- 1Pharmacology and Toxicology Department, Faculty of Pharmacy, Misr International University, Cairo, Egypt
- 2Department of Biomedical Sciences, College of Health Sciences, Abu Dhabi University, Abu Dhabi, United Arab Emirates
- 3Department of Biochemistry, Faculty of Pharmacy, Badr University in Cairo (BUC), Cairo, Egypt
- 4Biochemistry and Molecular Biology Department, Faculty of Pharmacy (Boys), Al-Azhar University, Nasr City, Egypt
- 5Biochemistry Department, Faculty of Pharmacy, Heliopolis University, Cairo, Egypt
- 6Department of Pharmacology, College of Medicine, University of Bisha, Bisha, Saudi Arabia
- 7Pharmacology and Toxicology Department, Faculty of Pharmacy, Badr University in Cairo (BUC), Cairo, Egypt
MicroRNAs (miRNAs) are short RNA molecules that are not involved in coding for proteins. They have a significant function in regulating gene expression after the process of transcription. Their participation in several biological processes has rendered them appealing subjects for investigating age-related disorders. Increasing data indicates that miRNAs can be influenced by dietary variables, such as macronutrients, micronutrients, trace minerals, and nutraceuticals. This review examines the influence of dietary factors and nutraceuticals on the regulation of miRNA in relation to the process of aging. We examine the present comprehension of miRNA disruption in age-related illnesses and emphasize the possibility of dietary manipulation as a means of prevention or treatment. Consolidating animal and human research is essential to validate the significance of dietary miRNA control in living organisms, despite the abundance of information already provided by several studies. This review elucidates the complex interaction among miRNAs, nutrition, and aging, offering valuable insights into promising areas for further research and potential therapies for age-related disorders.
1 Introduction
MicroRNAs (miRNAs) are a type of short noncoding RNAs that have emerged as significant actors in epigenome modulatory actions. These little RNA molecules, usually composed of 18–25 nucleotides, implement their regulatory influence by attaching to messenger RNA (mRNA) molecules, resulting in the inhibition of protein synthesis or the breakdown of mRNA (Bartel, 2004; Lauria and Iacomino, 2021). Multiple biological processes have been linked to miRNAs, including cellular differentiation, development, and disease pathogenesis (Witwer, 2015).
In the complex system of gene regulation, miRNAs are essential for cellular homeostasis maintenance and for fine-tuning gene expression (Bartel, 2018). Aging and age-related disorders are linked to disruptions in miRNA expression and function. Cardiovascular disease, neurological illness, and cancer are only a few of the age-related ailments linked to miRNA dysregulation (Quinlan et al., 2017; Kinser and Pincus, 2020). New therapeutic intervention opportunities may arise from a better understanding of miRNAs’ roles in aging and age-related diseases (ElShelmani et al., 2021a; Matai and Slack, 2023b).
The aging process is only one of several health and disease outcomes that are profoundly impacted by nutrition (Leitão et al., 2022). Nutraceuticals are bioactive substances with dietary components that have the potential to regulate miRNA expression and function, according to emerging data (Kocic et al., 2019). The gene expression patterns linked to aging and age-related disorders can be influenced by foods such as macronutrients, micronutrients, and trace minerals, which in turn can change miRNA profiles (Beckett et al., 2014; Quintanilha et al., 2017b). In addition, nutraceuticals have demonstrated potential as treatment methods for age-related diseases by altering miRNA expression (Alnuqaydan, 2020; Ghosh et al., 2021).
A comprehensive literature search was carried out between July 2023 and October 2023 to find research papers and reviews related to the involvement of Nutraceutical and miRNA-Mediated Aging Pathways. The search was restricted to English items published in the past decade. We used the following electronic medical databases: Science Direct and PubMed. The search approach included a mix of the terms “miRNAs,” “microRNAs,” “Aging,” “nutrition,” and “nutraceuticals.” Furthermore, phrases like “gene expression regulation,” “molecular mechanisms,” and “pathogenesis” were included to guarantee a thorough search. The chosen publications underwent a comprehensive assessment, and pertinent data was retrieved. The key material encompassed in the article consists of the study design, sample size, miRNA profiling methodologies, experimental models, and conclusions about miRNA dysregulation and its influence on aging etiology and therapy. The collected data were combined to recognize recurring patterns, tendencies, and areas of limited understanding in the discipline. The quality and reliability of the studies were evaluated based on known criteria tailored to each research type, such as the Newcastle-Ottawa Scale for cohort studies and the Cochrane Collaboration’s tool for randomized controlled trials.
2 miRNAs biogenic pathways and function
2.1 Canonical and non-canonical miRNA biogenesis pathways
The role of non-coding RNAs (ncRNAs), which include miRNAs, in gene regulation is substantial across all eukaryotic organisms. Research on these little but powerful regulators has increased in recent years, illuminating their extensive effects on many different biological processes, as well as their extraordinary adaptability and complex regulatory networks (Cui et al., 2019; Abd El Fattah et al., 2022; El-Sheikh et al., 2022; Li et al., 2022; Bakr et al., 2023).
DNA sequences that are referred to as miRNA genes or clusters of genes that are either exclusively or cooperatively generated as miRNA molecules are the molecular antecedents of miRNAs. On the other hand, miRNAs can be discovered in the areas of non-translated or intron genes that are responsible for the production of proteins. Hereafter, we have outlined the canonical and non-canonical processes of biogenesis (Rodriguez et al., 2004; Olena and Patton, 2010).
Within the canonical biogenesis process, the encoding of miRNA gene sequences by RNA polymerase II culminates in the primary miRNA (pri-miRNA) phase, which is the first step in the canonical pathway. The complex is responsible for transforming the pri-miRNA into the pre-miRNA, which is the precursor miRNA. The process of miRNA modification by Dicer begins with its transport to the cytoplasm via Exportin 5 (Denli et al., 2004; Newman and Hammond, 2010; Wei et al., 2021; Erturk et al., 2022). Single-stranded miRNAs that have reached maturity are responsible for directing the functional effector complex to modify complementary RNA targets (Figure 1) (Shang et al., 2023). The processing of some miRNAs will be carried out in a manner that is not deemed to be canonical. This particular area of study has been established with the support of a variety of various methods, such as transfer RNAs (tRNAs) derived miRNAs and miRtrons, in addition to small nucleolar RNAs (snoRNAs)-derived miRNAs and the independent approach that DICER has taken. The creation of these protocols allowed for the standardization of the many processes that are involved in the generation of miRNA (Ruby et al., 2007b; Babiarz et al., 2008; Ergin and Çetinkaya, 2022).
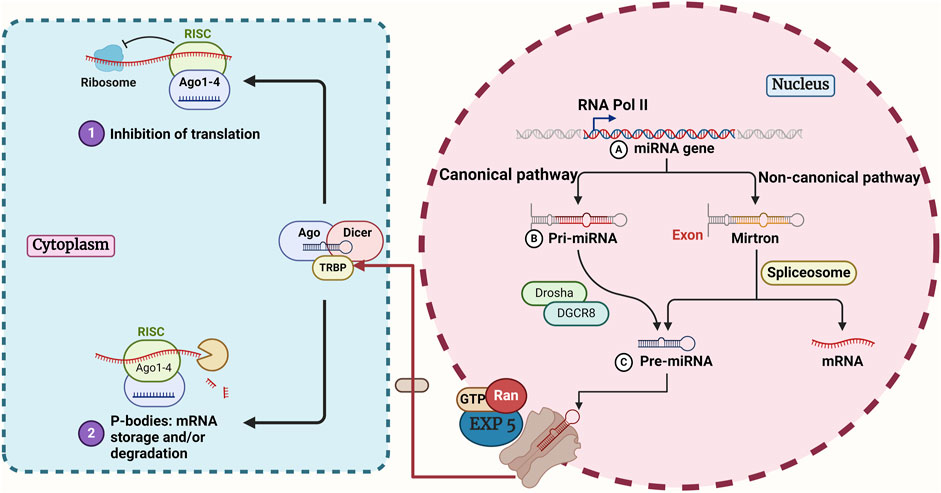
Figure 1. An overview of canonical and non-canonical pathways in miRNA biogenesis. In the canonical pathway, RNA Pol II transcribes the miRNA gene, and the microprocessor complex (Drosha and DGCR8) processes it to form a pre-miRNA. The non-canonical route is Drosha/DGCR8-independent and produces pre-miRNA directly from the entire intron. Both pathways converge as pre-miRNA is exported to the cytoplasm by EXP 5 and Ran-GTP. In the cytoplasm, Dicer processes pre-miRNA into a smaller double-stranded miRNA, which undergoes further cleavage to create a miRNA duplex. The duplex binds to Ago to form the RISC. The Ago-bound miRNA duplex unwinds, selecting either the 5p or 3p strand for the mature RISC complex. Ago, argonaute1; DGCR8, DiGeorge Critical Region 8; Dicer, an endoribonuclease enzyme that in humans is encoded by the DICER1 gene; Drosha, double-stranded RNA-specific endoribonuclease; EXP 5, Exportin-5; miRNA, microRNA; Ran, RAS-related nuclear protein; RISC, RNA-induced silencing complex; RNA Pol II, RNA polymerase II; TRBP, transactivation response element RNA-binding protein.
Transfer RNAs are an alternative source of non-canonical miRNAs. Angiogenin (ANG) or DICER uses the unique architecture of tRNAs as a substrate, cleaving the tRNA stem into fragments of tRNA-derived RNA (tDR) (Hasler et al., 2016). Also, some evidence suggests that AGO proteins can control gene expression in a manner analogous to miRNAs by loading certain tRNA segments (Abdelfattah et al., 2014). miRtrons are a kind of non-canonical pri-miRNAs that are encoded within the introns of coding genes. Like normal introns, miRtrons undergo early processing by nuclear splicing machinery, where they stabilize into hairpins with a shorter stem than conventional pri-miRNAs (Westholm and Lai, 2011). The debranching enzyme 1 (DBR1) is responsible for lariat-debranching rather than DROSHA/DGCR8 for these shorter hairpin configurations (Ruby et al., 2007a).
There is growing evidence indicating that some snoRNAs serve as a source for non-canonical miRNAs (Patterson et al., 2017). Notably, the processing of snoRNA-derived miRNAs and the stability of snoRNAs are influenced by key elements of the canonical miRNA biogenesis pathway, including DICER and DGCR8 (Langenberger et al., 2013). DGCR8, in conjunction with other proteins, could break down snoRNAs during their processing, therefore impacting the processing of miRNAs produced from snoRNAs (Macias et al., 2015). To mature, miRNAs may need the AGO2 slicer activity in a DICER-independent mode, which occurs when the stem-loop structure is too short to be cleaved by DICER (Cheloufi et al., 2010). Some of the benefits of small hairpin RNAs (shRNAs) processing that is not dependent on DICER include the ability to target genes in tumor cells that lack DICER and the preferential loading of small interfering RNAs (siRNAs) into AGO2, which improves RNAi (Herrera-Carrillo and Berkhout, 2017).
2.2 Function of miRNA
Even though miRNAs have been known for a considerable amount of time, it is only recently that their major role in an organism’s functioning has been understood. Cancer, skin issues, a broad range of lung disorders, neurological diseases, and other age-related diseases have all been the subject of extensive miRNA studies (Ugalde et al., 2011; Condrat et al., 2020; Semina et al., 2021). Evidence suggests that miRNAs can have a positive impact on a wide variety of critical biological processes. Inflammation, cell differentiation, and angiogenesis are all examples of such processes. Several complicated disorders have been linked to the improper regulation of non-coding RNAs. It is possible to affect cell function if the processing of these small but powerful regulators is disrupted (Abd El Fattah et al., 2023; El-Sheikh et al., 2023; Salman et al., 2023).
Research into complicated illnesses such as cancer, cardiovascular disease, and diabetes mellitus is facilitated by an increase in the quantity of data relevant to the interactions between miRNA and aging. These interactions apply to the process of aging. MiRNAs have also been shown to be responsible for the modulation of pathways that are involved in the sensing of nutrition (Ismail et al., 2019; Sohel, 2020; Abdelmaksoud et al., 2023).
To regulate a wide range of biological processes, miRNAs play an essential role. These regulatory molecules are likely to play a part in the etiology of inflammation and aging, and they might be utilized as therapeutic targets. There is little doubt about their involvement. Their actions are very context-dependent since miRNAs are engaged in post-transcriptional control of many messenger RNAs. Several factors impact these processes. These include the degree to which miRNAs interact with genes, the amount and affinity of miRNAs with their targets, the type of cell, the level of miRNA expression, and the concentration of miRNAs inside cells (Smith-Vikos and Slack, 2012; Quintanilha et al., 2017b; O'Brien et al., 2018). Epigenetics examines genetic control and acquired traits. Many epigenetic changes occur, including DNA methylation, histone modification, and miRNA channel silencing. (Al-Noshokaty et al., 2022; Rizk et al., 2022). The complicated mechanism that governs gene expression relies on miRNAs by the utilization of miRNA sponges, miRNA-Masking Antisense Oligonucleotides, or antisense oligonucleotides that specifically target miRNAs (AMOs) (Lima et al., 2018). In miRNA-sponge technology, mRNA molecules containing several target miRNA binding sites are expressed to act as a “sponge” or decoy for the target miRNAs (Ebert and Sharp, 2010). In other cases, instead of inhibiting the target miRNA, miR-Mask molecules shield the mRNA whose function is desired to retain. This technique is also known as BlockmiR, target protectors, or target site blockers (Wang, 2011; Beavers et al., 2015).
3 miRNAs and age-related diseases
3.1 Dysregulation of miRNAs in age-related diseases
One major risk factor for chronic illnesses in the elderly is aging. By attaching base pairs to their target mRNAs, miRNAs control the silencing of genes that occur post-transcription. Upon analyzing whole blood from healthy adults, recent studies found nonlinear variations in age-related miRNAs, with age having a greater influence than sex. In healthy aging, a transition in miRNAs to their 5′mature form was proved. With distinct disease biomarker sets for young and elderly patients, the inclusion of ill individuals highlighted pan-disease and disease-specific modifications in aging profiles (Meder et al., 2014; Fehlmann et al., 2020).
The altered expression of aging miRNA across various human and animal tissues has been confirmed through multiple studies (Smith-Vikos and Slack, 2012). Alterations in the expression of proteins, mRNA, and miRNA associated with aging and growth in the prefrontal cortex of rhesus macaques and humans have been noticed throughout their lives (Somel et al., 2010). Similar modifications in miRNA levels have been described in human skeletal muscle with aging (Drummond et al., 2011), as well as in bodily fluids like serum (Zhang H. et al., 2015). This lays the groundwork for recognizing the connection between disease and healthy aging as well as the creation of disease biomarkers particular to a person’s age (Table 1).
Extracellular vesicles (EV) are considered fragments of dead or senescent cells secreted upon physiological or pathological processes (Misawa et al., 2020). EVs are used to send signals for exchanging information among cells via a complex packet of lipids, and proteins rich in ncRNAs and miRNAs. They are also known as exosomes or microvesicles and have an important role in aging (Tkach and Théry, 2016; Cooks et al., 2018).
3.2 Role of miRNAs in different age-related diseases
Our current knowledge of the significant role miRNAs perform in age-related disorders is not sufficiently comprehensive due to the varied nature of intracellular and extracellular miRNAs. Nonetheless, interesting research has been done that will help us grasp the fundamental causes of these diseases. Herein, we will discuss a growing number of publications about particular miRNAs and their involvement in different age-related disorders.
3.2.1 miRNAs in Alzheimer’s disease
A fundamental neurodegenerative process linked to aging in individuals with Alzheimer’s, dementia, Huntington’s, and Parkinson’s disease patients is cognitive loss. Noncoding miRNAs have a major role in CNS, which has led to the discovery of promising novel clinical prospects for these illnesses, which lack current effective therapies (Abdelmaksoud et al., 2024).
The most frequent cause of dementia is Alzheimer’s disease (AD), which is characterized by a gradual degeneration of neurons and cognitive abilities (Bandakinda and Mishra, 2023). The let-7 miRNA family has been shown to have pro-apoptotic properties in the central nervous system and to control the proliferation and differentiation of neural stem cells. Numerous investigations have demonstrated that aberrant let-7 miRNA activity may contribute to the disease linked to dementia and cognitive decline via important neuronal signaling pathways (Fairchild et al., 2019). Sorensen et al. examined the blood and CSF of people suffering from AD and other forms of dementia in a different investigation (Sørensen et al., 2016). When comparing AD patients to healthy controls, they found 52 miRNAs in the CSF of nearly all patients, of which two (let-7i-5p and miR-15a-5p) were elevated and one (miR-29c-3p) was downregulated (Poursaei et al., 2022).
Through the G protein-coupled receptor BAI3, miR-142-5p stimulates neuronal synaptotoxicity and prevents apoptosis; its downregulation in the brain of an AD animal model may increase BAI3 expression. In cultured neurons, inhibition of miR-142-5p restores spatial learning and memory (Fu et al., 2021). Furthermore, Blood samples from AD patients show dysregulation of miR-122-5p, miR-210-3p, and miR-590-5p expression in comparison to healthy controls. Moreover, plasma levels of miR-342-5p may be able to predict the rate of cognitive deterioration in AD (Dakterzada et al., 2021; Mankhong et al., 2022). These results imply that dysregulated miRNAs may be a reflection of neuropathological outcomes in AD patients (Figure 2).
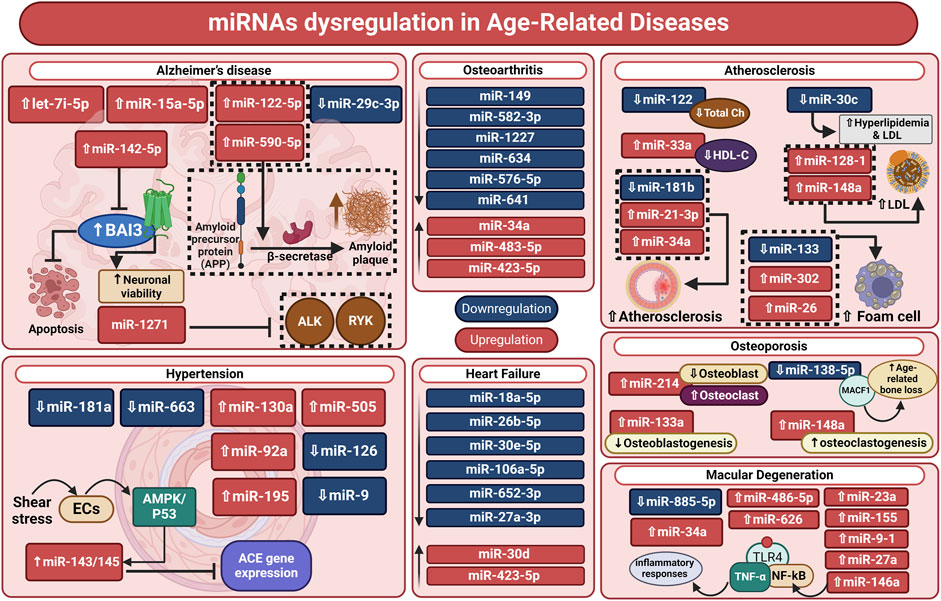
Figure 2. miRNA dysregulation in age-related diseases. Neurodegenerative and age-related disorders, including Alzheimer’s disease, cardiovascular conditions (hypertension, heart failure, and atherosclerosis), osteoarthritis, osteoporosis, and macular degeneration, are strongly associated with miRNA dysregulation. MicroRNAs are involved in disease etiology and may be biomarkers and therapeutic targets. Furthermore, miRNA dysregulation is crucial to understanding age-related diseases’ biological processes as well as personalized therapy. ACE: angiotensin-converting enzyme; ALK, anaplastic lymphoma kinase; AMPKs: AMP-activated protein kinases; BAI3, brain angiogenesis Inhibitor-3; ch, cholesterol; ECs, endothelial cells; HDL-C, high-density lipoprotein cholesterol; LDL, low-density lipoprotein; MACF1, Microtubule Actin Crosslinking Factor 1; miRNA, microRNA; NF-κB: nuclear factor kappa beta; RYK, receptor-like tyrosine kinase; TLR4: Toll-like receptor 4.
Insulin resistance is a major risk factor for Type 2 Diabetes and AD, two of the biggest global health concerns in aging, which have a similar reaction to the regulation of miR-1271 (Arnold et al., 2018). Anaplastic lymphoma kinase (ALK) and Receptor-Like Tyrosine Kinase (RYK), two non-canonical receptor tyrosine kinases (RTKs), have been shown to target miR-1271 in post-mortem AD and Type 2 diabetes tissues. miR-1271 caused the downregulation of both ALK and RYK, which were found to be in charge of the cytoskeleton structural degeneration in both AD and Type 2 diabetes mice (Majumder et al., 2021).
Moreover, exosomes derived from serum and cerebrospinal fluid can be used for miRNA profiling in neurodegenerative diseases such as AD. Many forms of dementia can be distinguished by the degree of expression of miR-193b, miR-135a, and miR-384 in serum and exosomes (Ting et al., 2018; Liu et al., 2021). Furthermore, the ongoing association seen between dysregulated miRNAs, namely miR-132 and miR-212, and cognitive decline in AD patients could lead to advancements in the clinical management of such individuals (Cha et al., 2019).
3.2.2 miRNAs in cardiovascular diseases
Elderly people are more likely to experience cardiovascular troubles due to their aging and fragility, even with advancements in detection and treatment. Over time, age-associated cardiovascular diseases (CVDs) such as atherosclerosis, heart failure, and hypertension can lead to a reduction in an individual’s standard of life and capacity to carry out routine tasks. Important modulators of many biological processes, miRNAs offer an intriguing prospective treatment target for CVDs. Furthermore, identifying trustworthy biomarkers for diagnosis, prognosis, and treatment response prediction is a significant challenge in the field of geriatric medicine. Because of their distinctive hallmark in CVDs and longevity in blood circulation, miRNAs are a very promising approach (Figure 2) (de Lucia et al., 2017).
3.2.2.1 miRNAs in hypertension
One of the most frequent diseases and a major risk factor for renal failure and cardiovascular illnesses is hypertension. Presently, only half of people with hypertension respond to treatment. A vital role in the pathophysiology of hypertension is played by circulating miRNAs, which are becoming useful biomarkers in essential hypertension and are inherently associated with every element of the renin-angiotensin-aldosterone system (Klimczak et al., 2017).
It has been demonstrated that miR-181a and miR-663 directly bind renin mRNA, and their levels were markedly decreased in hypertension individuals (Marques et al., 2011). The miR-143/145 cluster targets the angiotensin-converting enzyme, and it was activated by shear stress through the AMPK-p53 pathway, which decreased the expression of the ACE gene (Kohlstedt et al., 2013). Furthermore, loss of miR-143/145 resulted in overexpression of the Angiotensin-converting enzyme and a change in the phenotype of vascular smooth muscles from contractile to synthetic, which maintains the vascular remodeling that occurs during hypertension and raises the risk of developing neointimal lesions (Boettger et al., 2009). Two investigations additionally demonstrated that miR-21 and mir-221/222 are regulators of aberrant proliferation of vascular smooth muscles and neointimal hyperplasia in rats using a model of balloon-induced vascular wall injury (Chistiakov et al., 2015; Zhang et al., 2016).
Through a comparative analysis of the miRNA expression levels in hypertensive and control patients, it was shown that the human cytomegalovirus-encoded miRNA hcmv-miR-UL112 was upregulated in the diseased patients. This suggests this miRNA may have a role in controlling blood pressure (Li et al., 2011). Using a similar methodology, Yang et al. discovered elevated levels of miR-505 in hypertension after screening plasma samples from three separate patient cohorts (Yang Q. et al., 2014). Another investigation was carried out to find the miRNA signature in exosomes and peripheral blood taken from individuals who showed metabolic risk of CVDs. MiR-130a and −195 correlate positively with blood pressure levels, and are upregulated in hypertension. Likewise, miR-92 was upregulated in cardiovascular and metabolic disorders (Karolina et al., 2012). Furthermore, a study comparing hypertension patients to healthy controls revealed reduced expression levels of miR-9 and miR-126 (Figure 2) (Kontaraki et al., 2014).
3.2.2.2 miRNAs in heart failure
Circulating miRNAs of a cardiac origin mirror alterations in miRNAs seen in cardiac tissue as people age (Vegter et al., 2016). When compared to healthy controls who were at a higher risk of dying, miR-18a-5p, −26b-5p, −27a-3p, −30e-5p, −106a-5p, −199a-3p, −652-3p, and −199a-3p were considerably lower in older individuals suffering acute heart failure (Ovchinnikova et al., 2016). Circulating miR-21, miR-214, and miR-27b were found to be substantially upregulated in ischemia patients, but 13 different miRNAs were downregulated, according to metanalysis done in individuals with heart failure (Gholaminejad et al., 2021). Furtherly, Qiang et al. discovered that miR-126 and −508-5p could be used to predict death in individuals with heart failure (Qiang et al., 2013).
According to a separate investigation, individuals with heart failure-related dyspnea had considerably higher levels of miR-423-5p (Ellis et al., 2013). Based on a translational pilot investigation, miR-30d expression was upregulated as a defense mechanism in cardiac desynchrony zones. Additionally, there is a correlation between the responsiveness to resynchronization therapy and the initial plasma concentration of this miRNA (Melman et al., 2015). Upregulated miR-155 was linked to a favorable outcome from left ventricular assist device implantation, an essential therapy for those suffering from end-stage heart failure (Figure 2) (Wang T. et al., 2017).
3.2.2.3 miRNAs in atherosclerosis
Atherosclerosis is a multi-stage inflammatory disease of the arterial wall that is distinguished by endothelial dysfunction and lipoprotein accumulation, causing gradual remodeling of the artery intima. As the lesion ages, it becomes more advanced and eventually leads to the development of atherosclerotic plaque, which obstructs blood vessels and causes thrombotic episodes. Recent research has yielded new molecular insights into the regulation and clinical diagnosis of atherosclerosis and the corresponding miRNAs in aging related manner (Sharma et al., 2022).
Lipid profile dysregulation is regarded as a primary risk factor for atherosclerosis. miRNAs that can control the production of LDL and HDL reveal a potential therapeutic target for this disease. According to in vivo research, mice and non-human primates with miR-122 suppression had lower plasma levels of total cholesterol (Huang et al., 2022). In mice fed a high-fat diet, hepatic upregulation of miR-30c reduced hyperlipidemia and LDL production, and lessened lesions of atherosclerosis, while miR-33a decreased HDL-C in postprandial lipemia (Yaman et al., 2021). Numerous miRNAs were also shown to be connected with dyslipidemia when they were located close to loci for single-nucleotide polymorphisms in a genome-wide association investigation. Among these, the expression of many proteins involved in lipid metabolism and trafficking is regulated by miR-128-1, miR-148a, miR-130b, and miR-301b (Figure 2) (Wagschal et al., 2015).
MiR-148a and miR-128-1 inhibition increased LDL clearance in C57BL/6J mice and altered the amounts of lipoproteins in circulation in ApoE−/− mice given a western diet (Santos et al., 2021). Furthermore, in both mice and monkeys, suppression of miR-33 raised the amounts of HDL cholesterol in the bloodstream. Anti-miRNA-treated animals displayed decreased atherosclerotic lesion size and were able to infiltrate plaque macrophages and reduce the expression of inflammatory genes (Rayner et al., 2011; Goedeke et al., 2014).
The fact that atherosclerosis is a chronic inflammatory condition was the focus of numerous investigations. Data has demonstrated that miR-181b is a key modulator of inflammation in the endothelium. MiR-181b decline might contribute to the formation of atherosclerosis, as excessive expression of this miRNA managed to hinder leukocyte recruitment at the atherosclerotic plaque both in vitro, as well as in the plasma of patients suffering from coronary artery disease (Sun et al., 2014). By using a nanoparticle approach, Ma et al., 2016 have devised a procedure to deliver miR-146a and miR-181b directly to the atherogenic lesion, allowing them to decrease the size of atherosclerotic plaque and downregulate the production of chemokines. Through targeting genes involved in cholesterol metabolism, miR-27a/b also regulates the homeostasis of macrophages within the plaque (Zhang et al., 2014). A recent study demonstrated the pivotal function that miR-34a plays in controlling inflammation, atherosclerosis, and macrophage cholesterol export, in which reversing diet-induced metabolic problems and promoting atherosclerosis regression are achieved with therapeutic suppression of miR-34a (Xu et al., 2020).
MiR-133 has been found by Gabunia et al., 2017 to be a regulator of the production of foam cells in vascular smooth muscle, which lowers the proliferation and uptake of oxidized LDL by these cells. As a result, miR-133 might be a useful target for treating inflammatory vascular disorders. Further, it has been demonstrated that other miRNAs, such as miR-302 and miR-26, promote the development of foam cells (Johnson, 2019). Additionally, by promoting vascular smooth muscle migration and proliferation, exosomal miR-21-3p from nicotine-treated macrophages may hasten the onset of atherosclerosis (Zhu et al., 2019).
Additionally, miR-33 was discovered to be an essential regulator of lipoprotein metabolism and cellular lipid homeostasis. It also controls downstream target genes, such as ATP-binding cassette transporter G1 (ABCA1 and ABCG1). The preventive effects of miR-33 loss on the progression of atherosclerosis are due to its actions on macrophages. Through the activation of ABCA1 and ABCG1 in macrophages, therapeutic suppression of miR-33 in mice and nonhuman primates increases HDL levels and prevents the advancement of atherosclerosis by either boosting HDL or improving cholesterol outflow (Zhang et al., 2022). Furthermore, there are variations in the distribution and control of the miR-33 family, especially the advancement of atherosclerosis; miR-33b is thought to be more effective than miR-33a. Indeed, the mice deficient in apolipoprotein E/miR-33a−/−/miR-33b+/+ developed higher levels of atherosclerotic plaque when fed a diet high in fat and cholesterol than the mice deficient in apolipoprotein E/miR-33a+/+/miR-33b−/−, which was consistent with the liver’s prevalent levels of miR-33b and a deteriorated lipid profile (Koyama et al., 2019).
Early detection of susceptible carotid plaques may be useful in identifying high-risk stroke patients who could benefit from revascularization sooner rather than later. Atherosclerotic plaque progress biomarker miR-200c might be clinically helpful in identifying patients who are at high risk of embolism (Magenta et al., 2018). Endothelial dysfunction is more likely to occur in situations when oxidative stress is elevated, such as ischemia and aging. Recently, the relationship between miR-200c and sirtuin 1 (SIRT1), endothelial nitric oxide synthase (eNOS), and forkhead box O1 (FOXO1), three closely related proteins that regulate endothelial cell function and ROS production was examined. Through a decrease in NO and an increase in the acetylation of SIRT1 targets, FOXO1, and p53, miR-200c directly targets these proteins. Acetylation of FOXO1 reduced its transcriptional impact on target genes, namely, SIRT1, catalase, manganese superoxide dismutase, and ROS scavengers. Consequently, miR-200c strengthened this molecular circuitry by upregulating ROS and suppressing FOXO1 transcription. Furthermore, anti-miR-200c therapy restored limb perfusion and saved these targets in the mouse model of hindlimb ischemia (Carlomosti et al., 2017).
Circulatory miRNAs are now known as a novel class of atherosclerosis biomarkers, which surfaced to help with clinical diagnosis and open up new treatment options. A study was performed on candidate tissue-derived miRNAs from atherosclerotic plaque in individuals with stable and unstable coronary artery disease. MiR-125b-5p and miR-193b-3p were boosted in individuals with stable coronary artery disease, while miR-223-3p and miR-142-3p were upsurged in those with unstable type, suggesting that these candidate tissue-derived miRNAs could be markers of plaque instability (Singh et al., 2020).
3.2.3 miRNAs in bone and cartilage age-related conditions
Based on the available research, it is clear that miRNAs are strongly involved in the regulation of musculoskeletal system disorders and age-related events. Chiefly in frailty, miRNAs have been found to have an impact on the start and progression of age-related musculoskeletal diseases, including osteoporosis and osteoarthritis (Castanheira et al., 2021).
3.2.3.1 miRNAs in osteoarthritis
Numerous investigations have exhibited the significance of miRNAs in the process of cartilage formation, preservation, and degradation (Vonk et al., 2014). Using chondrocytes derived from osteoarthritis patients, the cells of donors with osteoarthritis showed higher levels of miR-483-5p. On the other hand, through affecting pathways linked to cartilage function, such as SIRT1, miR-149, −582-3p, −1227, −634, -576-5p, and −641 were downregulated in osteoarthritis (Díaz-Prado et al., 2012). Since suppressing miR-34a in an osteoarthritis rat model reduced cartilage breakdown, elevated levels of miR-34a are thought to contribute to osteoarthritis (Tao et al., 2020). Overexpression of miR-146a was also found to have a beneficial effect on cartilage preservation in an osteoarthritis animal model, while its downregulation hinders apoptosis and augments autophagy of chondrocytes (Zhang et al., 2021).
A meta-analysis was conducted recently to identify miRNAs that exhibit abnormal expression as osteoarthritis progresses in the elderly. According to the study, miR-146a-5p and miR-34a-5p were the most elevated miRNAs, while miR-127-5p and miR-140-5p were the most suppressed miRNAs. In osteoarthritis, mesenchymal stem cells and transforming growth factor-β were discovered to be the primary downstream effectors regulated by these miRNAs (Liu et al., 2023).
An efficient drug delivery method was recently created to improve miR-124 stability and its ability to reach chondrocytes, which is thought to be a strong target for its anti-inflammatory properties. By reducing chondrocyte apoptotic cascade, repressing extracellular matrix breakdown, and improving synovial thickness, the tetrahedral framework nucleic acids launching miR-124 efficiently prevent osteoarthritis from progressing and effectively preserve articular cartilage (Shi et al., 2023).
In another recent investigation on cartilage aging and osteoarthritis, multiple miRNAs were found to be differently expressed in youthful intact compared to osteoarthritic lesioned cartilage. After IL-1β treatment, the expression of miR-107, miR-143-3p, miR-361-5p, and miR-379-5p were declined in human primary chondrocytes. Additionally, the study demonstrates the significance of miR-107 and miR-143-3p in controlling protein translation, proliferation, and hypertrophy in chondrocytes (Figure 2) (Balaskas et al., 2023).
3.2.3.2 miRNAs in osteoporosis
It has also been demonstrated that miRNAs have a significant role in other age-related illnesses like osteoporosis and osteopenia. For instance, miR-133a inhibits osteoblastogenesis and may be a possible biomarker because osteoporosis patients have greater levels of miR-133a than do postmenopausal women in good health (Li Z. et al., 2018). Recently, miR-214 has been considered a potential marker of osteoporosis as its expression has a negative correlation with recognized markers of bone production (Sadu et al., 2023). Furthermore, in the femoral condyles of osteoporotic rats, anti-miRNA-214 increased osteoblast activity and decreased osteoclast activity, enhancing bone metabolism and delaying the onset of osteoporosis (Wang et al., 2023). It has been shown that miR-138-5p targets microtubule actin cross-linking factor 1 to control the differentiation of aged osteoblasts. Ultimately, the reduction in bone production and age-related bone loss in elderly mice was compensated by the therapeutic suppression of miR-138-5p (Chen et al., 2022). Additionally, by removing Forkhead box O1, miR-182 inhibits the osteogenesis of bone-forming osteoblasts (Chen et al., 2019). However, decreasing miR-148a expression in vivo resulted in reduced osteoclast formation and elevated bone mass in ovariectomized and control animals. Meanwhile, MiR-148a was demonstrated to promote osteoclastogenesis in vitro (Figure 2) (Tian et al., 2021; Pan et al., 2022).
3.2.4 miRNAs in macular degeneration
One of the leading causes of blindness in the world is age-related macular degeneration or AMD. Recent research indicates that epigenetic mechanisms, such as the regulation of gene expression by miRNAs, may be significant to AMD in addition to environmental and genetic variables, offering a promising new direction for therapy and research (Berber et al., 2017). According to a recent investigation, miR-885-5p was considerably downregulated in the serum of AMD patients, while miR-486-5p and miR-626 were more expressed than in the control group. The apoptotic and neovascularization pathways, which are involved in the pathophysiology of AMD, are known to depend critically on these miRNAs (Elbay et al., 2019). Other involved pathways in the pathophysiology of AMD include amyloid-β retinal deposition, which triggers apoptotic and inflammatory responses in addition to varying miRNA expression, thus causing dysregulation of the transforming growth factor-β pathway. Furthermore, it has been demonstrated that miR-155 and miR-27a impact 42 genes implicated in the transforming growth factor-β route, while miR-146a can target genes involved in inflammatory pathways, including nuclear factor-κB, tumor necrosis factor signaling pathways, and Toll-like receptors (Romano et al., 2017).
Additionally, IL-2, STAT3, and ERK were found to be a novel global pathway with activation hallmarks of AMD that are implicated in the cell-based inflammatory response (Makarev et al., 2014). Four miRNAs (miRNA-9, miRNA-125b, miRNA-146a, and miRNA-155) were shown to be increased in AD and AMD, according to the most recent research. The expression of complement factor H, a key inhibitor of the innate immunological and inflammatory response that is implicated in both AMD and AD, is subsequently downregulated because of these increased miRNAs. It has been shown that there are many increased miRNAs in the retina of AMD patients, which are also common in complement factor H deficiency, resulting in inflammatory neurodegeneration. MiRNA-7, miRNA-9-1, miRNA-23a/miRNA-27a, miRNA-34a, miRNA-125b-1, miRNA-146a, and miRNA-155 are highly expressed in the retinal macular region afflicted by AMD and the AD-affected superior temporal lobe neocortex (Figure 2) (Hill et al., 2015; Pogue and Lukiw, 2018).
Treatment targets for AMD are thought to include several miRNAs implicated in either retinal pigment epithelium atrophy or choroidal neovascularization (Urbańska et al., 2022). For neovascular AMD, anti-vascular endothelial growth factor therapy is a crucial treatment (Cornel et al., 2015). It has been established that a certain subset of miRNAs is crucial to angiogenesis. The miR-15/107 group, the miR-17∼92 cluster, miR-21, miR-132, miR-296, miR-378, and miR-519c are some of these miRNAs. It is thought that miR-23a mimics reduce macular related-apoptosis whereas miR-23a inhibition increases it (Ren et al., 2017; Urbańska et al., 2022).
The pathogenic significance of the newly detected miR-19a, miR-126, and miR-410 in AMD was determined by another recent bioinformatics technique. There was a substantial link found between AMD pathogenesis and these miRNAs, and their target genes. Because of this, they might serve as promising new targets for AMD patients’ treatments or prognostic biomarkers (ElShelmani et al., 2020). Using exosomes produced from AMD, the study also explained the functional roles of miRNAs in in vitro human cell line models. The findings show that, when compared to the control group, the expression of human apoptotic miRNAs is more impacted by dry AMD-derived exosomes than by wet-derived ones. After being exposed to dry AMD-derived exosomes, the used cell line expressed greater levels of let-7a-5p, miRNA-17-5p, miRNA195-5p, miRNA26b-5p, and miRNA-30c-5p (ElShelmani et al., 2021b).
3.3 Potential implication of miRNA in clinical trials to enhance longevity
With the first FDA-approved small RNA drugs entering clinical medicine, continuous research for the microRNA (miRNA) class of small RNAs has expanded its preclinical and clinical research application. The growing evidence from the huge number of reports indicates that miRNAs could be put to significant utility as biomarkers for pathogenic conditions, modulators of drug resistance, and/or drugs for medical intervention in almost all human health conditions (Hanna et al., 2019). In a study involving 16 non-Hispanic men aged between 50 and 60, whose lifespans ranged from 58 to 92 years, PCR arrays were used to track changes in miRNA levels in their serum over time. Notably, variations in the expression of these miRNAs were observed. Specifically, at the age of 50, 24 miRNAs were found to be significantly more active, while 73 showed reduced activity in those who lived longer (76–92 years), compared to those who had shorter lifespans (58–75 years). For the group that lived longer, miR-373-5p showed the highest increase in activity, whereas miR-15b-5p was the most decreased. Over the years, a strong correlation between the lifespan and the activity levels of nine specific miRNAs was observed, with significant changes noted in six of these (miR-211-5p, 374a-5p, 340-3p, 376c-3p, 5095, 1225-3p) when comparing the longer-lived to the shorter-lived participants. These six miRNAs affect 24 proteins linked to aging, such as PARP1, insulin-like growth factor-1 receptor (IGF1R), and IGF2R. Based on the findings, activity patterns of these six miRNAs are suggested to be valuable indicators of aging (Smith-Vikos et al., 2016). This suggests that a single miRNA candidate could have the ability to control whole biological pathways related to aging, thereby promoting longevity.
Despite significant advancements in preclinical studies, miRNA-based therapies are still in the preliminary phase of development. A limited number have advanced to clinical trials, with none making it to phase III or receiving approval from the FDA. Additionally, some have been discontinued due to concerns about toxicity. These obstacles underscore the need to overcome current obstacles to fully realize the potential of miRNA-based treatments in clinical settings (Seyhan, 2024). Currently, no miRNAs are undergoing clinical trials for longevity enhancement. However, the promise of miRNAs as treatments for a range of diseases is evident, and further research is crucial to assess their practicality in clinical environments.
4 Impact of nutritional factors and nutraceuticals on miRNA modulation
This review will discuss recent studies investigating the impact of nutritional factors and nutraceuticals on aging, which affect miRNA functions or expression. The interrelation between nutrition and age-related diseases has been studied for years as malnutrition, cachexia, or weight loss are related to cancer or other diseases (Witwer, 2012).
4.1 Impact of macronutrients on miRNA expression and function in aging
Macromolecules such as carbohydrates, fatty acids, and amino acids can protect or induce aging through miRNA modulation. Beta cell dysfunction is associated with overexpressed miR-29a in beta cells of Langerhans supplemented by high glucose media (Bagge et al., 2012). Moreover, miR-29a is also induced by saturated fatty acids repressing insulin receptor signaling −1 (IRS-1) and increasing insulin resistance in myocytes (Yang et al., 2014c) (Table 2). A high-fat diet is shown to increase the expression of miR-21 which promotes liver carcinogenesis (Heng et al., 2016). In addition, a high-fat diet could overexpress miR-195 impairing insulin sensitivity and glycogen metabolism in the liver (Yang et al., 2014d). Moreover, a high-fat diet is involved in the pathogenesis of atherosclerosis through upregulation of miR-210 inducing endothelial cell apoptosis (Li et al., 2017).
Hyperglycemia also induces the expression of miR-21 reducing the tumor suppressor protein phosphatase and tensin homolog deleted in chromosome 10 (PTEN) mediating the pathogenesis of nephropathy in diabetic patients (Dey et al., 2011). While protein is important for kidney health, low maternal protein intake accelerates epithelial-mesenchymal transition in the later adult life of the offspring through increasing expression of miR-192 and miR-200 (Sene et al., 2018). Xylobiose intake as an alternative to sucrose and present in bamboo can be favorable for diabetic patients as it regulates hepatic lipogenesis by overexpressing miR-122a/33a resulting in reducing triglyceride levels and inflammatory cytokines (Lim et al., 2016).
4.2 Influence of micronutrients and trace minerals on miRNA regulation
Non-optimum levels of micronutrients such as selenium, zinc, and vitamin D generate several aging-related diseases (McCann and Ames, 2011; Prasad, 2012; Sinha et al., 2013). They are included in miRNA modulation being included in their structure. Selenium is one of the micronutrients that has gained attention recently, due to its incorporation into selenoproteins such as glutathione peroxidase or other antioxidant enzymes (Bellinger et al., 2009). An in vitro experiment utilizing colorectal cancer cell lines in a selenium-deficient environment results in a changed expression of miR-185 with a feedback mechanism regulating selenoproteins as glutathione peroxidase 2 (Maciel-Dominguez et al., 2013). In selenium-deficient rats, miR-374 is overexpressed leading to a dysregulated Wnt signaling pathway and cardiac dysfunction (Xing et al., 2015). Moreover, combining resveratrol with selenium nanoparticles enhanced the effectiveness of resveratrol against Alzheimer’s disease reducing neuroinflammation through downregulating miR-134 (Abozaid et al., 2022). Vitamin D deficiency in diet upregulates miR-148a and miR-122-5p and it is related to bone loss and eventually osteoporosis in post-menopausal women (Al-Rawaf et al., 2021). Moreover, zinc deficiency is linked to an increased risk of esophageal cancer due to overexpression of miR-223, miR-21, and miR-31 (Fong et al., 2016).
Vitamin B12 and folic acid are essential for several biological processes and are also important for maternal nutrition for the offspring’s health. Imbalanced nutritional levels in maternity are linked to insulin resistance and type 2 diabetes mellitus in the offspring’s adult life through upregulating miR-483 which limits fats in the adipose tissue (Figure 3) (Mahajan et al., 2019).
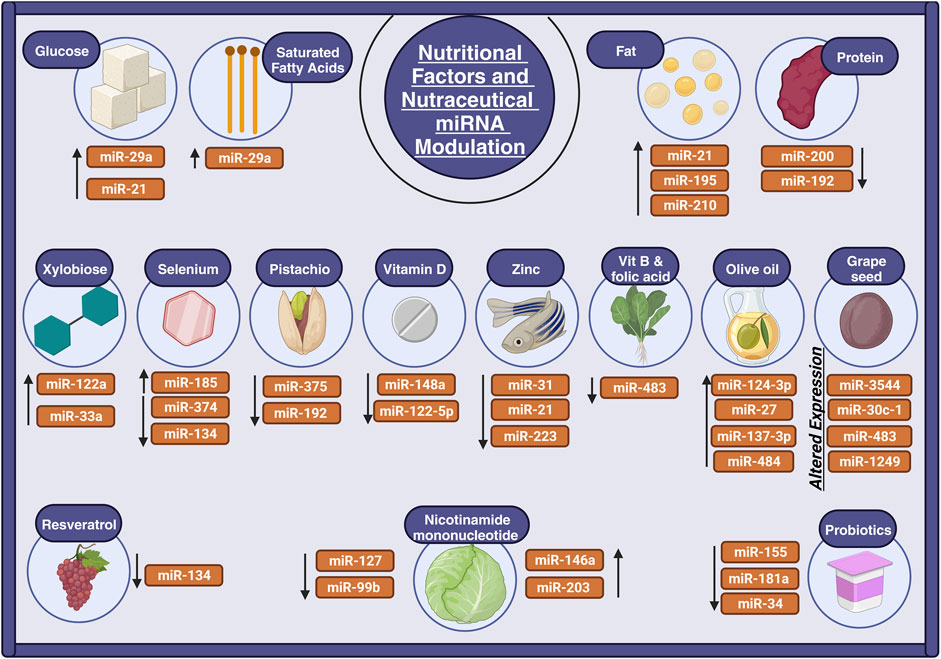
Figure 3. Nutritional factors and nutraceuticals’ role in miRNA regulation. The illustration depicts how nutrients and nutraceuticals modulate miRNAs in aging processes. MiRNA expression is influenced by macronutrients such as carbohydrates and fatty acids, as well as micronutrients like selenium and zinc, which impact aging and age-related diseases. Flavonoids and polyphenols in olive oil and grape seeds influence miRNA function in the fight against aging. Nutraceuticals, such as pistachios and probiotics, also affect miRNA expression, suggesting promising treatments for age-related diseases.
4.3 Modulation of miRNAs by flavonoids and polyphenols
Phenolic compounds or flavonoids act as anti-aging agents by regulating miRNA function. Olive oil rich in phenols intake in middle age increased the miRNA expression of miR-484, miR-137-3p, miR-27, and miR-124-3p resulting in reduced brain plasticity and improving neurological and behavioral function (Luceri et al., 2017a). Another study investigated the impact of grape seed intake rich in procyanidin on diabetic rats, it was found that the flavonoids of grape seed modulate glucose metabolism and improve pancreatic function through downregulating the expression of miR-1249, miR-483, miR-30c-1, and upregulating miR-3544 expression (Figure 3) (Castell-Auví et al., 2013).
4.4 Other nutraceuticals and their effects on miRNA expression
Chronic pistachio intake, rich in unsaturated fat, minerals, and vitamins, modulates insulin resistance through downregulating miR-192 and miR-375 involved in increasing plasma glucose levels and insulin resistance (Hernández-Alonso et al., 2017) (Table 2). Probiotics modulate different inflammatory diseases. For instance, probiotics such as Lactobacillus rhamnosus and Lactobacillus delbrueckii downregulate the expression of miR-181a and miR-155 involved in the inflammatory response of systemic lupus erythematosus (Vahidi et al., 2018). In addition, the intake of probiotics such as bifidobacterium modulates cell cycle senescence by regulating miR-34 and increasing Sirtuins 1 resulting in wellbeing and reduced aging (Haslberger et al., 2021). Nicotinamide mononucleotide supplementation enhances the antiaging properties of the aging aorta by upregulating miR-146a, and miR-203 and downregulating miR-99b and miR-127 (Figure 3) (Kiss et al., 2019).
5 Mechanisms and pathways
5.1 Molecular mechanisms underlying miRNA-mediated regulation of aging processes
MiRNAs are increasingly acknowledged as key players in aging and longevity. Several miRNAs directly impact lifespan by affecting major aging pathways. While most insights into miRNAs that influence longevity come from invertebrate studies, the mechanisms, and roles of miRNAs in aging are similarly observed in mammals.
Mechanisms in which miRNAs control gene expression often involve their “seed” sequences interacting mostly with the 3′-end, and less frequently with the 5′-end, of mRNA that is transcribed from targeted genes. In the past decade, many studies have focused on both quantitative and qualitative evaluations of miRNA expression, revealing significant alterations in miRNA expression patterns in different diseases. Consequently, analyzing miRNA expression profiles can be a crucial method for diagnosing and treating diseases (Gulyaeva and Kushlinskiy, 2016).
Cellular and molecular damage gradually accumulates over time, triggering the intricate process of aging. Ultimately, this accumulation results in a generalized decline in physiological functions, an elevated risk of mortality, and the end of life. Even while a variety of environmental and random circumstances have a role in an individual’s aging process, there is a significant basic inherited component to aging too. It has been proposed that systems crucial for organism development and cell proliferation may indirectly affect gene expression patterns that govern aging and senescence (Campisi, 2005). Furthermore, it has been found that several elements that play a universal function in all species control how long Caenorhabditis elegans survives. These include heat-shock factors (HSFs), sirtuins, AMP-activated protein kinases (AMPKs), mitogen-activated protein kinases (MAPKs), and signaling through the insulin/insulin-like growth factor-1 (IGF-1) signaling (IIS) pathway (Smith-Vikos and Slack, 2012).
5.1.1 Interplay between insulin signaling and the aging process
The most studied system in aging research, the IIS Signaling pathway, was initially found to control longevity in C. elegans (Friedman and Johnson, 1988; Kenyon et al., 1993). The insulin or IGF-1 receptor, also known as DAF-2 in C. elegans, is activated in this pathway. This activation starts a series of subsequent events that eventually control longevity. In situations of gathering and resource scarcity during development, DAF-2 affects the organism’s decision to enter dauer, a dormant state. In adulthood, DAF-2 reacts to insulin-like peptides that are mostly released by neurons in maturity, when its function changes to controlling longevity on a cellular level. Through a phosphorylation cascade involving PI3K (AGE-1 in C. elegans), the phosphatidylinositol (PtdIns)-dependent kinase (PDK) (counteracted by DAF-18, the C. elegans counterpart of the PtdInsP3 phosphatase PTEN), AKT, and SGK, it suppresses the FOXO transcription factor, DAF-16 in C. elegans. DAF-16 travels to the nucleus while this sequence is inactive, but phosphorylation retains it in the cytoplasm. It either activates or inactivates several genes that control metabolism, pathogen resistance, heat-shock proteins, superoxide dismutase, catalase, and metallothionein, among other components of the cellular stress response. This coordinated reaction results in increased longevity (Zhu et al., 2010).
It has been determined that DAF-2, DAF-16, and HSF-1 are all involved in the same pathway as LIN-4 and LIN-14 (Boehm and Slack, 2005). This suggests a theoretical approach where DAF-2 and LIN-14 either concurrently downregulate DAF-16’s function or where LIN-14 might function upstream of DAF-2. Furthermore, DAF-16 has been shown to decrease lin-4 expression, suggesting that there may be a negative feedback loop between lin-4 and DAF-16. It's crucial to remember that the aging process has not yet been proven to involve this particular mechanism (Figure 4) (Baugh and Sternberg, 2006).
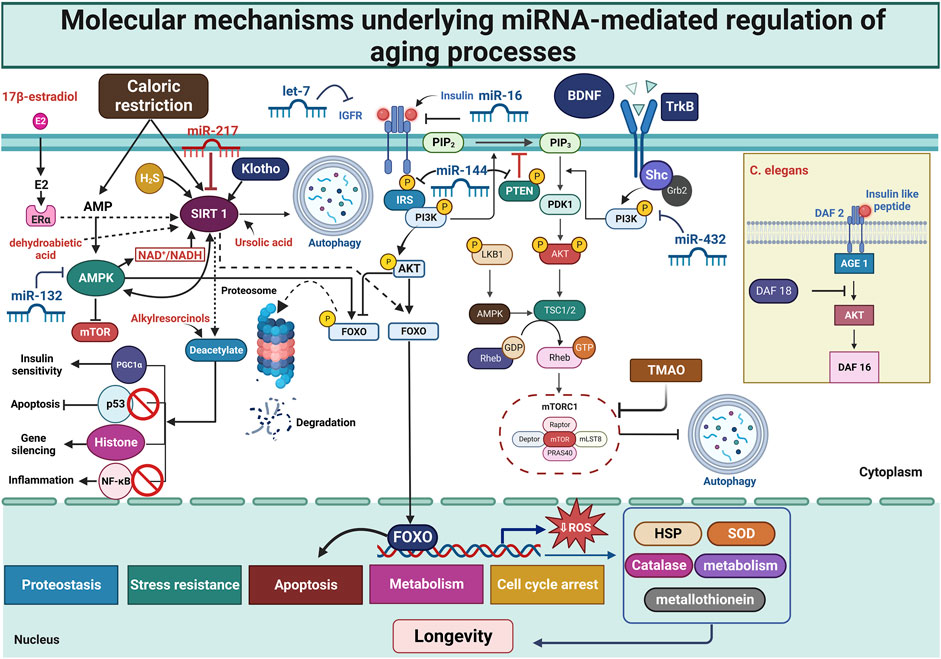
Figure 4. Molecular mechanisms underlying miRNA-mediated regulation of aging processes. This figure illustrates the molecular basis of miRNA-mediated control over aging, with a focus on nutrition-sensing pathways and aging-related signaling networks. Across various species, miRNAs play a crucial role in regulating lifespan and key aging mechanisms. Lifespan and age-related processes are governed by AMPK, Sirtuins, and the Insulin/IGF-1 signaling pathways. The interconnection between cell metabolism, stress response, and longevity is established through nutrient-sensing mechanisms such as mTOR and the IGF1/PI3K/AKT pathway. Diet affects miRNA modulation, highlighting the complex link between nutrition, molecular aging pathways, and lifespan regulation. A comprehensive understanding of the complex interplay between miRNAs and nutrient-sensing pathways can shed light on the causes of aging and identify potential drug targets. AGE, advanced glycation end products; Akt, protein kinase B; AMP, adenosine monophosphate; AMPKs, AMP-activated protein kinases; BDNF, brain-derived neurotrophic factor; ER, estrogen receptor; FOXO, Forkhead box protein O; HSP, heat shock protein; IGF-1, insulin-like growth factor-1; IGFR, insulin-like growth factor receptor; IRS, insulin receptor substrate; mTOR, mammalian target of rapamycin; mTORC, mTOR complex; NF-κB, nuclear factor kappa beta; PDK1, 3-phosphoinositide-dependent kinase 1; PGC1α, peroxisome proliferator-activated receptor gamma coactivator 1-alpha; PI3K, phosphoinositide-3-kinase; PIP2, phosphatidylinositol (4,5)-bisphosphate; PIP3, phosphatidylinositol (3,4,5)-trisphosphate; PRAS40, proline-rich Akt substrate of 40 kDa; PTEN, Phosphatase and Tensin Homolog; ROS, reactive oxygen species; SIRT1, Sirtuin 1; SOD, superoxide dismutase; TGF-β, Transforming growth factor β; TLR4, Toll-like receptor 4; TMAO, trimethylamine N-oxide; TrkB, Tropomyosin receptor kinase B; TSC, Tuberous sclerosis protein.
5.1.2 Role of AMP-Activated protein kinases in the aging process
Under regular circumstances, the AMPK, known for its role in prolonging longevity, is primarily responsible for maintaining metabolic balance and autophagy. This involves the elimination of damaged cellular components and molecules. A variety of molecules from the sirtuin family, known for their life-span extension capabilities, are involved in regulating metabolism and repairing DNA damage. These molecules have either mono-ADP-ribosyltransferase or deacylase activity. Additionally, another group of factors promoting longevity includes FOX (forkhead box) proteins. These proteins are a class of transcription factors that regulate the expression of genes linked to the development, division, growth, and lifespan of cells. Nicotinamide mononucleotide (NMN), a precursor to NAD+ that is associated with longer life spans, is produced by the enzyme nicotinamide phosphoribosyltransferase (NAMPT) (Siamak, 2020). Through the renewal of autophagic flux and the NAD+ synthetic rescue strategy, AMPK protects cells from oxidative stress-induced senescence by raising NAD+ levels in aged cells. (Han et al., 2016). The enzyme Klotho, which helps control insulin sensitivity and minimizes oxidative stress, declines with age, accelerating aging in mice. Furthermore, transsulfuration pathways and hydrogen sulfide are essential for preserving cells and extending life. The dysfunction of several cellular pathways, including PI3K/AKT, mTOR, insulin/IGF signaling, and p53, is another hallmark of aging. These pathways can result in immune system deterioration, metabolic problems, and poor cell maintenance. These alterations have the potential to cause cancer by reducing the number of stem cells and inducing senescence or death in cells (Figure 4) (Siamak, 2020).
5.1.3 The impact of SIRT1 signaling on the aging process
Elevated expression levels of sirtuins, especially SIRT2 and SIRT6, have been found to prolong the lifespan in many taxa, including budding yeast (S. cerevisiae), nematodes (C. elegans), fruit flies (D. melanogaster), and mice (Kaeberlein et al., 1999; Tissenbaum and Guarente, 2001; Rogina and Helfand, 2004; Kanfi et al., 2012). Sirtuin affects a variety of biological processes, which helps organisms survive more. More specifically, it has been shown that SIRT1 activation improves insulin sensitivity and lowers insulin resistance (Wang et al., 2019). Alkylresorcinols, belonging to the group of phenolic lipids, stimulate SIRT1-mediated deacetylation. This results in decreased acetylated histone levels in human monocyte cells and contributes to the development of lifespan in D. melanogaster (Kayashima et al., 2017). Ursolic acid possesses the ability to activate SIRT1 directly by binding to its external surface. This binding alters SIRT1’s configuration, switching it from a dormant to an active state. This interaction is observed in computational simulations (in silico), laboratory experiments in vitro, and in vivo, and it is significantly important in the aging process (Figure 4) (Kayashima et al., 2017). Kim et al., 2015 have found that dehydroabietic acid, a diterpene resin acid naturally present in conifer trees, can directly activate SIRT1. This activation contributes to reducing the buildup of lipofuscin and decreasing collagen secretion in humans. Additionally, this compound has demonstrated an ability to extend the lifespan of C. elegans. A03, a compound developed to interact with ApoE4 and boost SIRT1, has shown effectiveness in enhancing SIRT1 expression in the hippocampus of 5xFAD-ApoE4 (E4FAD) mice, a model for AD. This increase in SIRT1 expression has resulted in improved cognitive abilities in these mice (Campagna et al., 2018). 17β-estradiol triggers the ERα/SIRT1 pathway, which helps reduce oxidative stress, neuroinflammation, and neuronal apoptosis in male mice induced with d-galactose. Additionally, it elevates SIRT1 levels by promoting the degradation of PPARγ through the E3 ubiquitin ligase NEDD4-1, thereby delaying cellular aging (Han et al., 2013; Khan et al., 2019). Despite the numerous positive impacts of SIRT1 on aging and its widespread presence in the body, the mechanisms by which it contributes to anti-aging are not clearly understood.
5.1.4 The influence of mTOR signaling on aging
The fact that mTOR is linked to many aging-related processes, its function as a crucial regulator of longevity and aging has been extensively studied over the last 10 years (Weichhart, 2018; Liu et al., 2019; Papadopoli et al., 2019). While the detailed mechanisms remain partially understood, the link between mTOR signaling and aging is evident across species, from worms to mammals (Vellai et al., 2003; Kapahi et al., 2004; Kaeberlein et al., 2005; Guertin et al., 2006). The Ras-related GTP-binding protein (Rag), which controls amino acid signaling, and the tuberous sclerosis complex (TSC)-Rheb pathway are both involved in the regulation of mTORC1 (Arriola Apelo and Lamming, 2016; Soukas et al., 2019). Numerous studies indicate that mTORC1 responds to various environmental stimuli, including glucose, growth hormones, oxygen, and amino acids. It affects many biological processes, such as autophagy, cell division, and protein synthesis (Widlund et al., 2013; Arriola Apelo and Lamming, 2016). Similarly, mTORC2 is a downstream effector of IIS signaling following PI3K activation and plays a vital role in activating a range of kinases. For example, the important regulator of cell survival, Akt/PKB, is phosphorylated and activated by mTORC2 (Sarbassov et al., 2006; Arriola Apelo and Lamming, 2016). Given that mTOR has negative impacts on aging, it seemed plausible to believe that mTOR expression would increase in the older ages. However, it was found that as people aged, mTOR activity as well as that of its upstream signaling pathways, such as brain-derived neurotrophic factor (BDNF)/PI3K/Akt, decreased (Figure 4) (Yang F. et al., 2014). Numerous negative effects, such as glucose intolerance, diabetes, lowered activity levels, and immunosuppression, have been brought upon by interfering with mTORC2 (Arriola Apelo and Lamming, 2016; Chellappa et al., 2019). As a result, it has been demonstrated that reducing mTORC1 can increase lifespan and slow down the aging process, whereas blocking mTORC2 can have an adverse effect on health and longevity. Age-related deficiencies in spatial learning and memory are reduced when mTOR is long-term suppressed. However, an inverted U-shaped curve characterizing the link between mTOR activity and cognitive function suggests a dose-effect relationship (Chellappa et al., 2019). Trimethylamine-N-oxide (TMAO), an intestinal flora metabolite, suppresses mTOR signaling in SAMP8 and SAMR1 mice, which has a deleterious effect on age-related cognitive decline. This is evidenced by exacerbated synaptic damage and reduced expression of proteins associated with synaptic plasticity (Li D. et al., 2018).
5.1.5 Aging and inflammatory processes
Aging is marked by widespread chronic inflammation, associated with cellular aging, immune system decline, organ malfunction, and various diseases linked to aging. The Senescence-associated secretory phenotype (SASP) refers to a multifaceted process linked with cellular aging, marked by the secretion of pro-inflammatory cytokines from cells that are aging (Olivieri et al., 2015). Essential components of the SASP encompass a range of inflammatory cytokines, chemokines, proteases, and growth factors (Coppe et al., 2008; Davalos et al., 2013; Basisty et al., 2020). This occurrence plays a role in numerous age-related transformations, such as increased inflammation, changes in the immune system, and the proliferation of tumors (Birch and Gil, 2020). Recent research has determined shared components of the SASP across various cell types and triggers of senescence. Some of these components, like serine protease inhibitors, stanniocalcin, and growth differentiation factor 15, are also recognized as markers of aging in human blood plasma (Basisty et al., 2020). Elements such as impaired mitochondria, a persistent DNA damage response, (Rodier et al., 2009), and activation of proteins like C/EBPβ and NF-κB are critical in driving this process (Kulkarni et al., 2020).
5.1.6 Other factors contributing to the aging process
Numerous aging-related biological processes and molecular activities could be associated with ferroptosis. As cells age and undergo alterations in function and metabolism, they may become more prone to external influences, increasing their susceptibility to ferroptosis. Current studies have emphasized the contribution of ferroptosis in the acceleration of aging in skeletal muscles. In this scenario, aging skeletal muscles exhibit reduced expression of Tfr1 and increased expression of Slc39a14, predominantly observed on the cell membranes of skeletal muscle cells in aging mice. The heightened expression of Slc39a14 results in enhanced absorption of non-transferrin-bound iron, causing an accumulation of free iron ions in skeletal muscles, which in turn initiates ferroptosis (Ding et al., 2021).
Additionally, the connection between ferroptosis and aging seems to be bidirectional. Ferroptosis could play a role in triggering various age-related conditions, thereby accelerating the aging process in tissues and cells. On the other hand, the alterations in cellular function and metabolism that occur with aging may increase the cells’ vulnerability to ferroptosis, potentially worsening the progression of diseases.
5.2 Signaling pathways involved in miRNA modulation by nutrients and nutraceuticals
Aging research is progressively concentrating on alterations in nutrient sensing pathways, recognizing their potential to be modulated through pharmacological interventions as well as dietary modifications (Longo et al., 2015). Important nutrition sensing mechanisms, including the AMPK/Sirtuin/PGC1 and IGF1/PI3K/AKT/mTOR pathways, can be disrupted by aging. Numerous cellular activities, including protein synthesis, cell cycle progression, DNA replication, autophagy, stress response, and glucose homeostasis, are dependent on these pathways. Environmental factors such as pollution, physical exercise, smoking, and food can alter the biological mechanisms linked to aging.
Caloric restriction (CR) is recognized as an effective dietary strategy to extend lifespan healthily, highlighting diet as a key environmental factor influencing the aging process (Longo et al., 2015; Most et al., 2017). Dietary choices and compounds can impact molecular aging pathways, thereby altering the quality and healthfulness of aging. Recent research indicates that miRNAs play a role in regulating age-related activities, such as DNA replication, cellular aging (senescence), and programmed cell death (apoptosis). Additionally, miRNAs have been found to influence nutrient sensing pathways Thus, miRNAs not only regulate nutrient sensing pathways but can also be influenced by dietary factors (Figure 4) (Micó et al., 2017).
5.2.1 The role of nutrient sensing pathways in molecular aging
Studies indicate that mTOR, the insulin/IGF-1/PI3K/AKT, and the AMPK/SIRT1 pathways are important participants in the process by which CR prolongs life (Johnson et al., 2013; Longo et al., 2015; Altintas et al., 2016; Martins et al., 2016). These pathways demonstrate a connection between nutrition, age, and metabolism. Elevated glucose causes insulin production, which in turn raises IGF-1 levels. IGF-1 causes autophosphorylation when its receptor binds to it, which activates PI3K. PI3K then phosphorylates and activates AKT, which in turn phosphorylates and activates mTOR while suppressing FOXO. This complete signaling cascade is influenced by CR, which decreases glucose levels. Studies have demonstrated that prolonging the life span of species such as yeast, worms, flies, and mice can be achieved by decreasing mTOR signaling (Johnson et al., 2013). Longevity was increased in mice given the mTOR inhibitor rapamycin. Since rapamycin reduces glucose tolerance and doesn't mimic the effects of dietary restriction on insulin, IGF-1, or leptin levels, the exact mechanism is yet unknown (Miller et al., 2014). FOXO transcription factors play a critical role in pathways related to longevity that include antioxidant activity, stress response, and cellular processes like autophagy, apoptosis, and proliferation (Martins et al., 2016), which also play a role in extending lifespan. Reduced insulin/IGF-1-like signaling in C. elegans increases lifespan and stress tolerance via blocking the FOXO protein DAF-16 and resulting in the nuclear build-up of SKN-1, a process that is independent of DAF-16 inhibition and controlled by AKT1/2 and SGK-1 phosphorylation (Tullet et al., 2008). CR has been shown to change the transcriptional and post-transcriptional levels of genes associated with the PI3K/AKT/FOXO pathway in both humans and rats. Notably, certain polymorphisms in the FOXO3 gene have been linked to increased lifespan and a reduced risk of mortality from both overall and coronary heart disease (Willcox et al., 2016). Furthermore, CR was able to improve several pathways in both humans and rats, including mitochondrial function, oxidative phosphorylation, muscular contraction, glycolysis, and gluconeogenesis. Conversely, pathways linked to the “aging brain” and insulin/IGF-1 signaling became less active (Mercken et al., 2013a).
5.2.2 Role of miRNA as modulators of nutrient sensing pathway
Recent studies indicate that miRNAs have a notable impact on various aspects of aging, such as the disturbance of nutrient-sensing pathways, weakening of the immune system, cellular damage, and the onset of age-related diseases (López-Otín et al., 2013). The miRNA let-7, which targets the IGF1 receptor and mTOR among other components of the IGF1 pathway, has an impact on the IGF1/PI3K/AKT/mTOR pathway (Jung and Suh, 2014). Myoblast research has demonstrated that miR-432 inhibits myoblast development and proliferation by interfering with the PI3K/AKT/mTOR pathway (Chartoumpekis et al., 2012; Ma et al., 2017). Diet has been found to modulate this miRNA; for instance, miR-432 is downregulated in the adipose tissue of mice on a high-fat diet (HFD) (Chartoumpekis et al., 2012). Age-related decreases in miR-17-92 cause more DNA damage and oxidative stress (Grillari et al., 2010). MiRNAs also affect the AMPK/Sirtuins/PGC1-1 pathway, with let-7 controlling the production of SIRT1 in human biliary epithelial cells (Xie et al., 2014). miR-217 downregulates SIRT1, impacting endothelial cell aging through the silent information regulator 1 (SIR1) (Figure 4) (Menghini et al., 2009). Additionally, miR-133 inhibits AMPK expression, targeting this pathway at multiple points. Kurylowicz et al. observed in humans that a decrease in SIRT1 is associated with higher levels of miR-22-3p in obese individuals, while an increase in SIRT7 is linked to lower miR-125a-5p levels in thin individuals (Kurylowicz et al., 2016). Studies using in vitro and in vivo models have demonstrated that miR-16 reduces cell proliferation by targeting the IGF1R (Chen et al., 2013). Additionally, miR-144 plays a crucial role in regulating key pathways. It controls the IGF-1/PI3K/AKT pathway by targeting PTEN (Zhang et al., 2013) and IRS1 (Wu et al., 2016), and also directly influences mTOR (Xiang et al., 2016) and AMPK (Xiang et al., 2016). Olivieri et al. observed reduced levels of miR-182, miR-223, and miR-142-3p in the skeletal muscle of postmenopausal women. These miRNAs are involved in the regulation of IGF-1R and FOXO3A expression and activate the insulin/IGF-1 pathway by promoting the phosphorylation of AKT and mTOR (Olivieri et al., 2014). Notably, miR-142-3p was found to increase in the adipose tissue of mice on a HFD (Chartoumpekis et al., 2012), and a similar upregulation of miR-182 was observed in the liver of mice on an HFD (Tessitore et al., 2016).
Although the exact mechanisms through which CR prolongs lifespan remain unclear, miRNAs are assumed to be crucial. In rat studies, CR led to a decrease in miR-144 expression in cerebromicrovascular endothelial cells, which helps maintain NRF2 levels, a key regulator of cellular defense against oxidants (Csiszar et al., 2014). In rhesus monkeys aged rhesus, miR-144, which is typically upregulated in skeletal muscle with age, showed reduced upregulation. The same study found that other age-related miRNAs, which regulate the PI3K/AKT pathway and SIRT1, were significantly increased in older monkeys undergoing CR. Additionally, the role of miR-221, which is downregulated by both CR (Mercken et al., 2013b) and polyunsaturated fatty acids (Ortega et al., 2015), is noteworthy.
In contrast, the regulation miR-145 doesn't appear to be affected by CR, though it is increased by a HFD (Sangiao-Alvarellos et al., 2014). It is noteworthy to acknowledge the ongoing debate regarding alternative dietary approaches to promote longevity, such as protein restriction, which has the potential to alter the expression of specific miRNAs. Furthermore, it has been observed that a low-protein diet reduces the expression of miR-124a in the pancreatic islets of pregnant rats (de Siqueira et al., 2018), whereas, in overweight or obese males, a high-protein diet decreased HDL-associated miR-223 levels (Tabet et al., 2016). These findings suggest that different miRNAs can be affected by dietary interventions associated with longevity, such as CR or protein restriction. However, the precise consequences of these changes on extending lifespan still require further investigation to be fully elucidated.
5.2.3 Nutraceuticals: regulation of miRNAs
Research has established a connection between typical neural development and the onset of neural disorders, which arises from a complex interplay between genetic factors and environmental influences, including nutrition. The role of diet in maintaining brain health throughout life is well-documented, with research indicating that what we eat affects neuron development, regeneration, function, and the maintenance of neural networks (Maher, 2000; Dauncey, 2009; Nurk et al., 2009; Gomez-Pinilla and Nguyen, 2012; Milte et al., 2012; Sinn et al., 2012). Interestingly, nutrition is one of the factors that can control gene expression without altering the DNA sequence itself. Recent focus has shifted to the role of miRNAs in both development and disease, including how their expression is influenced by nutrition. Although there has been extensive exploration of the role of nutrition in nervous system development and related issues like aging and neurodegenerative disorders, studies on the regulation of miRNAs in brain pathology by nutraceuticals are relatively sparse (Li D. et al., 2018). This review will cover a few existing studies on how nutraceuticals can regulate miRNAs associated with brain pathologies, including cancers and neurodegenerative disorders.
5.2.3.1 Resveratrol
The primary mechanism by which resveratrol reduces proinflammatory lipid mediators is via inhibiting cyclooxygenase 1 and 2 (COX-1 and COX-2), as well as by reducing the mobility and activity of NF-κB’s p65 subunit when stimulated by TNF. This leads to reducing the levels of pro-inflammatory cytokines like TNF-alpha, IL-1 beta, and IL-6 (Latruffe et al., 2015). Additionally, resveratrol inhibits JNK and its upstream kinase MEK activation, which is advantageous for AP-1 activity suppression. Tili et al., 2010 demonstrated in human monocytes (THP-1), resveratrol could modulate AP-1 through epigenetic means by upregulating anti-inflammatory miR-663, targeting JunB and JunD, and downregulating pro-inflammatory miR-155. Song et al., 2016 further showed that longer exposure to resveratrol increased miR-Let7A expression in THP-1 cells, leading to reduced TNF-α and IL-6.
In a human study, researchers conducted a randomized, placebo-controlled trial involving 35 men with type-2 diabetes and hypertension. Over a year, participants were given capsules containing either a placebo (maltodextrin), grape extract supplemented with over 8 mg of resveratrol (GE-RES), or grape extract without resveratrol (GE). Compared to the control group, the GE-RES group showed alterations in miRNA associated with inflammation: miR-21, −181b, −663, and −30c2 levels increased, while miR-155 and −34a levels decreased. This suggests that resveratrol’s modulation of miRNAs might provide a protective effect against physiological variations (Tomé-Carneiro et al., 2013).
5.2.3.2 Vitamins
Several vitamins have been shown to affect immunity and contribute to the prevention of disease. A good example of this is vitamin D, which can regulate the transcription of miRNA genes through its active form, dihydroxy vitamin D (1,25(OH)2D). This active form binds to the transcription factor vitamin D receptor (VDR) and influences the stability of miRNA or the genes responsible for miRNA processing (such as Drosha and Dicer) to enhance the maturity of miRNAs (Dambal et al., 2017; Zeljic et al., 2017).
Vitamin D inhibits inflammation through its effects on miRNAs, particularly miR-155, which has an antagonistic relationship with the vitamin D receptor (VDR). In patients with primary biliary cholangitis, the SOCS1 protein in the liver and PBMCs is correlated with the levels of miR-155 (Kempinska-Podhorodecka et al., 2017). Furthermore, vitamin D decreases miR-146a, miR-150, and miR-155 in murine adipocytes to suppress NF-κB signaling, which in turn affects p65 and IκB phosphorylation (Karkeni et al., 2018). VDR activators, like calcitriol and paricalcitol, suppress miR-29b and miR-30c in nephrectomized rats, impacting genes like COL1A1, MMP-2, and CTGF, thus reducing cardiac fibrosis (Panizo et al., 2017). A 12-week treatment was shown to reduce inflammatory and atherosclerotic cytokines and miRNAs in patients with moderate chronic renal disease (Mansouri et al., 2017). Moreover, the research indicates that supplementing with vitamin D enhances the presence of miRNAs that suppress tumors in the prostate tissue of patients (Giangreco et al., 2013)
Vitamin A derivatives, such as various forms of retinoic acid, are known to control gene expression in both normal and disease conditions (Perri et al., 2017). In an experiment with mouse embryonic stem cells, exposure to retinoic acid resulted in the upregulation of 31 miRNAs and the downregulation of 175 miRNAs. The decreased miRNAs miR-200b and miR-200c were distinguished for their noteworthy ability to significantly upregulate the expression of two pluripotent genes that are critical for development and epithelial phenotyping: Oct4 and Nanog (Zhang J. et al., 2015). The expression of miR-10a and the Retinoic Acid Receptor Beta (RARβ) is significantly increased by retinoic acid (RA) in two breast cancer cell lines, T47D and SK-BR-3. Due to their associations with tumor suppression in breast tissue samples, reduced expression of RARβ and miR-10a has been related to breast cancer (Khan et al., 2015).
5.2.3.3 Curcumin
Besides inflammation recent research has revealed that curcumin can influence certain miRNAs in various cancer cell types through its impact on the inflammatory response. Kronski et al. (Kronski et al., 2014) found that treating breast cancer cells with curcumin leads to an increase in miR-181b levels, which in turn reduces the production of pro-inflammatory cytokines CXCL1 and −2. This reduction contributes to a decreased metastatic potential in these cells. Additionally, research on breast stromal fibroblasts has demonstrated that the tumor-suppressing protein p16INK4A, which is regulated by miR-146b-5p, lowers the carcinogenic effects of these cells by regulating IL-6 production and secretion. This particular miR targets a specific sequence in the IL-6 3′UTR. Treatment with curcumin may increase the levels of p16INK4A and miR-146b-5p, which could then result in the reduction of IL-6 (Al-Ansari and Aboussekhra, 2015).
5.2.3.4 Quercetin
Quercetin’s role in reducing inflammation is connected to its capability to suppress both the ERK and JNK proteins, as well as their phosphorylated forms, and to lower the production of TNF-α induced by these proteins.
In research by Boesch-Saadatmandi et al. (Boesch-Saadatmandi et al., 2012), it was revealed that female mice consuming a diet high in quercetin (2 mg/g) showed notably increased levels of hepatic miR-125b and miR-122 compared to those on a regular diet. miR-125b is known for its capacity to diminish inflammation, whereas miR-122 plays a key role in the control of lipid metabolism. In a separate study focusing on the influence of quercetin and its main metabolites on miR-155, researchers observed that the use of quercetin and isorhamnetin on LPS-stimulated macrophages resulted in a reduction in miR-155 expression. This effect could suggest a potential mechanism by which this polyphenol hinders NF-κB activation, consequently contributing to the attenuation of inflammation (Boesch-Saadatmandi et al., 2011).
Diets high in saturated fatty acids, particularly from high-fat foods, are linked to health issues like gut imbalance, low-grade inflammation, and insulin resistance (Cani et al., 2007; O'Keefe et al., 2008; Ruiz-Núñez et al., 2013; Martinez et al., 2017). Certain saturated fats, like lauric and palmitic acids, trigger inflammation in various cells by activating specific receptors, leading to increased inflammatory gene expression (Wong et al., 2009; Wang Y. et al., 2017). Recent studies also suggest that saturated fats can influence these processes through epigenetic changes, including the action of miRNAs.
5.2.3.5 Polyunsaturated fatty acids
Lipid mediators formed from essential fatty acids, such as thromboxanes, lipoxins, resolvins, and leukotrienes, are a few examples of how polyunsaturated fatty acids (PUFA) might affect the inflammatory response. Studies have demonstrated that these lipid mediators can control the expression of genes by means of miRNAs (Fredman et al., 2012; Krishnamoorthy et al., 2012; Visioli et al., 2012). In a study by Zheng et al., 2015 rats that were about 3 weeks old were given a Western-style diet for a week. After that, the rats were divided into three groups, one of which received an omega-3 PUFA diet (a combination of EPA and DHA), another of which received an omega-6 PUFA diet (linoleic acid), and a control group. After 16 weeks, in comparison to the control group, the omega-3 group showed a reduction in subcutaneous fat and pro-inflammatory cytokines. Unlike the omega-6 and control groups, this group’s levels of miRNAs (rno-miR-19b-3p, −146b-5p, and −183-5p) were decreased. These miRNAs were found to suppress genes involved in inflammatory pathways.
Additionally, during 8 weeks, thirty healthy participants in a human trial ate 30 g of polyunsaturated fatty acid-rich almonds and pecans every day. Eleven miRNAs were altered in their plasma as a result: levels of miR-192, miR-486-5p, miR-19b, miR-106a, miR-130b, miR-18a, and miR-769-5p were elevated and levels of miR-328, miR-330-3p, miR-221, and miR-125a-5p were lowered. Notably, favorable changes in the levels of the plasma protein C-reactive were associated with miR-221 and miR-130b (Ortega et al., 2015).
6 Implications for prevention and treatment of age-related diseases
6.1 miRNA involvement in the therapeutic impact of nutraceuticals on aging and age-related diseases
Aging and age-related diseases occur due to multi-faceted pathologic incidences. One of these pathologic incidences is the chronological increase in senescent cells (SCs) which undergo irreversible cell cycle arrest, and simultaneously increase the release of various chemicals that stimulate inflammation and oxidative stress signaling pathways. This SCs phenotype is identified as a senescence-associated secretory phenotype (SASP) (Prasnikar et al., 2021). Therefore, foods high in polyphenols and other bioactive compounds, which have anti-inflammatory and antioxidant properties, may be used as “anti-senescence” or “senolytic” foods in a nutraceutical strategy for enhanced cellular longevity and better aging (Gurau et al., 2018). Indeed, nutraceuticals rich in polyphenols have shown excellent results in many age-related diseases owing to their antioxidant and anti-inflammatory effects (Zhor et al., 2023).
Epigenetic dysregulation is a part of the aging process, and many nutraceuticals are regarded as promising candidates to counteract these age-related epigenetic changes (Bacalini et al., 2014). Likewise, changes in miRNA expression play a prominent role in regulating the mRNA expression of genes involved in the shaping of aging and age-related diseases (Matai and Slack, 2023a). Therefore, it’s expected that diets or nutraceuticals that control specific miRNA expression can enhance healthy aging or halt the progression of age-related diseases. So far, it has been reported that dietary intake of micronutrients, macronutrients, vitamins, minerals, flavonoids, and polyphenols can modulate the expression of various miRNAs (Dimmeler and Nicotera, 2013; Milenkovic et al., 2013; Beckett et al., 2014; Yu et al., 2021). However, future research will need to determine whether dietary manipulation of miRNAs contributes to the prevention or treatment of age-related illnesses.
As we age, we experience a low-grade systemic inflammation, a condition that has been previously termed “inflammaging.” This condition is marked by an increase in the levels of pro-inflammatory cytokines (mostly TNF-α and IL-6) and circulating acute-phase proteins (Franceschi et al., 2000; Franceschi, 2007). Frailty and the onset and advancement of age-related illnesses, such as cancer, osteoporosis, neurodegenerative diseases, atherosclerotic cardiovascular diseases, and type 2 diabetes mellitus are linked to this inflammaging phenomenon (Vasto et al., 2007; Cevenini et al., 2013). Numerous research provided evidence in favor of the theory that achieving extreme longevity likely necessitates a unique control of inflamma-miRs gene expression (ElSharawy et al., 2012; Olivieri et al., 2013). Quercetin, a plant flavonol, shielded CCD-18Co myofibroblasts from reactive oxygen species (ROS) and prevented inflammation by enhancing the activity of antioxidant enzymes and stimulating the upregulation of miR-146a, a negative regulator of pro-inflammatory nuclear factor kappa B (NFκB) activation (Noratto et al., 2011b).
Changes in miRNA expression were evident in brain aging and neurodegenerative diseases. Whether these changes in miRNA expression are the cause or consequence of brain aging is still a riddle (Somel et al., 2010). In the study of Luceri et al. (Luceri et al., 2017b), mice fed extra-virgin olive oil (EVOO) rich in phenols (H-EVOO) for 6 months demonstrated improvements in their motor and cognitive functions when compared to controls given the same olive oil but devoid of phenolics (L-EVOO). Interestingly, mice fed L-EVOO demonstrated a substantial modification in the expression of 175 miRNA, unlike mice fed H-EVOO which showed alterations in the expression of only 20 miRNAs compared to young mice. These results demonstrate the capacity of phenolic-rich olive oil to mitigate age-related modifications in miRNA expression. Moreover, miR-29, miR-30, miR-34, miR-101, miR-124, and miR-181, which have been linked to neurodegenerative diseases or senescence (Dimmeler and Nicotera, 2013), were among the miRNAs that were either low or not expressed in the cortex of young and H-EVOO-fed mice and strongly expressed in mice fed L-EVOO (Figure 5).
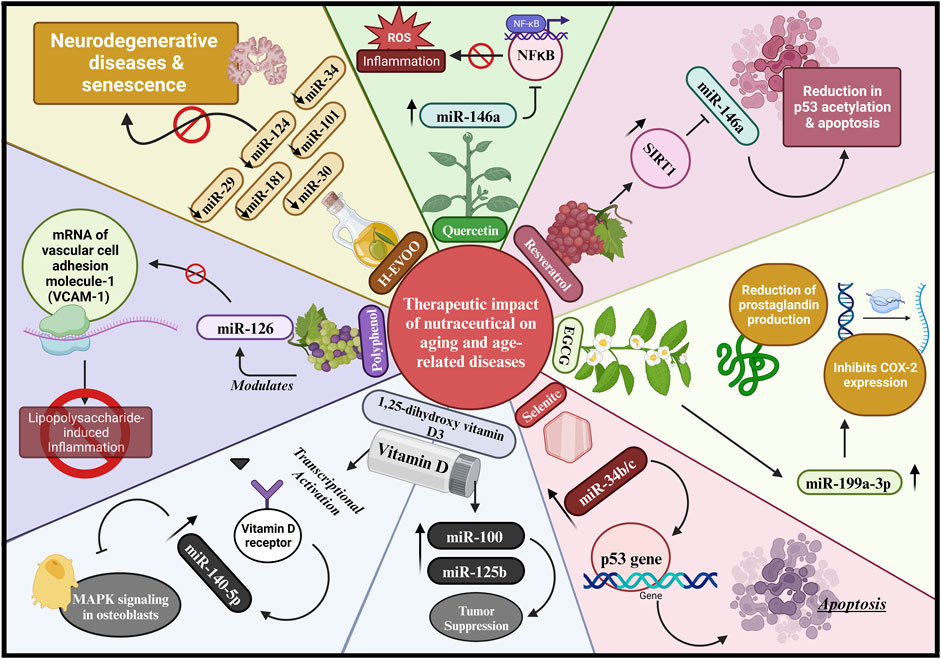
Figure 5. miRNA involvement in the therapeutic impact of nutraceuticals on aging and age-related diseases. Nutraceuticals rich in polyphenols and bioactive compounds exhibit anti-inflammatory and antioxidant effects, potentially enhancing cellular longevity and mitigating age-related changes. These compounds can influence miRNA expression, offering promising strategies for promoting healthy aging and preventing or treating age-related diseases. COX-2, cyclooxygenase 2; EGCG, epigallocatechin-3-gallate; H-EVOO, high-extra virgin olive oil; MAPKs, mitogen-activated protein kinases; NF-κB, nuclear factor kappa beta; ROS, reactive oxygen species; SIRT1, sirtuin 1.
As stated by Sir William Osler in his historical textbook in 1898: “Longevity is a vascular question, which has been well expressed in the axiom that man is only as old as his arteries” (COOK, 1908). Indeed, aging is intricately linked to the development of CVDs. Growing older is the primary risk factor for vascular endothelial dysfunction, which raises the possibility of CVDs, one of the leading causes of death in developed nations (Gaidai et al., 2023). These CVDs are mostly attributable to the sedentary lifestyle in the developed world, characterized by a diet rich in saturated fats and sugars (Kirkpatrick and Maki, 2021). On the contrary, a reduction in the onset of CVDs could be attributable to health-promoting dietary patterns, such as the Mediterranean diet rich in nutraceuticals (Accardi et al., 2016). An in vitro study conducted by Noratto et al. (2011a) showed that treatment of lipopolysaccharide-induced inflammation in human umbilical vein endothelial Cells (HUVEC) with a polyphenol extracted from grapes reduced the mRNA expression of vascular cell adhesion molecule-1 (VCAM-1) as a result of modulating the expression of miR-126.
Bone health deteriorates and the incidence of osteoporosis increases with increased age (Pignolo et al., 2021). Vitamin D is a key regulator of bone health by promoting calcium absorption, and its active form, 1,25-dihydroxyvitamin D (1,25-(OH)2-D), acts as a steroid hormone and exerts pleiotropic effects (Veldurthy et al., 2016). In a previous study by Jiao et al., 2020, the beneficial role of miR-140-5p in bone health was disclosed. Results revealed that miR-140-5p promoted osteogenic differentiation and led to a significant increase in osteocalcin expression, bone mineral density, and bone mass in addition to enhancing bone healing of miR-140-5p-transgenic mice with fracture. In the study conducted by Luo et al., 2018, a positive correlation between miR-140-5p and 1,25-(OH)2-D was revealed. In this study, miR-140-5p was upregulated in 2 types of murine osteoblasts treated with 1,25-(OH)2-D. The enhanced expression of miR-140-5p by 1,25-(OH)2D3 occurred through transcriptional activation of the vitamin D receptor and this activated vitamin D/vitamin D receptor/miR-140-5p axis led to a downstream inhibition of MAPK signaling in osteoblasts (Figure 5).
Cancer is regarded as one of the age-related diseases due to the build-up of mutations throughout life (Laconi et al., 2020). miR-100 and miR-125b were shown to have tumor suppressor effects and their expression levels were found to be reduced in several cancers. The study conducted by Giangreco et al., 2013 revealed another health benefit for 1,25-dihydroxy vitamin D3 in prostate cancer patients, in which vitamin D3 supplementation managed to enhance the expression of these tumor-suppressive miRNAs. This suggests that miRNAs may be important physiological mediators of vitamin D3 action in the early detection and prevention of prostate cancer.
All-trans-retinoic acid treatment upregulates miR-29a and miR-142-3p. In patients with acute myeloid leukemia (AML) and healthy controls, enhanced expression of either miRNA-induced myeloid differentiation. This shows that miR-29a and miR-142-3p are important regulators of healthy myeloid differentiation and that the development of AML is influenced by their decreased expression (Wang et al., 2012). Selenite is a nutraceutical and dietary form of selenium. It was shown that treatment of human prostate cancer cells (LNCaP) with selenite upregulated the expression of p53 and miR-34b/c, where miR-34 is known to target the p53 gene, and induction of p53 enhances apoptosis. This indicates that selenite may be beneficial for both the prevention and treatment of human prostate cancer (Sarveswaran et al., 2010).
Resveratrol, found in red grapes, is a popular bioactive compound that was extensively studied during the last 2 decades and found to have a myriad of protective effects against a wide variety of diseases (Meng et al., 2020). Adding to its well-known benefits, resveratrol can be protective against age-related problems such as hearing loss in which miR-34a was found to be involved in resveratrol’s protective mechanism. In the study of Xiong et al., 2015, miR-34a expression, p53 acetylation, and apoptosis were found to be enhanced in the cochlea of C57BL/6 mice with aging, however, an age-linked reduction in SIRT1 was noted. In the inner ear HEI-OC1 cell line, an inverse relation was revealed between miR-34a and SIRT1 expression; where the overexpression of miR-34a inhibited SIRT1 expression, which led to a rise in p53 acetylation and apoptosis. Inversely, silencing of miR-34a led to a significant increase in SIRT1 expression, and consequent reduction in p53 acetylation, and apoptosis. Resveratrol, being a SIRT1 activator, following 2-month administration, significantly attenuated miR-34a expression, which led to a significant reduction in hearing loss and hair cell loss in C57BL/6 mice (Figure 5).
Epigallocatechin-3-O-gallate (EGCG) is the most abundant and active polyphenol in green tea. The study of Rasheed et al., 2016 investigated the effect of EGCG in an in vitro model of osteoarthritis, one of the age-related chronic diseases. The study revealed that EGCG inhibited COX2 expression and prostaglandin E2 (PGE2) production via upregulating miR-199a-3p expression in an IL-1β-stimulated human osteoarthritis chondrocytes.
Notably, the majority of the data supporting the modification of miRNA by nutraceuticals in aging comes from cell lines and some of this data comes from in vivo pre-clinical studies. Translational research that can advance these studies from bench-to bedside or from pre-clinical to clinical studies in older adults will help determine if nutraceutical manipulation of miRNAs can improve healthy aging or halt the occurrence or progression of age-related diseases.
6.2 Strategies for utilizing miRNA-based interventions in age-related diseases
miRNAs have a crucial function in regulating gene expression post-transcription. They have emerged as important regulators of cellular functions and can be altered by dietary factors. Specific miRNAs selectively target genes responsible for encoding proteins and enzymes involved in pathways that sense nutrition. This targeting has the potential to greatly impact the regulation of the aging process (Lai et al., 2019). Several studies have demonstrated that diet and nutraceuticals can potentially control the expression of miRNAs linked to aging, neurodegenerative disorders, and brain tumors. Nutrients possess the capacity to control the amounts of miRNA, which plays a pivotal role in influencing gene expression. Therefore, the control of miRNA aging through nutrition and nutraceuticals holds great potential for treating age-related diseases and improving overall healthy aging.
The approaches for employing miRNA-based interventions in age-related diseases encompass the identification of disease-linked miRNAs, the manipulation of miRNA expression through nutrition and nutraceuticals, the creation of miRNA-based therapeutics, and the investigation of miRNAs as biomarkers for age-related diseases. These methods show potential for creating new strategies to encourage healthy aging and tackle age-related ailments (Lai et al., 2019).
Studies have demonstrated that different miRNAs play a role in the regulation of age-related illnesses, including AD, age-related macular degeneration, neurological disorders, and cancer (Natoli and Fernando, 2018; Eshkoor et al., 2022). Studies have shown that nutritional components and nutraceuticals can control the activity of miRNAs that have a role in age-related disorders. This suggests that they could be used as potential treatments (Ghosh et al., 2021).
miRNAs have been recognized as promising candidates for therapeutic intervention in age-related macular degeneration and other age-related ailments. Several miRNA-based medicines are currently undergoing Phase 2 clinical studies, indicating the efficacy of miRNAs as therapeutic targets (Natoli and Fernando, 2018).
This topic investigates the impact of nutrition on the alteration of gene expression through epigenetic mechanisms associated with miRNAs. This study assesses the impact of nutrients and bioactive chemicals on the expression of miRNA, which may have consequences for regulating inflammation and chronic diseases (Quintanilha et al., 2017a). Specific miRNAs have been proposed as prospective biomarkers, diagnostic tools, or desirable targets for the management and treatment of age-related illnesses, such as age-related macular degeneration (Cruz-Aguilar et al., 2023).
7 Nutraceuticals’ impact on aging and related diseases
In a previously reported systematic review, 200 adults were assessed on their use of different nutraceuticals. It was found that using nutraceuticals has improved mitochondrial oxidative capacity and bioenergetics (Lippi et al., 2022). A balanced diet supplemented with limonene controlled inflammation in elderly individuals decreasing fibrinogen and inflammatory markers (Ostan et al., 2016). In a retrospective study, administration of folic acid and vitamin B was linked to speed of processing, recall, identification, and verbal capability in middle-aged and elderly women (Bryan et al., 2002). Moreover, nutraceuticals containing fish oil and coenzyme Q10 are associated with reduced risk and retard the progression of Parkinson’s disease (Mischley et al., 2017). Further, a polyphenolics-rich diet inhibits lipid peroxidation in the CNS reducing aging-related diseases in a double-blinded clinical study (Arcusa et al., 2023).
8 Conclusion
MiRNAs hold great potential as a field of study for comprehending the molecular pathways that drive aging and age-related illnesses. The data given in this review emphasizes the possible influence of dietary variables and nutraceuticals in regulating miRNA expression and function. Existing research indicates that certain macronutrients, micronutrients, trace minerals, and nutraceuticals might impact miRNA profiles. Nevertheless, the majority of the existing research has been carried out in laboratory settings, and further investigations are required to clarify the impact of dietary miRNA manipulation on living organisms, including animal models and human patients. Subsequent studies should prioritize the examination of the specific mechanisms via which dietary variables and nutraceuticals regulate miRNA, as well as the exploration of the therapeutic possibilities of miRNA-based therapies in age-related disorders. By enhancing our comprehension of the intricate nutritional coordination facilitated by miRNAs, we might potentially establish novel approaches to encourage the process of healthy aging and counteract age-related disorders.
Author contributions
RS: Writing–review and editing, Writing–original draft, Conceptualization. NE: Writing–review and editing, Writing–original draft. AD: Writing–review and editing, Writing–original draft, Conceptualization. AA: Writing–review and editing, Writing–original draft. NA: Writing–review and editing, Writing–original draft. OM: Writing–review and editing, Writing–original draft. SA: Writing–review and editing, Writing–original draft, Visualization. SD: Writing–review and editing, Writing–original draft, Conceptualization.
Funding
The author(s) declare that no financial support was received for the research, authorship, and/or publication of this article.
Acknowledgments
The authors are thankful to the Deanship of Graduate Studies and Scientific Research at University of Bisha for supporting this work through the Fast-Track Research Support Program.
Conflict of interest
The authors declare that the research was conducted in the absence of any commercial or financial relationships that could be construed as a potential conflict of interest.
Publisher’s note
All claims expressed in this article are solely those of the authors and do not necessarily represent those of their affiliated organizations, or those of the publisher, the editors and the reviewers. Any product that may be evaluated in this article, or claim that may be made by its manufacturer, is not guaranteed or endorsed by the publisher.
References
Abdelfattah, A. M., Park, C., and Choi, M. Y. (2014). Update on non-canonical microRNAs. Biomol. Concepts 5 (4), 275–287. doi:10.1515/bmc-2014-0012
Abd El Fattah, Y. K., Abulsoud, A. I., AbdelHamid, S. G., AbdelHalim, S., and Hamdy, N. M. (2023). CCDC144NL-AS1/hsa-miR-143-3p/HMGA2 interaction: in-silico and clinically implicated in CRC progression, correlated to tumor stage and size in case-controlled study; step toward ncRNA precision. Int. J. Biol. Macromol. 253, 126739. doi:10.1016/j.ijbiomac.2023.126739
Abd El Fattah, Y. K., Abulsoud, A. I., AbdelHamid, S. G., and Hamdy, N. M. (2022). Interactome battling of lncRNA CCDC144NL-AS1: its role in the emergence and ferocity of cancer and beyond. Int. J. Biol. Macromol. 222, 1676–1687. doi:10.1016/j.ijbiomac.2022.09.209
Abdelmaksoud, N. M., Abulsoud, A. I., Abdelghany, T. M., Elshaer, S. S., Rizk, S. M., and Senousy, M. A. (2023). Mitochondrial remodeling in colorectal cancer initiation, progression, metastasis, and therapy: a review. Pathology - Res. Pract. 246, 154509. doi:10.1016/j.prp.2023.154509
Abdelmaksoud, N. M., Sallam, A.-A. M., Abulsoud, A. I., El-Dakroury, W. A., Abdel Mageed, S. S., Al-Noshokaty, T. M., et al. (2024). Unraveling the role of miRNAs in the diagnosis, progression, and therapeutic intervention of Alzheimer’s disease. Pathology - Res. Pract. 253, 155007. doi:10.1016/j.prp.2023.155007
Abozaid, O. A. R., Sallam, M. W., El-Sonbaty, S., Aziza, S., Emad, B., and Ahmed, E. S. A. (2022). Resveratrol-selenium nanoparticles alleviate neuroinflammation and neurotoxicity in a rat model of Alzheimer’s disease by regulating sirt1/miRNA-134/gsk3β expression. Biol. Trace Elem. Res. 200 (12), 5104–5114. doi:10.1007/s12011-021-03073-7
Accardi, G., Aiello, A., Gambino, C. M., Virruso, C., Caruso, C., and Candore, G. (2016). Mediterranean nutraceutical foods: strategy to improve vascular ageing. Mech. Ageing Dev. 159, 63–70. doi:10.1016/j.mad.2016.02.007
Al-Ansari, M. M., and Aboussekhra, A. (2015). miR-146b-5p mediates p16-dependent repression of IL-6 and suppresses paracrine procarcinogenic effects of breast stromal fibroblasts. Oncotarget 6 (30), 30006–30016. doi:10.18632/oncotarget.4933
Al-Noshokaty, T. M., Mesbah, N. M., Abo-Elmatty, D. M., Abulsoud, A. I., and Abdel-Hamed, A. R. (2022). Selenium nanoparticles overcomes sorafenib resistance in thioacetamide induced hepatocellular carcinoma in rats by modulation of mTOR, NF-κB pathways and LncRNA-AF085935/GPC3 axis. J. Life Sci. 303, 120675. doi:10.1016/j.lfs.2022.120675
Alnuqaydan, A. M. (2020). Targeting micro-RNAs by natural products: a novel future therapeutic strategy to combat cancer. Am. J. Transl. Res. 12 (7), 3531–3556.
Al-Rawaf, H. A., Alghadir, A. H., and Gabr, S. A. (2021). Circulating MicroRNA expression, vitamin D, and hypercortisolism as predictors of osteoporosis in elderly postmenopausal women. Dis. Markers 2021, 3719919. doi:10.1155/2021/3719919
Altintas, O., Park, S., and Lee, S. J. (2016). The role of insulin/IGF-1 signaling in the longevity of model invertebrates, C. elegans and D. melanogaster. BMB Rep. 49 (2), 81–92. doi:10.5483/bmbrep.2016.49.2.261
Arcusa, R., Carillo, J. Á., Cerdá, B., Durand, T., Gil-Izquierdo, Á., Medina, S., et al. (2023). Ability of a polyphenol-rich nutraceutical to reduce central nervous system lipid peroxidation by analysis of oxylipins in urine: a randomized, double-blind, placebo-controlled clinical trial. Antioxidants 12 (3), 721. doi:10.3390/antiox12030721
Arnold, S. E., Arvanitakis, Z., Macauley-Rambach, S. L., Koenig, A. M., Wang, H.-Y., Ahima, R. S., et al. (2018). Brain insulin resistance in type 2 diabetes and Alzheimer disease: concepts and conundrums. Nat. Rev. Neurol. 14 (3), 168–181. doi:10.1038/nrneurol.2017.185
Arriola Apelo, S. I., and Lamming, D. W. (2016). Rapamycin: an InhibiTOR of aging emerges from the soil of easter island. J. Gerontol. A Biol. Sci. Med. Sci. 71 (7), 841–849. doi:10.1093/gerona/glw090
Babiarz, J. E., Ruby, J. G., Wang, Y., Bartel, D. P., and Blelloch, R. (2008). Mouse ES cells express endogenous shRNAs, siRNAs, and other Microprocessor-independent, Dicer-dependent small RNAs. Genes Dev. 22 (20), 2773–2785. doi:10.1101/gad.1705308
Bacalini, M. G., Friso, S., Olivieri, F., Pirazzini, C., Giuliani, C., Capri, M., et al. (2014). Present and future of anti-ageing epigenetic diets. Mech. Ageing Dev. 136-137, 101–115. doi:10.1016/j.mad.2013.12.006
Bagge, A., Clausen, T. R., Larsen, S., Ladefoged, M., Rosenstierne, M. W., Larsen, L., et al. (2012). MicroRNA-29a is up-regulated in beta-cells by glucose and decreases glucose-stimulated insulin secretion. Biochem. Biophysical Res. Commun. 426 (2), 266–272. doi:10.1016/j.bbrc.2012.08.082
Bakr, M., Abd-Elmawla, M. A., Elimam, H., Gamal El-Din, H., Fawzy, A., Abulsoud, A. I., et al. (2023). Telomerase RNA component lncRNA as potential diagnostic biomarker promotes CRC cellular migration and apoptosis evasion via modulation of β-catenin protein level. Non-coding RNA Res. 8 (3), 302–314. doi:10.1016/j.ncrna.2023.03.004
Balaskas, P., Goljanek-Whysall, K., Clegg, P. D., Fang, Y., Cremers, A., Smagul, A., et al. (2023). MicroRNA signatures in cartilage ageing and osteoarthritis. Biomedicines 11 (4), 1189. doi:10.3390/biomedicines11041189
Bandakinda, M., and Mishra, A. (2023). Insights into role of microRNA in Alzheimer's disease: from contemporary research to bedside perspective. Int. J. Biol. Macromol. 253, 126561. doi:10.1016/j.ijbiomac.2023.126561
Bartel, D. P. (2004). MicroRNAs: genomics, biogenesis, mechanism, and function. Cell 116 (2), 281–297. doi:10.1016/s0092-8674(04)00045-5
Basisty, N., Kale, A., Jeon, O. H., Kuehnemann, C., Payne, T., Rao, C., et al. (2020). A proteomic atlas of senescence-associated secretomes for aging biomarker development. PLoS Biol. 18 (1), e3000599. doi:10.1371/journal.pbio.3000599
Baugh, L. R., and Sternberg, P. W. (2006). DAF-16/FOXO regulates transcription of cki-1/Cip/Kip and repression of lin-4 during C. elegans L1 arrest. Curr. Biol. 16 (8), 780–785. doi:10.1016/j.cub.2006.03.021
Beavers, K. R., Nelson, C. E., and Duvall, C. L. (2015). MiRNA inhibition in tissue engineering and regenerative medicine. Adv. Drug Deliv. Rev. 88, 123–137. doi:10.1016/j.addr.2014.12.006
Beckett, E. L., Yates, Z., Veysey, M., Duesing, K., and Lucock, M. (2014). The role of vitamins and minerals in modulating the expression of microRNA. Nutr. Res. Rev. 27 (1), 94–106. doi:10.1017/S0954422414000043
Bellinger, F. P., Raman, A. V., Reeves, M. A., and Berry, M. J. (2009). Regulation and function of selenoproteins in human disease. Biochem. J. 422 (1), 11–22. doi:10.1042/bj20090219
Berber, P., Grassmann, F., Kiel, C., and Weber, B. H. (2017). An eye on age-related macular degeneration: the role of MicroRNAs in disease pathology. Mol. Diagn Ther. 21 (1), 31–43. doi:10.1007/s40291-016-0234-z
Birch, J., and Gil, J. (2020). Senescence and the SASP: many therapeutic avenues. Genes Dev. 34 (23-24), 1565–1576. doi:10.1101/gad.343129.120
Boehm, M., and Slack, F. (2005). A developmental timing microRNA and its target regulate life span in C. elegans. Science 310 (5756), 1954–1957. doi:10.1126/science.1115596
Boesch-Saadatmandi, C., Loboda, A., Wagner, A. E., Stachurska, A., Jozkowicz, A., Dulak, J., et al. (2011). Effect of quercetin and its metabolites isorhamnetin and quercetin-3-glucuronide on inflammatory gene expression: role of miR-155. J. Nutr. Biochem. 22 (3), 293–299. doi:10.1016/j.jnutbio.2010.02.008
Boesch-Saadatmandi, C., Wagner, A. E., Wolffram, S., and Rimbach, G. (2012). Effect of quercetin on inflammatory gene expression in mice liver in vivo - role of redox factor 1, miRNA-122 and miRNA-125b. Pharmacol. Res. 65 (5), 523–530. doi:10.1016/j.phrs.2012.02.007
Boettger, T., Beetz, N., Kostin, S., Schneider, J., Krüger, M., Hein, L., et al. (2009). Acquisition of the contractile phenotype by murine arterial smooth muscle cells depends on the Mir143/145 gene cluster. J. Clin. Invest. 119 (9), 2634–2647. doi:10.1172/jci38864
Bryan, J., Calvaresi, E., and Hughes, D. (2002). Short-term folate, vitamin B-12 or vitamin B-6 supplementation slightly affects memory performance but not mood in women of various ages. J. Nutr. 132 (6), 1345–1356. doi:10.1093/jn/132.6.1345
Campagna, J., Spilman, P., Jagodzinska, B., Bai, D., Hatami, A., Zhu, C., et al. (2018). A small molecule ApoE4-targeted therapeutic candidate that normalizes sirtuin 1 levels and improves cognition in an Alzheimer's disease mouse model. Sci. Rep. 8 (1), 17574. doi:10.1038/s41598-018-35687-8
Campisi, J. (2005). Senescent cells, tumor suppression, and organismal aging: good citizens, bad neighbors. Cell 120 (4), 513–522. doi:10.1016/j.cell.2005.02.003
Cani, P. D., Amar, J., Iglesias, M. A., Poggi, M., Knauf, C., Bastelica, D., et al. (2007). Metabolic endotoxemia initiates obesity and insulin resistance. Diabetes 56 (7), 1761–1772. doi:10.2337/db06-1491
Carlomosti, F., D'Agostino, M., Beji, S., Torcinaro, A., Rizzi, R., Zaccagnini, G., et al. (2017). Oxidative stress-induced miR-200c disrupts the regulatory loop among SIRT1, FOXO1, and eNOS. Antioxid. Redox Signal 27 (6), 328–344. doi:10.1089/ars.2016.6643
Castanheira, C. I., Anderson, J. R., Fang, Y., Milner, P. I., Goljanek-Whysall, K., House, L., et al. (2021). Mouse microRNA signatures in joint ageing and post-traumatic osteoarthritis. Osteoarthr. Cartil. Open 3 (4), 100186. doi:10.1016/j.ocarto.2021.100186
Castell-Auví, A., Cedó, L., Movassat, J., Portha, B., Sánchez-Cabo, F., Pallarès, V., et al. (2013). Procyanidins modulate MicroRNA expression in pancreatic islets. J. Agric. Food Chem. 61 (2), 355–363. doi:10.1021/jf303972f
Cevenini, E., Monti, D., and Franceschi, C. (2013). Inflamm-ageing. Curr. Opin. Clin. Nutr. Metab. Care 16 (1), 14–20. doi:10.1097/MCO.0b013e32835ada13
Cha, D. J., Mengel, D., Mustapic, M., Liu, W., Selkoe, D. J., Kapogiannis, D., et al. (2019). miR-212 and miR-132 are downregulated in neurally derived plasma exosomes of Alzheimer’s patients. Front. Neurosci. 13, 1208. doi:10.3389/fnins.2019.01208
Chartoumpekis, D. V., Zaravinos, A., Ziros, P. G., Iskrenova, R. P., Psyrogiannis, A. I., Kyriazopoulou, V. E., et al. (2012). Differential expression of microRNAs in adipose tissue after long-term high-fat diet-induced obesity in mice. PLoS One 7 (4), e34872. doi:10.1371/journal.pone.0034872
Chellappa, K., Brinkman, J. A., Mukherjee, S., Morrison, M., Alotaibi, M. I., Carbajal, K. A., et al. (2019). Hypothalamic mTORC2 is essential for metabolic health and longevity. Aging Cell 18 (5), e13014. doi:10.1111/acel.13014
Cheloufi, S., Dos Santos, C. O., Chong, M. M., and Hannon, G. J. (2010). A dicer-independent miRNA biogenesis pathway that requires Ago catalysis. Nature 465 (7298), 584–589. doi:10.1038/nature09092
Chen, D., Xiang, M., Gong, Y., Xu, L., Zhang, T., He, Y., et al. (2019). LIPUS promotes FOXO1 accumulation by downregulating miR-182 to enhance osteogenic differentiation in hPDLCs. Biochimie 165, 219–228. doi:10.1016/j.biochi.2019.08.005
Chen, L., Wang, Q., Wang, G. D., Wang, H. S., Huang, Y., Liu, X. M., et al. (2013). miR-16 inhibits cell proliferation by targeting IGF1R and the Raf1-MEK1/2-ERK1/2 pathway in osteosarcoma. FEBS Lett. 587 (9), 1366–1372. doi:10.1016/j.febslet.2013.03.007
Chen, Z., Huai, Y., Chen, G., Liu, S., Zhang, Y., Li, D., et al. (2022). MiR-138-5p targets MACF1 to aggravate aging-related bone loss. Int. J. Biol. Sci. 18 (13), 4837–4852. doi:10.7150/ijbs.71411
Chistiakov, D. A., Sobenin, I. A., Orekhov, A. N., and Bobryshev, Y. V. (2015). Human miR-221/222 in physiological and atherosclerotic vascular remodeling. Biomed. Res. Int. 2015, 354517. doi:10.1155/2015/354517
Condrat, C. E., Thompson, D. C., Barbu, M. G., Bugnar, O. L., Boboc, A., Cretoiu, D., et al. (2020). miRNAs as biomarkers in disease: latest findings regarding their role in diagnosis and prognosis. Cells 9 (2), 276. doi:10.3390/cells9020276
Cook, H. W. (1908). The cardiovascular system in prognosis.: with special reference to life insurance examinations. J. Am. Med. Assoc. LI (20), 1692–1694. doi:10.1001/jama.1908.25410200040001i
Cooks, T., Pateras, I. S., Jenkins, L. M., Patel, K. M., Robles, A. I., Morris, J., et al. (2018). Mutant p53 cancers reprogram macrophages to tumor supporting macrophages via exosomal miR-1246. Nat. Commun. 9 (1), 771. doi:10.1038/s41467-018-03224-w
Coppe, J. P., Patil, C. K., Rodier, F., Sun, Y., Munoz, D. P., Goldstein, J., et al. (2008). Senescence-associated secretory phenotypes reveal cell-nonautonomous functions of oncogenic RAS and the p53 tumor suppressor. PLoS Biol. 6 (12), 2853–2868. doi:10.1371/journal.pbio.0060301
Cornel, S., Adriana, I. D., Mihaela, T. C., Speranta, S., Algerino, S., Mehdi, B., et al. (2015). Anti-vascular endothelial growth factor indications in ocular disease. Rom. J. Ophthalmol. 59 (4), 235–242.
Cruz-Aguilar, M., Groman-Lupa, S., and Jiménez-Martínez, M. C. (2023). MicroRNAs as potential biomarkers and therapeutic targets in age-related macular degeneration. Front. Ophthalmol. 3, 5. doi:10.3389/fopht.2023.1023782
Csiszar, A., Gautam, T., Sosnowska, D., Tarantini, S., Banki, E., Tucsek, Z., et al. (2014). Caloric restriction confers persistent anti-oxidative, pro-angiogenic, and anti-inflammatory effects and promotes anti-aging miRNA expression profile in cerebromicrovascular endothelial cells of aged rats. Am. J. Physiol. Heart Circ. Physiol. 307 (3), H292–H306. doi:10.1152/ajpheart.00307.2014
Cui, M., Wang, H., Yao, X., Zhang, D., Xie, Y., Cui, R., et al. (2019). Circulating MicroRNAs in cancer: potential and challenge. Front. Genet. 10, 626. doi:10.3389/fgene.2019.00626
Dakterzada, F., David Benítez, I., Targa, A., Lladó, A., Torres, G., Romero, L., et al. (2021). Reduced levels of miR-342-5p in plasma are associated with worse cognitive evolution in patients with mild Alzheimer’s disease. Front. Aging Neurosci. 13, 705989. doi:10.3389/fnagi.2021.705989
Dambal, S., Giangreco, A. A., Acosta, A. M., Fairchild, A., Richards, Z., Deaton, R., et al. (2017). microRNAs and DICER1 are regulated by 1,25-dihydroxyvitamin D in prostate stroma. J. Steroid Biochem. Mol. Biol. 167, 192–202. doi:10.1016/j.jsbmb.2017.01.004
Dauncey, M. J. (2009). New insights into nutrition and cognitive neuroscience. Proc. Nutr. Soc. 68 (4), 408–415. doi:10.1017/s0029665109990188
Davalos, A. R., Kawahara, M., Malhotra, G. K., Schaum, N., Huang, J., Ved, U., et al. (2013). p53-dependent release of Alarmin HMGB1 is a central mediator of senescent phenotypes. J. Cell Biol. 201 (4), 613–629. doi:10.1083/jcb.201206006
de Lucia, C., Komici, K., Borghetti, G., Femminella, G. D., Bencivenga, L., Cannavo, A., et al. (2017). microRNA in cardiovascular aging and age-related cardiovascular diseases. Front. Med. 4, 74. doi:10.3389/fmed.2017.00074
Denli, A. M., Tops, B. B., Plasterk, R. H., Ketting, R. F., and Hannon, G. J. (2004). Processing of primary microRNAs by the Microprocessor complex. Nature 432 (7014), 231–235. doi:10.1038/nature03049
de Siqueira, K. C., de Lima, F. M., Lima, F. S., Taki, M. S., da Cunha, C. F., de Lima Reis, S. R., et al. (2018). miR-124a expression contributes to the monophasic pattern of insulin secretion in islets from pregnant rats submitted to a low-protein diet. Eur. J. Nutr. 57 (4), 1471–1483. doi:10.1007/s00394-017-1425-z
Dey, N., Das, F., Mariappan, M. M., Mandal, C. C., Ghosh-Choudhury, N., Kasinath, B. S., et al. (2011). MicroRNA-21 orchestrates high glucose-induced signals to TOR complex 1, resulting in renal cell pathology in diabetes. J. Biol. Chem. 286 (29), 25586–25603. doi:10.1074/jbc.M110.208066
Díaz-Prado, S., Cicione, C., Muiños-López, E., Hermida-Gómez, T., Oreiro, N., Fernández-López, C., et al. (2012). Characterization of microRNA expression profiles in normal and osteoarthritic human chondrocytes. BMC Musculoskelet. Disord. 13 (1), 144. doi:10.1186/1471-2474-13-144
Dimmeler, S., and Nicotera, P. (2013). MicroRNAs in age-related diseases. EMBO Mol. Med. 5 (2), 180–190. doi:10.1002/emmm.201201986
Ding, H., Chen, S., Pan, X., Dai, X., Pan, G., Li, Z., et al. (2021). Transferrin receptor 1 ablation in satellite cells impedes skeletal muscle regeneration through activation of ferroptosis. J. Cachexia Sarcopenia Muscle 12 (3), 746–768. doi:10.1002/jcsm.12700
Drummond, M. J., McCarthy, J. J., Sinha, M., Spratt, H. M., Volpi, E., Esser, K. A., et al. (2011). Aging and microRNA expression in human skeletal muscle: a microarray and bioinformatics analysis. Physiol. Genomics 43 (10), 595–603. doi:10.1152/physiolgenomics.00148.2010
Ebert, M. S., and Sharp, P. A. (2010). MicroRNA sponges: progress and possibilities. Rna 16 (11), 2043–2050. doi:10.1261/rna.2414110
Elbay, A., Ercan, Ç., Akbaş, F., Bulut, H., and Ozdemir, H. (2019). Three new circulating microRNAs may be associated with wet age-related macular degeneration. Scand. J. Clin. Lab. Invest. 79 (6), 388–394. doi:10.1080/00365513.2019.1637931
Ellis, K. L., Cameron, V. A., Troughton, R. W., Frampton, C. M., Ellmers, L. J., and Richards, A. M. (2013). Circulating microRNAs as candidate markers to distinguish heart failure in breathless patients. Eur. J. Heart Fail 15 (10), 1138–1147. doi:10.1093/eurjhf/hft078
ElSharawy, A., Keller, A., Flachsbart, F., Wendschlag, A., Jacobs, G., Kefer, N., et al. (2012). Genome-wide miRNA signatures of human longevity. Aging Cell 11 (4), 607–616. doi:10.1111/j.1474-9726.2012.00824.x
El-Sheikh, N. M., Abulsoud, A. I., Fawzy, A., Wasfey, E. F., and Hamdy, N. M. (2023). LncRNA NNT-AS1/hsa-miR-485–5p/HSP90 axis in-silico and clinical prospect correlated-to histologic grades-based CRC stratification: a step toward ncRNA Precision. Pathology - Res. Pract. 247, 154570. doi:10.1016/j.prp.2023.154570
El-Sheikh, N. M., Abulsoud, A. I., Wasfey, E. F., and Hamdy, N. M. (2022). Insights on the potential oncogenic impact of long non-coding RNA nicotinamide nucleotide transhydrogenase antisense RNA 1 in different cancer types; integrating pathway(s) and clinical outcome(s) association. Pathol. Res. Pract. 240, 154183. doi:10.1016/j.prp.2022.154183
ElShelmani, H., Wride, M. A., Saad, T., Rani, S., Kelly, D. J., and Keegan, D. (2020). Identification of novel serum microRNAs in age-related macular degeneration. Transl. Vis. Sci. Technol. 9 (4), 28. doi:10.1167/tvst.9.4.28
ElShelmani, H., Wride, M. A., Saad, T., Rani, S., Kelly, D. J., and Keegan, D. (2021a). The role of deregulated MicroRNAs in age-related macular degeneration pathology. Transl. Vis. Sci. Technol. 10 (2), 12. doi:10.1167/tvst.10.2.12
ElShelmani, H., Wride, M. A., Saad, T., Rani, S., Kelly, D. J., and Keegan, D. (2021b). The role of deregulated MicroRNAs in age-related macular degeneration pathology. Transl. Vis. Sci. Technol. 10 (2), 12. doi:10.1167/tvst.10.2.12
Erturk, E., Onur, O. E., Akgun, O., Tuna, G. P., Yildiz, Y., and Ari, F. (2022). Mitochondrial miRNAs (MitomiRs): their potential roles in breast and other cancers. Mitochondrion 66, 74–81. doi:10.1016/j.mito.2022.08.002
Eshkoor, S. A., Ghodsian, N., and Akhtari-Zavare, M. (2022). MicroRNAs influence and longevity. Egypt. J. Med. Hum. Genet. 23 (1), 105–116. doi:10.1186/s43042-022-00316-7
Fairchild, C. L. A., Cheema, S. K., Wong, J., Hino, K., Simó, S., and La Torre, A. (2019). Let-7 regulates cell cycle dynamics in the developing cerebralcortex and retina. Sci. Rep. 9 (1), 15336. doi:10.1038/s41598-019-51703-x
Fehlmann, T., Lehallier, B., Schaum, N., Hahn, O., Kahraman, M., Li, Y., et al. (2020). Common diseases alter the physiological age-related blood microRNA profile. Nat. Commun. 11 (1), 5958. doi:10.1038/s41467-020-19665-1
Fong, L. Y., Taccioli, C., Jing, R., Smalley, K. J., Alder, H., Jiang, Y., et al. (2016). MicroRNA dysregulation and esophageal cancer development depend on the extent of zinc dietary deficiency. Oncotarget 7 (10), 10723–10738. doi:10.18632/oncotarget.7561
Franceschi, C. (2007). Inflammaging as a major characteristic of old people: can it be prevented or cured? Nutr. Rev. 65 (12 Pt 2), S173–S176. doi:10.1111/j.1753-4887.2007.tb00358.x
Franceschi, C., Bonafe, M., Valensin, S., Olivieri, F., De Luca, M., Ottaviani, E., et al. (2000). Inflamm-aging. An evolutionary perspective on immunosenescence. Ann. N. Y. Acad. Sci. 908, 244–254. doi:10.1111/j.1749-6632.2000.tb06651.x
Fredman, G., Li, Y., Dalli, J., Chiang, N., and Serhan, C. N. (2012). Self-limited versus delayed resolution of acute inflammation: temporal regulation of pro-resolving mediators and microRNA. Sci. Rep. 2, 639. doi:10.1038/srep00639
Friedman, D. B., and Johnson, T. E. (1988). A mutation in the age-1 gene in Caenorhabditis elegans lengthens life and reduces hermaphrodite fertility. Genetics 118 (1), 75–86. doi:10.1093/genetics/118.1.75
Fu, C.-H., Han, X.-Y., Tong, L., Nie, P.-Y., Hu, Y.-D., and Ji, L.-L. (2021). miR-142 downregulation alleviates the impairment of spatial learning and memory, reduces the level of apoptosis, and upregulates the expression of pCaMKII and Bai3 in the hippocampus of APP/PS1 transgenic mice. Behav. Brain Res. 414, 113485. doi:10.1016/j.bbr.2021.113485
Gabunia, K., Herman, A. B., Ray, M., Kelemen, S. E., England, R. N., Cadena, R. D., et al. (2017). Induction of MiR133a expression by IL-19 targets LDLRAP1 and reduces oxLDL uptake in VSMC. J. Mol. Cell. Cardiol. 105, 38–48. doi:10.1016/j.yjmcc.2017.02.005
Gaidai, O., Cao, Y., and Loginov, S. (2023). Global cardiovascular diseases death rate prediction. Curr. Probl. Cardiol. 48 (5), 101622. doi:10.1016/j.cpcardiol.2023.101622
Gholaminejad, A., Zare, N., Dana, N., Shafie, D., Mani, A., and Javanmard, S. H. (2021). A meta-analysis of microRNA expression profiling studies in heart failure. Heart Fail Rev. 26 (4), 997–1021. doi:10.1007/s10741-020-10071-9
Ghosh, S., Kumar, V., Mukherjee, H., Lahiri, D., and Roy, P. (2021). Nutraceutical regulation of miRNAs involved in neurodegenerative diseases and brain cancers. Heliyon 7 (6), e07262. doi:10.1016/j.heliyon.2021.e07262
Giangreco, A. A., Vaishnav, A., Wagner, D., Finelli, A., Fleshner, N., Van der Kwast, T., et al. (2013). Tumor suppressor microRNAs, miR-100 and -125b, are regulated by 1,25-dihydroxyvitamin D in primary prostate cells and in patient tissue. Cancer Prev. Res. (Phila) 6 (5), 483–494. doi:10.1158/1940-6207.CAPR-12-0253
Goedeke, L., Salerno, A., Ramírez, C. M., Guo, L., Allen, R. M., Yin, X., et al. (2014). Long-term therapeutic silencing of miR-33 increases circulating triglyceride levels and hepatic lipid accumulation in mice. EMBO Mol. Med. 6 (9), 1133–1141. doi:10.15252/emmm.201404046
Gomez-Pinilla, F., and Nguyen, T. T. (2012). Natural mood foods: the actions of polyphenols against psychiatric and cognitive disorders. Nutr. Neurosci. 15 (3), 127–133. doi:10.1179/1476830511y.0000000035
Grillari, J., Hackl, M., and Grillari-Voglauer, R. (2010). miR-17-92 cluster: ups and downs in cancer and aging. Biogerontology 11 (4), 501–506. doi:10.1007/s10522-010-9272-9
Guertin, D. A., Stevens, D. M., Thoreen, C. C., Burds, A. A., Kalaany, N. Y., Moffat, J., et al. (2006). Ablation in mice of the mTORC components raptor, rictor, or mLST8 reveals that mTORC2 is required for signaling to Akt-FOXO and PKCalpha, but not S6K1. Dev. Cell 11 (6), 859–871. doi:10.1016/j.devcel.2006.10.007
Gulyaeva, L. F., and Kushlinskiy, N. E. (2016). Regulatory mechanisms of microRNA expression. J. Transl. Med. 14 (1), 143. doi:10.1186/s12967-016-0893-x
Gurau, F., Baldoni, S., Prattichizzo, F., Espinosa, E., Amenta, F., Procopio, A. D., et al. (2018). Anti-senescence compounds: a potential nutraceutical approach to healthy aging. Ageing Res. Rev. 46, 14–31. doi:10.1016/j.arr.2018.05.001
Han, L., Wang, P., Zhao, G., Wang, H., Wang, M., Chen, J., et al. (2013). Upregulation of SIRT1 by 17β-estradiol depends on ubiquitin-proteasome degradation of PPAR-γ mediated by NEDD4-1. Protein Cell 4 (4), 310–321. doi:10.1007/s13238-013-2124-z
Han, X., Tai, H., Wang, X., Wang, Z., Zhou, J., Wei, X., et al. (2016). AMPK activation protects cells from oxidative stress-induced senescence via autophagic flux restoration and intracellular NAD(+) elevation. Aging Cell 15 (3), 416–427. doi:10.1111/acel.12446
Hanna, J., Hossain, G. S., and Kocerha, J. (2019). The potential for microRNA therapeutics and clinical research. Front. Genet. 10, 478. doi:10.3389/fgene.2019.00478
Haslberger, A., Lilja, S., Bäck, H., Stoll, C., Mayer, A., Pointner, A., et al. (2021). Increased Sirtuin expression, senescence regulating miRNAs, mtDNA, and Bifidobateria correlate with wellbeing and skin appearance after Sirtuin-activating drink. Bioact. Compd. Health Dis. 4 (4), 45–62. doi:10.31989/bchd.v4i4.801
Hasler, D., Lehmann, G., Murakawa, Y., Klironomos, F., Jakob, L., Grässer, F. A., et al. (2016). The lupus autoantigen La prevents mis-channeling of tRNA fragments into the human MicroRNA pathway. Mol. Cell 63 (1), 110–124. doi:10.1016/j.molcel.2016.05.026
Heng, W., Raymond, N., Xin, C., Clifford, J. S., and Guisheng, S. (2016). MicroRNA-21 is a potential link between non-alcoholic fatty liver disease and hepatocellular carcinoma via modulation of the HBP1-p53-Srebp1c pathway. Gut 65 (11), 1850–1860. doi:10.1136/gutjnl-2014-308430
Hernández-Alonso, P., Giardina, S., Salas-Salvadó, J., Arcelin, P., and Bulló, M. (2017). Chronic pistachio intake modulates circulating microRNAs related to glucose metabolism and insulin resistance in prediabetic subjects. Eur. J. Nutr. 56 (6), 2181–2191. doi:10.1007/s00394-016-1262-5
Herrera-Carrillo, E., and Berkhout, B. (2017). Dicer-independent processing of small RNA duplexes: mechanistic insights and applications. Nucleic Acids Res. 45 (18), 10369–10379. doi:10.1093/nar/gkx779
Hill, J. M., Pogue, A. I., and Lukiw, W. J. (2015). Pathogenic microRNAs common to brain and retinal degeneration; recent observations in Alzheimer's disease and age-related macular degeneration. Front. Neurol. 6, 232. doi:10.3389/fneur.2015.00232
Huang, X. Y., Chen, J. X., Ren, Y., Fan, L. C., Xiang, W., and He, X. J. (2022). Exosomal miR-122 promotes adipogenesis and aggravates obesity through the VDR/SREBF1 axis. Obesity 30 (3), 666–679. doi:10.1002/oby.23365
Ismail, A., Abulsoud, A. I., Mansour, O. A., and Fawzy, A. (2019). Diagnostic significance of miR-639 and miR-10b in βreast cancer patients. Meta Gene 19, 155–159. doi:10.1016/j.mgene.2018.11.006
Jiao, J., Feng, G., Wu, M., Wang, Y., Li, R., and Liu, J. (2020). MiR-140-5p promotes osteogenic differentiation of mouse embryonic bone marrow mesenchymal stem cells and post-fracture healing of mice. Cell Biochem. Funct. 38 (8), 1152–1160. doi:10.1002/cbf.3585
Johnson, J. L. (2019). Elucidating the contributory role of microRNA to cardiovascular diseases (a review). Vasc. Pharmacol. 114, 31–48. doi:10.1016/j.vph.2018.10.010
Johnson, S. C., Rabinovitch, P. S., and Kaeberlein, M. (2013). mTOR is a key modulator of ageing and age-related disease. Nature 493 (7432), 338–345. doi:10.1038/nature11861
Jung, H. J., and Suh, Y. (2014). Regulation of IGF -1 signaling by microRNAs. Front. Genet. 5, 472. doi:10.3389/fgene.2014.00472
Kaeberlein, M., McVey, M., and Guarente, L. (1999). The SIR2/3/4 complex and SIR2 alone promote longevity in Saccharomyces cerevisiae by two different mechanisms. Genes Dev. 13 (19), 2570–2580. doi:10.1101/gad.13.19.2570
Kaeberlein, M., Powers, R. W., Steffen, K. K., Westman, E. A., Hu, D., Dang, N., et al. (2005). Regulation of yeast replicative life span by TOR and Sch9 in response to nutrients. Science 310 (5751), 1193–1196. doi:10.1126/science.1115535
Kanfi, Y., Naiman, S., Amir, G., Peshti, V., Zinman, G., Nahum, L., et al. (2012). The sirtuin SIRT6 regulates lifespan in male mice. Nature 483 (7388), 218–221. doi:10.1038/nature10815
Kapahi, P., Zid, B. M., Harper, T., Koslover, D., Sapin, V., and Benzer, S. (2004). Regulation of lifespan in Drosophila by modulation of genes in the TOR signaling pathway. Curr. Biol. 14 (10), 885–890. doi:10.1016/j.cub.2004.03.059
Karkeni, E., Bonnet, L., Marcotorchino, J., Tourniaire, F., Astier, J., Ye, J., et al. (2018). Vitamin D limits inflammation-linked microRNA expression in adipocytes in vitro and in vivo: a new mechanism for the regulation of inflammation by vitamin D. Epigenetics 13 (2), 156–162. doi:10.1080/15592294.2016.1276681
Karolina, D. S., Tavintharan, S., Armugam, A., Sepramaniam, S., Pek, S. L., Wong, M. T., et al. (2012). Circulating miRNA profiles in patients with metabolic syndrome. J. Clin. Endocrinol. Metab. 97 (12), E2271–E2276. doi:10.1210/jc.2012-1996
Kayashima, Y., Katayanagi, Y., Tanaka, K., Fukutomi, R., Hiramoto, S., and Imai, S. (2017). Alkylresorcinols activate SIRT1 and delay ageing in Drosophila melanogaster. Sci. Rep. 7, 43679. doi:10.1038/srep43679
Kempinska-Podhorodecka, A., Milkiewicz, M., Wasik, U., Ligocka, J., Zawadzki, M., Krawczyk, M., et al. (2017). Decreased expression of vitamin D receptor affects an immune response in primary biliary cholangitis via the VDR-miRNA155-SOCS1 pathway. Int. J. Mol. Sci. 18 (2), 289. doi:10.3390/ijms18020289
Kenyon, C., Chang, J., Gensch, E., Rudner, A., and Tabtiang, R. (1993). A C. elegans mutant that lives twice as long as wild type. Nature 366 (6454), 461–464. doi:10.1038/366461a0
Khan, M., Ullah, R., Rehman, S. U., Shah, S. A., Saeed, K., Muhammad, T., et al. (2019). 17β-Estradiol modulates SIRT1 and halts oxidative stress-mediated cognitive impairment in a male aging mouse model. Cells 8 (8), 928. doi:10.3390/cells8080928
Khan, S., Wall, D., Curran, C., Newell, J., Kerin, M. J., and Dwyer, R. M. (2015). MicroRNA-10a is reduced in breast cancer and regulated in part through retinoic acid. BMC Cancer 15, 345. doi:10.1186/s12885-015-1374-y
Kim, J., Kang, Y. G., Lee, J. Y., Choi, D. H., Cho, Y. U., Shin, J. M., et al. (2015). The natural phytochemical dehydroabietic acid is an anti-aging reagent that mediates the direct activation of SIRT1. Mol. Cell Endocrinol. 412, 216–225. doi:10.1016/j.mce.2015.05.006
Kinser, H. E., and Pincus, Z. (2020). MicroRNAs as modulators of longevity and the aging process. Hum. Genet. 139 (3), 291–308. doi:10.1007/s00439-019-02046-0
Kirkpatrick, C. F., and Maki, K. C. (2021). Dietary influences on atherosclerotic cardiovascular disease risk. Curr. Atheroscler. Rep. 23 (10), 62. doi:10.1007/s11883-021-00954-z
Kiss, T., Giles, C. B., Tarantini, S., Yabluchanskiy, A., Balasubramanian, P., Gautam, T., et al. (2019). Nicotinamide mononucleotide (NMN) supplementation promotes anti-aging miRNA expression profile in the aorta of aged mice, predicting epigenetic rejuvenation and anti-atherogenic effects. Geroscience 41 (4), 419–439. doi:10.1007/s11357-019-00095-x
Klimczak, D., Jazdzewski, K., and Kuch, M. (2017). Regulatory mechanisms in arterial hypertension: role of microRNA in pathophysiology and therapy. Blood Press. 26 (1), 2–8. doi:10.3109/08037051.2016.1167355
Kocic, H., Damiani, G., Stamenkovic, B., Tirant, M., Jovic, A., Tiodorovic, D., et al. (2019). Dietary compounds as potential modulators of microRNA expression in psoriasis. Ther. Adv. Chronic Dis. 10, 2040622319864805. doi:10.1177/2040622319864805
Kohlstedt, K., Trouvain, C., Boettger, T., Shi, L., Fisslthaler, B., and Fleming, I. (2013). AMP-activated protein kinase regulates endothelial cell angiotensin-converting enzyme expression via p53 and the post-transcriptional regulation of microRNA-143/145. Circ. Res. 112 (8), 1150–1158. doi:10.1161/circresaha.113.301282
Kontaraki, J. E., Marketou, M. E., Zacharis, E. A., Parthenakis, F. I., and Vardas, P. E. (2014). MicroRNA-9 and microRNA-126 expression levels in patients with essential hypertension: potential markers of target-organ damage. J. Am. Soc. Hypertens. 8 (6), 368–375. doi:10.1016/j.jash.2014.03.324
Koyama, S., Horie, T., Nishino, T., Baba, O., Sowa, N., Miyasaka, Y., et al. (2019). Identification of differential roles of MicroRNA-33a and -33b during atherosclerosis progression with genetically modified mice. J. Am. Heart Assoc. 8 (13), e012609. doi:10.1161/jaha.119.012609
Krishnamoorthy, S., Recchiuti, A., Chiang, N., Fredman, G., and Serhan, C. N. (2012). Resolvin D1 receptor stereoselectivity and regulation of inflammation and proresolving microRNAs. Am. J. Pathol. 180 (5), 2018–2027. doi:10.1016/j.ajpath.2012.01.028
Kronski, E., Fiori, M. E., Barbieri, O., Astigiano, S., Mirisola, V., Killian, P. H., et al. (2014). miR181b is induced by the chemopreventive polyphenol curcumin and inhibits breast cancer metastasis via down-regulation of the inflammatory cytokines CXCL1 and -2. Mol. Oncol. 8 (3), 581–595. doi:10.1016/j.molonc.2014.01.005
Kulkarni, A. S., Gubbi, S., and Barzilai, N. (2020). Benefits of metformin in attenuating the hallmarks of aging. Cell Metab. 32 (1), 15–30. doi:10.1016/j.cmet.2020.04.001
Kurylowicz, A., Owczarz, M., Polosak, J., Jonas, M. I., Lisik, W., Jonas, M., et al. (2016). SIRT1 and SIRT7 expression in adipose tissues of obese and normal-weight individuals is regulated by microRNAs but not by methylation status. Int. J. Obes. (Lond) 40 (11), 1635–1642. doi:10.1038/ijo.2016.131
Laconi, E., Marongiu, F., and DeGregori, J. (2020). Cancer as a disease of old age: changing mutational and microenvironmental landscapes. Br. J. Cancer 122 (7), 943–952. doi:10.1038/s41416-019-0721-1
Lai, W.-F., Lin, M., and Wong, W.-T. (2019). Tackling aging by using miRNA as a target and a tool. Trends Mol. Med. 25 (8), 673–684. doi:10.1016/j.molmed.2019.04.007
Langenberger, D., Çakir, M. V., Hoffmann, S., and Stadler, P. F. (2013). Dicer-processed small RNAs: rules and exceptions. J. Exp. Zool. B Mol. Dev. Evol. 320 (1), 35–46. doi:10.1002/jez.b.22481
Latruffe, N., Lançon, A., Frazzi, R., Aires, V., Delmas, D., Michaille, J. J., et al. (2015). Exploring new ways of regulation by resveratrol involving miRNAs, with emphasis on inflammation. Ann. N. Y. Acad. Sci. 1348 (1), 97–106. doi:10.1111/nyas.12819
Lauria, F., and Iacomino, G. (2021). The landscape of circulating miRNAs in the post-genomic era. Genes (Basel) 13 (1), 94. doi:10.3390/genes13010094
Leitão, C., Mignano, A., Estrela, M., Fardilha, M., Figueiras, A., Roque, F., et al. (2022). The effect of nutrition on aging-A systematic review focusing on aging-related biomarkers. Nutrients 14 (3), 554. doi:10.3390/nu14030554
Li, C., Dou, P., Wang, T., Lu, X., Xu, G., and Lin, X. (2022). Defining disease-related modules based on weighted miRNA synergistic network. Comput. Biol. Med. 152, 106382. doi:10.1016/j.compbiomed.2022.106382
Li, D., Ke, Y., Zhan, R., Liu, C., Zhao, M., Zeng, A., et al. (2018a). Trimethylamine-N-oxide promotes brain aging and cognitive impairment in mice. Aging Cell 17 (4), e12768. doi:10.1111/acel.12768
Li, S., Zhu, J., Zhang, W., Chen, Y., Zhang, K., Popescu, L. M., et al. (2011). Signature microRNA expression profile of essential hypertension and its novel link to human cytomegalovirus infection. Circulation 124 (2), 175–184. doi:10.1161/circulationaha.110.012237
Li, Y., Yang, C., Zhang, L., and Yang, P. (2017). MicroRNA-210 induces endothelial cell apoptosis by directly targeting PDK1 in the setting of atherosclerosis. Cell. Mol. Biol. Lett. 22 (1), 3. doi:10.1186/s11658-017-0033-5
Li, Z., Zhang, W., and Huang, Y. (2018b). MiRNA-133a is involved in the regulation of postmenopausal osteoporosis through promoting osteoclast differentiation. Acta Biochimica Biophysica Sinica 50 (3), 273–280. doi:10.1093/abbs/gmy006
Lim, E., Lim, J. Y., Kim, E., Kim, Y.-S., Shin, J.-H., Seok, P. R., et al. (2016). Xylobiose, an alternative sweetener, ameliorates diabetes-related metabolic changes by regulating hepatic lipogenesis and miR-122a/33a in db/db mice. Nutrients 8 (12), 791. doi:10.3390/nu8120791
Lima, J. F., Cerqueira, L., Figueiredo, C., Oliveira, C., and Azevedo, N. F. (2018). Anti-miRNA oligonucleotides: a comprehensive guide for design. RNA Biol. 15 (3), 338–352. doi:10.1080/15476286.2018.1445959
Lippi, L., Uberti, F., Folli, A., Turco, A., Curci, C., d’Abrosca, F., et al. (2022). Impact of nutraceuticals and dietary supplements on mitochondria modifications in healthy aging: a systematic review of randomized controlled trials. Aging Clin. Exp. Res. 34 (11), 2659–2674. doi:10.1007/s40520-022-02203-y
Liu, C.-G., Zhao, Y., Lu, Y., and Wang, P.-C. (2021). ABCA1-Labeled exosomes in serum contain higher MicroRNA-193b levels in Alzheimer’s disease. BioMed Res. Int. 2021, 5450397. doi:10.1155/2021/5450397
Liu, D., Xu, L., Zhang, X., Shi, C., Qiao, S., Ma, Z., et al. (2019). Snapshot: implications for mTOR in aging-related ischemia/reperfusion injury. Aging Dis. 10 (1), 116–133. doi:10.14336/ad.2018.0501
Liu, H., Yan, L., Li, X., Li, D., Wang, G., Shen, N.-N., et al. (2023). MicroRNA expression in osteoarthritis: a meta-analysis. Clin. Exp. Med. 23, 3737–3749. doi:10.1007/s10238-023-01063-8
Longo, V. D., Antebi, A., Bartke, A., Barzilai, N., Brown-Borg, H. M., Caruso, C., et al. (2015). Interventions to slow aging in humans: are we ready? Aging Cell 14 (4), 497–510. doi:10.1111/acel.12338
López-Otín, C., Blasco, M. A., Partridge, L., Serrano, M., and Kroemer, G. (2013). The hallmarks of aging. Cell 153 (6), 1194–1217. doi:10.1016/j.cell.2013.05.039
Luceri, C., Bigagli, E., Pitozzi, V., and Giovannelli, L. (2017a). A nutrigenomics approach for the study of anti-aging interventions: olive oil phenols and the modulation of gene and microRNA expression profiles in mouse brain. Eur. J. Nutr. 56 (2), 865–877. doi:10.1007/s00394-015-1134-4
Luceri, C., Bigagli, E., Pitozzi, V., and Giovannelli, L. (2017b). A nutrigenomics approach for the study of anti-aging interventions: olive oil phenols and the modulation of gene and microRNA expression profiles in mouse brain. Eur. J. Nutr. 56 (2), 865–877. doi:10.1007/s00394-015-1134-4
Luo, W., Liu, L., Yang, L., Dong, Y., Liu, T., Wei, X., et al. (2018). The vitamin D receptor regulates miR-140-5p and targets the MAPK pathway in bone development. Metabolism 85, 139–150. doi:10.1016/j.metabol.2018.03.018
Ma, M., Wang, X., Chen, X., Cai, R., Chen, F., Dong, W., et al. (2017). MicroRNA-432 targeting E2F3 and P55PIK inhibits myogenesis through PI3K/AKT/mTOR signaling pathway. RNA Biol. 14 (3), 347–360. doi:10.1080/15476286.2017.1279786
Ma, S., Tian, X. Y., Zhang, Y., Mu, C., Shen, H., Bismuth, J., et al. (2016). E-selectin-targeting delivery of microRNAs by microparticles ameliorates endothelial inflammation and atherosclerosis. Sci. Rep. 6 (1), 22910. doi:10.1038/srep22910
Macias, S., Cordiner, R. A., Gautier, P., Plass, M., and Cáceres, J. F. (2015). DGCR8 acts as an adaptor for the exosome complex to degrade double-stranded structured RNAs. Mol. Cell 60 (6), 873–885. doi:10.1016/j.molcel.2015.11.011
Maciel-Dominguez, A., Swan, D., Ford, D., and Hesketh, J. (2013). Selenium alters miRNA profile in an intestinal cell line: evidence that miR-185 regulates expression of GPX2 and SEPSH2. Mol. Nutr. Food Res. 57 (12), 2195–2205. doi:10.1002/mnfr.201300168
Magenta, A., Sileno, S., D'Agostino, M., Persiani, F., Beji, S., Paolini, A., et al. (2018). Atherosclerotic plaque instability in carotid arteries: miR-200c as a promising biomarker. Clin. Sci. (Lond) 132 (22), 2423–2436. doi:10.1042/cs20180684
Mahajan, A., Sapehia, D., Thakur, S., Mohanraj, P. S., Bagga, R., and Kaur, J. (2019). Effect of imbalance in folate and vitamin B12 in maternal/parental diet on global methylation and regulatory miRNAs. Sci. Rep. 9 (1), 17602. doi:10.1038/s41598-019-54070-9
Maher, T. J. (2000). Effects of nutrients on brain function. Prog. Brain Res. 122, 187–194. doi:10.1016/s0079-6123(08)62138-x
Majumder, P., Chanda, K., Das, D., Singh, B. K., Chakrabarti, P., Jana, N. R., et al. (2021). A nexus of miR-1271, PAX4 and ALK/RYK influences the cytoskeletal architectures in Alzheimer's Disease and Type 2 Diabetes. Biochem. J. 478 (17), 3297–3317. doi:10.1042/bcj20210175
Makarev, E., Cantor, C., Zhavoronkov, A., Buzdin, A., Aliper, A., and Csoka, A. B. (2014). Pathway activation profiling reveals new insights into age-related macular degeneration and provides avenues for therapeutic interventions. Aging (Albany NY) 6 (12), 1064–1075. doi:10.18632/aging.100711
Mankhong, S., Kim, S., Moon, S., Choi, S.-H., Kwak, H.-B., Park, D.-H., et al. (2022). Circulating micro-RNAs differentially expressed in Korean Alzheimer’s patients with brain aβ accumulation activate amyloidogenesis. Journals Gerontology Ser. A 78 (2), 292–303. doi:10.1093/gerona/glac106
Mansouri, L., Lundwall, K., Moshfegh, A., Jacobson, S. H., Lundahl, J., and Spaak, J. (2017). Vitamin D receptor activation reduces inflammatory cytokines and plasma MicroRNAs in moderate chronic kidney disease - a randomized trial. BMC Nephrol. 18 (1), 161. doi:10.1186/s12882-017-0576-8
Marques, F. Z., Campain, A. E., Tomaszewski, M., Zukowska-Szczechowska, E., Yang, Y. H., Charchar, F. J., et al. (2011). Gene expression profiling reveals renin mRNA overexpression in human hypertensive kidneys and a role for microRNAs. Hypertension 58 (6), 1093–1098. doi:10.1161/hypertensionaha.111.180729
Martinez, K. B., Leone, V., and Chang, E. B. (2017). Western diets, gut dysbiosis, and metabolic diseases: are they linked? Gut Microbes 8 (2), 130–142. doi:10.1080/19490976.2016.1270811
Martins, R., Lithgow, G. J., and Link, W. (2016). Long live FOXO: unraveling the role of FOXO proteins in aging and longevity. Aging Cell 15 (2), 196–207. doi:10.1111/acel.12427
Matai, L., and Slack, F. J. (2023a). MicroRNAs in age-related proteostasis and stress responses. Noncoding RNA 9 (2), 26. doi:10.3390/ncrna9020026
Matai, L., and Slack, F. J. (2023b). MicroRNAs in age-related proteostasis and stress responses. Noncoding. RNA 9 (2), 26. doi:10.3390/ncrna9020026
McCann, J. C., and Ames, B. N. (2011). Adaptive dysfunction of selenoproteins from the perspective of the triage theory: why modest selenium deficiency may increase risk of diseases of aging. FASEB J. 25 (6), 1793–1814. doi:10.1096/fj.11-180885
Meder, B., Backes, C., Haas, J., Leidinger, P., Stähler, C., Großmann, T., et al. (2014). Influence of the confounding factors age and sex on microRNA profiles from peripheral blood. Clin. Chem. 60 (9), 1200–1208. doi:10.1373/clinchem.2014.224238
Melman, Y. F., Shah, R., Danielson, K., Xiao, J., Simonson, B., Barth, A., et al. (2015). Circulating MicroRNA-30d is associated with response to cardiac resynchronization therapy in heart failure and regulates cardiomyocyte apoptosis: a translational pilot study. Circulation 131 (25), 2202–2216. doi:10.1161/circulationaha.114.013220
Meng, X., Zhou, J., Zhao, C. N., Gan, R. Y., and Li, H. B. (2020). Health benefits and molecular mechanisms of resveratrol: a narrative review. Foods 9 (3), 340. doi:10.3390/foods9030340
Menghini, R., Casagrande, V., Cardellini, M., Martelli, E., Terrinoni, A., Amati, F., et al. (2009). MicroRNA 217 modulates endothelial cell senescence via silent information regulator 1. Circulation 120 (15), 1524–1532. doi:10.1161/circulationaha.109.864629
Mercken, E. M., Crosby, S. D., Lamming, D. W., JeBailey, L., Krzysik-Walker, S., Villareal, D. T., et al. (2013a). Calorie restriction in humans inhibits the PI3K/AKT pathway and induces a younger transcription profile. Aging Cell 12 (4), 645–651. doi:10.1111/acel.12088
Mercken, E. M., Majounie, E., Ding, J., Guo, R., Kim, J., Bernier, M., et al. (2013b). Age-associated miRNA alterations in skeletal muscle from rhesus monkeys reversed by caloric restriction. Aging (Albany NY) 5 (9), 692–703. doi:10.18632/aging.100598
Micó, V., Berninches, L., Tapia, J., and Daimiel, L. (2017). NutrimiRAging: micromanaging nutrient sensing pathways through nutrition to promote healthy aging. Int. J. Mol. Sci. 18 (5), 915. doi:10.3390/ijms18050915
Milenkovic, D., Jude, B., and Morand, C. (2013). miRNA as molecular target of polyphenols underlying their biological effects. Free Radic. Biol. Med. 64, 40–51. doi:10.1016/j.freeradbiomed.2013.05.046
Miller, R. A., Harrison, D. E., Astle, C. M., Fernandez, E., Flurkey, K., Han, M., et al. (2014). Rapamycin-mediated lifespan increase in mice is dose and sex dependent and metabolically distinct from dietary restriction. Aging Cell 13 (3), 468–477. doi:10.1111/acel.12194
Milte, C. M., Parletta, N., Buckley, J. D., Coates, A. M., Young, R. M., and Howe, P. R. (2012). Eicosapentaenoic and docosahexaenoic acids, cognition, and behavior in children with attention-deficit/hyperactivity disorder: a randomized controlled trial. Nutrition 28 (6), 670–677. doi:10.1016/j.nut.2011.12.009
Misawa, T., Tanaka, Y., Okada, R., and Takahashi, A. (2020). Biology of extracellular vesicles secreted from senescent cells as senescence-associated secretory phenotype factors. Geriatrics Gerontology Int. 20 (6), 539–546. doi:10.1111/ggi.13928
Mischley, L. K., Lau, R. C., and Bennett, R. D. (2017). Role of diet and nutritional supplements in Parkinson’s disease progression. Oxidative Med. Cell. Longev. 2017, 6405278. doi:10.1155/2017/6405278
Most, J., Tosti, V., Redman, L. M., and Fontana, L. (2017). Calorie restriction in humans: an update. Ageing Res. Rev. 39, 36–45. doi:10.1016/j.arr.2016.08.005
Natoli, R., and Fernando, N. (2018). MicroRNA as therapeutics for age-related macular degeneration. Retin. Degener. Dis. Mech. Exp. Ther. 1074, 37–43. doi:10.1007/978-3-319-75402-4_5
Newman, M. A., and Hammond, S. M. (2010). Emerging paradigms of regulated microRNA processing. Genes Dev. 24 (11), 1086–1092. doi:10.1101/gad.1919710
Noratto, G. D., Angel-Morales, G., Talcott, S. T., and Mertens-Talcott, S. U. (2011a). Polyphenolics from acai (Euterpe oleracea Mart.) and red muscadine grape (Vitis rotundifolia) protect human umbilical vascular Endothelial cells (HUVEC) from glucose- and lipopolysaccharide (LPS)-induced inflammation and target microRNA-126. J. Agric. Food Chem. 59 (14), 7999–8012. doi:10.1021/jf201056x
Noratto, G. D., Kim, Y., Talcott, S. T., and Mertens-Talcott, S. U. (2011b). Flavonol-rich fractions of yaupon holly leaves (Ilex vomitoria, Aquifoliaceae) induce microRNA-146a and have anti-inflammatory and chemopreventive effects in intestinal myofibroblast CCD-18Co cells. Fitoterapia 82 (4), 557–569. doi:10.1016/j.fitote.2011.01.013
Nurk, E., Refsum, H., Drevon, C. A., Tell, G. S., Nygaard, H. A., Engedal, K., et al. (2009). Intake of flavonoid-rich wine, tea, and chocolate by elderly men and women is associated with better cognitive test performance. J. Nutr. 139 (1), 120–127. doi:10.3945/jn.108.095182
O'Brien, J., Hayder, H., Zayed, Y., and Peng, C. (2018). Overview of MicroRNA biogenesis, mechanisms of actions, and circulation. Front. Endocrinol. (Lausanne) 9, 402. doi:10.3389/fendo.2018.00402
O'Keefe, J. H., Gheewala, N. M., and O'Keefe, J. O. (2008). Dietary strategies for improving post-prandial glucose, lipids, inflammation, and cardiovascular health. J. Am. Coll. Cardiol. 51 (3), 249–255. doi:10.1016/j.jacc.2007.10.016
Olena, A. F., and Patton, J. G. (2010). Genomic organization of microRNAs. J. Cell Physiol. 222 (3), 540–545. doi:10.1002/jcp.21993
Olivieri, F., Ahtiainen, M., Lazzarini, R., Pöllänen, E., Capri, M., Lorenzi, M., et al. (2014). Hormone replacement therapy enhances IGF-1 signaling in skeletal muscle by diminishing miR-182 and miR-223 expressions: a study on postmenopausal monozygotic twin pairs. Aging Cell 13 (5), 850–861. doi:10.1111/acel.12245
Olivieri, F., Albertini, M. C., Orciani, M., Ceka, A., Cricca, M., Procopio, A. D., et al. (2015). DNA damage response (DDR) and senescence: shuttled inflamma-miRNAs on the stage of inflamm-aging. Oncotarget 6 (34), 35509–35521. doi:10.18632/oncotarget.5899
Olivieri, F., Lazzarini, R., Recchioni, R., Marcheselli, F., Rippo, M. R., Di Nuzzo, S., et al. (2013). MiR-146a as marker of senescence-associated pro-inflammatory status in cells involved in vascular remodelling. Age (Dordr) 35 (4), 1157–1172. doi:10.1007/s11357-012-9440-8
Ortega, F. J., Cardona-Alvarado, M. I., Mercader, J. M., Moreno-Navarrete, J. M., Moreno, M., Sabater, M., et al. (2015). Circulating profiling reveals the effect of a polyunsaturated fatty acid-enriched diet on common microRNAs. J. Nutr. Biochem. 26 (10), 1095–1101. doi:10.1016/j.jnutbio.2015.05.001
Ostan, R., Béné, M. C., Spazzafumo, L., Pinto, A., Donini, L. M., Pryen, F., et al. (2016). Impact of diet and nutraceutical supplementation on inflammation in elderly people. Results from the RISTOMED study, an open-label randomized control trial. Clin. Nutr. 35 (4), 812–818. doi:10.1016/j.clnu.2015.06.010
Ovchinnikova, E. S., Schmitter, D., Vegter, E. L., Ter Maaten, J. M., Valente, M. A., Liu, L. C., et al. (2016). Signature of circulating microRNAs in patients with acute heart failure. Eur. J. Heart Fail 18 (4), 414–423. doi:10.1002/ejhf.332
Pan, B., Zheng, L., Liu, S., Fang, J., Lou, C., Hu, X., et al. (2022). MiR-148a deletion protects from bone loss in physiological and estrogen-deficient mice by targeting NRP1. Cell Death Discov. 8 (1), 470. doi:10.1038/s41420-022-01261-5
Panizo, S., Carrillo-López, N., Naves-Díaz, M., Solache-Berrocal, G., Martínez-Arias, L., Rodrigues-Díez, R. R., et al. (2017). Regulation of miR-29b and miR-30c by vitamin D receptor activators contributes to attenuate uraemia-induced cardiac fibrosis. Nephrol. Dial. Transpl. 32 (11), 1831–1840. doi:10.1093/ndt/gfx060
Papadopoli, D., Boulay, K., Kazak, L., Pollak, M., Mallette, F., Topisirovic, I., et al. (2019). mTOR as a central regulator of lifespan and aging. F1000Res 8, F1000 Faculty Rev-998. doi:10.12688/f1000research.17196.1
Patterson, D. G., Roberts, J. T., King, V. M., Houserova, D., Barnhill, E. C., Crucello, A., et al. (2017). Human snoRNA-93 is processed into a microRNA-like RNA that promotes breast cancer cell invasion. NPJ Breast Cancer 3, 25. doi:10.1038/s41523-017-0032-8
Perri, M., Caroleo, M. C., Liu, N., Gallelli, L., De Sarro, G., Kagechika, H., et al. (2017). 9-cis Retinoic acid modulates myotrophin expression and its miR in physiological and pathophysiological cell models. Exp. Cell Res. 354 (1), 25–30. doi:10.1016/j.yexcr.2017.03.022
Pignolo, R. J., Law, S. F., and Chandra, A. (2021). Bone aging, cellular senescence, and osteoporosis. JBMR Plus 5 (4), e10488. doi:10.1002/jbm4.10488
Pogue, A. I., and Lukiw, W. J. (2018). Up-regulated pro-inflammatory MicroRNAs (miRNAs) in Alzheimer's disease (AD) and age-related macular degeneration (AMD). Cell Mol. Neurobiol. 38 (5), 1021–1031. doi:10.1007/s10571-017-0572-3
Poursaei, E., Abolghasemi, M., Bornehdeli, S., Shanehbandi, D., Asadi, M., Sadeghzadeh, M., et al. (2022). Evaluation of hsa-let-7d-5p, hsa-let-7g-5p and hsa-miR-15b-5p plasma levels in patients with Alzheimer’s disease. Psychiatr. Genet. 32 (1), 25–29. doi:10.1097/ypg.0000000000000303
Prasad, A. S. (2012). Discovery of human zinc deficiency: 50 years later. J. Trace Elem. Med. Biol. 26 (2-3), 66–69. doi:10.1016/j.jtemb.2012.04.004
Prasnikar, E., Borisek, J., and Perdih, A. (2021). Senescent cells as promising targets to tackle age-related diseases. Ageing Res. Rev. 66, 101251. doi:10.1016/j.arr.2020.101251
Qiang, L., Hong, L., Ningfu, W., Huaihong, C., and Jing, W. (2013). Expression of miR-126 and miR-508-5p in endothelial progenitor cells is associated with the prognosis of chronic heart failure patients. Int. J. Cardiol. 168 (3), 2082–2088. doi:10.1016/j.ijcard.2013.01.160
Quinlan, S., Kenny, A., Medina, M., Engel, T., and Jimenez-Mateos, E. M. (2017). MicroRNAs in neurodegenerative diseases. Int. Rev. Cell Mol. Biol. 334, 309–343. doi:10.1016/bs.ircmb.2017.04.002
Quintanilha, B. J., Reis, B. Z., Duarte, G. B. S., Cozzolino, S. M., and Rogero, M. M. (2017a). Nutrimiromics: role of microRNAs and nutrition in modulating inflammation and chronic diseases. Nutrients 9 (11), 1168. doi:10.3390/nu9111168
Quintanilha, B. J., Reis, B. Z., Duarte, G. B. S., Cozzolino, S. M. F., and Rogero, M. M. (2017b). Nutrimiromics: role of microRNAs and nutrition in modulating inflammation and chronic diseases. Nutrients 9 (11), 1168. doi:10.3390/nu9111168
Rasheed, Z., Rasheed, N., and Al-Shobaili, H. A. (2016). Epigallocatechin-3-O-gallate up-regulates microRNA-199a-3p expression by down-regulating the expression of cyclooxygenase-2 in stimulated human osteoarthritis chondrocytes. J. Cell Mol. Med. 20 (12), 2241–2248. doi:10.1111/jcmm.12897
Rayner, K. J., Sheedy, F. J., Esau, C. C., Hussain, F. N., Temel, R. E., Parathath, S., et al. (2011). Antagonism of miR-33 in mice promotes reverse cholesterol transport and regression of atherosclerosis. J. Clin. investigation 121 (7), 2921–2931. doi:10.1172/JCI57275
Ren, C., Liu, Q., Wei, Q., Cai, W., He, M., Du, Y., et al. (2017). Circulating miRNAs as potential biomarkers of age-related macular degeneration. Cell. Physiology Biochem. 41 (4), 1413–1423. doi:10.1159/000467941
Rizk, N. I., Abulsoud, A. I., Kamal, M. M., Kassem, D. H., and Hamdy, N. M. (2022). Exosomal-long non-coding RNAs journey in colorectal cancer: evil and goodness faces of key players. Life Sci. 292, 120325. doi:10.1016/j.lfs.2022.120325
Rodier, F., Coppe, J. P., Patil, C. K., Hoeijmakers, W. A., Munoz, D. P., Raza, S. R., et al. (2009). Persistent DNA damage signalling triggers senescence-associated inflammatory cytokine secretion. Nat. Cell Biol. 11 (8), 973–979. doi:10.1038/ncb1909
Rodriguez, A., Griffiths-Jones, S., Ashurst, J. L., and Bradley, A. (2004). Identification of mammalian microRNA host genes and transcription units. Genome Res. 14 (10a), 1902–1910. doi:10.1101/gr.2722704
Rogina, B., and Helfand, S. L. (2004). Sir2 mediates longevity in the fly through a pathway related to calorie restriction. Proc. Natl. Acad. Sci. U. S. A. 101 (45), 15998–16003. doi:10.1073/pnas.0404184101
Romano, G. L., Platania, C. B. M., Drago, F., Salomone, S., Ragusa, M., Barbagallo, C., et al. (2017). Retinal and circulating miRNAs in age-related macular degeneration: an in vivo animal and human study. Front. Pharmacol. 8, 168. doi:10.3389/fphar.2017.00168
Ruby, J. G., Jan, C. H., and Bartel, D. P. (2007a). Intronic microRNA precursors that bypass Drosha processing. Nature 448 (7149), 83–86. doi:10.1038/nature05983
Ruby, J. G., Jan, C. H., and Bartel, D. P. (2007b). Intronic microRNA precursors that bypass Drosha processing. Nature 448 (7149), 83–86. doi:10.1038/nature05983
Ruiz-Núñez, B., Pruimboom, L., Dijck-Brouwer, D. A., and Muskiet, F. A. (2013). Lifestyle and nutritional imbalances associated with Western diseases: causes and consequences of chronic systemic low-grade inflammation in an evolutionary context. J. Nutr. Biochem. 24 (7), 1183–1201. doi:10.1016/j.jnutbio.2013.02.009
Sadu, L., Krishnan, R., Akshaya, R., Saranya, I., Das, U. R., Satishkumar, S., et al. (2023). Roles of miR-214 in bone physiology and disease. Biocell 47 (4), 751–760. doi:10.32604/biocell.2023.026911
Salman, I. T., Abulsoud, A. I., Abo-Elmatty, D. M., Fawzy, A., Mesbah, N. M., and Saleh, S. M. (2023). The long non-coding RNA ZFAS1 promotes colorectal cancer progression via miR200b/ZEB1 axis. Pathology - Res. Pract. 247, 154567. doi:10.1016/j.prp.2023.154567
Sangiao-Alvarellos, S., Pena-Bello, L., Manfredi-Lozano, M., Tena-Sempere, M., and Cordido, F. (2014). Perturbation of hypothalamic microRNA expression patterns in male rats after metabolic distress: impact of obesity and conditions of negative energy balance. Endocrinology 155 (5), 1838–1850. doi:10.1210/en.2013-1770
Santos, M. E. S., de Oliveira Paraense, R. S., de Souza Fonseca, R. R., Pereira, D. L. A., Cordeiro, C. E. S., dos Santos Corrêa, T. L., et al. (2021). Profile of circulating MicroRNAs in low density lipoprotein uptake: literature review. Rev. Eletrônica Acervo Saúde 13 (1), e4546. doi:10.25248/reas.e4546.2021
Sarbassov, D. D., Ali, S. M., Sengupta, S., Sheen, J. H., Hsu, P. P., Bagley, A. F., et al. (2006). Prolonged rapamycin treatment inhibits mTORC2 assembly and Akt/PKB. Mol. Cell 22 (2), 159–168. doi:10.1016/j.molcel.2006.03.029
Sarveswaran, S., Liroff, J., Zhou, Z., Nikitin, A. Y., and Ghosh, J. (2010). Selenite triggers rapid transcriptional activation of p53, and p53-mediated apoptosis in prostate cancer cells: implication for the treatment of early-stage prostate cancer. Int. J. Oncol. 36 (6), 1419–1428. doi:10.3892/ijo_00000627
Semina, E. V., Rysenkova, K. D., Troyanovskiy, K. E., Shmakova, A. A., and Rubina, K. A. (2021). MicroRNAs in cancer: from gene expression regulation to the metastatic niche reprogramming. Biochem. Mosc. 86 (7), 785–799. doi:10.1134/S0006297921070014
Sene, L. B., Rizzi, V. H. G., Gontijo, J. A. R., and Boer, P. A. (2018). Gestational low-protein intake enhances whole-kidney miR-192 and miR-200 family expression and epithelial-to-mesenchymal transition in rat adult male offspring. J. Exp. Biol. 221 (10), jeb171694. doi:10.1242/jeb.171694
Seyhan, A. A. (2024). Trials and tribulations of MicroRNA therapeutics. Int. J. Mol. Sci. 25 (3), 1469. doi:10.3390/ijms25031469
Shang, R., Lee, S., Senavirathne, G., and Lai, E. C. (2023). microRNAs in action: biogenesis, function and regulation. Nat. Rev. Genet. 24 (12), 816–833. doi:10.1038/s41576-023-00611-y
Sharma, A. R., Sharma, G., Bhattacharya, M., Lee, S. S., and Chakraborty, C. (2022). Circulating miRNA in atherosclerosis: a clinical biomarker and early diagnostic tool. Curr. Mol. Med. 22 (3), 250–262. doi:10.2174/1566524021666210315124438
Shi, S., Chen, T., Lu, W., Chen, Y., Xiao, D., and Lin, Y. (2023). Amelioration of osteoarthritis via tetrahedral framework nucleic acids delivering microrna-124 for cartilage regeneration. Adv. Funct. Mater. 33 (46), 2305558. doi:10.1002/adfm.202305558
Singh, S., de Ronde, M. W. J., Kok, M. G. M., Beijk, M. A., De Winter, R. J., van der Wal, A. C., et al. (2020). MiR-223-3p and miR-122-5p as circulating biomarkers for plaque instability. Open Heart 7 (1), e001223. doi:10.1136/openhrt-2019-001223
Sinha, A., Cheetham, T. D., and Pearce, S. H. S. (2013). Prevention and treatment of vitamin D deficiency. Calcif. Tissue Int. 92 (2), 207–215. doi:10.1007/s00223-012-9663-9
Sinn, N., Milte, C. M., Street, S. J., Buckley, J. D., Coates, A. M., Petkov, J., et al. (2012). Effects of n-3 fatty acids, EPA v. DHA, on depressive symptoms, quality of life, memory and executive function in older adults with mild cognitive impairment: a 6-month randomised controlled trial. Br. J. Nutr. 107 (11), 1682–1693. doi:10.1017/s0007114511004788
Smith-Vikos, T., Liu, Z., Parsons, C., Gorospe, M., Ferrucci, L., Gill, T. M., et al. (2016). A serum miRNA profile of human longevity: findings from the Baltimore Longitudinal Study of Aging (BLSA). Aging (Albany NY) 8 (11), 2971–2987. doi:10.18632/aging.101106
Smith-Vikos, T., and Slack, F. J. (2012). MicroRNAs and their roles in aging. J. Cell Sci. 125 (Pt 1), 7–17. doi:10.1242/jcs.099200
Sohel, M. M. H. (2020). Macronutrient modulation of mRNA and microRNA function in animals: a review. Anim. Nutr. 6 (3), 258–268. doi:10.1016/j.aninu.2020.06.002
Somel, M., Guo, S., Fu, N., Yan, Z., Hu, H. Y., Xu, Y., et al. (2010). MicroRNA, mRNA, and protein expression link development and aging in human and macaque brain. Genome Res. 20 (9), 1207–1218. doi:10.1101/gr.106849.110
Song, J., Jun, M., Ahn, M. R., and Kim, O. Y. (2016). Involvement of miR-Let7A in inflammatory response and cell survival/apoptosis regulated by resveratrol in THP-1 macrophage. Nutr. Res. Pract. 10 (4), 377–384. doi:10.4162/nrp.2016.10.4.377
Sørensen, S. S., Nygaard, A.-B., and Christensen, T. (2016). miRNA expression profiles in cerebrospinal fluid and blood of patients with Alzheimer’s disease and other types of dementia – an exploratory study. Transl. Neurodegener. 5 (1), 6. doi:10.1186/s40035-016-0053-5
Soukas, A. A., Hao, H., and Wu, L. (2019). Metformin as anti-aging therapy: is it for everyone? Trends Endocrinol. Metab. 30 (10), 745–755. doi:10.1016/j.tem.2019.07.015
Sun, X., Sit, A., and Feinberg, M. W. (2014). Role of miR-181 family in regulating vascular inflammation and immunity. Trends Cardiovasc. Med. 24 (3), 105–112. doi:10.1016/j.tcm.2013.09.002
Tabet, F., Cuesta Torres, L. F., Ong, K. L., Shrestha, S., Choteau, S. A., Barter, P. J., et al. (2016). High-density lipoprotein-associated miR-223 is altered after diet-induced weight loss in overweight and obese males. PLoS One 11 (3), e0151061. doi:10.1371/journal.pone.0151061
Tao, H., Cheng, L., and Yang, R. (2020). Downregulation of miR-34a promotes proliferation and inhibits apoptosis of rat osteoarthritic cartilage cells by activating PI3K/Akt pathway. Clin. Interventions Aging 15, 373–385. doi:10.2147/CIA.S241855
Tessitore, A., Cicciarelli, G., Del Vecchio, F., Gaggiano, A., Verzella, D., Fischietti, M., et al. (2016). MicroRNA expression analysis in high fat diet-induced NAFLD-NASH-HCC progression: study on C57BL/6J mice. BMC Cancer 16, 3. doi:10.1186/s12885-015-2007-1
Tian, G., Hu, K., Qiu, S., Xie, Y., Cao, Y., Ni, S., et al. (2021). Exosomes derived from PC-3 cells suppress osteoclast differentiation by downregulating miR-148a and blocking the PI3K/AKT/mTOR pathway. Exp. Ther. Med. 22 (5), 1304–1310. doi:10.3892/etm.2021.10739
Tili, E., Michaille, J. J., Adair, B., Alder, H., Limagne, E., Taccioli, C., et al. (2010). Resveratrol decreases the levels of miR-155 by upregulating miR-663, a microRNA targeting JunB and JunD. Carcinogenesis 31 (9), 1561–1566. doi:10.1093/carcin/bgq143
Ting, -.Y. T., Geng, -.L. C., Chao, -.G. S., Yi, -.Z., and Chang, -.W. P. (2018). The serum exosome derived MicroRNA-135a, -193b, and-384 were potential Alzheimer's disease biomarkers. Biomed. Environ. Sci. 31 (2)–87. doi:10.3967/bes2018.011
Tissenbaum, H. A., and Guarente, L. (2001). Increased dosage of a sir-2 gene extends lifespan in Caenorhabditis elegans. Nature 410 (6825), 227–230. doi:10.1038/35065638
Tkach, M., and Théry, C. (2016). Communication by extracellular vesicles: where we are and where we need to go. Cell 164 (6), 1226–1232. doi:10.1016/j.cell.2016.01.043
Tomé-Carneiro, J., Larrosa, M., Yáñez-Gascón, M. J., Dávalos, A., Gil-Zamorano, J., Gonzálvez, M., et al. (2013). One-year supplementation with a grape extract containing resveratrol modulates inflammatory-related microRNAs and cytokines expression in peripheral blood mononuclear cells of type 2 diabetes and hypertensive patients with coronary artery disease. Pharmacol. Res. 72, 69–82. doi:10.1016/j.phrs.2013.03.011
Tullet, J. M., Hertweck, M., An, J. H., Baker, J., Hwang, J. Y., Liu, S., et al. (2008). Direct inhibition of the longevity-promoting factor SKN-1 by insulin-like signaling in C. elegans. Cell 132 (6), 1025–1038. doi:10.1016/j.cell.2008.01.030
Ugalde, A. P., Español, Y., and López-Otín, C. (2011). Micromanaging aging with miRNAs: new messages from the nuclear envelope. Nucleus 2 (6), 549–555. doi:10.4161/nucl.2.6.17986
Urbańska, K., Stępień, P. W., Nowakowska, K. N., Stefaniak, M., Osial, N., Chorągiewicz, T., et al. (2022). The role of dysregulated miRNAs in the pathogenesis, diagnosis and treatment of age-related macular degeneration. Int. J. Mol. Sci. 23 (14), 7761. doi:10.3390/ijms23147761
Vahidi, Z., Samadi, M., Mahmoudi, M., RezaieYazdi, Z., Sahebari, M., Tabasi, N., et al. (2018). Lactobacillus rhamnosus and Lactobacillus delbrueckii ameliorate the expression of miR-155 and miR-181a in SLE patients. J. Funct. Foods 48, 228–233. doi:10.1016/j.jff.2018.07.025
Vasto, S., Candore, G., Balistreri, C. R., Caruso, M., Colonna-Romano, G., Grimaldi, M. P., et al. (2007). Inflammatory networks in ageing, age-related diseases and longevity. Mech. Ageing Dev. 128 (1), 83–91. doi:10.1016/j.mad.2006.11.015
Vegter, E. L., Schmitter, D., Hagemeijer, Y., Ovchinnikova, E. S., van der Harst, P., Teerlink, J. R., et al. (2016). Use of biomarkers to establish potential role and function of circulating microRNAs in acute heart failure. Int. J. Cardiol. 224, 231–239. doi:10.1016/j.ijcard.2016.09.010
Veldurthy, V., Wei, R., Oz, L., Dhawan, P., Jeon, Y. H., and Christakos, S. (2016). Vitamin D, calcium homeostasis and aging. Bone Res. 4, 16041. doi:10.1038/boneres.2016.41
Vellai, T., Takacs-Vellai, K., Zhang, Y., Kovacs, A. L., Orosz, L., and Müller, F. (2003). Genetics: influence of TOR kinase on lifespan in C. elegans. Nature 426 (6967), 620. doi:10.1038/426620a
Visioli, F., Giordano, E., Nicod, N. M., and Dávalos, A. (2012). Molecular targets of omega 3 and conjugated linoleic Fatty acids - "micromanaging" cellular response. Front. Physiol. 3, 42. doi:10.3389/fphys.2012.00042
Vonk, L. A., Kragten, A. H., Dhert, W., Saris, D. B., and Creemers, L. B. (2014). Overexpression of hsa-miR-148a promotes cartilage production and inhibits cartilage degradation by osteoarthritic chondrocytes. Osteoarthr. Cartil. 22 (1), 145–153. doi:10.1016/j.joca.2013.11.006
Wagschal, A., Najafi-Shoushtari, S. H., Wang, L., Goedeke, L., Sinha, S., Delemos, A. S., et al. (2015). Genome-wide identification of microRNAs regulating cholesterol and triglyceride homeostasis. Nat. Med. 21 (11), 1290–1297. doi:10.1038/nm.3980
Wang, C., Wang, P., Li, F., Li, Y., Zhao, M., Feng, H., et al. (2023). Adenovirus-associated anti-miRNA-214 regulates bone metabolism and prevents local osteoporosis in rats. Front. Bioeng. Biotechnol. 11, 1164252. doi:10.3389/fbioe.2023.1164252
Wang, T., O'Brien, E. C., Rogers, J. G., Jacoby, D. L., Chen, M. E., Testani, J. M., et al. (2017a). Plasma levels of MicroRNA-155 are upregulated with long-term left ventricular assist device support. Asaio J. 63 (5), 536–541. doi:10.1097/mat.0000000000000564
Wang, X. S., Gong, J. N., Yu, J., Wang, F., Zhang, X. H., Yin, X. L., et al. (2012). MicroRNA-29a and microRNA-142-3p are regulators of myeloid differentiation and acute myeloid leukemia. Blood 119 (21), 4992–5004. doi:10.1182/blood-2011-10-385716
Wang, Y., He, J., Liao, M., Hu, M., Li, W., Ouyang, H., et al. (2019). An overview of Sirtuins as potential therapeutic target: structure, function and modulators. Eur. J. Med. Chem. 161, 48–77. doi:10.1016/j.ejmech.2018.10.028
Wang, Y., Qian, Y., Fang, Q., Zhong, P., Li, W., Wang, L., et al. (2017b). Saturated palmitic acid induces myocardial inflammatory injuries through direct binding to TLR4 accessory protein MD2. Nat. Commun. 8, 13997. doi:10.1038/ncomms13997
Wang, Z. (2011). The principles of MiRNA-masking antisense oligonucleotides technology. Methods Mol. Biol. 676, 43–49. doi:10.1007/978-1-60761-863-8_3
Wei, X., Ke, H., Wen, A., Gao, B., Shi, J., and Feng, Y. (2021). Structural basis of microRNA processing by Dicer-like 1. Nat. Plants 7 (10), 1389–1396. doi:10.1038/s41477-021-01000-1
Weichhart, T. (2018). mTOR as regulator of lifespan, aging, and cellular senescence: a mini-review. Gerontology 64 (2), 127–134. doi:10.1159/000484629
Westholm, J. O., and Lai, E. C. (2011). Mirtrons: microRNA biogenesis via splicing. Biochimie 93 (11), 1897–1904. doi:10.1016/j.biochi.2011.06.017
Widlund, A. L., Baur, J. A., and Vang, O. (2013). mTOR: more targets of resveratrol? Expert Rev. Mol. Med. 15, e10. doi:10.1017/erm.2013.11
Willcox, B. J., Tranah, G. J., Chen, R., Morris, B. J., Masaki, K. H., He, Q., et al. (2016). The FoxO3 gene and cause-specific mortality. Aging Cell 15 (4), 617–624. doi:10.1111/acel.12452
Witwer, K. W. (2012). XenomiRs and miRNA homeostasis in health and disease: evidence that diet and dietary miRNAs directly and indirectly influence circulating miRNA profiles. RNA Biol. 9 (9), 1147–1154. doi:10.4161/rna.21619
Witwer, K. W. (2015). Circulating microRNA biomarker studies: pitfalls and potential solutions. Clin. Chem. 61 (1), 56–63. doi:10.1373/clinchem.2014.221341
Wong, S. W., Kwon, M. J., Choi, A. M., Kim, H. P., Nakahira, K., and Hwang, D. H. (2009). Fatty acids modulate Toll-like receptor 4 activation through regulation of receptor dimerization and recruitment into lipid rafts in a reactive oxygen species-dependent manner. J. Biol. Chem. 284 (40), 27384–27392. doi:10.1074/jbc.M109.044065
Wu, X., Cui, C. L., Chen, W. L., Fu, Z. Y., Cui, X. Y., and Gong, X. (2016). miR-144 suppresses the growth and metastasis of laryngeal squamous cell carcinoma by targeting IRS1. Am. J. Transl. Res. 8 (1), 1–11.
Xiang, C., Cui, S. P., and Ke, Y. (2016). MiR-144 inhibits cell proliferation of renal cell carcinoma by targeting MTOR. J. Huazhong Univ. Sci. Technol. Med. Sci. 36 (2), 186–192. doi:10.1007/s11596-016-1564-0
Xie, H., Lei, N., Gong, A. Y., Chen, X. M., and Hu, G. (2014). Cryptosporidium parvum induces SIRT1 expression in host epithelial cells through downregulating let-7i. Hum. Immunol. 75 (8), 760–765. doi:10.1016/j.humimm.2014.05.007
Xing, Y., Liu, Z., Yang, G., Gao, D., and Niu, X. (2015). MicroRNA expression profiles in rats with selenium deficiency and the possible role of the Wnt/β-catenin signaling pathway in cardiac dysfunction. Int. J. Mol. Med. 35 (1), 143–152. doi:10.3892/ijmm.2014.1976
Xiong, H., Pang, J., Yang, H., Dai, M., Liu, Y., Ou, Y., et al. (2015). Activation of miR-34a/SIRT1/p53 signaling contributes to cochlear hair cell apoptosis: implications for age-related hearing loss. Neurobiol. Aging 36 (4), 1692–1701. doi:10.1016/j.neurobiolaging.2014.12.034
Xu, Y., Xu, Y., Zhu, Y., Sun, H., Juguilon, C., Li, F., et al. (2020). Macrophage miR-34a is a key regulator of cholesterol efflux and atherosclerosis. Mol. Ther. 28 (1), 202–216. doi:10.1016/j.ymthe.2019.09.008
Yaman, S. O., Orem, A., Yucesan, F. B., Kural, B. V., and Orem, C. (2021). Evaluation of circulating miR-122, miR-30c and miR-33a levels and their association with lipids, lipoproteins in postprandial lipemia. Life Sci. 264, 118585. doi:10.1016/j.lfs.2020.118585
Yang, F., Chu, X., Yin, M., Liu, X., Yuan, H., Niu, Y., et al. (2014a). mTOR and autophagy in normal brain aging and caloric restriction ameliorating age-related cognition deficits. Behav. Brain Res. 264, 82–90. doi:10.1016/j.bbr.2014.02.005
Yang, Q., Jia, C., Wang, P., Xiong, M., Cui, J., Li, L., et al. (2014b). MicroRNA-505 identified from patients with essential hypertension impairs endothelial cell migration and tube formation. Int. J. Cardiol. 177 (3), 925–934. doi:10.1016/j.ijcard.2014.09.204
Yang, W.-M., Jeong, H.-J., Park, S.-Y., and Lee, W. (2014c). Induction of miR-29a by saturated fatty acids impairs insulin signaling and glucose uptake through translational repression of IRS-1 in myocytes. FEBS Lett. 588 (13), 2170–2176. doi:10.1016/j.febslet.2014.05.011
Yang, W.-M., Jeong, H.-J., Park, S.-Y., and Lee, W. (2014d). Saturated fatty acid-induced miR-195 impairs insulin signaling and glycogen metabolism in HepG2 cells. FEBS Lett. 588 (21), 3939–3946. doi:10.1016/j.febslet.2014.09.006
Yu, Y., Zhang, J., Wang, J., and Sun, B. (2021). MicroRNAs: the novel mediators for nutrient-modulating biological functions. Trends Food Sci. Technol. 114, 167–175. doi:10.1016/j.tifs.2021.05.028
Zeljic, K., Supic, G., and Magic, Z. (2017). New insights into vitamin D anticancer properties: focus on miRNA modulation. Mol. Genet. Genomics 292 (3), 511–524. doi:10.1007/s00438-017-1301-9
Zhang, H., Yang, H., Zhang, C., Jing, Y., Wang, C., Liu, C., et al. (2015a). Investigation of microRNA expression in human serum during the aging process. J. Gerontol. A Biol. Sci. Med. Sci. 70 (1), 102–109. doi:10.1093/gerona/glu145
Zhang, H., Zheng, W., Li, D., and Zheng, J. (2021). miR-146a-5p promotes chondrocyte apoptosis and inhibits autophagy of osteoarthritis by targeting NUMB. Cartilage 13 (2_Suppl. l), 1467S–1477S. doi:10.1177/19476035211023550
Zhang, J., Gao, Y., Yu, M., Wu, H., Ai, Z., Wu, Y., et al. (2015b). Retinoic acid induces embryonic stem cell differentiation by altering both encoding RNA and microRNA expression. PLoS One 10 (7), e0132566. doi:10.1371/journal.pone.0132566
Zhang, L. Y., Ho-Fun Lee, V., Wong, A. M., Kwong, D. L., Zhu, Y. H., Dong, S. S., et al. (2013). MicroRNA-144 promotes cell proliferation, migration and invasion in nasopharyngeal carcinoma through repression of PTEN. Carcinogenesis 34 (2), 454–463. doi:10.1093/carcin/bgs346
Zhang, M., Wu, J.-F., Chen, W.-J., Tang, S.-L., Mo, Z.-C., Tang, Y.-Y., et al. (2014). MicroRNA-27a/b regulates cellular cholesterol efflux, influx and esterification/hydrolysis in THP-1 macrophages. Atherosclerosis 234 (1), 54–64. doi:10.1016/j.atherosclerosis.2014.02.008
Zhang, X., Rotllan, N., Canfrán-Duque, A., Sun, J., Toczek, J., Moshnikova, A., et al. (2022). Targeted suppression of miRNA-33 using pHLIP improves atherosclerosis regression. Circ. Res. 131 (1), 77–90. doi:10.1161/circresaha.121.320296
Zhang, Y., Liu, Y. J., Liu, T., Zhang, H., and Yang, S. J. (2016). Plasma microRNA-21 is a potential diagnostic biomarker of acute myocardial infarction. Eur. Rev. Med. Pharmacol. Sci. 20 (2), 323–329.
Zheng, Z., Ge, Y., Zhang, J., Xue, M., Li, Q., Lin, D., et al. (2015). PUFA diets alter the microRNA expression profiles in an inflammation rat model. Mol. Med. Rep. 11 (6), 4149–4157. doi:10.3892/mmr.2015.3318
Zhor, C., Wafaa, L., Ghzaiel, I., Kessas, K., Zarrouk, A., Ksila, M., et al. (2023). Effects of polyphenols and their metabolites on age-related diseases. Biochem. Pharmacol. 214, 115674. doi:10.1016/j.bcp.2023.115674
Zhu, C., Ji, C. B., Zhang, C. M., Gao, C. L., Zhu, J. G., Qin, D. N., et al. (2010). The lin-4 gene controls fat accumulation and longevity in Caenorhabditis elegans. Int. J. Mol. Sci. 11 (12), 4814–4825. doi:10.3390/ijms11124814
Zhu, J., Liu, B., Wang, Z., Wang, D., Ni, H., Zhang, L., et al. (2019). Exosomes from nicotine-stimulated macrophages accelerate atherosclerosis through miR-21-3p/PTEN-mediated VSMC migration and proliferation. Theranostics 9 (23), 6901–6919. doi:10.7150/thno.37357
Glossary
Keywords: aging, age-related diseases, miRNAs, nutrition, nutraceuticals
Citation: Salama RM, Eissa N, Doghish AS, Abulsoud AI, Abdelmaksoud NM, Mohammed OA, Abdel Mageed SS and Darwish SF (2024) Decoding the secrets of longevity: unraveling nutraceutical and miRNA-Mediated aging pathways and therapeutic strategies. Front. Aging 5:1373741. doi: 10.3389/fragi.2024.1373741
Received: 20 January 2024; Accepted: 04 March 2024;
Published: 28 March 2024.
Edited by:
Ricardo P. Garay, Centre National de la Recherche Scientifique (CNRS), FranceReviewed by:
Alessandra Magenta, Consiglio Nazionale delle Ricerche (CNR), ItalyPrabu Paramasivam, University of New Mexico Health Sciences Center, United States
Copyright © 2024 Salama, Eissa, Doghish, Abulsoud, Abdelmaksoud, Mohammed, Abdel Mageed and Darwish. This is an open-access article distributed under the terms of the Creative Commons Attribution License (CC BY). The use, distribution or reproduction in other forums is permitted, provided the original author(s) and the copyright owner(s) are credited and that the original publication in this journal is cited, in accordance with accepted academic practice. No use, distribution or reproduction is permitted which does not comply with these terms.
*Correspondence: Rania M. Salama, cmFuaWEuc2FsYW1hQG1pdWVneXB0LmVkdS5lZw==