- 1Department of Immunology, School of Medicine, Shiraz University of Medical Sciences, Shiraz, Iran
- 2Department of Bacteriology and Immunology and the Translational Immunology Research Program (TRIMM), The University of Helsinki and HUSLAB, Helsinki University Hospital, Helsinki, Finland
- 3Autoimmune Diseases Research Center, Shiraz University of Medical Sciences, Shiraz, Iran
Aging is a time-dependent progressive physiological process, which results in impaired immune system function. Age-related changes in immune function increase the susceptibility to many diseases such as infections, autoimmune diseases, and cancer. Different metabolic pathways including glycolysis, tricarboxylic acid cycle, amino acid metabolism, pentose phosphate pathway, fatty acid oxidation and fatty acid synthesis regulate the development, differentiation, and response of adaptive and innate immune cells. During aging all these pathways change in the immune cells. In addition to the changes in metabolic pathways, the function and structure of mitochondria also have changed in the immune cells. Thereby, we will review changes in the metabolism of different innate immune cells during the aging process.
1 Introduction
Aging is a complex process in which time-dependent progressive physiological changes result in impaired biological functions and decreased quality of life (López-Otín et al., 2013). The immune system is one of the major biological systems affected by aging. Age-related changes in the structure and function of the immune system components lead to an increase in the susceptibility of older people to infections, autoimmune diseases, and cancer, a decrease in response to vaccines, and low-grade chronic inflammation (inflammaging) in blood and tissues (Sadighi Akha, 2018). Inflammaging can be triggered by cellular senescence, accumulation of damaged self-debris, impaired autophagy, mitochondrial dysfunction, decline of protein hemostasis (proteostasis), and microbiota dysbiosis (Franceschi and Campisi, 2014; Sanada et al., 2018; Teissier et al., 2022). These events elicit constitutive production of pro-inflammatory cytokines like interleukin-6 (IL-6) and tumor necrosis factor alpha (TNF-α) (Bruunsgaard, 2006; Maggio et al., 2006). The continuous presence of age-related inflammatory responses may cause metabolic dysregulation; in return, the dysregulation can exacerbate inflammaging (Kim et al., 2020). Consequently, inflammaging is a notable risk factor for age-related diseases and death (Franceschi et al., 2000).
Immune cells encounter different metabolic demands based on their state (resting or activated) and tissue environment (different oxygen levels and nutrient accessibility) (O'Neill et al., 2016; Loftus and Finlay, 2016). Overall, six main metabolic pathways regulate the metabolism of immune cells: glycolysis, tricarboxylic acid (TCA) cycle, amino acid metabolism, pentose phosphate pathway, fatty acid oxidation (FAO), and fatty acid synthesis (O'Neill et al., 2016). Aging could affect each of these metabolic pathways in adaptive and innate immune cells. This could lead to changes in immune cell functions and alterations in the microenvironment (Sadighi Akha, 2018; Martin et al., 2021). Some of these will be mentioned below.
Based on different studies, T cells go through metabolic and epigenetic changes during aging. These include decreased glycolysis, mitochondrial biogenesis and one-carbon metabolism, while increasing reactive oxygen species (ROS) (Ron-Harel et al., 2018; Quinn et al., 2019; Quinn et al., 2020; Nian et al., 2021; Møller et al., 2022). Also, studies have revealed that B cells during aging upregulate Glut1 expression and increase glucose uptake, oxidative phosphorylation (OXPHOS), anaerobic glycolysis and FAO (Kurupati et al., 2019; Frasca et al., 2021; Frasca et al., 2022).
Moreover, age-related changes in innate immune cells’ metabolic fitness have been evaluated in various studies. So, this mini-review will sum up the observations and reports about age-related changes in innate immune cell metabolism. We have illustrated these changes in Figure 1 for better understanding. We also provided an overview of age-associated changes in human and mouse innate immune cells in Table 1.
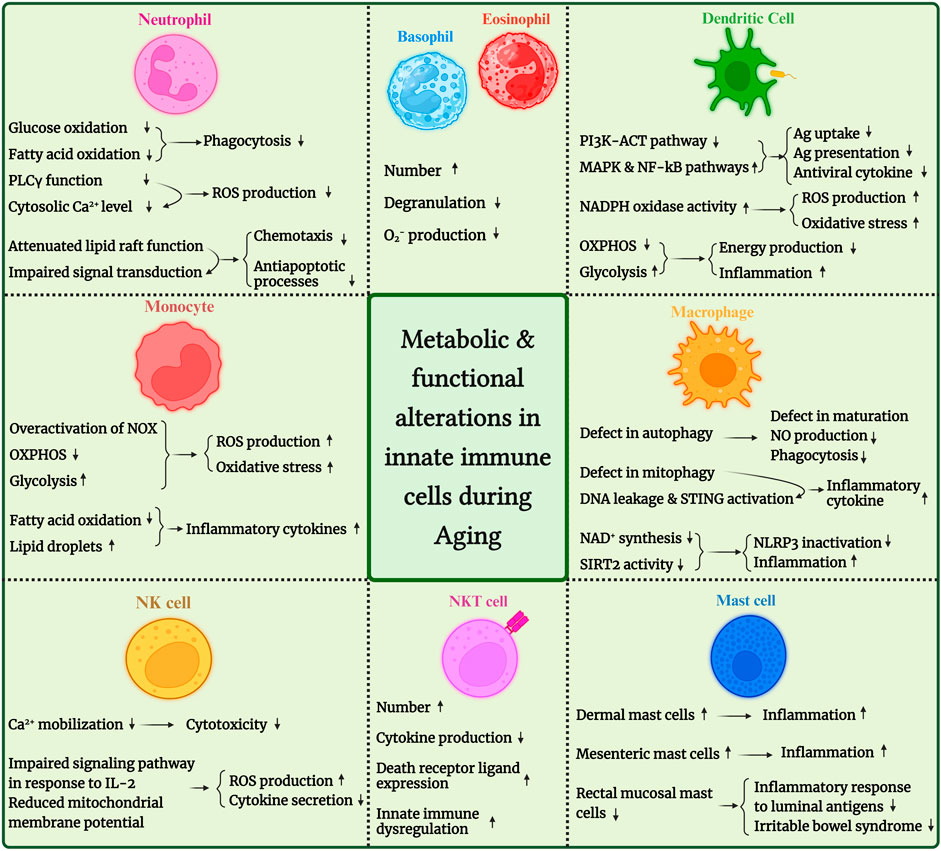
FIGURE 1. An overview of metabolic and functional alterations in innate immune cells during aging. PDK4, pyruvate dehydrogenase kinase 4; SIRT, sirtuin; ROS, reactive oxygen species; NLRP3: NLR family pyrin domain containing 3; STING, stimulator of interferon genes; IP3, inositol triphosphate.
2 Metabolic alterations in innate immune cells
2.1 Neutrophil
Neutrophils are the most abundant leukocytes in the human peripheral blood. They are essential in the first line of defense against invading microorganisms (Curi et al., 2020). The first step for killing microorganisms by the neutrophil is the engulfment of foreign particles by phagocytosis. This process is disrupted in aging because of low levels of taurine. Taurine acts as an antioxidant and plays a vital role in neutrophil phagocytosis. In both human and mice taurine exerts an important impact on energy metabolism. Taurine deficiency impaired complex I activation and subsequent an elevation occurs in NADH/NAD + ratio. This Inhibit the function of dehydrogenase enzymes and reduce glucose oxidation during glycolysis. Lower levels of taurine also reduce PPARα transcription factor and as a result suppress fatty acid oxidation. With these metabolic alterations a reduction occurs in the level of adenosine triphosphate (ATP) in the neutrophils of aged people. As a result, there is an increased energy demand or decreased energy production in these cells. These changes show that how taurine deficiency and subsequent detrimental changes in metabolism attenuate neutrophil function (Richer et al., 2018; Schaffer and Kim, 2018; Tappia et al., 2018; Bae et al., 2022; Miyazaki et al., 2022).
Another problem reported on neutrophils of aged people is related to a dysregulation of intracellular calcium influx. Investigations have revealed a decrease in intracellular calcium influx. This phenomenon has been attributed to a reduced phospholipase C-γ (PLCγ) function and significantly reduced generation of diacylglycerol (DAG) and inositol triphosphate (IP3). Subsequently, less calcium will be released through the IP3 sensitive calcium channel in the endoplasmic reticulum. Lower cytosolic calcium concentrations in stimulated aged neutrophils lead to a reduced phagocytic capacity and diminished ROS production (Lipschitz et al., 1988; Wenisch et al., 2000; Gomez et al., 2008; Immler et al., 2018). Taken together, aging manipulates metabolic activity of neutrophils in a way that attenuates the effector function of these cells.
Neutrophils in both aged people and mouse also seem to have dysfunctional signal transduction. Lipid rafts are part of the phospholipid bilayer membrane and are rich in cholesterol and phospholipid. They are essential for the regulation of signal transmission from different receptors. Lipid raft function may be attenuated in human-aged neutrophils because the physicochemical properties of neutrophil membranes alter with age. It has been found that the fluidity of the neutrophil membrane increases as a consequence of changes in membrane composition. So, the cholesterol content of the neutrophil membrane increases, while membrane phospholipid levels do not change. Moreover, lipid raft distribution is disorganized with aging, which reduces lipid raft accumulation and disrupts downstream signaling events in aged neutrophils (Fulop et al., 2004; Zhang et al., 2022). Thus, signaling through receptors such as granulocyte macrophage-colony stimulating factor (GM-CSF) or formyl-methionyl-leucyl-phenylalanine (fMLP) receptor, activation of the Janus kinase/signal transducer and activator of transcription (JAK/STAT), extracellular signal-related kinases 1 and 2 (ERK1/2), PLCγ/protein kinase C (PKC) and phosphoinositide 3-kinase (PI3K)/protein kinase B (Akt) pathways will be reduced. In turn, this leads to reduced superoxide generation, chemotaxis, and antiapoptotic processes (Tortorella et al., 2006; Panda et al., 2009; Fulop et al., 2014).
In conclusion it seems that age related changes in metabolism correlate with impairment in neutrophil functions. So that neutrophils in elderly individuals and mouse have problems in signal transduction, phagocytosis, energy utilization and production.
2.2 Eosinophil and basophil
Eosinophils and basophils are among polymorphonuclear cells (PMN) that play crucial roles in combating against parasitic infection (Wechsler et al., 2021). Very low evidence is available regarding age associated changes in the function and number of eosinophil and basophil. Higher number of eosinophils have been detected in aged mice (Busse et al., 2007). Analyzing age-related changes in eosinophil function demonstrate a reduction in eosinophil degranulation and superoxide anions production in response to stimuli in the older asthmatic patients (Mathur et al., 2008). The influence of aging on basophil was analyzed in one study and higher number of basophils was detected in bone marrow and spleen of older mice (Van Beek et al., 2018).
2.3 Dendritic cell
Dendritic cells (DCs) are crucial for activating and instructing T cells and creating a bridge between innate and adaptive immunity (Hilligan and Ronchese, 2020). These cells are characterized by their efficient conversion of internalized antigens into peptide-major histocompatibility complexes (p-MHC) required to orchestrate T-cell responses. Age-related changes in DC function have been examined in mice model by Gang Li and his colleagues. They observed an impaired capacity of aged DCs in migration, antigen uptake and presentation, as well as reduced costimulatory molecule expression. They concluded that age-associated defects in DC functions contribute to the impaired adaptive immune response against microbial pathogens (Linton et al., 2005; Li et al., 2012). Furthermore, aging is associated with changes in several signaling pathways in mice and human. Phosphorylation of AKT decreased in aged DCs and subsequently reduced induction of the PI3K pathway. Reduced phosphorylation of AKT in aged DCs may cause overactivation of mitogen-activated protein kinase (MAPK) and nuclear factor-kB (NF-kB) pathways. Impaired induction of interferon regulatory factor 1 (IRF-1) and IRF-7 have also been detected in aged DCs. Defects in these pathways negatively influence DC capacity in T cell activation. Decreased antigen phagocytosis, reduced migration, and impaired production of anti-viral cytokines are among the defects affecting functions in aged DC (Agrawal et al., 2007a; Agrawal et al., 2009; Maletto et al., 2010; Gardner et al., 2017). Prakash et al. investigated the effect of age on DC function. Their results indicated that monocyte-derived DCs from the aged individuals are impaired in their capacity to produce interferon (IFN)-I and IFN-III in response to the influenza virus. They also observed age-associated epigenetic changes in the chromatin structure, which could be linked to reduced gene expression and subsequently reduced cytokine production (Prakash et al., 2013).
In addition to the mentioned problems regarding the DCs during aging, it is worth to mention that reduced gene expression in the mitochondrial electron transport chain also occurs. Aged DCs in both human and mice exhibited reduced mitochondrial membrane potential, energy production, and baseline OXPHOS (Agrawal et al., 2007b; Chougnet et al., 2015; Rahmatpanah et al., 2019). Nevertheless, the expression of glucose and amino acid transporters is upregulated in DCs from aged subjects, which is a way to compensate for reduced energy generation. Moreover, glucose and amino acids are primary activators of mammalian target of rapamycin complex 1 (mTORC1) signaling. mTORC1 regulates anabolic processes and promotes glycolysis and secretory phenotype (many cytokines, chemokines, and growth factors promoting inflammation and tumor growth) in aged DCs. So, mTOR inhibition has increased longevity in mice and human (Papadopoli et al., 2019; Rahmatpanah et al., 2019).
In inflammatory cells, ROS is derived from the electron transport chain and produced by the action of the nicotinamide adenine dinucleotide phosphate (NADPH) oxidase enzyme. In DCs from elderly individuals and old mouse, ROS production is increased, while the activity of ROS converting enzymes is decreased. These changes promote ROS accumulation in aged DCs and consequently oxidation of proteins, carbohydrates, lipids, and nucleic acids, a process known as oxidative stress. Oxidized proteins accumulate and disrupt the function of aged DCs (Linton and Thoman, 2014). It can be concluded that overactivation of MAPK and NF-kB pathways, increased activation of NADPH oxidase enzyme in electron transport chain and subsequent accumulation of ROS and oxidation of macromolecules, reduced oxidative phosphorylation and increased glycolysis pathway are among the age-related changes occur in DC metabolism in both human and mouse and are followed by DC dysfunction.
2.4 Monocyte
Monocytes are circulating blood cells that make up 10% of peripheral leukocytes in humans and 4% in mice. They perform various immune effector functions, such as recognizing pathogens through Toll-like receptors (TLRs) and other pattern recognition receptors (PRRs) and then secreting pro-inflammatory cytokines, presenting antigens, assisting in tissue remodeling and wound healing, and producing anti-inflammatory cytokines and lipid mediators to help reduce inflammation (Guilliams et al., 2018). Monocyte classification is based on CD14 and CD16 expression. The population of human primary monocytes may be divided into three subsets: classical (CD14high/CD16-), intermediate (CD14high/CD16+), and non-classical (CD14low/CD16+) (Ong et al., 2018).
Monocytes are among the phagocytic cells of the innate immune system, which face many changes in aging, such as increased production of cytokines and inflammatory mediators, decreased phagocytosis, changes in the population of subsets and metabolic changes (De Maeyer and Chambers, 2021).
During aging, lower mitochondrial respiratory capacity and higher levels of ROS have been detected in classical monocytes from elderly individuals. Damaged mitochondria or increased activity of NADPH oxidase could be the deriver of elevation in ROS level (Pence and Yarbro, 2018; Saare et al., 2020). On the other hand, in aged monocytes, levels of pyruvate dehydrogenase kinase (PDK4), which is an inhibitor of pyruvate dehydrogenase (PDH), are increased. As a consequence, this causes a shift in the metabolic pathway from OXPHOS to lactate production. In addition, glucose uptake is increased in aged monocytes, which indicates more efficient glycolysis in aged monocytes (Saare et al., 2020). In contrast, one study indicated no variations in glycolysis between young and aged monocytes in ex vivo (Pence and Yarbro, 2019). The monocyte isolation method, along with other possible causes of variance like subject number, population demographics, age ranges, etc., may contribute to these disparate results.
In aged monocytes, levels of enzymes that convert phosphatidylcholine to arachidonic acid are significantly decreased in the aged monocytes. As a result, the production of anti-inflammatory mediators is downregulated (Saare et al., 2020). According to one study, increased quantities of lipid droplets (LDs) were detected in the monocytes of older mouse and people, and this increase was linked to decreased FAO. Also, the pro-inflammatory phenotype of monocytes in older people may be caused by downregulated peroxisome proliferator-activated receptor (PPAR)-alpha. This was positively connected with LD accumulation and rising TNF-α concentration (Wang et al., 2021). In conclusion, glycolysis increased along with elevated in glucose uptake in aged monocytes while OXPHOS decreased and ROS increased. Furthermore, deposition of lipid droplets could contribute to the production of inflammatory cytokines during aging in monocytes.
Altogether, aging is associated with detrimental changes in the metabolism of monocytes. Mitochondrial dysfunction, overproduction of ROS, reduced OXPHOS, increased glycolysis and decreased FAO are among these metabolic alterations that adversely affect monocyte function.
2.5 Macrophage
Macrophages are essential for innate immunity because they serve as sentinels to combat infections, hasten the healing of wounds, and control the emergence of a particular acquired immune response (De Maeyer and Chambers, 2021).
In aged macrophages, autophagy is defective in both mouse and human (Stahl et al., 2018). According to a study, this defect in autophagy is due to increased hypermethylation in the promoter of autophagy-related 5 (ATG5) and microtubule-associated proteins 1A/1B light chain 3 B (LC3B) genes which promote the autophagic process. As a consequence, that causes a decrease in their expression in aged macrophages compared to young macrophages (Khalil et al., 2016). Consequently, the dysfunction of autophagy causes a decrease in the maturation of macrophages, nitric oxide (NO) production, and phagocytic properties (Stranks et al., 2015). In addition to autophagy, mitophagy, a kind of macroautophagy that deletes old and damaged mitochondria, is impaired in aged macrophages from mouse and human. Defects in mitophagy result from defects in mitochondrial polyubiquitination by PTEN-induced kinase 1 (PINK1)/Parkin as well as defects in lysosome biogenesis and function. Due to the defect in mitochondrial mitophagy in aged macrophages, mitochondrial DNA leaks into the cytosol during sterile damage and activates the cyclic GMP-AMP synthase (cGAS)/stimulator of interferon genes (STING) pathway, promoting inflammatory cytokine production (Zhong et al., 2022).
In the context of metabolism, Nicotinamide adenine dinucleotide (NAD+) is an electron acceptor involved in OXPHOS, glycolysis, and many metabolic pathways. It is produced through the de novo, salvage, and Preiss-Handler pathways (Li et al., 2020). NAD+ decreased during aging in macrophages. Blocking the de novo NAD+ synthesis pathway in macrophages induces inflammatory phenotype, impairs OXPHOS, and increases ROS, which indicates the critical role of the de novo NAD+ synthesis pathway in controlling the metabolism of macrophages. Aged macrophages express less quinoline phosphoribosyl transferase (QPRT), as a critical enzyme in the de novo pathway compared to young macrophages. Consequently, decrease the de novo NAD+ synthesis pathway. This phenomenon increased inflammatory phenotype of macrophages and disruption of mitochondrial processes (Minhas et al., 2019). Also, it has been shown that the inflammatory macrophage depends on the production of NAD+ from the salvage pathway. Stimulation of macrophage with lipopolysaccharide (LPS) increases the critical enzyme in the salvage pathway. Boosting the salvage pathway is necessary to prevent DNA damage caused by ROS and maintain the inflammatory phenotype (Cameron et al., 2019). Furthermore, a study infers that aging macrophages shift their NAD+ metabolism from the de novo synthesis pathway to the salvage synthesis pathway (Pence, 2021).
The activity of Sirtuins can be impacted by alteration in NAD+ metabolism since they are NAD+-dependent deacetylases that control critical metabolic pathways in cells. Sirtuin 2 (SIRT2) deacetylates NLR family pyrin domain containing 3 (NLRP3) and inactivates the NLRP3 inflammasome in macrophages. Moreover, induction of the overexpression of SIRT2 in aged macrophages causes a decrease in the production of interleukin-1β (IL-1β) and the activation of NLRP3. In other words, one of the causes of inflammation can be related to the decrease in SIRT2 activity (He et al., 2020). SIRT3 is also an NAD+-dependent deacetylase that affects the function of mitochondrial proteins and OXPHOS. SIRT3 deacetylates the complex I of the electron transport chain. In addition, decreasing de novo NAD+ synthesis reduces SIRT3 activity, leading to impaired OXPHOS (Minhas et al., 2019). In conclusion, the decline in NAD+'s de novo synthesis pathway and changes in NAD+-dependent deacetylases, such as SIRT2 and SIRT3, play critical roles in macrophage function and age-related inflammation.
Defect in autophagy, defect in mitophagy, defect in NAD+ synthesis and NAD+ dependent deacetylases, and defect in OXPHOS are among the disturbance occurred in the metabolism of macrophages in old mouse and elderly individuals. These deleterious changes directly correlate with macrophage dysfunction.
2.6 NK cell
Natural killer (NK) cells are the primary defense lymphocyte against viral infection and represent 10%–15% of peripheral blood lymphocytes, which are responsible for antimicrobial response, adoptive immunity induction, and clearance of senescent cells (Gounder et al., 2018; Solana et al., 2018). In older people, decreased activity of NK cells is associated with more tumor prevalence, lower vaccination efficacy, accumulation of senescent cells, and infection susceptibility, which shorten survival (Miranda et al., 2018).
Release of lytic granules into the immune synapse (IS) is one of the NK cell cytotoxic events, which requires several checkpoints to perform the alteration of phosphoinositide molecules by PLC, calcium mobilization, and polarization of secretory lysosomes to the IS. Calcium ions (Ca2+) release is also required for mitochondria movement to the IS and fusion of lysosomes to the NK cell membrane. After interaction of a receptor with the relevant ligand, the cytoplasmic tail gets phosphorylated, recruits the PI3K, and provides two signaling pathways (Brauning et al., 2022). The first pathway is MAPK and NFκB pathway, which are activated by PI3K and PLC, respectively. The second one is the transformation of phosphatidylinositol 4,5-bisphosphate (PIP2) to phosphatidylinositol (Franceschi and Campisi, 2014; Sanada et al., 2018; Teissier et al., 2022)-triphosphate (PIP3), which leads to the production of IP3, the second messenger (Dustin and Long, 2010). IP3 is essential in this process by stimulating Ca2+ release from the internal store. IP3 production is shown to be significantly reduced in older humans’ NK cells (Mariani et al., 1998). Also, F. Borrego et al. reported that the Ca2+ mobilization in NK cells of older is decreased (Borrego et al., 1999). Therefore, reduced IP3 production and impaired Ca2+ mobilization might contribute to lower NK cell cytotoxicity in older humans (Brauning et al., 2022).
Decreased NK cell cytotoxic functions in elderly people and aged mouse are related to lower secretion of IFN-γ, downregulation of perforin and granzyme, and impaired NK cell cytotoxicity (NKCC) (Judge et al., 2020). IL-2 is a potent provoker of NK cytokine production and NKCC. It was shown that IL-2 could increase the production of IFN-γ and TNF-α from NK cells of older donors, but on a lower scale compared to younger donors (Menees et al., 2021; Brauning et al., 2022). Therefore, the signaling pathway in response to IL-2 might be impaired in older ones. Also, it revealed that increased secretion of IFN-γ from NK cells in response to IL-2 is associated with an increase in mitochondrial mass and membrane potential in peroxisome proliferator-activated receptor-𝛾 coactivator 1- α (PGC-1α)-dependent manner (Miranda et al., 2016). PGC-1α is a member of PGC-1 family, which acts as a transcriptional coactivator. These molecules form a protein complex by engaging nuclear receptors and transcriptional factors to regulate gene expression, which modulates mitochondrial biogenesis and respiratory function (Miranda et al., 2016). D. Miranda et al. indicated that purified NK cells from young donors have the potential to increase the PGC-1α by three folds after IL-2 stimulation. At the same time, there is an altered IL-2 signaling in NK cells of older donors, which affects mitochondrial functions and leads to increased production of ROS (Miranda et al., 2018). ROS is a molecule derived from superoxide (O2−), and eight regions within mitochondria can produce O2− (Sena and Chandel, 2012).
In summary, aging affects NK cell metabolism in different aspects, which can lead to decreased activity. NK cells of older people exhibited an altered response to IL-2 by increasing ROS production instead of increasing expression of PGC-1α. Another alteration of the NK cell in older people is impaired IP3 production and Ca2+ mobilization. These changes lead to lower NK cell cytotoxicity and cytokine production.
2.7 NKT cell
NKT cells as a distinct subset of immune cells are characterized based on the expression of T cell receptor and also some NK cell expressing markers. These cells activate upon recognition of CD1d-lipid complex presented by different immune cell types. NKT cells play important roles in defense against cancer and microbial infections through cytokine production, direct cytotoxicity and induction of apoptosis via death receptor-ligand interaction (Chen and Liao, 2007; Fujii et al., 2013; Bae et al., 2019). Aging is associated with increase in the absolute number and percentage of NKT cells both in the circulation and lymphoid organs. In elderly individuals, NKT cells produce lower levels of TH1 cytokines. and express higher level of death receptor ligand (Plackett et al., 2004; Shaw et al., 2013). Studies performed on mouse model indicate that aging potentiate inflammatory response of NKT cells and promote innate immune dysregulation (Inui et al., 2002; Kawabata et al., 2008).
2.8 Mast cell
Mast cells are central players of allergy reaction and a variety of disease. These cells originate from hematopoietic stem cells in bone marrow and terminally differentiate in peripheral tissues. Mast cells are wildly distributed in different tissues including mucosal and connective tissues (Dahlin et al., 2022; Wagner et al., 2023). Human dermal mast cells increase in quantity with age. Dermis inflammation induced by both external and internal factors such as changes in temperature and air humidity and also mast cell chemoattractant produced in inflammatory process are involved in elevated levels of mast cells in derm (Petrov et al., 2013). Aging-associated inflammatory environment is accompanied by an increase in the number of mast cells located in close proximity to human mesenteric lymphatic vessels (Chatterjee and Gashev, 2012). Aging is associated with a reduction in the numbers of rectal mucosal mast cells. This may influence the low-grade inflammatory response to luminal antigens and contribute to the reduction of irritable bowel syndrome observed in older individuals (Dunlop et al., 2004).
As we discussed in this article, aging is associated with detrimental changes in the metabolism of innate immune cells. Some of these changes are shared among a number of innate immune cells. Reduced function of PLCγ and subsequent lower cytosolic calcium concentrations in aged neutrophils and NK cells, decreased oxidative phosphorylation and increased glycolysis pathway in aged dendritic cells and monocytes can be mentioned as an example of these common metabolic changes. However, inconsistent changes occur in the production of ROS in different innate immune cells. So that aging is accompanied with decreased ROS production in aged neutrophils, eosinophils and basophils. While increased levels of ROS have been detected in aged DCs, monocytes and NK cells. Several factors are involved in these inconsistent changes in ROS production. Lower level of taurine and subsequent disturbed function of electron transport chain complex I as well as disorganized lipid raft distribution and consequent impaired signal transduction are among the causes of reduced ROS production in aged neutrophils. Increased activity of NADPH oxidase enzyme in electron transport chain promotes more ROS production in aged DCs and monocytes. Furthermore, defect in IL2 signaling pathway is associated with reduced mitochondrial membrane potential and more ROS production in aged NK cells.
As a whole, common or inconsistent changes in the metabolism of innate immune cells contribute to multiple functional impairments. Defect in cytotoxicity, phagocytosis, antigen presentation, cytokine production and chemotaxis are among these functional disorders.
3 Consequences of aging on predisposition to diseases
As described in this review, metabolic alterations are one of the many mechanisms that lead to the malfunction of innate immune cells and the production of inflammatory mediators (inflammaging). Subsequently, inflammaging can contribute to the development of several age-related diseases, such as neurodegenerative diseases, rheumatoid arthritis, cancer, cardiovascular, and metabolic diseases (Barbé-Tuana et al., 2020).
Furthermore, mitochondria, as a vital organelle for metabolism, become defective during aging and contribute to diverse aging-related diseases. Impaired oxidative phosphorylation, mitophagy defect, mDNA release, accumulation of ROS, and oxidative stress increase the risk of heart failure, Alzheimer’s disease, osteoarthritis, osteoporosis, and aging-related macular degeneration (AMD) (Guo et al., 2022).
Also, during aging, the immune response to vaccines and pathogens declines due to the dysregulation of innate and adaptive immune systems. Several studies have declared the effect of aging on response to chronic viral infections and the effectiveness of vaccination. They observed the increased severity of viral and bacterial infections and impaired responses to vaccinations (Hainz et al., 2005; Tohme et al., 2011; Goldstein, 2012; Yang et al., 2016). Innate immune cells, especially DCs, are critical to triggering effective immune responses to vaccines. As mentioned in the DC section, the impaired capacity of aged DCs in migration, antigen uptake, and presentation contributes to the impaired adaptive immune response. Indeed, defects in several signaling pathways, like TLRs, negatively influence DC capacity in the expression of costimulatory molecules, cytokine production, and T cell activation. Altogether, aging-related dysfunction of innate immunity accounts for a decrease in elderly persons’ immune response to vaccines and pathogens (Panda et al., 2010; Chen et al., 2022).
4 Conclusion
Aging, as a physiologic progressive process, gives rise to different intrinsic and functional changes in immune cells; in return, these changes, over time, accelerate the aging process. Metabolic pathways possess a pivotal role in the survival and functions of immune cells by regulating energy demands and production. During aging, innate immune cells, as a first-line immunity and activator of adaptive immunity, face various metabolic alterations such as increasing glycolysis, decreasing FAO, and OXPHOS. These metabolic alterations lead to impaired phagocytosis, antiviral cytokine production, antigen presentation, and cytotoxicity of innate immune cells, besides increased production of inflammatory cytokines. Consequently, the immune cells cannot cope with infections and malignant cells, which make aged individuals susceptible to infectious diseases, cancer, and inflammatory diseases. Therefore, targeting these pathways in therapeutic strategies might be an approach to slow down the aging process.
Author contributions
ZS: Investigation, Validation, Writing–original draft. SM: Investigation, Validation, Writing–original draft. FM: Conceptualization, Validation, Writing–original draft. KH: Conceptualization, Validation, Writing–original draft. SM: Validation, Supervision, Writing–review and editing. KK: Supervision, Validation, Writing–review and editing, Conceptualization.
Funding
The author(s) declare that no financial support was received for the research, authorship, and/or publication of this article.
Conflict of interest
The authors declare that the research was conducted in the absence of any commercial or financial relationships that could be construed as a potential conflict of interest.
Publisher’s note
All claims expressed in this article are solely those of the authors and do not necessarily represent those of their affiliated organizations, or those of the publisher, the editors and the reviewers. Any product that may be evaluated in this article, or claim that may be made by its manufacturer, is not guaranteed or endorsed by the publisher.
References
Agrawal, A., Agrawal, S., Cao, J.-N., Su, H., Osann, K., and Gupta, S. (2007a). Altered innate immune functioning of dendritic cells in elderly humans: a role of phosphoinositide 3-kinase-signaling pathway. J. Immunol. 178 (11), 6912–6922. doi:10.4049/jimmunol.178.11.6912
Agrawal, A., Agrawal, S., and Gupta, S. (2007b). Dendritic cells in human aging. Exp. Gerontol. 42 (5), 421–426. doi:10.1016/j.exger.2006.11.007
Agrawal, A., Tay, J., Ton, S., Agrawal, S., and Gupta, S. (2009). Increased reactivity of dendritic cells from aged subjects to self-antigen, the human DNA. J. Immunol. 182 (2), 1138–1145. doi:10.4049/jimmunol.182.2.1138
Bae, E.-A., Seo, H., Kim, I.-K., Jeon, I., and Kang, C.-Y. (2019). Roles of NKT cells in cancer immunotherapy. Archives pharmacal Res. 42, 543–548. doi:10.1007/s12272-019-01139-8
Bae, M., Ahmed, K., and Yim, J.-E. (2022). Beneficial effects of taurine on metabolic parameters in animals and humans. J. Obes. Metabolic Syndrome 31 (2), 134–146. doi:10.7570/jomes21088
Barbé-Tuana, F., Funchal, G., Schmitz, C. R. R., Maurmann, R. M., and Bauer, M. E. (2020). The interplay between immunosenescence and age-related diseases. Semin. Immunopathol. 42 (5), 545–557. Springer. doi:10.1007/s00281-020-00806-z
Borrego, F., Alonso, M. C., Galiani, M. D., Carracedo, J., Ramirez, R., Ostos, B., et al. (1999). NK phenotypic markers and IL2 response in NK cells from elderly people. Exp. Gerontol. 34 (2), 253–265. doi:10.1016/s0531-5565(98)00076-x
Brauning, A., Rae, M., Zhu, G., Fulton, E., Admasu, T. D., Stolzing, A., et al. (2022). Aging of the immune system: focus on natural killer cells phenotype and functions. Cells 11 (6), 1017. doi:10.3390/cells11061017
Bruunsgaard, H. (2006). The clinical impact of systemic low-level inflammation in elderly populations. With special reference to cardiovascular disease, dementia and mortality. Dan. Med. Bull. 53 (3), 285–309.
Busse, P. J., Zhang, T. F., Srivastava, K., Schofield, B., and Li, X. M. (2007). Effect of ageing on pulmonary inflammation, airway hyperresponsiveness and T and B cell responses in antigen-sensitized and-challenged mice. Clin. Exp. Allergy 37 (9), 1392–1403. doi:10.1111/j.1365-2222.2007.02775.x
Cameron, A. M., Castoldi, A., Sanin, D. E., Flachsmann, L. J., Field, C. S., Puleston, D. J., et al. (2019). Inflammatory macrophage dependence on NAD+ salvage is a consequence of reactive oxygen species–mediated DNA damage. Nat. Immunol. 20 (4), 420–432. doi:10.1038/s41590-019-0336-y
Chatterjee, V., and Gashev, A. A. (2012). Aging-associated shifts in functional status of mast cells located by adult and aged mesenteric lymphatic vessels. Am. J. Physiology-Heart Circulatory Physiology 303 (6), H693–H702. doi:10.1152/ajpheart.00378.2012
Chen, J., Deng, J. C., and Goldstein, D. R. (2022). How aging impacts vaccine efficacy: known molecular and cellular mechanisms and future directions. Trends Mol. Med. 28 (12), 1100–1111. doi:10.1016/j.molmed.2022.09.008
Chen, Y.-J., and Liao, H.-F. (2007). NK/NKT cells and aging. Int. J. Gerontology 1 (2), 65–76. doi:10.1016/s1873-9598(08)70025-5
Chougnet, C. A., Thacker, R. I., Shehata, H. M., Hennies, C. M., Lehn, M. A., Lages, C. S., et al. (2015). Loss of phagocytic and antigen cross-presenting capacity in aging dendritic cells is associated with mitochondrial dysfunction. J. Immunol. 195 (6), 2624–2632. doi:10.4049/jimmunol.1501006
Curi, R., Levada-Pires, A. C., Silva, E. B. D., Poma, S. O., Zambonatto, R. F., Domenech, P., et al. (2020). The critical role of cell metabolism for essential neutrophil functions. Cell Physiol. Biochem. 54 (4), 629–647. doi:10.33594/000000245
Dahlin, J. S., Maurer, M., Metcalfe, D. D., Pejler, G., Sagi-Eisenberg, R., and Nilsson, G. (2022). The ingenious mast cell: contemporary insights into mast cell behavior and function. Allergy 77 (1), 83–99. doi:10.1111/all.14881
De Maeyer, R. P. H., and Chambers, E. S. (2021). The impact of ageing on monocytes and macrophages. Immunol. Lett. 230, 1–10. doi:10.1016/j.imlet.2020.12.003
Dunlop, S. P., Jenkins, D., and Spiller, R. C. (2004). Age-related decline in rectal mucosal lymphocytes and mast cells. Eur. J. gastroenterol hepatol. 16 (10), 1011–1015. doi:10.1097/00042737-200410000-00010
Dustin, M. L., and Long, E. O. (2010). Cytotoxic immunological synapses. Immunol. Rev. 235 (1), 24–34. doi:10.1111/j.0105-2896.2010.00904.x
Franceschi, C., Bonafè, M., Valensin, S., Olivieri, F., De Luca, M., Ottaviani, E., et al. (2000). Inflamm-aging. An evolutionary perspective on immunosenescence. Ann. N. Y. Acad. Sci. 908, 244–254. doi:10.1111/j.1749-6632.2000.tb06651.x
Franceschi, C., and Campisi, J. (2014). Chronic inflammation (inflammaging) and its potential contribution to age-associated diseases. J. Gerontol. A Biol. Sci. Med. Sci. 69 (Suppl. 1), S4–S9. doi:10.1093/gerona/glu057
Frasca, D., Diaz, A., Romero, M., and Blomberg, B. B. (2021). Metformin enhances B cell function and antibody responses of elderly individuals with type-2 diabetes mellitus. Front. Aging 2, 715981. doi:10.3389/fragi.2021.715981
Frasca, D., Pallikkuth, S., and Pahwa, S. (2022). Effects of aging on metabolic characteristics of human B cells. J. Acquir Immune Defic. Syndr. 89 (Suppl. 1), S23–S28. doi:10.1097/QAI.0000000000002860
Fujii, S.-I., Shimizu, K., Okamoto, Y., Kunii, N., Nakayama, T., Motohashi, S., et al. (2013). NKT cells as an ideal anti-tumor immunotherapeutic. Front. Immunol. 4, 409. doi:10.3389/fimmu.2013.00409
Fulop, T., Larbi, A., Douziech, N., Fortin, C., Guérard, K. P., Lesur, O., et al. (2004). Signal transduction and functional changes in neutrophils with aging. Aging Cell 3 (4), 217–226. doi:10.1111/j.1474-9728.2004.00110.x
Fulop, T., Le Page, A., Fortin, C., Witkowski, J. M., Dupuis, G., and Larbi, A. (2014). Cellular signaling in the aging immune system. Curr. Opin. Immunol. 29, 105–111. doi:10.1016/j.coi.2014.05.007
Gardner, J. K., Mamotte, C. D. S., Jackaman, C., and Nelson, D. J. (2017). Modulation of dendritic cell and T cell cross-talk during aging: the potential role of checkpoint inhibitory molecules. Ageing Res. Rev. 38, 40–51. doi:10.1016/j.arr.2017.07.002
Goldstein, D. R. (2012). Role of aging on innate responses to viral infections. J. Gerontol. A Biol. Sci. Med. Sci. 67 (3), 242–246. doi:10.1093/gerona/glr194
Gomez, C. R., Nomellini, V., Faunce, D. E., and Kovacs, E. J. (2008). Innate immunity and aging. Exp. Gerontol. 43 (8), 718–728. doi:10.1016/j.exger.2008.05.016
Gounder, S. S., Abdullah, B. J. J., Radzuanb, NEIBM, Zain, FDBM, Sait, N. B. M., Chua, C., et al. (2018). Effect of aging on NK cell population and their proliferation at ex vivo culture condition. Anal. Cell. Pathol (Amst). 2018, 7871814. doi:10.1155/2018/7871814
Guilliams, M., Mildner, A., and Yona, S. (2018). Developmental and functional heterogeneity of monocytes. Immunity 49 (4), 595–613. doi:10.1016/j.immuni.2018.10.005
Guo, J., Huang, X., Dou, L., Yan, M., Shen, T., Tang, W., et al. (2022). Aging and aging-related diseases: from molecular mechanisms to interventions and treatments. Signal Transduct. Target Ther. 7 (1), 391. doi:10.1038/s41392-022-01251-0
Hainz, U., Jenewein, B., Asch, E., Pfeiffer, K. P., Berger, P., and Grubeck-Loebenstein, B. (2005). Insufficient protection for healthy elderly adults by tetanus and TBE vaccines. Vaccine 23 (25), 3232–3235. doi:10.1016/j.vaccine.2005.01.085
He, M., Chiang, H.-H., Luo, H., Zheng, Z., Qiao, Q., Wang, L., et al. (2020). An acetylation switch of the NLRP3 inflammasome regulates aging-associated chronic inflammation and insulin resistance. Cell metab. 31 (3), 580–591. doi:10.1016/j.cmet.2020.01.009
Hilligan, K. L., and Ronchese, F. (2020). Antigen presentation by dendritic cells and their instruction of CD4+ T helper cell responses. Cell Mol. Immunol. 17 (6), 587–599. doi:10.1038/s41423-020-0465-0
Immler, R., Simon, S. I., and Sperandio, M. (2018). Calcium signalling and related ion channels in neutrophil recruitment and function. Eur. J. Clin. investigation 48, e12964. doi:10.1111/eci.12964
Inui, T., Nakagawa, R., Ohkura, S., Habu, Y., Koike, Y., Motoki, K., et al. (2002). Age-associated augmentation of the synthetic ligand-mediated function of mouse NK1. 1 Ag+ T cells: their cytokine production and hepatotoxicity in vivo and in vitro. J. Immunol. 169 (11), 6127–6132. doi:10.4049/jimmunol.169.11.6127
Judge, S. J., Murphy, W. J., and Canter, R. J. (2020). Characterizing the dysfunctional NK cell: assessing the clinical relevance of exhaustion, anergy, and senescence. Front. Cell. Infect. Microbiol. 10, 49. doi:10.3389/fcimb.2020.00049
Kawabata, T., Kinoshita, M., Inatsu, A., Habu, Y., Nakashima, H., Shinomiya, N., et al. (2008). Functional alterations of liver innate immunity of mice with aging in response to CpG-oligodeoxynucleotide. Hepatology 48 (5), 1586–1597. doi:10.1002/hep.22489
Khalil, H., Tazi, M., Caution, K., Ahmed, A., Kanneganti, A., Assani, K., et al. (2016). Aging is associated with hypermethylation of autophagy genes in macrophages. Epigenetics. 11 (5), 381–388. doi:10.1080/15592294.2016.1144007
Kim, D. H., Bang, E., Arulkumar, R., Ha, S., Chung, K. W., Park, M. H., et al. (2020). Senoinflammation: a major mediator underlying age-related metabolic dysregulation. Exp. Gerontol. 134, 110891. doi:10.1016/j.exger.2020.110891
Kurupati, R. K., Haut, L. H., Schmader, K. E., and Ertl, H. C. (2019). Age-related changes in B cell metabolism. Aging (Albany NY) 11 (13), 4367–4381. doi:10.18632/aging.102058
Li, G., Smithey, M. J., Rudd, B. D., and Nikolich-Žugich, J. (2012). Age-associated alterations in CD8α+ dendritic cells impair CD8 T-cell expansion in response to an intracellular bacterium. Aging Cell. 11 (6), 968–977. doi:10.1111/j.1474-9726.2012.00867.x
Li, P.-H., Zhang, R., Cheng, L.-Q., Liu, J.-J., and Chen, H.-Z. (2020). Metabolic regulation of immune cells in proinflammatory microenvironments and diseases during ageing. Ageing Res. Rev. 64, 101165. doi:10.1016/j.arr.2020.101165
Linton, P. J., Li, S. P., Zhang, Y., Bautista, B., Huynh, Q., and Trinh, T. (2005). Intrinsic versus environmental influences on T-cell responses in aging. Immunol. Rev. 205 (1), 207–219. doi:10.1111/j.0105-2896.2005.00266.x
Linton, P. J., and Thoman, M. L. (2014). Immunosenescence in monocytes, macrophages, and dendritic cells: lessons learned from the lung and heart. Immunol. Lett. 162 (1 Pt B), 290–297. doi:10.1016/j.imlet.2014.06.017
Lipschitz, D. A., Udupa, K. B., and Boxer, L. A. (1988). The role of calcium in the age-related decline of neutrophil function. Blood. 71 (3), 659–665. doi:10.1182/blood.v71.3.659.bloodjournal713659
Loftus, R. M., and Finlay, D. K. (2016). Immunometabolism: cellular metabolism turns immune regulator. J. Biol. Chem. 291 (1), 1–10. doi:10.1074/jbc.R115.693903
López-Otín, C., Blasco, M. A., Partridge, L., Serrano, M., and Kroemer, G. (2013). The hallmarks of aging. Cell. 153 (6), 1194–1217. doi:10.1016/j.cell.2013.05.039
Maggio, M., Guralnik, J. M., Longo, D. L., and Ferrucci, L. (2006). Interleukin-6 in aging and chronic disease: a magnificent pathway. J. Gerontol. A Biol. Sci. Med. Sci. 61 (6), 575–584. doi:10.1093/gerona/61.6.575
Maletto, B., Morón, G., and Cristina Pistoresi-Palencia, M. (2010). Innate immune system modulation during aging: contributions of macrophages and dendritic cells. Curr. Immunol. Rev. 6 (4), 329–338. doi:10.2174/1573395511006040329
Mariani, E., Mariani, A. R., Meneghetti, A., Tarozzi, A., Cocco, L., and Facchini, A. (1998). Age-dependent decreases of NK cell phosphoinositide turnover during spontaneous but not Fc-mediated cytolytic activity. Int. Immunol. 10 (7), 981–989. doi:10.1093/intimm/10.7.981
Martin, D. E., Torrance, B. L., Haynes, L., and Bartley, J. M. (2021). Targeting aging: lessons learned from immunometabolism and cellular senescence. Front. Immunol. 12, 714742. doi:10.3389/fimmu.2021.714742
Mathur, S. K., Schwantes, E. A., Jarjour, N. N., and Busse, W. W. (2008). Age-related changes in eosinophil function in human subjects. Chest 133 (2), 412–419. doi:10.1378/chest.07-2114
Menees, K. B., Earls, R. H., Chung, J., Jernigan, J., Filipov, N. M., Carpenter, J. M., et al. (2021). Sex-and age-dependent alterations of splenic immune cell profile and NK cell phenotypes and function in C57BL/6J mice. Immun. Ageing. 18, 3–13. doi:10.1186/s12979-021-00214-3
Minhas, P. S., Liu, L., Moon, P. K., Joshi, A. U., Dove, C., Mhatre, S., et al. (2019). Macrophage de novo NAD+ synthesis specifies immune function in aging and inflammation. Nat. Immunol. 20 (1), 50–63. doi:10.1038/s41590-018-0255-3
Miranda, D., Jara, C., Ibañez, J., Ahumada, V., Acuña-Castillo, C., Martin, A., et al. (2016). PGC-1α-Dependent mitochondrial adaptation is necessary to sustain IL-2-induced activities in human NK cells. Mediat. Inflamm. 2016, 9605253. doi:10.1155/2016/9605253
Miranda, D., Jara, C., Mejias, S., Ahumada, V., Cortez-San Martin, M., Ibañez, J., et al. (2018). Deficient mitochondrial biogenesis in IL-2 activated NK cells correlates with impaired PGC1-α upregulation in elderly humans. Exp. Gerontol. 110, 73–78. doi:10.1016/j.exger.2018.05.014
Miyazaki, T., Ito, T., Baseggio Conrado, A., and Murakami, S. (2022). Editorial for special issue on “regulation and effect of taurine on metabolism”. Metabolites. 12 (9), 795. doi:10.3390/metabo12090795
Møller, S. H., Hsueh, P. C., Yu, Y. R., Zhang, L., and Ho, P. C. (2022). Metabolic programs tailor T cell immunity in viral infection, cancer, and aging. Cell Metab. 34 (3), 378–395. doi:10.1016/j.cmet.2022.02.003
Nian, Y., Iske, J., Maenosono, R., Minami, K., Heinbokel, T., Quante, M., et al. (2021). Targeting age-specific changes in CD4(+) T cell metabolism ameliorates alloimmune responses and prolongs graft survival. Aging Cell. 20 (2), e13299. doi:10.1111/acel.13299
O'Neill, L. A., Kishton, R. J., and Rathmell, J. (2016). A guide to immunometabolism for immunologists. Nat. Rev. Immunol. 16 (9), 553–565. doi:10.1038/nri.2016.70
Ong, S. M., Hadadi, E., Dang, T. M., Yeap, W. H., Tan, C. T., Ng, T. P., et al. (2018). The pro-inflammatory phenotype of the human non-classical monocyte subset is attributed to senescence. Cell Death Dis. 9 (3), 266. doi:10.1038/s41419-018-0327-1
Panda, A., Arjona, A., Sapey, E., Bai, F., Fikrig, E., Montgomery, R. R., et al. (2009). Human innate immunosenescence: causes and consequences for immunity in old age. Trends Immunol. 30 (7), 325–333. doi:10.1016/j.it.2009.05.004
Panda, A., Qian, F., Mohanty, S., van Duin, D., Newman, F. K., Zhang, L., et al. (2010). Age-associated decrease in TLR function in primary human dendritic cells predicts influenza vaccine response. J. Immunol. 184 (5), 2518–2527. doi:10.4049/jimmunol.0901022
Papadopoli, D., Boulay, K., Kazak, L., Pollak, M., Mallette, F., Topisirovic, I., et al. (2019). mTOR as a central regulator of lifespan and aging. F1000Res. 8, F1000 Faculty Rev-998. doi:10.12688/f1000research.17196.1
Pence, B. D. (2021). Recent developments and future perspectives in aging and macrophage immunometabolism. AIMS Mol. Sci. 8 (3), 193–201. doi:10.3934/molsci.2021015
Pence, B. D., and Yarbro, J. R. (2018). Aging impairs mitochondrial respiratory capacity in classical monocytes. Exp. Gerontol. 108, 112–117. doi:10.1016/j.exger.2018.04.008
Pence, B. D., and Yarbro, J. R. (2019). Classical monocytes maintain ex vivo glycolytic metabolism and early but not later inflammatory responses in older adults. Immun. Ageing. 16, 3. doi:10.1186/s12979-019-0143-1
Petrov, V., Vasilyeva, O., Kornilova, N., and Gunin, A. (2013). Age-related changes in mast cells and eosinophils of human dermis. Russ. J. Dev. Biol. 44, 139–143. doi:10.1134/s1062360413030041
Plackett, T. P., Boehmer, E. D., Faunce, D. E., and Kovacs, E. J. (2004). Aging and innate immune cells. J. Leukoc. Biol. 76 (2), 291–299. doi:10.1189/jlb.1103592
Prakash, S., Agrawal, S., Cao, J.-N., Gupta, S., and Agrawal, A. (2013). Impaired secretion of interferons by dendritic cells from aged subjects to influenza: role of histone modifications. Age (Dordr). 35, 1785–1797. doi:10.1007/s11357-012-9477-8
Quinn, K. M., Hussain, T., Kraus, F., Formosa, L. E., Lam, W. K., Dagley, M. J., et al. (2020). Metabolic characteristics of CD8(+) T cell subsets in young and aged individuals are not predictive of functionality. Nat. Commun. 11 (1), 2857. doi:10.1038/s41467-020-16633-7
Quinn, K. M., Palchaudhuri, R., Palmer, C. S., and La Gruta, N. L. (2019). The clock is ticking: the impact of ageing on T cell metabolism. Clin. Transl. Immunol. 8 (11), e01091. doi:10.1002/cti2.1091
Rahmatpanah, F., Agrawal, S., Scarfone, V. M., Kapadia, S., Mercola, D., and Agrawal, A. (2019). Transcriptional profiling of age-associated gene expression changes in human circulatory CD1c+ myeloid dendritic cell subset. J. Gerontol. A Biol. Sci. Med. Sci. 74 (1), 9–15. doi:10.1093/gerona/gly106
Richer, B. C., Salei, N., Laskay, T., and Seeger, K. (2018). Changes in neutrophil metabolism upon activation and aging. Inflammation. 41 (2), 710–721. doi:10.1007/s10753-017-0725-z
Ron-Harel, N., Notarangelo, G., Ghergurovich, J. M., Paulo, J. A., Sage, P. T., Santos, D., et al. (2018). Defective respiration and one-carbon metabolism contribute to impaired naive T cell activation in aged mice. Proc. Natl. Acad. Sci. 115 (52), 13347–13352. doi:10.1073/pnas.1804149115
Saare, M., Tserel, L., Haljasmägi, L., Taalberg, E., Peet, N., Eimre, M., et al. (2020). Monocytes present age-related changes in phospholipid concentration and decreased energy metabolism. Aging Cell. 19 (4), e13127. doi:10.1111/acel.13127
Sadighi Akha, A. A. (2018). Aging and the immune system: an overview. J. Immunol. Methods. 463, 21–26. doi:10.1016/j.jim.2018.08.005
Sanada, F., Taniyama, Y., Muratsu, J., Otsu, R., Shimizu, H., Rakugi, H., et al. (2018). Source of chronic inflammation in aging. Front. Cardiovasc Med. 5, 12. doi:10.3389/fcvm.2018.00012
Schaffer, S., and Kim, H. W. (2018). Effects and mechanisms of taurine as a therapeutic agent. Biomol. Ther. 26 (3), 225–241. doi:10.4062/biomolther.2017.251
Sena, L. A., and Chandel, N. S. (2012). Physiological roles of mitochondrial reactive oxygen species. Mol. Cell. 48 (2), 158–167. doi:10.1016/j.molcel.2012.09.025
Shaw, A. C., Goldstein, D. R., and Montgomery, R. R. (2013). Age-dependent dysregulation of innate immunity. Nat. Rev. Immunol. 13 (12), 875–887. doi:10.1038/nri3547
Solana, C., Tarazona, R., and Solana, R. (2018). Immunosenescence of natural killer cells, inflammation, and Alzheimer's disease. Int. J. Alzheimer's Dis. 2018, 3128758. doi:10.1155/2018/3128758
Stahl, E. C., Haschak, M. J., Popovic, B., and Brown, B. N. (2018). Macrophages in the aging liver and age-related liver disease. Front. Immunol. 9, 2795. doi:10.3389/fimmu.2018.02795
Stranks, A. J., Hansen, A. L., Panse, I., Mortensen, M., Ferguson, D. J., Puleston, D. J., et al. (2015). Autophagy controls acquisition of aging features in macrophages. J. innate Immun. 7 (4), 375–391. doi:10.1159/000370112
Tappia, P. S., Adameova, A., and Dhalla, N. S. (2018). Attenuation of diabetes-induced cardiac and subcellular defects by sulphur-containing amino acids. Curr. Med. Chem. 25 (3), 336–345. doi:10.2174/0929867324666170705115207
Teissier, T., Boulanger, E., and Cox, L. S. (2022). Interconnections between inflammageing and immunosenescence during ageing. Cells. 11 (3), 359. doi:10.3390/cells11030359
Tohme, R. A., Awosika-Olumo, D., Nielsen, C., Khuwaja, S., Scott, J., Xing, J., et al. (2011). Evaluation of hepatitis B vaccine immunogenicity among older adults during an outbreak response in assisted living facilities. Vaccine. 29 (50), 9316–9320. doi:10.1016/j.vaccine.2011.10.011
Tortorella, C., Simone, O., Piazzolla, G., Stella, I., Cappiello, V., and Antonaci, S. (2006). Role of phosphoinositide 3-kinase and extracellular signal-regulated kinase pathways in granulocyte macrophage–colony-stimulating factor failure to delay fas-induced neutrophil apoptosis in elderly humans. Journals Gerontology Ser. A Biol. Sci. Med. Sci. 61 (11), 1111–1118. doi:10.1093/gerona/61.11.1111
Van Beek, A. A., Fransen, F., Meijer, B., De Vos, P., Knol, E. F., and Savelkoul, H. F. (2018). Aged mice display altered numbers and phenotype of basophils, and bone marrow-derived basophil activation, with a limited role for aging-associated microbiota. Immun. Ageing. 15 (1), 32–11. doi:10.1186/s12979-018-0135-6
Wagner, A., Alam, S. B., and Kulka, M. (2023). The effects of age, origin, and biological sex on rodent mast cell (BMMC and MC/9) and basophil (RBL-2H3) phenotype and function. Cell. Immunol. 391, 104751. doi:10.1016/j.cellimm.2023.104751
Wang, M., Yan, Y., Zhang, Z., Yao, X., Duan, X., Jiang, Z., et al. (2021). Programmed PPAR-α downregulation induces inflammaging by suppressing fatty acid catabolism in monocytes. iScience. 24 (7), 102766. doi:10.1016/j.isci.2021.102766
Wechsler, M. E., Munitz, A., Ackerman, S. J., Drake, M. G., Jackson, D. J., Wardlaw, A. J., et al. (2021). Eosinophils in health and disease: a state-of-the-art review. Mayo Clin. Proc. 96 (10), 2694–2707. Elsevier. doi:10.1016/j.mayocp.2021.04.025
Wenisch, C., Patruta, S., Daxböck, F., Krause, R., and Hörl, W. (2000). Effect of age on human neutrophil function. J. Leukoc. Biol. 67 (1), 40–45. doi:10.1002/jlb.67.1.40
Yang, S., Tian, G., Cui, Y., Ding, C., Deng, M., Yu, C., et al. (2016). Factors influencing immunologic response to hepatitis B vaccine in adults. Sci. Rep. 6, 27251. doi:10.1038/srep27251
Zhang, S., Zhu, N., Gu, J., Li, H.-F., Qiu, Y., Liao, D.-F., et al. (2022). Crosstalk between lipid rafts and aging: new frontiers for delaying aging. Aging Dis. 13 (4), 1042–1055. doi:10.14336/AD.2022.0116
Keywords: aging, innate immunity, metabolic pathways, immunometabolism, age-related diseases
Citation: Saleh Z, Mirzazadeh S, Mirzaei F, Heidarnejad K, Meri S and Kalantar K (2024) Alterations in metabolic pathways: a bridge between aging and weaker innate immune response. Front. Aging 5:1358330. doi: 10.3389/fragi.2024.1358330
Received: 19 December 2023; Accepted: 06 February 2024;
Published: 05 March 2024.
Edited by:
Brandt D. Pence, University of Memphis, United StatesReviewed by:
Roel De Maeyer, University of Oxford, United KingdomCopyright © 2024 Saleh, Mirzazadeh, Mirzaei, Heidarnejad, Meri and Kalantar. This is an open-access article distributed under the terms of the Creative Commons Attribution License (CC BY). The use, distribution or reproduction in other forums is permitted, provided the original author(s) and the copyright owner(s) are credited and that the original publication in this journal is cited, in accordance with accepted academic practice. No use, distribution or reproduction is permitted which does not comply with these terms.
*Correspondence: Kurosh Kalantar, a2FsYW50YXJrQHN1bXMuYWMuaXI=
†These authors have contributed equally to this work and share first authorship