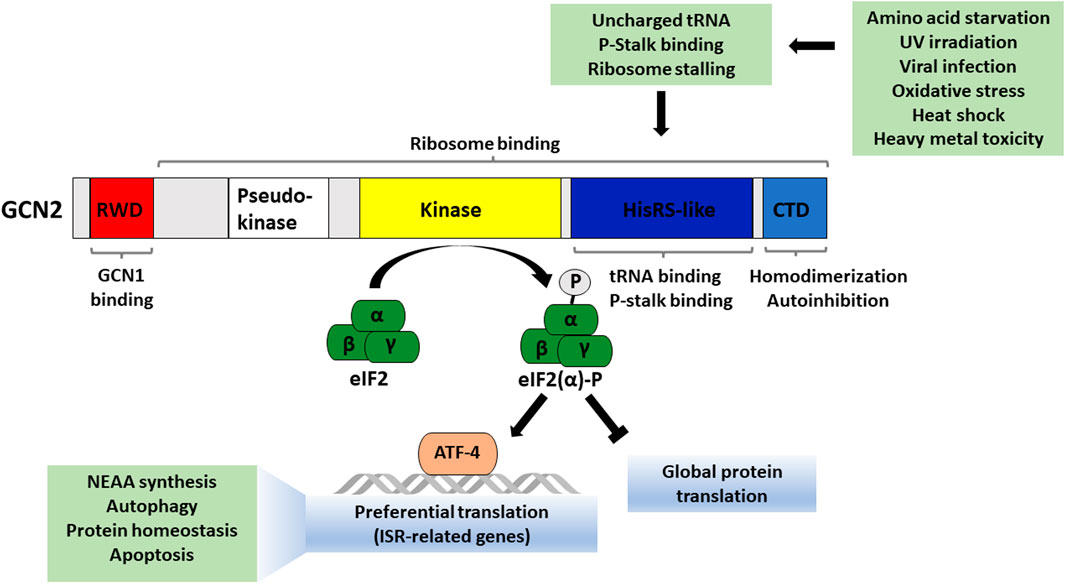
Figure 1. Overview of GCN2 protein domains and factors that activate GCN2. Active GCN2 phosphorylates the α subunit of eukaryotic translation initiation factor 2 which reduces global protein translation and induces selective stress-adaptive gene transcription, with targets including ATF4.
As populations worldwide are rapidly aging, it is critical to identify strategies to extend healthspan and prevent or delay age-related diseases. Here, we review the role of GCN2 in the ISR and its potential as a therapeutic target in age-related diseases.
Structure and activation of GCN2
GCN2 was first identified as an amino acid sensor, and amino acid deprivation is its canonical activating stimulus. However, it has also been shown that other stress stimuli can activate GCN2, including UV irradiation, oxidative stress, ribosome collisions, ribosomal p-stalk binding and proteasome inhibition (Deng et al., 2002; Jiang and Wek, 2005; Anda et al., 2017; Harding et al., 2019; Inglis et al., 2019; Wu et al., 2020).
GCN2 consists of five conserved domains, including an N-terminal RWD domain (named due to its presence in RING finger and WD repeat containing proteins and DEAD-like helicases), pseudokinase domain, kinase domain, HisRS-like domain (with sequence similarity to histidyl-tRNA synthetase) and C-terminal domain (CTD) (Figure 1). Under conditions of amino acid sufficiency, GCN2 is in an inactive dimeric form, and the physical interaction between its kinase domain, HisRS-like domain, and CTD prevents its activation (Lageix et al., 2015). Binding of uncharged tRNAs is required for sensing amino acid deprivation by GCN2. Upon binding of uncharged tRNAs to its HisRS-like domain, allosteric changes in GCN2’s structure cause the loss of autoinhibitory contacts between its domains. GCN2 gets activated via autophosphorylation (Threonine-882 and -887 in S. cerevisiae, Threonine-898 and -903 in mouse, and Threonine-899 and -904 in human) in the activation loop of its kinase domain (Romano et al., 1998; Sood et al., 2000; Cherkasova et al., 2010). Finally, active GCN2 phosphorylates its substrate, eIF2α (Qiu et al., 2001; Padyana et al., 2005; Gárriz et al., 2009). Phosphorylated eIF2α forms a complex with eIF2B, preventing its function as a guanine nucleotide exchange factor. When recycling of GDP for GTP on eIF2 is inhibited, the ternary complex cannot form, and translation cannot initiate. This set of events leads to a decrease in global translation. At the same time, there is also an increase in the translation of a specific set of stress-responsive transcripts, including the transcription factor ATF4 (Blais et al., 2004).
The full crystal structure of GCN2 in inactive and active states and in complex structure with its interacting partners (GCN1, GCN20, and ribosomal components) have yet to be elucidated. Our current knowledge of GCN2 structure is limited to its kinase domain and CTD (Padyana et al., 2005; He et al., 2014; Maia de Oliveira et al., 2020). Further studies on the complete crystal structure of GCN2 will help to better understand the structural regulation of GCN2 which will eventually aid in the development of structure-based drugs to modulate GCN2 activity.
Interacting partners of GCN2
Several conserved proteins interact with GCN2 directly or indirectly to modulate its activity. Gcn1 (general control non-derepressible 1) and Gcn20 (an ATP-binding cassette family protein) are important for the activation of yeast Gcn2 during amino acid starvation in vivo but not required for GCN2 kinase function in vitro (Hinnebusch, 1985; Marton et al., 1993; Vazquez de Aldana et al., 1995). Gcn1 also has binding domains for ribosome components and Gcn20, both of which are important for Gcn2 activation (Vazquez de Aldana et al., 1995; Marton et al., 1997; Pochopien et al., 2021). The Gcn1/Gcn20 protein complex binds to the GCN2 N-terminus, and this binding is required for efficient GCN2 activation in vivo (Garcia-Barrio et al., 2000). GCN1 is subject to negative regulation by yeast Yih1 (mammalian IMPACT), which prevents GCN1 binding to GCN2, thus indirectly inhibiting GCN2 (Pereira et al., 2005). GCN1 is highly conserved across yeast, Arabidopsis, Drosophila and mammals (Marton et al., 1993; Izquierdo et al., 2018) with multiple examples of cross-species interaction between GCN1 and GCN2 homologs (Garcia-Barrio et al., 2000). While Gcn20 does not interact with the ribosome or Gcn2 directly, it supports the interaction between Gcn1 and Gcn2 and tethering of the complex to the ribosome. Within the complex, Gcn1 directly binds to the RWD domain of Gcn2 and functions as a chaperone to transfer uncharged tRNA from the ribosomal A-site to the HisRS-like domain of Gcn2 upon amino acid starvation, thus facilitating Gcn2 activation (Sattlegger and Hinnebusch, 2000; 2005).
GCN2 targets multiple downstream effectors to control translation initiation. In addition to eIF2α (yeast Sui2), GCN2 also phosphorylates the beta subunit of the eIF2 complex (yeast Sui3) upon amino acid deprivation. This promotes association with the translation initiation factor eIF5 and restricts recycling of the eIF2 ternary complex (Dokládal et al., 2021). Additionally, GCN2 engages in an inhibitory feedback loop through which GCN20 phosphorylation weakens GCN1-GCN20 complex formation and suppresses activation of GCN2 by uncharged tRNAs (Dokládal et al., 2021). These multiple interaction partners represent the various layers of control in the GCN2 signaling pathway with targeted disruption of these interactions representing a promising strategy to control GCN2 activation.
GCN2 in longevity
GCN2 has been implicated in the control of lifespan across multiple species. In Saccharomyces cerevisiae Gcn2 activity is required for lifespan extension by amino acid restriction (Wu et al., 2013). Another study in yeast showed that Gcn2 activation upon tRNA overexpression suppresses global translation and extends the lifespan of young cells in a Gcn4 (yeast ATF4)-dependent manner (Hu et al., 2018). In Drosophila melanogaster GCN2/ATF4 regulate the induction of the translational repressor 4E-BP to extend lifespan upon ER stress and nutrient restriction (Kang et al., 2017). Tissue-specific knockdown of GCN2 in the gut and fat body, two energy-sensing organs in Drosophila which are counterparts of mammalian liver and adipose tissue, abolishes the beneficial effect of dietary amino acid restriction on lifespan (Kim et al., 2020).
In Caenorhabditis elegans, deletion or RNAi knockdown of gcn-2 produces minimal phenotype in the basal unstressed condition in terms of lifespan, growth, development, movement, and fecundity (Rousakis et al., 2013). However, lifespan extension in eat-2 and let-363 mutants were lost upon gcn-2 knockout, suggesting a role for gcn-2 in lifespan extension by dietary restriction and TOR inhibition (Rousakis et al., 2013). Deletion of the gcn-2 negative regulator impt-1 resulted in ISR activation and lifespan extension in C. elegans (Ferraz et al., 2016). Lifespan extension by impt-1 deletion was dependent on both gcn-2 and the C. elegans ATF4 homolog atf-5 (Ferraz et al., 2016).
Data across species demonstrate that activation of GCN2 by multiple stimuli, including amino acid restriction, is associated with increased lifespan. Multiple mechanisms may contribute downstream of GCN2 activation. First, interventions that reduce global protein translation are associated with extended lifespan (Hansen et al., 2007). Second, increased selective translation of stress-responsive factors, including ATF4, can drive geroprotective molecular programs such as increased autophagy (Li et al., 2014; Statzer et al., 2022). This combination of reduced global translation and induction of pro-survival pathways during perceived nutrient deprivation provides the conditions for the organism to save energy and ensure survival requirements are optimally met. While the causal evidence in non-mammalian systems is robust, data on the role of GCN2 in mammalian aging is currently only associative. Evidence is still lacking on whether GCN2 signaling is altered in aging, or whether GCN2 is required for lifespan extension by nutrient restriction in mammals. Further, whether direct modulation of GCN2 activity or levels impacts the mammalian aging process also remains an important open question.
GCN2 in healthy aging
Aging is a complex process that drives changes across physiologic and molecular domains, collectively giving rise to pathological conditions that increase an individual’s susceptibility to age-related diseases. A properly functioning ISR maintains a delicate balance between health and disease in organisms across the lifespan. GCN2 contributes to the critical regulation by the ISR to drive cell survival or death and has been associated with inflammatory, metabolic, neurological and metastatic diseases. Despite substantial progress, the potential therapeutic applications of GCN2 remain an ongoing and dynamic field of research.
GCN2 plays a significant role in the immune system and is associated with various diseases related to immune function as reviewed in Xia et al. (2018). GCN2 is reported to suppress the activation of the inflammasome and control inflammation in the intestine (Ravindran et al., 2016). GCN2-deficient mice display hypersensitivity to DSS-induced colitis with increased weight loss, enhanced inflammation, Th17 response, colonic shortening, and impairment of the epithelial barrier. Conversely, GCN2 activation by amino acid restriction significantly protects against DSS colitis by inhibiting reactive oxygen species-mediated inflammasome activation, suppressing pathogenic Th17 response and promoting autophagy to reduce inflammation (Ravindran et al., 2016; Revelo et al., 2016). Tissue-specific GCN2 deletion in intestinal epithelial cells and CD11c+ antigen-presenting cells (APCs) also leads to increased intestinal inflammation, weight loss and colon shortening in mice. These data in colitis models support GCN2 as a potential target in intestinal inflammatory diseases with therapeutic strategies including activation by either small molecules or amino acid-restricted diets.
GCN2 supports adaptation to amino acid stress by the immune system, while GCN2 loss renders the immune system more sensitive to the cytotoxic effects of amino acid deprivation, leading to enhanced lymphocyte loss (Bunpo et al., 2010). Under basal conditions, the immune system prevents autoimmunity by clearing apoptotic cells and driving immune tolerance toward self-antigens. Myeloid GCN2 deletion in an autoimmunity-prone mouse model suppresses the development of tolerance and increases autoimmunity, renal pathology and overall mortality (Ravishankar et al., 2015). This is driven by a lack of apoptotic clearance as GCN2 null liver macrophages are unable to clear damaged red blood cells and recycle iron upon hemolytic stress (Toboz et al., 2022). Mechanistically, apoptotic cells activate the tryptophan-catabolizing enzyme IDO1 in macrophages which signals to GCN2 to regulate cytokine production, increasing anti-inflammatory IL-10 responses while decreasing pro-inflammatory IL-12 (Ravishankar et al., 2015). GCN2 is required for IDO1 produced by dendritic cells to signal and suppress proliferation in T-cells (Munn et al., 2005; Baban et al., 2009; Sharma et al., 2009). GCN2 activation in mice suppresses pro-inflammatory cues and decreases the recruitment of macrophages to the kidney during glomerular inflammation (Chaudhary et al., 2015), while the inhibition of autophagy or knockout of IDO1 or GCN2 in mice exacerbates nephritis to fatal end-stage renal failure. Conversely, increasing kidney IDO1 activity or treating mice with a GCN2 agonist drives autophagy and protects mice from nephritic kidney damage.
Many studies have also implicated GCN2 activity in cancer, and a growing body of evidence supports modulating GCN2 activity as a potential cancer therapeutic strategy (Gold and Masson, 2022). A common characteristic of the tumor microenvironment is limited nutrient availability. As cells require an increased supply of nutrients due to enhanced anabolism, the consumption of available nutrients can rapidly drive nutrient deprivation within tumors (DeBerardinis et al., 2008). To deal with the nutrient stress, some cancer cells activate GCN2, which drives adaptations, including increased expression of amino acid transporters and non-essential amino acid synthetic enzymes (Wang et al., 2013; Cordova et al., 2022). For example, GCN2 drives the expression of asparagine synthetase (ASNS) via eIF2a/ATF4, which is required for cancer cell survival upon asparaginase treatment. Co-treatment with asparaginase and GCN2 inhibition or deletion synergize with asparaginase against multiple cancer cell types (Ye et al., 2010; Wang et al., 2013; Nakamura et al., 2018). Similarly, hepatocellular carcinoma cells become auxotrophic for arginine due to suppressed urea cycle and are sensitive to GCN2 inhibition or deletion due to a requirement for GCN2-driven expression of the transporter SLC7A1 (Missiaen et al., 2022). GCN2 also promotes VEGF-driven angiogenesis, supporting tumor cell growth and survival by increasing nutrient supply (Ye et al., 2010; Longchamp et al., 2018; Zhang et al., 2021).
Autophagy is an important process for cancer cell survival as it recycles proteins and other macromolecules during nutrient deprivation and improves the resistance of cancer cells to stress conditions that normally lead to cell death including hypoxia (Galluzzi et al., 2015). Under leucine deprivation, ATF4 drives transcription of autophagy genes in a GCN2-dependent manner, supporting cell survival (B’chir et al., 2013). These findings all support the suppression of GCN2 activity as a promising therapeutic strategy to suppress adaptive amino acid transport/synthesis, angiogenesis, and autophagy in cancer cells.
Cancer therapy-induced cardiotoxicity caused by chemotherapy including doxorubicin (Dox) is a significant challenge in cancer treatment and is strongly linked to morbidity and mortality (Carver et al., 2007). Dox-treated GCN2 knockout mice display less myocardial fibrosis, contractile dysfunction, cardiomyocyte death, and oxidative stress as compared to wildtype mice (Lu et al., 2014; Wang et al., 2018). On the other hand, GCN2 knockout mice were significantly more susceptible to hepatotoxicity during asparaginase treatment, showing increased hepatic oxidative stress and inflammation (Wilson et al., 2013). These findings support modulation of GCN2 activity not only for treating cancer but also for attenuating undesired side effects of specific cancer treatments, although the directionality of effects may vary depending on the specific therapeutic.
In contrast to the potential therapeutic benefits of GCN2 inhibition or deletion in cancer, active GCN2 is required for a proper response to infection (Philips and Khan, 2021; Tomé, 2021; Zhao et al., 2023). A requirement for GCN2 in the inflammatory response to lipopolysaccharide (LPS) has been shown in vitro and in vivo (Liu et al., 2014). Under low tryptophan conditions, macrophages require active GCN2 for LPS-driven cytokine production, whereas macrophages lacking GCN2 show reduced cytokine levels upon LPS exposure. Similarly, monocytic lineage specific GCN2 knockout mice lack the ability to maximally induce cytokines in response to an LPS challenge (Liu et al., 2014).
GCN2 also has a restrictive effect against viral infections. The silencing of GCN2 results in increased HIV-1 infection and GCN2 loss of function mutant mice display increased susceptibility to viral infection (Pino et al., 2012; Won et al., 2012; Cosnefroy et al., 2013; Jaspart et al., 2017). Additionally, GCN2-deficient mice show increased viral titers in the brain after infection with Sindbis Virus (SV) (Berlanga et al., 2006). The blocking of early viral SV RNA translation by GCN2 inhibits SV replication, pointing out another important function of GCN2 in the cellular response to viruses. Upon yellow fever vaccination, activated GCN2 programs dendritic cells to promote stress granule formation and autophagy, and also increases antigen presentation to CD4+ and CD8+ T cells (Ravindran et al., 2014; Battu et al., 2017). Interestingly, RNA-sequencing data using CCD841 cells shows that GCN2 promotes the expression of angiotensin-converting enzyme 2 (ACE2), a primary receptor for SARS-CoV-2, upon leucine deprivation, while GCN2 knockdown decreases its expression (Hu et al., 2022). Thus, inhibition of GCN2 might also be an antiviral approach against specific viruses the require ACE2 for cell entry, including COVID-19. It is also important to note that GCN2 activation might be required for the survival of some parasites in the host. A parasite, Toxoplasma gondii, consumes arginine within its host cell, which in turn leads to GCN2 activation to provide additional arginine to the infected cells (Augusto et al., 2019). GCN2 deletion reduces arginine levels, suppressing parasite proliferation. These findings support the requirement for GCN2 activation to mount a proper inflammatory response upon bacterial or viral infection and also demonstrate specific viral or parasitic infections where GCN2 inhibition may be beneficial (Afroz et al., 2020).
Gcn2 is highly expressed in the hippocampus and functions in long-term memory formation, synaptic plasticity, and learning, while GCN2 knockout mice display impaired hippocampus-dependent memory and learning (Costa-Mattioli et al., 2005; Ohno, 2014). In APP/PS1 Alzheimer`s disease (AD) model mice, GCN2 deletion alleviates defects in synaptic plasticity and memory (Ma et al., 2013). Interestingly, in another AD mouse model (5XFAD), GCN2 knockout causes no improvements in memory decline, potentially due to compensatory PERK overactivation in the brains (Devi and Ohno, 2013). Considering the differences in the genetic background of model animals, the role of GCN2 in memory decline needs to be further investigated.
Some mutations or SNPs in the gene encoding human GCN2, EIF2AK4, have been associated with specific diseases. Loss of function mutations in human GCN2 or decreased GCN2 expression are robustly associated with various types of pulmonary hypertension (Eyries et al., 2014; Eichstaedt et al., 2016; Hadinnapola et al., 2017; Nossent et al., 2018; Manaud et al., 2020; Chen et al., 2021; English et al., 2022; Emanuelli et al., 2024). A genome-wide association study in a Japanese population revealed a risk allele, EIF2AK4 SNP rs2250402, associated with type 2 diabetes mellitus in humans (Yasuda et al., 2008; Kanno et al., 2020). A follow-up study revealed that this allele was associated with reduced insulin secretion, and that mice lacking GCN2 had reduced pancreatic beta cell mass when fed a high fat diet (Kanno et al., 2020). Further genomic studies are also needed in different ethnic groups to validate the generalizability of these risk variants in EIF2AK4 across populations.
GCN2 also plays an important role in critical organ function. GCN2 deletion mitigates muscle atrophy induced by denervation while its overexpression exacerbates it (Guo et al., 2018). Additionally, patients suffering from myocardial ischemia and hypoxia-reperfusion display elevated levels of GCN2 and GCN2 expression is elevated in an oxygen-glucose deprivation/reoxygenation (OGD/R) model while its knockdown decreases oxidative stress, inflammation and apoptosis in embryonic rat cardiomyocytes upon OGD/R (Pu et al., 2019; Zhang et al., 2022). GCN2 deletion or GCN2iB treatment in diabetic mice both improves cardiac function by decreasing oxidative stress and lipotoxicity (Feng et al., 2019; Yuan et al., 2022). In this case, strategies reducing the activity of GCN2 may be a therapeutic strategy in treating muscle atrophy or OGD/R in cardiac muscle. Similarly, GCN2 knockout mice had significantly lower serum urea and creatinine in hepatic and renal ischemia-reperfusion models, however they did not gain additional protection from a tryptophan-deficient diet (Peng et al., 2012). GCN2 affects the production of collagen type I by hepatic satellite cells and protects mice from liver injury and fibrosis (Arriazu et al., 2013). It also regulates hepatic gluconeogenesis, as liver-specific GCN2 KO mice show a deficiency in maintaining glucose homeostasis during fasting (Xu et al., 2013). While the role of GCN2 in disease is complex, the fine-tuning of the activity or expression level of GCN2 protein under different physiological conditions may represent a promising therapeutic approach for multiple diseases.
Discussion
Aging is a remarkably complex process characterized by dramatically increased susceptibility to a variety of diseases. GCN2 has been studied across various diseases models, including immune disorders, cancer, infection, and memory loss which are all age-associated disorders or exacerbated with aging. The involvement of GCN2 in such a wide variety of age-related diseases underscores the importance of a more comprehensive understanding of this critical nutrient-sensing kinase. Such understanding is crucial for the advancement of novel and efficient therapeutic candidates, aiming to mitigate disease symptoms and contribute to a healthier aging experience.
There are some challenges in drug development to regulate GCN2 activity with direct physical interaction. Firstly, the complete crystal structure of human GCN2 has yet to be elucidated. The 3D structure of GCN2 changes significantly as it converts from inactive to active states. As an example of the challenge this present, one of the most widely used GCN2 inhibitors was recently shown to act as a GCN2 activator at certain doses (Carlson et al., 2023). A detailed understanding of the state-specific structure of the kinase and its domains will help researchers design drugs that can specifically target GCN2, potentially even when it is in certain states, to enhance or suppress its activity. Additionally, human GCN2 has 3 isoforms. The function of each isoform, its tissue-specific functionality, and its working mechanism in different stress conditions might differ.
As the RWD domain in the N terminal region of GCN2 interacts with GCN1, targeting this domain may be an effective way to control GCN2 activation. Blocking this domain with specific small molecules might hinder its interaction with GCN1 and render the kinase inactive. Targeting GCN2 activity indirectly by regulating the function, structure, or availability of its interacting molecules may also be possible. One way to regulate GCN2 activation might be achieved through blocking the binding of ribosomal P-stalk (Harding et al., 2019; Inglis et al., 2019), for example, by targeting the C-terminus of P-stalk proteins or domain II of uL10, which were shown to be the regions that interact with GCN2 to activate it in vitro.
During periods of nutrient scarcity, GCN2 establishes a connection between nutrient sensing and longevity by globally inhibiting translation and selectively activating stress responses, preserving homeostasis. This mechanism has demonstrated a robust ability to enhance lifespan and healthspan in invertebrates. However, GCN2’s impact on aging in more complex organisms remains underexplored. Despite the uncertain role of GCN2 in core mammalian aging processes, it is clear that GCN2 plays a critical role in multiple diseases of aging in mammals and holds great promise as a therapeutic target. Further work is needed to develop therapeutic strategies that allow for the targeted modulation of GCN2 levels and activity to treat diseases of aging.
Author contributions
OA: Writing–original draft. MM: Writing–review and editing.
Funding
The authors declare that no financial support was received for the research, authorship, and/or publication of this article.
Conflict of interest
The authors declare that the research was conducted in the absence of any commercial or financial relationships that could be construed as a potential conflict of interest.
Publisher’s note
All claims expressed in this article are solely those of the authors and do not necessarily represent those of their affiliated organizations, or those of the publisher, the editors and the reviewers. Any product that may be evaluated in this article, or claim that may be made by its manufacturer, is not guaranteed or endorsed by the publisher.
References
Afroz, S., Battu, S., Giddaluru, J., and Khan, N. (2020). Dengue virus induced COX-2 signaling is regulated through nutrient sensor GCN2. Front. Immunol. 11, 1831. doi:10.3389/fimmu.2020.01831
Anda, S., Zach, R., and Grallert, B. (2017). Activation of Gcn2 in response to different stresses. PLoS One 12, e0182143. doi:10.1371/journal.pone.0182143
Arriazu, E., Ruiz De Galarreta, M., López-Zabalza, M. J., Leung, T. M., Nieto, N., and Iraburu, M. J. (2013). GCN2 kinase is a key regulator of fibrogenesis and acute and chronic liver injury induced by carbon tetrachloride in mice. Lab. Investig. 93, 303–310. doi:10.1038/labinvest.2012.173
Augusto, L., Amin, P. H., Wek, R. C., and Sullivan, W. J. (2019). Regulation of arginine transport by GCN2 eIF2 kinase is important for replication of the intracellular parasite Toxoplasma gondii. PLoS Pathog. 15, e1007746. doi:10.1371/journal.ppat.1007746
Baban, B., Chandler, P. R., Sharma, M. D., Pihkala, J., Koni, P. A., Munn, D. H., et al. (2009). Ido activates regulatory T cells and blocks their conversion into Th17-like T cells. J. Immunol. 183, 2475–2483. doi:10.4049/jimmunol.0900986
Battu, S., Minhas, G., Mishra, A., and Khan, N. (2017). Amino acid sensing via general control nonderepressible-2 kinase and immunological programming. Front. Immunol. 8, 1719. doi:10.3389/fimmu.2017.01719
B’chir, W., Maurin, A.-C., Carraro, V., Averous, J., Jousse, C., Muranishi, Y., et al. (2013). The eIF2α/ATF4 pathway is essential for stress-induced autophagy gene expression. Nucleic Acids Res. 41, 7683–7699. doi:10.1093/nar/gkt563
Berlanga, J. J., Ventoso, I., Harding, H. P., Deng, J., Ron, D., Sonenberg, N., et al. (2006). Antiviral effect of the mammalian translation initiation factor 2alpha kinase GCN2 against RNA viruses. EMBO J. 25, 1730–1740. doi:10.1038/sj.emboj.7601073
Blais, J. D., Filipenko, V., Bi, M., Harding, H. P., Ron, D., Koumenis, C., et al. (2004). Activating transcription factor 4 is translationally regulated by hypoxic stress. Mol. Cell. Biol. 24, 7469–7482. doi:10.1128/MCB.24.17.7469-7482.2004
Bunpo, P., Cundiff, J. K., Reinert, R. B., Wek, R. C., Aldrich, C. J., and Anthony, T. G. (2010). The eIF2 kinase GCN2 is essential for the murine immune system to adapt to amino acid deprivation by asparaginase. J. Nutr. 140, 2020–2027. doi:10.3945/jn.110.129197
Carlson, K. R., Georgiadis, M. M., Tameire, F., Staschke, K. A., and Wek, R. C. (2023). Activation of Gcn2 by small molecules designed to be inhibitors. J. Biol. Chem. 299, 104595. doi:10.1016/j.jbc.2023.104595
Carver, J. R., Shapiro, C. L., Ng, A., Jacobs, L., Schwartz, C., Virgo, K. S., et al. (2007). American Society of Clinical Oncology clinical evidence review on the ongoing care of adult cancer survivors: cardiac and pulmonary late effects. J. Clin. Oncol. 25, 3991–4008. doi:10.1200/JCO.2007.10.9777
Chaudhary, K., Shinde, R., Liu, H., Gnana-Prakasam, J. P., Veeranan-Karmegam, R., Huang, L., et al. (2015). Amino acid metabolism inhibits antibody-driven kidney injury by inducing autophagy. J. Immunol. 194, 5713–5724. doi:10.4049/jimmunol.1500277
Chen, Z., Zhang, J., Wei, D., Chen, J., and Yang, J. (2021). GCN2 regulates ATF3-p38 MAPK signaling transduction in pulmonary veno-occlusive disease. J. Cardiovasc Pharmacol. Ther. 26, 677–689. doi:10.1177/10742484211015535
Cherkasova, V., Qiu, H., and Hinnebusch, A. G. (2010). Snf1 promotes phosphorylation of the alpha subunit of eukaryotic translation initiation factor 2 by activating Gcn2 and inhibiting phosphatases Glc7 and Sit4. Mol. Cell. Biol. 30, 2862–2873. doi:10.1128/MCB.00183-10
Cordova, R. A., Misra, J., Amin, P. H., Klunk, A. J., Damayanti, N. P., Carlson, K. R., et al. (2022). GCN2 eIF2 kinase promotes prostate cancer by maintaining amino acid homeostasis. Elife 11, e81083. doi:10.7554/eLife.81083
Cosnefroy, O., Jaspart, A., Calmels, C., Parissi, V., Fleury, H., Ventura, M., et al. (2013). Activation of GCN2 upon HIV-1 infection and inhibition of translation. Cell. Mol. Life Sci. 70, 2411–2421. doi:10.1007/s00018-013-1272-x
Costa-Mattioli, M., Gobert, D., Harding, H., Herdy, B., Azzi, M., Bruno, M., et al. (2005). Translational control of hippocampal synaptic plasticity and memory by the eIF2alpha kinase GCN2. Nature 436, 1166–1173. doi:10.1038/nature03897
Costa-Mattioli, M., and Walter, P. (2020). The integrated stress response: from mechanism to disease. Science 368, eaat5314. doi:10.1126/science.aat5314
DeBerardinis, R. J., Lum, J. J., Hatzivassiliou, G., and Thompson, C. B. (2008). The biology of cancer: metabolic reprogramming fuels cell growth and proliferation. Cell. Metab. 7, 11–20. doi:10.1016/j.cmet.2007.10.002
Deng, J., Harding, H. P., Raught, B., Gingras, A.-C., Berlanga, J. J., Scheuner, D., et al. (2002). Activation of GCN2 in UV-irradiated cells inhibits translation. Curr. Biol. 12, 1279–1286. doi:10.1016/s0960-9822(02)01037-0
Devi, L., and Ohno, M. (2013). Deletion of the eIF2α Kinase GCN2 fails to rescue the memory decline associated with Alzheimer’s disease. PLoS One 8, e77335. doi:10.1371/journal.pone.0077335
Diallinas, G., and Thireos, G. (1994). Genetic and biochemical evidence for yeast GCN2 protein kinase polymerization. Gene 143, 21–27. doi:10.1016/0378-1119(94)90599-1
Dokládal, L., Stumpe, M., Pillet, B., Hu, Z., Osuna, G., Kressler, D., et al. (2021). Global phosphoproteomics pinpoints uncharted Gcn2-mediated mechanisms of translational control. Mol. Cell. 81, 1879–1889.e6. doi:10.1016/j.molcel.2021.02.037
Eichstaedt, C. A., Song, J., Benjamin, N., Harutyunova, S., Fischer, C., Grünig, E., et al. (2016). EIF2AK4 mutation as “second hit” in hereditary pulmonary arterial hypertension. Respir. Res. 17, 141. doi:10.1186/s12931-016-0457-x
Emanuelli, G., Zhu, J., Li, W., Morrell, N. W., and Marciniak, S. J. (2024). Functional validation of EIF2AK4 (GCN2) missense variants associated with pulmonary arterial hypertension. Hum. Mol. Genet. 33, 1495–1505. doi:10.1093/hmg/ddae082
English, A. M., Green, K. M., and Moon, S. L. (2022). A (dis)integrated stress response: genetic diseases of eIF2α regulators. Wiley Interdiscip. Rev. RNA 13, e1689. doi:10.1002/wrna.1689
Eyries, M., Montani, D., Girerd, B., Perret, C., Leroy, A., Lonjou, C., et al. (2014). EIF2AK4 mutations cause pulmonary veno-occlusive disease, a recessive form of pulmonary hypertension. Nat. Genet. 46, 65–69. doi:10.1038/ng.2844
Feng, W., Lei, T., Wang, Y., Feng, R., Yuan, J., Shen, X., et al. (2019). GCN2 deficiency ameliorates cardiac dysfunction in diabetic mice by reducing lipotoxicity and oxidative stress. Free Radic. Biol. Med. 130, 128–139. doi:10.1016/j.freeradbiomed.2018.10.445
Ferraz, R. C., Camara, H., De-Souza, E. A., Pinto, S., Pinca, A. P. F., Silva, R. C., et al. (2016). IMPACT is a GCN2 inhibitor that limits lifespan in Caenorhabditis elegans. BMC Biol. 14, 87. doi:10.1186/s12915-016-0301-2
Gallinetti, J., Harputlugil, E., and Mitchell, J. R. (2013). Amino acid sensing in dietary-restriction-mediated longevity: roles of signal-transducing kinases GCN2 and TOR. Biochem. J. 449, 1–10. doi:10.1042/BJ20121098
Galluzzi, L., Pietrocola, F., Pedro, B.-S., Amaravadi, R. K., Baehrecke, E. H., Cecconi, F., et al. (2015). Autophagy in malignant transformation and cancer progression. EMBO J. 34, 856–880. doi:10.15252/embj.201490784
Garcia-Barrio, M., Dong, J., Ufano, S., and Hinnebusch, A. G. (2000). Association of GCN1-GCN20 regulatory complex with the N-terminus of eIF2alpha kinase GCN2 is required for GCN2 activation. EMBO J. 19, 1887–1899. doi:10.1093/emboj/19.8.1887
Gárriz, A., Qiu, H., Dey, M., Seo, E.-J., Dever, T. E., and Hinnebusch, A. G. (2009). A network of hydrophobic residues impeding helix alphaC rotation maintains latency of kinase Gcn2, which phosphorylates the alpha subunit of translation initiation factor 2. Mol. Cell. Biol. 29, 1592–1607. doi:10.1128/MCB.01446-08
Gold, L. T., and Masson, G. R. (2022). GCN2: roles in tumour development and progression. Biochem. Soc. Trans. 50, 737–745. doi:10.1042/BST20211252
Guo, Y., Wang, H., Tang, Y., Wang, Y., Zhang, M., Yang, Z., et al. (2018). GCN2 deficiency protects mice from denervation-induced skeletal muscle atrophy via inhibiting FoxO3a nuclear translocation. Protein Cell. 9, 966–970. doi:10.1007/s13238-018-0504-0
Hadinnapola, C., Bleda, M., Haimel, M., Screaton, N., Swift, A., Dorfmüller, P., et al. (2017). Phenotypic characterization of EIF2AK4 mutation carriers in a large cohort of patients diagnosed clinically with pulmonary arterial hypertension. Circulation 136, 2022–2033. doi:10.1161/CIRCULATIONAHA.117.028351
Hansen, M., Taubert, S., Crawford, D., Libina, N., Lee, S.-J., and Kenyon, C. (2007). Lifespan extension by conditions that inhibit translation in Caenorhabditis elegans. Aging Cell. 6, 95–110. doi:10.1111/j.1474-9726.2006.00267.x
Harding, H. P., Ordonez, A., Allen, F., Parts, L., Inglis, A. J., Williams, R. L., et al. (2019). The ribosomal P-stalk couples amino acid starvation to GCN2 activation in mammalian cells. eLife 8, e50149. doi:10.7554/eLife.50149
He, H., Singh, I., Wek, S. A., Dey, S., Baird, T. D., Wek, R. C., et al. (2014). Crystal structures of GCN2 protein kinase C-terminal domains suggest regulatory differences in yeast and mammals. J. Biol. Chem. 289, 15023–15034. doi:10.1074/jbc.M114.560789
Hinnebusch, A. G. (1985). A hierarchy of trans-acting factors modulates translation of an activator of amino acid biosynthetic genes in Saccharomyces cerevisiae. Mol. Cell. Biol. 5, 2349–2360. doi:10.1128/mcb.5.9.2349
Holcik, M., and Sonenberg, N. (2005). Translational control in stress and apoptosis. Nat. Rev. Mol. Cell. Biol. 6, 318–327. doi:10.1038/nrm1618
Hu, X., Niu, Y., Luo, P., Xiao, F., Yuan, F., Yin, H., et al. (2022). Amino acid sensor GCN2 promotes SARS-CoV-2 receptor ACE2 expression in response to amino acid deprivation. Commun. Biol. 5, 651. doi:10.1038/s42003-022-03609-0
Hu, Z., Xia, B., Postnikoff, S. D., Shen, Z.-J., Tomoiaga, A. S., Harkness, T. A., et al. (2018). Ssd1 and Gcn2 suppress global translation efficiency in replicatively aged yeast while their activation extends lifespan. Elife 7, e35551. doi:10.7554/eLife.35551
Inglis, A. J., Masson, G. R., Shao, S., Perisic, O., McLaughlin, S. H., Hegde, R. S., et al. (2019). Activation of GCN2 by the ribosomal P-stalk. Proc. Natl. Acad. Sci. U. S. A. 116, 4946–4954. doi:10.1073/pnas.1813352116
Izquierdo, Y., Kulasekaran, S., Benito, P., López, B., Marcos, R., Cascón, T., et al. (2018). Arabidopsis nonresponding to oxylipins locus NOXY7 encodes a yeast GCN1 homolog that mediates noncanonical translation regulation and stress adaptation. Plant Cell. Environ. 41, 1438–1452. doi:10.1111/pce.13182
Jaspart, A., Calmels, C., Cosnefroy, O., Bellecave, P., Pinson, P., Claverol, S., et al. (2017). GCN2 phosphorylates HIV-1 integrase and decreases HIV-1 replication by limiting viral integration. Sci. Rep. 7, 2283. doi:10.1038/s41598-017-02276-0
Jiang, H.-Y., and Wek, R. C. (2005). GCN2 phosphorylation of eIF2alpha activates NF-kappaB in response to UV irradiation. Biochem. J. 385, 371–380. doi:10.1042/BJ20041164
Kang, M.-J., Vasudevan, D., Kang, K., Kim, K., Park, J.-E., Zhang, N., et al. (2017). 4E-BP is a target of the GCN2-ATF4 pathway during Drosophila development and aging. J. Cell. Biol. 216, 115–129. doi:10.1083/jcb.201511073
Kanno, A., Asahara, S.-I., Furubayashi, A., Masuda, K., Yoshitomi, R., Suzuki, E., et al. (2020). GCN2 regulates pancreatic β cell mass by sensing intracellular amino acid levels. JCI Insight 5, e128820. doi:10.1172/jci.insight.128820
Kim, K., Park, J.-E., Yeom, J., Park, N., Trần, T.-X., and Kang, M.-J. (2020). Tissue-specific roles of GCN2 in aging and autosomal dominant retinitis pigmentosa. Biochem. Biophys. Res. Commun. 533, 1054–1060. doi:10.1016/j.bbrc.2020.09.120
Lageix, S., Zhang, J., Rothenburg, S., and Hinnebusch, A. G. (2015). Interaction between the tRNA-binding and C-terminal domains of Yeast Gcn2 regulates kinase activity in vivo. PLoS Genet. 11, e1004991. doi:10.1371/journal.pgen.1004991
Li, W., Li, X., and Miller, R. A. (2014). ATF4 activity: a common feature shared by many kinds of slow-aging mice. Aging Cell. 13, 1012–1018. doi:10.1111/acel.12264
Liu, H., Huang, L., Bradley, J., Liu, K., Bardhan, K., Ron, D., et al. (2014). GCN2-dependent metabolic stress is essential for endotoxemic cytokine induction and pathology. Mol. Cell. Biol. 34, 428–438. doi:10.1128/MCB.00946-13
Longchamp, A., Mirabella, T., Arduini, A., MacArthur, M. R., Das, A., Treviño-Villarreal, J. H., et al. (2018). Amino acid restriction triggers angiogenesis via GCN2/ATF4 regulation of VEGF and H2S production. Cell. 173, 117–129. doi:10.1016/j.cell.2018.03.001
Lu, J., O’Hara, E. B., Trieselmann, B. A., Romano, P. R., and Dever, T. E. (1999). The interferon-induced double-stranded RNA-activated protein kinase PKR will phosphorylate serine, threonine, or tyrosine at residue 51 in eukaryotic initiation factor 2alpha. J. Biol. Chem. 274, 32198–32203. doi:10.1074/jbc.274.45.32198
Lu, Z., Xu, X., Fassett, J., Kwak, D., Liu, X., Hu, X., et al. (2014). Loss of the eukaryotic initiation factor 2α kinase general control nonderepressible 2 protects mice from pressure overload-induced congestive heart failure without affecting ventricular hypertrophy. Hypertension 63, 128–135. doi:10.1161/HYPERTENSIONAHA.113.02313
Ma, T., Trinh, M. A., Wexler, A. J., Bourbon, C., Gatti, E., Pierre, P., et al. (2013). Suppression of eIF2α kinases alleviates Alzheimer’s disease-related plasticity and memory deficits. Nat. Neurosci. 16, 1299–1305. doi:10.1038/nn.3486
Maia de Oliveira, T., Korboukh, V., Caswell, S., Holt, W., Lamb, M., Hird, A. W., et al. (2020). The structure of human GCN2 reveals a parallel, back-to-back kinase dimer with a plastic DFG activation loop motif. Biochem. J. 477, 275–284. doi:10.1042/BCJ20190196
Manaud, G., Nossent, E. J., Lambert, M., Ghigna, M.-R., Boët, A., Vinhas, M.-C., et al. (2020). Comparison of human and experimental pulmonary veno-occlusive disease. Am. J. Respir. Cell. Mol. Biol. 63, 118–131. doi:10.1165/rcmb.2019-0015OC
Marton, M. J., Crouch, D., and Hinnebusch, A. G. (1993). GCN1, a translational activator of GCN4 in Saccharomyces cerevisiae, is required for phosphorylation of eukaryotic translation initiation factor 2 by protein kinase GCN2. Mol. Cell. Biol. 13, 3541–3556. doi:10.1128/mcb.13.6.3541
Marton, M. J., Vazquez de Aldana, C. R., Qiu, H., Chakraburtty, K., and Hinnebusch, A. G. (1997). Evidence that GCN1 and GCN20, translational regulators of GCN4, function on elongating ribosomes in activation of eIF2alpha kinase GCN2. Mol. Cell. Biol. 17, 4474–4489. doi:10.1128/MCB.17.8.4474
Missiaen, R., Anderson, N. M., Kim, L. C., Nance, B., Burrows, M., Skuli, N., et al. (2022). GCN2 inhibition sensitizes arginine-deprived hepatocellular carcinoma cells to senolytic treatment. Cell. Metab. 34, 1151–1167.e7. doi:10.1016/j.cmet.2022.06.010
Munn, D. H., Sharma, M. D., Baban, B., Harding, H. P., Zhang, Y., Ron, D., et al. (2005). GCN2 kinase in T cells mediates proliferative arrest and anergy induction in response to indoleamine 2,3-dioxygenase. Immunity 22, 633–642. doi:10.1016/j.immuni.2005.03.013
Nakamura, A., Nambu, T., Ebara, S., Hasegawa, Y., Toyoshima, K., Tsuchiya, Y., et al. (2018). Inhibition of GCN2 sensitizes ASNS-low cancer cells to asparaginase by disrupting the amino acid response. Proc. Natl. Acad. Sci. U. S. A. 115, E7776–E7785. doi:10.1073/pnas.1805523115
Narasimhan, J., Staschke, K. A., and Wek, R. C. (2004). Dimerization is required for activation of eIF2 kinase Gcn2 in response to diverse environmental stress conditions. J. Biol. Chem. 279, 22820–22832. doi:10.1074/jbc.M402228200
Nossent, E. J., Antigny, F., Montani, D., Bogaard, H. J., Ghigna, M. R., Lambert, M., et al. (2018). Pulmonary vascular remodeling patterns and expression of general control nonderepressible 2 (GCN2) in pulmonary veno-occlusive disease. J. Heart Lung Transpl. 37, 647–655. doi:10.1016/j.healun.2017.09.022
Ohno, M. (2014). Roles of eIF2α kinases in the pathogenesis of Alzheimer’s disease. Front. Mol. Neurosci. 7, 22. doi:10.3389/fnmol.2014.00022
Padyana, A. K., Qiu, H., Roll-Mecak, A., Hinnebusch, A. G., and Burley, S. K. (2005). Structural basis for autoinhibition and mutational activation of eukaryotic initiation factor 2alpha protein kinase GCN2. J. Biol. Chem. 280, 29289–29299. doi:10.1074/jbc.M504096200
Pakos-Zebrucka, K., Koryga, I., Mnich, K., Ljujic, M., Samali, A., and Gorman, A. M. (2016). The integrated stress response. EMBO Rep. 17, 1374–1395. doi:10.15252/embr.201642195
Peng, W., Robertson, L., Gallinetti, J., Mejia, P., Vose, S., Charlip, A., et al. (2012). Surgical stress resistance induced by single amino acid deprivation requires Gcn2 in mice. Sci. Transl. Med. 4, 118ra11. doi:10.1126/scitranslmed.3002629
Pereira, C. M., Sattlegger, E., Jiang, H.-Y., Longo, B. M., Jaqueta, C. B., Hinnebusch, A. G., et al. (2005). IMPACT, a protein preferentially expressed in the mouse brain, binds GCN1 and inhibits GCN2 activation. J. Biol. Chem. 280, 28316–28323. doi:10.1074/jbc.M408571200
Philips, A. M., and Khan, N. (2021). Amino acid sensing pathway: a major check point in the pathogenesis of obesity and COVID-19. Obes. Rev. 22, e13221. doi:10.1111/obr.13221
Pino, J., Jiménez, J. L., Ventoso, I., Castelló, A., Muñoz-Fernández, M. Á., de Haro, C., et al. (2012). GCN2 has inhibitory effect on human immunodeficiency virus-1 protein synthesis and is cleaved upon viral infection. PLoS One 7, e47272. doi:10.1371/journal.pone.0047272
Pochopien, A. A., Beckert, B., Kasvandik, S., Berninghausen, O., Beckmann, R., Tenson, T., et al. (2021). Structure of Gcn1 bound to stalled and colliding 80S ribosomes. Proc. Natl. Acad. Sci. U. S. A. 118, e2022756118. doi:10.1073/pnas.2022756118
Pu, Y., Wu, D., Lu, X., and Yang, L. (2019). Effects of GCN2/eIF2α on myocardial ischemia/hypoxia reperfusion and myocardial cells injury. Am. J. Transl. Res. 11, 5586–5598.
Qiu, H., Dong, J., Hu, C., Francklyn, C. S., and Hinnebusch, A. G. (2001). The tRNA-binding moiety in GCN2 contains a dimerization domain that interacts with the kinase domain and is required for tRNA binding and kinase activation. EMBO J. 20, 1425–1438. doi:10.1093/emboj/20.6.1425
Ravindran, R., Khan, N., Nakaya, H. I., Li, S., Loebbermann, J., Maddur, M. S., et al. (2014). Vaccine activation of the nutrient sensor GCN2 in dendritic cells enhances antigen presentation. Science 343, 313–317. doi:10.1126/science.1246829
Ravindran, R., Loebbermann, J., Nakaya, H. I., Khan, N., Ma, H., Gama, L., et al. (2016). The amino acid sensor GCN2 controls gut inflammation by inhibiting inflammasome activation. Nature 531, 523–527. doi:10.1038/nature17186
Ravishankar, B., Liu, H., Shinde, R., Chaudhary, K., Xiao, W., Bradley, J., et al. (2015). The amino acid sensor GCN2 inhibits inflammatory responses to apoptotic cells promoting tolerance and suppressing systemic autoimmunity. Proc. Natl. Acad. Sci. U. S. A. 112, 10774–10779. doi:10.1073/pnas.1504276112
Revelo, X. S., Winer, S., and Winer, D. A. (2016). Starving intestinal inflammation with the amino acid sensor GCN2. Cell. Metab. 23, 763–765. doi:10.1016/j.cmet.2016.04.020
Romano, P. R., Garcia-Barrio, M. T., Zhang, X., Wang, Q., Taylor, D. R., Zhang, F., et al. (1998). Autophosphorylation in the activation loop is required for full kinase activity in vivo of human and yeast eukaryotic initiation factor 2alpha kinases PKR and GCN2. Mol. Cell. Biol. 18, 2282–2297. doi:10.1128/MCB.18.4.2282
Rousakis, A., Vlassis, A., Vlanti, A., Patera, S., Thireos, G., and Syntichaki, P. (2013). The general control nonderepressible-2 kinase mediates stress response and longevity induced by target of rapamycin inactivation in Caenorhabditis elegans. Aging Cell. 12, 742–751. doi:10.1111/acel.12101
Sattlegger, E., and Hinnebusch, A. G. (2000). Separate domains in GCN1 for binding protein kinase GCN2 and ribosomes are required for GCN2 activation in amino acid-starved cells. EMBO J. 19, 6622–6633. doi:10.1093/emboj/19.23.6622
Sattlegger, E., and Hinnebusch, A. G. (2005). Polyribosome binding by GCN1 is required for full activation of eukaryotic translation initiation factor 2{alpha} kinase GCN2 during amino acid starvation. J. Biol. Chem. 280, 16514–16521. doi:10.1074/jbc.M414566200
Sharma, M. D., Hou, D.-Y., Liu, Y., Koni, P. A., Metz, R., Chandler, P., et al. (2009). Indoleamine 2,3-dioxygenase controls conversion of Foxp3+ Tregs to TH17-like cells in tumor-draining lymph nodes. Blood 113, 6102–6111. doi:10.1182/blood-2008-12-195354
Sood, R., Porter, A. C., Olsen, D. A., Cavener, D. R., and Wek, R. C. (2000). A mammalian homologue of GCN2 protein kinase important for translational control by phosphorylation of eukaryotic initiation factor-2alpha. Genetics 154, 787–801. doi:10.1093/genetics/154.2.787
Statzer, C., Meng, J., Venz, R., Bland, M., Robida-Stubbs, S., Patel, K., et al. (2022). ATF-4 and hydrogen sulfide signalling mediate longevity in response to inhibition of translation or mTORC1. Nat. Commun. 13, 967. doi:10.1038/s41467-022-28599-9
Toboz, P., Amiri, M., Tabatabaei, N., Dufour, C. R., Kim, S. H., Fillebeen, C., et al. (2022). The amino acid sensor GCN2 controls red blood cell clearance and iron metabolism through regulation of liver macrophages. Proc. Natl. Acad. Sci. U. S. A. 119, e2121251119. doi:10.1073/pnas.2121251119
Tomé, D. (2021). Amino acid metabolism and signalling pathways: potential targets in the control of infection and immunity. Eur. J. Clin. Nutr. 75, 1319–1327. doi:10.1038/s41430-021-00943-0
Vazquez de Aldana, C. R., Marton, M. J., and Hinnebusch, A. G. (1995). GCN20, a novel ATP binding cassette protein, and GCN1 reside in a complex that mediates activation of the eIF-2 alpha kinase GCN2 in amino acid-starved cells. EMBO J. 14, 3184–3199. doi:10.1002/j.1460-2075.1995.tb07321.x
Wang, Y., Lei, T., Yuan, J., Wu, Y., Shen, X., Gao, J., et al. (2018). GCN2 deficiency ameliorates doxorubicin-induced cardiotoxicity by decreasing cardiomyocyte apoptosis and myocardial oxidative stress. Redox Biol. 17, 25–34. doi:10.1016/j.redox.2018.04.009
Wang, Y., Ning, Y., Alam, G. N., Jankowski, B. M., Dong, Z., Nör, J. E., et al. (2013). Amino acid deprivation promotes tumor angiogenesis through the GCN2/ATF4 pathway. Neoplasia 15, 989–997. doi:10.1593/neo.13262
Wek, S. A., Zhu, S., and Wek, R. C. (1995). The histidyl-tRNA synthetase-related sequence in the eIF-2 alpha protein kinase GCN2 interacts with tRNA and is required for activation in response to starvation for different amino acids. Mol. Cell. Biol. 15, 4497–4506. doi:10.1128/MCB.15.8.4497
Wilson, G. J., Bunpo, P., Cundiff, J. K., Wek, R. C., and Anthony, T. G. (2013). The eukaryotic initiation factor 2 kinase GCN2 protects against hepatotoxicity during asparaginase treatment. Am. J. Physiol. Endocrinol. Metab. 305, E1124–E1133. doi:10.1152/ajpendo.00080.2013
Won, S., Eidenschenk, C., Arnold, C. N., Siggs, O. M., Sun, L., Brandl, K., et al. (2012). Increased susceptibility to DNA virus infection in mice with a GCN2 mutation. J. Virol. 86, 1802–1808. doi:10.1128/JVI.05660-11
Wu, C. C.-C., Peterson, A., Zinshteyn, B., Regot, S., and Green, R. (2020). Ribosome collisions trigger general stress responses to regulate cell fate. Cell. 182, 404–416. doi:10.1016/j.cell.2020.06.006
Wu, Z., Song, L., Liu, S. Q., and Huang, D. (2013). Independent and additive effects of glutamic acid and methionine on yeast longevity. PLoS One 8, e79319. doi:10.1371/journal.pone.0079319
Xia, X., Lei, L., Qin, W., Wang, L., Zhang, G., and Hu, J. (2018). GCN2 controls the cellular checkpoint: potential target for regulating inflammation. Cell. Death Discov. 4, 20. doi:10.1038/s41420-017-0022-5
Xu, X., Hu, J., McGrath, B. C., and Cavener, D. R. (2013). GCN2 regulates the CCAAT enhancer binding protein beta and hepatic gluconeogenesis. Am. J. Physiol. Endocrinol. Metab. 305, E1007–E1017. doi:10.1152/ajpendo.00063.2013
Yasuda, K., Miyake, K., Horikawa, Y., Hara, K., Osawa, H., Furuta, H., et al. (2008). Variants in KCNQ1 are associated with susceptibility to type 2 diabetes mellitus. Nat. Genet. 40, 1092–1097. doi:10.1038/ng.207
Ye, J., Kumanova, M., Hart, L. S., Sloane, K., Zhang, H., De Panis, D. N., et al. (2010). The GCN2-ATF4 pathway is critical for tumour cell survival and proliferation in response to nutrient deprivation. EMBO J. 29, 2082–2096. doi:10.1038/emboj.2010.81
Yuan, J., Li, F., Cui, B., Gao, J., Yu, Z., and Lu, Z. (2022). Inhibition of GCN2 alleviates cardiomyopathy in type 2 diabetic mice via attenuating lipotoxicity and oxidative stress. Antioxidants (Basel) 11, 1379. doi:10.3390/antiox11071379
Zhang, F., Zeng, Q.-Y., Xu, H., Xu, A.-N., Liu, D.-J., Li, N.-Z., et al. (2021). Selective and competitive functions of the AAR and UPR pathways in stress-induced angiogenesis. Cell. Discov. 7, 98. doi:10.1038/s41421-021-00332-8
Zhang, G., Wang, X., Rothermel, B. A., Lavandero, S., and Wang, Z. V. (2022). The integrated stress response in ischemic diseases. Cell. Death Differ. 29, 750–757. doi:10.1038/s41418-021-00889-7
Keywords: GCN2, ISR, amino acids, aging, gerotherapeutic, geroprotection, cancer
Citation: Altintas O and MacArthur MR (2024) General control nonderepressible 2 (GCN2) as a therapeutic target in age-related diseases. Front. Aging 5:1447370. doi: 10.3389/fragi.2024.1447370
Received: 11 June 2024; Accepted: 28 August 2024;
Published: 10 September 2024.
Edited by:
Daniela Bakula, University of Copenhagen, DenmarkReviewed by:
Morten Scheibye-Knudsen, University of Copenhagen, DenmarkCopyright © 2024 Altintas and MacArthur. This is an open-access article distributed under the terms of the Creative Commons Attribution License (CC BY). The use, distribution or reproduction in other forums is permitted, provided the original author(s) and the copyright owner(s) are credited and that the original publication in this journal is cited, in accordance with accepted academic practice. No use, distribution or reproduction is permitted which does not comply with these terms.
*Correspondence: Michael R. MacArthur, bW05NTQ0QHByaW5jZXRvbi5lZHU=