- Institute for Molecular Biosciences, Faculty of Biosciences, Goethe University, Frankfurt, Germany
Biomembranes fulfill several essential functions. They delimitate cells and control the exchange of compounds between cells and the environment. They generate specialized cellular reaction spaces, house functional units such as the respiratory chain (RC), and are involved in content trafficking. Biomembranes are dynamic and able to adjust their properties to changing conditions and requirements. An example is the inner mitochondrial membrane (IMM), which houses the RC involved in the formation of adenosine triphosphate (ATP) and the superoxide anion as a reactive oxygen species (ROS). The IMM forms a characteristic ultrastructure that can adapt to changing physiological situations. In the fungal aging model Podospora anserina, characteristic age-related changes of the mitochondrial ultrastructure occur. More recently, the impact of membranes on aging was extended to membranes involved in autophagy, an important pathway involved in cellular quality control (QC). Moreover, the effect of oleic acid on the lifespan was linked to basic biochemical processes and the function of membranes, providing perspectives for the elucidation of the mechanistic effects of this nutritional component, which positively affects human health and aging.
1 Introduction
Aging of biological systems is a complex process leading to functional degeneration and, ultimately, the death of the system. It is under the control of genetic, environmental, and stochastic factors. Over the many years of intensive research using different biological systems ranging from unicellular organisms to the human species, several aging hallmarks such as molecular damage, genetic instabilities, impairments in repair and degradation, telomere shortening, and mitochondrial dysfunction were identified (López-Otín et al., 2013; Kennedy et al., 2014; Szklarczyk et al., 2014).
Podospora anserina is a multicellular filamentous ascomycete closely related to the unicellular ascomycete Saccharomyces cerevisiae (baker’s yeast). The life cycle of P. anserina starts with the germination of an ascospore, the product of sexual reproduction, and the formation of a vegetation body (mycelium) that consists of a network of branched filamentous cells called hyphae, which grow on their tips (Figure 1). After a strain-specific growth period, the hyphal tip growth slows down until it comes to a complete stop, and the hyphae burst at their tips (Rizet, 1953). P. anserina was intensively used as an experimental model to unravel the molecular basis of organismic aging, and a strong mitochondrial etiology of aging was demonstrated early. Most importantly, the reorganization of the mitochondrial DNA (mtDNA) during the lifespan was found to lead to molecular degeneration, causing the death of the system. The stabilization of mtDNA was found to be a key to extending the lifespan (Osiewacz et al., 1989). Other specific genetic manipulations, including those lowering the cellular load of reactive oxygen species (ROS) or improving molecular pathways involved in mitochondrial quality control, were uncovered as being effective in increasing the lifespan of P. anserina. The various experimental studies are discussed in different comprehensive reviews (Esser and Tudzynski, 1980; Osiewacz, 2002; Scheckhuber and Osiewacz, 2008; Hamann and Osiewacz, 2018; Osiewacz and Schürmanns, 2021).
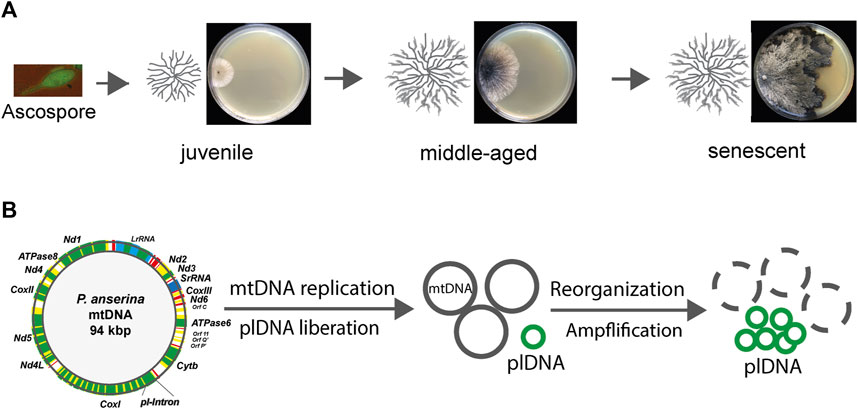
FIGURE 1. Age-related reorganization of mtDNA during aging of Podospora anserina. (A) Starting from a unicellular ascospore, the product of sexual reproduction, a juvenile mycelium develops that grows at the periphery. After a strain-specific period of growth, it reaches a senescent stage, at which growth ceases and ultimately stops. Finally, the filamentous cells at the periphery burst. The figure shows a developing mycelium schematically (left) on an agar plate containing a solid growth substrate. (B) Schematical alterations of the mtDNA. In juvenile cultures, the mtDNA is a circular molecule of 94 kbp, with 13 genes (green) encoding respiratory chain proteins, clusters coding mitochondrial tRNAs (red), and two rRNA genes (blue). The coding sequences of some genes are interrupted by intron sequences (yellow). During aging, the first intron (pl-intron) of cytochrome c oxidase gene subunit I (CoxI) is liberated and amplified (plDNA). This molecule acts a mutator and leads to the deletion of large parts of the mtDNA.
In this review, I will focus on aspects from which an important role of biomembranes and their building blocks in aging emerged. I will first summarize the early work that uncovered the important role of the IMM, which houses the RC as a key protein complex to carry out the bioenergetic function of mitochondria. Next, I will discuss age-related dynamic changes in the mitochondrial architecture and morphology and the role of biomembranes in mitochondrial quality control. Finally, I will deal with recent studies linking cellular membrane fluxes and metabolic responses to alterations in nutrition. These rather new findings provide perspectives for more detailed investigations, including careful translational research to test the situation in diverse organisms, including the complex human species with its many different organs, tissues, and cells.
2 Respiration
Soon after the first description of P. anserina aging in 1953, genetic investigations revealed an extrachromosomal basis of this process (Rizet, 1953; Marcou, 1961). Subsequently, mitochondria were identified to carry the corresponding genetic traits. Specifically, it was found that the mitochondrial DNA (mtDNA) becomes reorganized during aging (Figure 1). A part of this DNA, which was later demonstrated to exactly correspond to the first intron in the gene coding for subunit I of cytochrome c oxidase (COXI), is liberated from mtDNA and becomes amplified. This DNA is a covalently closed circular DNA resembling the structure of bacterial plasmids and, therefore, was termed plasmid-like DNA (plDNA) or, due to its accumulation in senescent cultures, αsen DNA (Stahl et al., 1978; Cummings et al., 1979; Kück et al., 1981; Osiewacz and Esser, 1984). This genetic element behaves like a mutator and is able to reintegrate into the mtDNA, generating large sequence duplications between which genetic reorganizations can occur (Sellem et al., 1993). As a consequence, large parts of the mtDNA are deleted during aging (Kück et al., 1985) (Figure 1). The mtDNA encodes 13 essential proteins of three RC complexes (I, III, and IV) and the ATP-synthase complex, where most of the cellular energy unit ATP is generated by oxidative phosphorylation (OXPHOS). RC complex II is exclusively encoded by the nuclear DNA. In addition, a set of tRNAs and two rRNAs are encoded by the mtDNA.
In addition to ATP, the RC generates superoxide anions (in short, superoxide), charged ROS that cannot directly cross the phospholipid bilayers of membranes. Superoxide can be converted to hydrogen peroxide, which is uncharged and membrane-permeable (Fridovich, 1999; Sies, 2017; Osiewacz and Schürmanns, 2021). Hydrogen peroxide can be detoxified to water by peroxidases or catalase. Alternatively, in the presence of copper (I) and iron (II), hydrogen peroxide can give rise to the formation of the hydroxyl radical, which is highly reactive and very effective in causing damage to all kinds of molecules in its immediate neighborhood. For this ROS, no enzymatic detoxification system exists. Overall, the activity of the RC is both essential and potentially dangerous.
Respiration in P. anserina is very flexible. Impairments of individual components of the RC can be overcome by the induction of genes coding for alternative RC components. Such cellular responses provided very important insights into the role of mitochondria in lifespan control. For example, in mutants such as ex1 and ex2, large parts of the mtDNA, including the pl-intron, are deleted (Kück et al., 1985; Schulte et al., 1988; Schulte et al., 1989). These mutants appear to be immortal since they were cultivated for more than 20 years instead of only a few weeks as the wild type without expressing the senescence syndrome. Due to the deletion of the gene encoding cytochrome c oxidase subunit I (COXI), standard COX-dependent respiration is not possible. Instead, respiration proceeds via an alternative terminal oxidase (AOX), which is integrated into the inner mitochondrial membrane (IMM). The gene encoding this protein is induced in a variety of RC mutants of P. anserina. The AOX accepts electrons from the ubiquinol pool in the IMM and transfers them directly to oxygen. Following this route, complex III, which is a major generator of superoxide, and complex IV of the cytochrome c-dependent standard RC are bypassed. As a consequence, less ROS are generated. Since ROS are the main contributors to the aging process, the process of the corresponding mutants is altered. The exact way in which ROS act in the control of aging is still a matter of debate (Harman, 1972; Zintel et al., 2010; Hekimi et al., 2011; Barja, 2019). In P. anserina, a strong impact of ROS generation by the RC was repeatedly demonstrated (Borghouts et al., 2002a; Borghouts et al., 2002b; Gredilla et al., 2006; Scheckhuber et al., 2011).
3 Mitochondrial ultrastructure
The aging of P. anserina is strongly linked to the activity of the RC, which is localized in the specialized invaginations of the IMM called cristae. The architecture of this part of the IMM, the cristal membrane (CM), is established by protein complexes and phospholipids. In P. anserina, pronounced alterations of the ultrastructure occur during wild-type aging. These changes can be induced by specific experimental interventions (Figure 2).
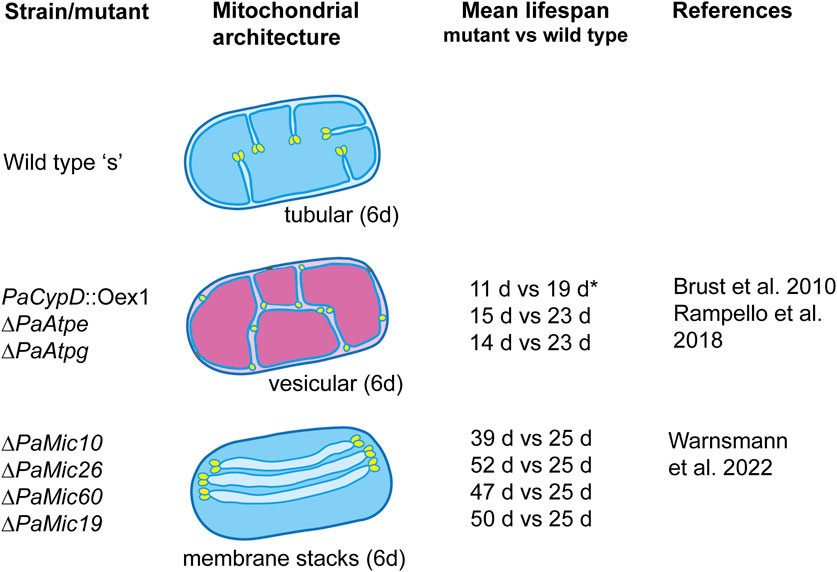
FIGURE 2. Architecture of mitochondria in 6-day-old (juvenile) and 20-day-old (senescent) mycelia of the wild-type “s” and of selected lifespan mutants of Podospora anserina. The altered ultrastructure has a pronounced effect on the mitochondrial function and lifespan. Functional mitochondria are indicated in blue. Functionally impaired mitochondria are indicated in magenta. The mean lifespan of each mutant is compared to the lifespan of the wild type grown under the same growth conditions (i.e., medium and incubation temperature). All strains, except PaCypD_Oex1 (grown on the PASM medium), were grown on the standard M2 medium. The medium composition is described in the indicated references.
The first evidence of the impact of the mitochondrial ultrastructure on aging was observed in a mutant in which the gene coding for cyclophilin D (PaCYPD), a peptidyl prolyl-cis, trans-isomerase that increases in abundance during aging of the P. anserina wild type (Groebe et al., 2007), was overexpressed (Brust et al., 2010). The protein is involved in the opening of the mitochondrial permeability transition pore (mPTP), a large protein complex of unsolved biochemical structure (Halestrap, 2005; Bernardi et al., 2023), which is located in the IMM and is active in the induction of programmed cell death (PCD). The PaCypD overexpressor mutant is characterized by a much reduced lifespan. In comparison to the wild type, the mean lifespan of two independent overexpressors was reduced by 61% and 50%, respectively. Most remarkably, in mutant cultures that are 6 days old, a young age in the wild type, the mitochondrial ultrastructure is already like that in senescent mitochondria. Instead of containing tubular cristae, the mitochondrial matrix is filled with vesicles (Brust et al., 2010). A thorough analysis of wild-type mitochondria from cultures of different ages unraveled the dynamic reorganization of the IMM that occurs during aging (Daum et al., 2013). Mitochondria from young cultures contain typical lamellar cristae reaching out into the matrix (Figure 2). At the tips of the cristae, rows of F1Fo-ATP-synthase dimers are located. At the cristae base, the cristae junction (CJ), large protein complexes called the “mitochondrial contact site and cristae organization system” (MICOS), consisting of an MIC10 and MIC60 subcomplex, are involved in negative curvature formation and in establishing contact of the IMM with the outer mitochondrial membrane (OMM) (Marschall et al., 2022; Warnsmann et al., 2022). During wild-type aging, it was shown that the F1Fo-ATP-synthase dimers dissociate, the tubular cristae recede, and finally, the IMM forms a vesicular (reticular) system of membranes filling the mitochondrial matrix space. Moreover, the IMM and OMM were occasionally found to be in close contact and connected by an electron-dense material of unknown composition. At these contact sites, the OMM can rupture, releasing the matrix vesicle into the cytoplasm. This release of mitochondrial material induces PCD, which in P. anserina is executed by metacaspases and mitochondrial components such as apoptosis-inducing factor 1 (PaAIP1) (Hamann et al., 2007; Hamann et al., 2008; Strobel and Osiewacz, 2013; Minina et al., 2020).
The critical impact of the F1Fo-ATP-synthase dimers and mitochondrial ultrastructure on aging was further underlined by a study of two mutants in which the two assembly factors PaATPE and PaATPG of the ATP-synthase dimers complex were ablated (Figure 2). Mitochondria from 6-day-old cultures of these mutants are characterized by a vesicular ultrastructure, impaired mitochondrial function, and a mean lifespan that is reduced by 33% and 42%, respectively (Rampello et al., 2018).
The age-related remodeling from lamellar cristae to a reticulate inner membrane system requires changes at the base of the cristae, the cristae junctions, and the MICOS. Two recent studies unraveled this impact with some unexpected and surprising results. The ablation of four of the five identified proteins with homology to yeast MIC components resulted in changes of the mitochondrial architecture, affected mitochondrial functions, and caused a pronounced increase of 56%–108% of the mean wild-type lifespan. The majority of mitochondria from PaMic10-, PaMic19-, PaMic60-, and PaMic26-deleted strains contain stacks of floating tubular cristae that are not connected to the inner boundary membrane (IBM), the part of the IMM connected to the CM (Figure 2). The PaMic12-deletion strain an exception with wild-type-like cristae, filamentous mitochondria, and a mean lifespan that does not differ from that of the wild type (Warnsmann et al., 2022). Each of the free-floating tubular membranes contains two tips generated by the convex membrane curvature. Although not firmly shown yet, this curvature is likely to be generated by rows of F1Fo-ATP-synthase dimers, as they were demonstrated at the tips of typical wild-type cristae that are connected to the IBM (Davies et al., 2012; Daum et al., 2013). This situation may lead to strong energetic consequences that contribute to the unexpected lifespan extension of the mutants.
The MICOS contains two subcomplexes, MIC10 and MIC60. In P. anserina, three genes encoding homologs of PaMIC10 and its putative regulator proteins PaMIC26 and PaMIC27 were identified. A MIC12 homolog of the yeast MIC10 subcomplex seems to be missing in P. anserina. Oligomerization of MIC10 is known to lead to the concave curvature of the IMM at the cristae junctions. The MIC60 subcomplex is constituted by two proteins, MIC60 and its regulatory subunit MIC19, which are known to form contact sites between the IMM and the OMM in yeast and mammals. The homologs of P. anserina are PaMIC60 and PaMIC19, respectively. Simultaneous deletion of the PaMic10 and PaMic60 gene resulted in a synergistical lifespan increase, indicating that the two MIC subcomplexes affect two independent pathways that impact aging. Subsequent analyses revealed that the lifespan-extending effect of PaMIC10 mutants results from a mild induction of oxidative stress leading to mitohormesis, an adaptive response, as it is also known in other organisms such as Caenorhabditis elegans (Maglioni et al., 2014; Schiavi et al., 2015), which are beneficial for the organism and may lead to an increased lifespan (Ristow and Zarse, 2010; Yun and Finkel, 2014; Barcena et al., 2018). This conclusion is supported by the addition of the antioxidant ascorbic acid to the growth medium, which reverts longevity of the PaMic10 and PaMic26 mutants. Moreover, while 80 μM of the superoxide generator paraquat increased the lifespan of the wild type, it decreased the lifespan of the two PaMic10 subcomplex-deleted mutants. This suggests that in these mutants, the basic ROS levels were higher than in the wild type, and exogenous paraquat increased the ROS levels beyond beneficial and hormetic levels. In contrast, the effect on the lifespan of the PaMic60 subcomplex mutants and the wild type by paraquat did not differ, and ascorbic acid added to the growth medium decreased the lifespan of the mutants to the wild-type levels (Warnsmann et al., 2022). This suggests that the lifespan-increasing effect in PaMic60 subcomplex mutants is not due to a ROS-dependent mitohormetic response.
A lipidomic analysis revealed that the mitochondrial phospholipid profile and the acyl composition of the mitochondrial signature phospholipid cardiolipin (CL) differ from those of wild-type mitochondria. CL is a phospholipid synthesized in the mitochondria by cardiolipin synthase (CRD) as premature CL (pCL) that subsequently is remodeled to monolysocardiolipin (MMCL) by phospholipase and finally by trans-acylase to mature CL (mCL). In P. anserina, CRD and phospholipid homeostasis are regulated by the IMM-bound protease PaIAP, which adapts membrane plasticity in response to natural fluctuations of environmental conditions (i.e., growth temperature) (Löser et al., 2021). Depending on the number and kind of acyl residues, different forms of CL exist. In the mitochondria of the PaMic10 and PaMic26 mutants, compared to the wild type, CL72:8, which contains four linoleic acids, was found to be increased. In mammals, this CL was linked to enhanced mitochondrial function (Stefanyk et al., 2010; Oemer et al., 2020; Oemer et al., 2021). In addition to its impact on the enzymatic functions (e.g., of the RC), the cone-shaped IMM phospholipid CL contributes to membrane curvature formation. Thus, the changed phospholipid composition found in PaMic60-mutants is linked to changes in ultrastructure, affects mitochondrial membrane fluidity and enzyme activity, and contributes to the observed increase in lifespan.
4 Mitochondrial morphology
Starting from a single ascospore of P. anserina, there develops a multicellular vegetation body that requires an increase in the number and distribution of mitochondria. This process proceeds via the incorporation of the membrane material and of proteins into the IMM and OMM of the existing mitochondria. In this way, small ellipsoid mitochondria grow to filamentous units, which subsequently may divide. Depending on the physiological situations, individual mitochondria can also fuse to form larger filaments or branched filamentous networks. Mitochondrial fission and fusion are processes depending on the dynamic reorganization of the two mitochondrial membranes. They are controlled by nuclear encoded proteins (Shaw and Nunnari, 2002; Westermann, 2008; Klecker and Westermann, 2021).
In P. anserina, it was found that the mitochondrial morphology changes during aging (Figure 3) from filamentous to ellipsoid. The latter morphotype accumulates in old age as a result of increased fission resulting from an increased expression of the PaDnm1 gene, which encodes the mitochondrial dynamin-like protein PaDNM1, a homolog of human DRP1. The deletion of PaDnm1 revealed that the process of fission is strongly delayed and the mitochondria remain filamentous most of the time during the lifespan of the mutant. Only in senescent cultures, a PaDNM1-independent fragmentation occurs. As indicated by no differences in the growth rate and fertility, the fitness of the mutant is not affected, demonstrating that it is the healthy period of the lifetime, the healthspan, which is extended from 22 days of the wild type to 244 days in the mutant (Scheckhuber et al., 2007). This healthspan extension occurs along with a decrease in cellular ROS generation and a delayed release of hydrogen peroxide from the mycelium to the growth medium. Moreover, the PaDnm1-deleted mutant was found to be more resistant to the induction of apoptosis by the apoptosis elicitor etoposide. The observed effects on mitochondrial morphology and lifespan in P. anserina were also observed in a Dnm1-deleted mutant of S. cerevisiae (Scheckhuber et al., 2007). The impact of the mitochondrial dynamics on the aging of P. anserina was further supported by a subsequent study in which the abundance of other fusion and fission proteins was increased (Scheckhuber et al., 2008). Complementary to the lifespan-extending effect of the PaDnm1-deleted strain, the overexpression of this gene had a lifespan-shortening effect. Moreover, while the overexpression of another fission gene, PaFis1, did not show any effect on the lifespan, the double-mutant overexpressing PaDnm1 and PaFis1 led to a reduction of the lifespan beyond that of the PaDnm1 overexpressor. However, in the overexpression study, the overexpression of another potential fission gene, PaMdv1, and the fusion gene PaFzo1 did not show any effect on the lifespan although the mitochondrial morphology was changed.
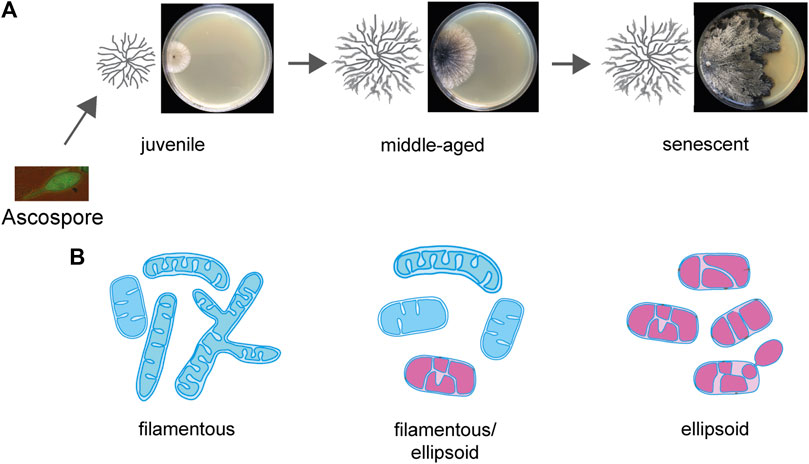
FIGURE 3. Age-related alterations of mitochondrial morphology during aging of Podospora anserina. (A) Morphology of an aging Podospora anserina culture grown on a solid medium. (B) Age-related changes in mitochondria. In juvenile cultures, the mitochondrial morphotype is filamentous, containing tubular cristae. In senescent mitochondria, the morphotype is predominantly ellipsoid and contains a vesicular IMM system. Fully functional mitochondria are indicated in blue, and functionally impaired mitochondria are indicated in magenta.
5 Membrane trafficking and autophagy
Another important role of biomembranes with a strong impact on aging is related to autophagy, the controlled degradation of surplus or damaged cellular components by the lysosome or the vacuole. In P. anserina, it is the vacuole, as a cellular compartment enclosed by a single phospholipid bilayer, that performs this function. After induction, cellular components designated for degradation are either directly taken up via invaginations of the vacuolar membrane (microautophagy) or by the fusion of cargo-containing vesicles, termed autophagosomes, with the vacuolar membrane (macroautophagy). In P. anserina, autophagy is a “longevity assurance mechanism” because its inactivation via the deletion of essential genes controlling the pathway leads to a lifespan reduction (Knuppertz et al., 2014; Henkel et al., 2020). One such gene is PaAtg24 coding for the sorting nexin PaATG24, a homolog of human SNX4. Sorting nexins are known to be involved in vesicle transport, membrane trafficking, and protein sorting. The ablation of PaATG24 impacts the morphology and size of the vacuoles, which are much smaller than those in the wild type. Moreover, non-selective (general autophagy) and selective autophagy of peroxisomes (pexophagy) and mitochondria (mitophagy) are also affected. General autophagy and pexophagy are almost completely blocked, and mitophagy is slightly decreased. During aging, a PaATG24-independent form of mitophagy is induced, which, however, appears not to be efficient enough to remove the dysfunctional mitochondria to prevent aging (Henkel et al., 2020). Subsequently, it was found that the ablation of the sorting nexin leads to mislocalization of PaSNC1, a “vesicle-associated receptor protein” (v-SNARE) that plays a key role in proper membrane–vesicle fusion (Ma et al., 2017). As a consequence, endosomes accumulate in the cytosol, membranes needed for the vacuole proliferation become limited, and the vacuole size and the efficiency of autophagy are strongly reduced. This scenario is supported by experiments in which P. anserina wild-type and mutant strains were grown on a medium in which the fermentable carbon source dextrin, which is metabolized by glycolysis and the Krebs cycle, was replaced by oleic acid. Metabolization of his carbon source requires peroxisomes (Figure 4). Most interestingly, an oleic acid diet led to a mean lifespan extension of the wild type from 24 to 30 days and from 19 to 37 days of the PaAtg24-deleted mutant (Schürmanns et al., 2022). This effect occurs along with the formation of lipid droplets, which are used for oleate uptake and its transport to peroxisomes for metabolization. Lipid droplets may also fuse with the vacuole, providing membranes for the proliferation of this organelle to the size of the wild-type vacuoles. As a consequence, efficient autophagy, as an important quality-control pathway, is restored in the mutant, contributing to the lifespan extension of the PaAtg24-deleted mutant, which is short-lived when grown on a standard growth medium with fermentable carbon sources.
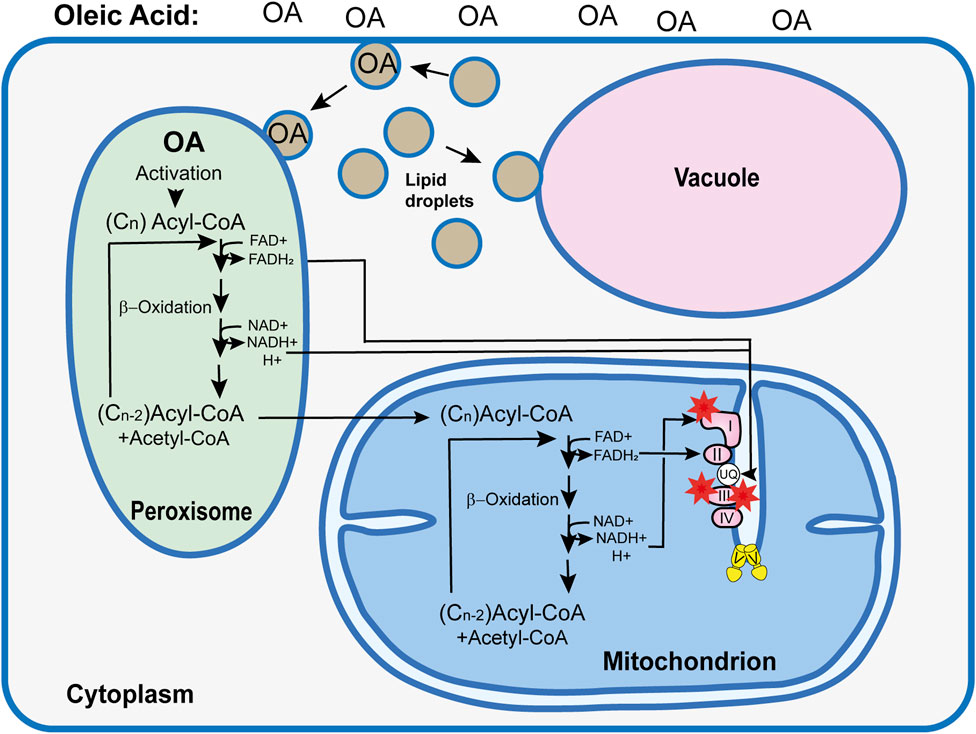
FIGURE 4. Simplified model explaining the consequences of an oleic acid (OA) diet on the Podospora anserina wild type. Use of oleic acid (C18H34O2) as the sole carbon source requires the metabolization of this long-chain fatty acid in peroxisome via ß-oxidation. In subsequent reaction cycles, this fatty acid is broken down to acetyl-Co and acyl-CoA. During this process, FADH2 and NADH+ H+ are formed, which, most likely via shuttle systems (not shown) (Wanders et al., 2015), are transported into the mitochondrial RC, where they contribute to ATP and superoxide (red asterisks) generation. Short acyl-CoA molecules enter the mitochondria, where they are further metabolized. In contrast to the NADH imported into the mitochondria that enters the RC at the ubiquinol (UQ) pool, NADH generated by mitochondrial ß-oxidation transfers electrons to mitochondrial complex I, increasing the risk of superoxide production. In Podospora anserina, an OA diet induces the formation of lipid droplets. These organelles transport OA to the peroxisome. In addition, they are active in maintaining membrane homeostasis via the transport of phospholipids to the vacuole membrane. This function became apparent from the analysis of the PaAtg1-deleted mutant, which was affected in membrane trafficking and in vacuolar function and autophagy (Henkel et al., 2020; Schürmanns et al., 2022).
6 Metabolic changes induced by oleic acid
The induction of lipid droplets by oleic acid occurs not only in the PaAtg24-deleted mutant but also in the wild type and is correlated with a lifespan extension (Schürmanns, 2022; Schürmanns et al., 2022). Apart from the effect of making biomembranes available for vacuole proliferation, an altered bioenergetic metabolism starting with ß-oxidation in peroxisomes is followed by the transfer of peroxisomal FADH2 and NADH, most likely via specific membrane-bound protein complexes (not shown), to mitochondria (Wanders et al., 2015) and feeding electrons into the RC (Figure 4). In contrast to NADH generated inside mitochondria, which transfers electrons to complex I of the RC, electron transfer of NADH from peroxisomes has to be imported in the IMS and, subsequently, using an alternative external NADH dehydrogenase to the ubiquinol pool in the IMM. In this way, complex I as a major superoxide generator (Barja, 2013) is bypassed (Figure 4). In the PaAtg24-deleted mutant, hydrogen peroxide that is formed by superoxide dismutase was shown to be lower than that generated by the standard respiratory chain of the wild type. However, after partial metabolization of oleic acid, acyl-CoA breakdown products with six or fewer C-carbon atoms are further metabolized by mitochondrial ß-oxidation. Electrons from the resulting redox equivalents can then enter the RC at complex I, and superoxide can be generated at this complex (Jezek et al., 2023). However, compared to the utilization of fermentable carbon sources, this type of metabolism is strongly reduced when the strains are grown on oleic acid as the sole carbon source. Under these conditions, hydrogen peroxide secretion as a measure of the cellular ROS load is strongly decreased (Schürmanns et al., 2022).
Overall, metabolic changes, as well as restoration of membrane trafficking and vacuole formation leading to a restoration of autophagy efficiency, contribute to the pronounced lifespan extension of the PaAtg24-deleted mutant, which, grown on a standard growth medium with fermentable carbon sources, is short-lived. Moreover, the observed lifespan of the wild type grown on oleic acid demonstrates a strong beneficial impact of this nutrient on aging.
7 Conclusion
In P. anserina, aging has a strong mitochondrial etiology. In old age, the efficient reorganization of the mtDNA biosynthesis of mitochondria is blocked and functional mitochondria cannot be sufficiently delivered to the growing parts of the vegetation body. This leads to growth stop and PCD. There are various pathways (e.g., ROS scavenging, mitochondrial dynamics, and degradation of damaged compounds) that can delay this process of degeneration, leading to longevity. Recent investigations emphasized the crucial relevance of biomembranes in such processes, which was already indicated in early work, demonstrating the important role of bioenergetic processes at the IMM. Specifically, the role of membrane trafficking, vacuoles, and autophagy underlined the strong impact of biomembranes. Furthermore, the impact of oleic acid on the longevity of P. anserina is striking because olive oil, which is rich in oleic acid, is a prominent component in Mediterranean diets, which is known to be beneficial for human health and successful aging (Trichopoulou and Dilis, 2007). At the mechanistic level, this positive effect results from reduced metabolic stress combined with effective cellular remodeling, which strongly depend on the biomembrane function and dynamics.
Author contributions
HDO: conceptualization, writing–original draft, and writing–review and editing.
Funding
The author declares that financial support was received for the research, authorship, and/or publication of this article. The work of HDO was continuously supported by the Deutsche Forschungsgemeinschaft (DFG, German Research Foundation), Project-ID 25913077-SFB1177 and Os75/17-2.
Conflict of interest
The author declares that the research was conducted in the absence of any commercial or financial relationships that could be construed as a potential conflict of interest.
The author declares being an editorial board member of Frontiers, at the time of submission. This had no impact on the peer review process and the final decision.
Publisher’s note
All claims expressed in this article are solely those of the authors and do not necessarily represent those of their affiliated organizations, or those of the publisher, the editors, and the reviewers. Any product that may be evaluated in this article, or claim that may be made by its manufacturer, is not guaranteed or endorsed by the publisher.
References
Barcena, C., Mayoral, P., and Quiros, P. M. (2018). Mitohormesis, an antiaging paradigm. Int. Rev. Cell. Mol. Biol. 340, 35–77. doi:10.1016/bs.ircmb.2018.05.002
Barja, G. (2013). Updating the mitochondrial free radical theory of aging: an integrated view, key aspects, and confounding concepts. Antioxid. Redox Signal. 19, 1420–1445. doi:10.1089/ars.2012.5148
Barja, G. (2019). Towards a unified mechanistic theory of aging. Exp. Gerontol. 124, 110627. doi:10.1016/j.exger.2019.05.016
Bernardi, P., Gerle, C., Halestrap, A. P., Jonas, E. A., Karch, J., Mnatsakanyan, N., et al. (2023). Identity, structure, and function of the mitochondrial permeability transition pore: controversies, consensus, recent advances, and future directions. Cell. Death Differ. 30, 1869–1885. doi:10.1038/s41418-023-01187-0
Borghouts, C., Scheckhuber, C. Q., Stephan, O., and Osiewacz, H. D. (2002a). Copper homeostasis and aging in the fungal model system Podospora anserina: differential expression of PaCtr3 encoding a copper transporter. Int. J. Biochem. Cell. Biol. 34, 1355–1371. doi:10.1016/s1357-2725(02)00078-x
Borghouts, C., Scheckhuber, C. Q., Werner, A., and Osiewacz, H. D. (2002b). Respiration, copper availability and SOD activity in P. anserina strains with different lifespan. Biogerontol 3, 143–153. doi:10.1023/a:1015696404723
Brust, D., Daum, B., Breunig, C., Hamann, A., Kühlbrandt, W., and Osiewacz, H. D. (2010). Cyclophilin D links programmed cell death and organismal aging in Podospora anserina. Aging Cell 9, 761–775. doi:10.1111/j.1474-9726.2010.00609.x
Cummings, D. J., Belcour, L., and Grandchamp, C. (1979). Mitochondrial DNA from Podospora anserina and the occurrence of multimeric circular DNA in senescent cultures. Mol. Gen. Genet. 171, 239–250. doi:10.1007/BF00267578
Daum, B., Walter, A., Horst, A., Osiewacz, H. D., and Kühlbrandt, W. (2013). Age-dependent dissociation of ATP synthase dimers and loss of inner-membrane cristae in mitochondria. Proc. Natl. Acad. Sci. U. S. A. 110, 15301–15306. doi:10.1073/pnas.1305462110
Davies, K. M., Anselmi, C., Wittig, I., Faraldo-Gomez, J. D., and Kühlbrandt, W. (2012). Structure of the yeast F1Fo-ATP synthase dimer and its role in shaping the mitochondrial cristae. Proc. Natl. Acad. Sci. U. S. A. 109, 13602–13607. doi:10.1073/pnas.1204593109
Esser, K., and Tudzynski, P. (1980). “Senescence in fungi,” in Senescence in plants. Editor K. V. Thimann (Boca Raton: CRC Press), 67–83.
Fridovich, I. (1999). Fundamental aspects of reactive oxygen species, or what's the matter with oxygen? Ann. N. Y. Acad. Sci. 893, 13–18. doi:10.1111/j.1749-6632.1999.tb07814.x
Gredilla, R., Grief, J., and Osiewacz, H. D. (2006). Mitochondrial free radical generation and lifespan control in the fungal aging model Podospora anserina. Exp. Gerontol. 41, 439–447. doi:10.1016/j.exger.2006.01.010
Groebe, K., Krause, F., Kunstmann, B., Unterluggauer, H., Reifschneider, N. H., Scheckhuber, C. Q., et al. (2007). Differential proteomic profiling of mitochondria from Podospora anserina, rat and human reveals distinct patterns of age-related oxidative changes. Exp. Gerontol. 42, 887–898. doi:10.1016/j.exger.2007.07.001
Hamann, A., Brust, D., and Osiewacz, H. D. (2007). Deletion of putative apoptosis factors leads to lifespan extension in the fungal ageing model Podospora anserina. Mol. Microbiol. 65, 948–958. doi:10.1111/j.1365-2958.2007.05839.x
Hamann, A., Brust, D., and Osiewacz, H. D. (2008). Apoptosis pathways in fungal growth, development and ageing. Trends Microbiol. 16, 276–283. doi:10.1016/j.tim.2008.03.003
Hamann, A., and Osiewacz, H. D. (2018). “Podospora anserina: a filamentous fungus with a strong mitochondrial etiology of aging,” in Conn’s handbook of models for human aging. Editors J. L. Ram, and P. M. Conn (London, San Diego, Oxford: Academic Press), 431–444.
Harman, D. (1972). The biologic clock: the mitochondria? J. Am. Geriatr. Soc. 20, 145–147. doi:10.1111/j.1532-5415.1972.tb00787.x
Hekimi, S., Lapointe, J., and Wen, Y. (2011). Taking a "good" look at free radicals in the aging process. Trends Cell. Biol. 21, 569–576. doi:10.1016/j.tcb.2011.06.008
Henkel, V., Schürmanns, L., Brunner, M., Hamann, A., and Osiewacz, H. D. (2020). Role of sorting nexin PaATG24 in autophagy, aging and development of Podospora anserina. Mech. Ageing Dev. 186, 111211. doi:10.1016/j.mad.2020.111211
Jezek, P., Jaburek, M., Holendova, B., Engstova, H., and Dlaskova, A. (2023). Mitochondrial cristae morphology reflecting metabolism, superoxide formation, redox homeostasis, and pathology. Antioxid. Redox Signal. 39, 635–683. doi:10.1089/ars.2022.0173
Kennedy, B. K., Berger, S. L., Brunet, A., Campisi, J., Cuervo, A. M., Epel, E. S., et al. (2014). Geroscience: linking aging to chronic disease. Cell 159, 709–713. doi:10.1016/j.cell.2014.10.039
Klecker, T., and Westermann, B. (2021). Pathways shaping the mitochondrial inner membrane. Open Biol. 11, 210238. doi:10.1098/rsob.210238
Knuppertz, L., Hamann, A., Pampaloni, F., Stelzer, E., and Osiewacz, H. D. (2014). Identification of autophagy as a longevity-assurance mechanism in the aging model Podospora anserina. Autophagy 10, 822–834. doi:10.4161/auto.28148
Kück, U., Osiewacz, H. D., Schmidt, U., Kappelhoff, B., Schulte, E., Stahl, U., et al. (1985). The onset of senescence is affected by DNA rearrangements of a discontinuous mitochondrial gene in Podospora anserina. Curr. Genet. 9, 373–382. doi:10.1007/BF00421608
Kück, U., Stahl, U., and Esser, K. (1981). Plasmid-like DNA is part of mitochondrial DNA in Podospora anserina. Curr. Genet. 3, 151–156. doi:10.1007/BF00365719
López-Otín, C., Blasco, M. A., Partridge, L., Serrano, M., and Kroemer, G. (2013). The hallmarks of aging. Cell 153, 1194–1217. doi:10.1016/j.cell.2013.05.039
Löser, T., Joppe, A., Hamann, A., and Osiewacz, H. D. (2021). Mitochondrial phospholipid homeostasis is regulated by the i-AAA protease PaIAP and affects organismic aging. Cells 10, 2775. doi:10.3390/cells10102775
Ma, M., Burd, C. G., and Chi, R. J. (2017). Distinct complexes of yeast Snx4 family SNX-BARs mediate retrograde trafficking of Snc1 and Atg27. Traffic 18, 134–144. doi:10.1111/tra.12462
Maglioni, S., Schiavi, A., Runci, A., Shaik, A., and Ventura, N. (2014). Mitochondrial stress extends lifespan in C. elegans through neuronal hormesis. Exp. Gerontol. 56, 89–98. doi:10.1016/j.exger.2014.03.026
Marcou, D. (1961). Notion de longévité et nature cytoplasmatique du déterminant de sénescence chez quelques champignons. Ann. Sci. Nat. Bot. Biol. Veg. Ser. 12, 653–764.
Marschall, L. M., Warnsmann, V., Meessen, A. C., Löser, T., and Osiewacz, H. D. (2022). Lifespan extension of Podospora anserina Mic60-subcomplex mutants depends on cardiolipin remodeling. Int. J. Mol. Sci. 23, 4741. doi:10.3390/ijms23094741
Minina, E. A., Staal, J., Alvarez, V. E., Berges, J. A., Berman-Frank, I., Beyaert, R., et al. (2020). Classification and nomenclature of metacaspases and paracaspases: no more confusion with caspases. Mol. Cell 77, 927–929. doi:10.1016/j.molcel.2019.12.020
Oemer, G., Edenhofer, M. L., Wohlfarter, Y., Lackner, K., Leman, G., Koch, J., et al. (2021). Fatty acyl availability modulates cardiolipin composition and alters mitochondrial function in HeLa cells. J. Lipid Res. 62, 100111. doi:10.1016/j.jlr.2021.100111
Oemer, G., Koch, J., Wohlfarter, Y., Alam, M. T., Lackner, K., Sailer, S., et al. (2020). Phospholipid acyl chain diversity controls the tissue-specific assembly of mitochondrial cardiolipins. Cell Rep. 30, 4281–4291. doi:10.1016/j.celrep.2020.02.115
Osiewacz, H. D. (2002). Genes, mitochondria and aging in filamentous fungi. Ageing Res. Rev. 1, 425–442. doi:10.1016/s1568-1637(02)00010-7
Osiewacz, H. D., and Esser, K. (1984). The mitochondrial plasmid of Podospora anserina: a mobile intron of a mitochondrial gene. Curr. Genet. 8, 299–305. doi:10.1007/BF00419728
Osiewacz, H. D., Hermanns, J., Marcou, D., Triffi, M., and Esser, K. (1989). Mitochondrial DNA rearrangements are correlated with a delayed amplification of the mobile intron (plDNA) in a long-lived mutant of Podospora anserina. Mutat. Res. 219, 9–15. doi:10.1016/0921-8734(89)90036-2
Osiewacz, H. D., and Schürmanns, L. (2021). A network of pathways controlling cellular homeostasis affects the onset of senescence in Podospora anserina. J. Fungi 7, 263. doi:10.3390/jof7040263
Rampello, N. G., Stenger, M., Westermann, B., and Osiewacz, H. D. (2018). Impact of F1Fo-ATP-synthase dimer assembly factors on mitochondrial function and organismic aging. Microb. Cell 5, 198–207. doi:10.15698/mic2018.04.625
Ristow, M., and Zarse, K. (2010). How increased oxidative stress promotes longevity and metabolic health: the concept of mitochondrial hormesis (mitohormesis). Exp. Gerontol. 45, 410–418. doi:10.1016/j.exger.2010.03.014
Rizet, G. (1953). Sur l'impossibilite d'obtenir la multiplication vegetative ininterrompue illimite de l'ascomycete Podospora anserina. C. R. Acad. Sci. Paris 237, 838–855.
Scheckhuber, C. Q., Erjavec, N., Tinazli, A., Hamann, A., Nyström, T., and Osiewacz, H. D. (2007). Reducing mitochondrial fission results in increased life span and fitness of two fungal ageing models. Nat. Cell Biol. 9, 99–105. doi:10.1038/ncb1524
Scheckhuber, C. Q., Houthoofd, K., Weil, A. C., Werner, A., De, V. A., Vanfleteren, J. R., et al. (2011). Alternative oxidase dependent respiration leads to an increased mitochondrial content in two long-lived mutants of the ageing model Podospora anserina. PLoS.One 6, e16620. doi:10.1371/journal.pone.0016620
Scheckhuber, C. Q., and Osiewacz, H. D. (2008). Podospora anserina: a model organism to study mechanisms of healthy ageing. Mol. Genet. Genomics 280, 365–374. doi:10.1007/s00438-008-0378-6
Scheckhuber, C. Q., Rödel, E., and Wüstehube, J. (2008). Regulation of mitochondrial dynamics--characterization of fusion and fission genes in the ascomycete Podospora anserina. Biotechnol. J. 3, 781–790. doi:10.1002/biot.200800010
Schiavi, A., Maglioni, S., Palikaras, K., Shaik, A., Strappazzon, F., Brinkmann, V., et al. (2015). Iron-starvation-induced mitophagy mediates lifespan extension upon mitochondrial stress in C. elegans. Curr. Biol. 25, 1810–1822. doi:10.1016/j.cub.2015.05.059
Schulte, E., Kück, U., and Esser, K. (1988). Extrachromosomal mutants from Podospora anserina: permanent vegetative growth in spite of multiple recombination events in the mitochondrial genome. Mol. Gen. Genet. 211, 342–349. doi:10.1007/bf00330614
Schulte, E., Kück, U., and Esser, K. (1989). Multipartite structure of mitochondrial DNA in a fungal longlife mutant. Plasmid 21, 79–84. doi:10.1016/0147-619x(89)90089-9
Schürmanns, L. (2022). Die Rolle von Peroxisomen in Entwicklungs-und Alterungsprozessen des Ascomyceten Podospora anserina. Dissertation. Frankfurt/Main, Germany: Johann Wolfgang Goethe University.
Schürmanns, L., Hamann, A., and Osiewacz, H. D. (2022). Lifespan increase of Podospora anserina by oleic acid is linked to alterations in energy metabolism, membrane trafficking and autophagy. Cells 11, 519. doi:10.3390/cells11030519
Sellem, C. H., Lecellier, G., and Belcour, L. (1993). Transposition of a group II intron. Nature 366, 176–178. doi:10.1038/366176a0
Shaw, J. M., and Nunnari, J. (2002). Mitochondrial dynamics and division in budding yeast. Trends Cell. Biol. 12, 178–184. doi:10.1016/s0962-8924(01)02246-2
Sies, H. (2017). Hydrogen peroxide as a central redox signaling molecule in physiological oxidative stress: oxidative eustress. Redox Biol. 11, 613–619. doi:10.1016/j.redox.2016.12.035
Stahl, U., Lemke, P. A., Tudzynski, P., Kück, U., and Esser, K. (1978). Evidence for plasmid like DNA in a filamentous fungus, the ascomycete Podospora anserina. Mol. Gen. Genet. 162, 341–343. doi:10.1007/BF00268860
Stefanyk, L. E., Coverdale, N., Roy, B. D., Peters, S. J., and LeBlanc, P. J. (2010). Skeletal muscle type comparison of subsarcolemmal mitochondrial membrane phospholipid fatty acid composition in rat. J. Membr. Biol. 234, 207–215. doi:10.1007/s00232-010-9247-4
Strobel, I., and Osiewacz, H. D. (2013). Poly(ADP-ribose) polymerase is a substrate recognized by two metacaspases of Podospora anserina. Eukaryot. Cell 12, 900–912. doi:10.1128/EC.00337-12
Szklarczyk, R., Nooteboom, M., and Osiewacz, H. D. (2014). Control of mitochondrial integrity in ageing and disease. Phil. Trans. R. Soc. B 369, 20130439. doi:10.1098/rstb.2013.0439
Trichopoulou, A., and Dilis, V. (2007). Olive oil and longevity. Mol. Nutr. Food Res. 51, 1275–1278. doi:10.1002/mnfr.200700134
Wanders, R. J., Waterham, H. R., and Ferdinandusse, S. (2015). Metabolic interplay between peroxisomes and other subcellular organelles including mitochondria and the endoplasmic reticulum. Front. Cell. Dev. Biol. 3, 83. doi:10.3389/fcell.2015.00083
Warnsmann, V., Marschall, L. M., Meessen, A. C., Wolters, M., Schürmanns, L., Basoglu, M., et al. (2022). Disruption of the MICOS complex leads to an aberrant cristae structure and an unexpected, pronounced lifespan extension in Podospora anserina. J. Cell. Biochem. 123, 1306–1326. doi:10.1002/jcb.30278
Westermann, B. (2008). Molecular machinery of mitochondrial fusion and fission. J. Biol. Chem. 283, 13501–13505. doi:10.1074/jbc.R800011200
Yun, J., and Finkel, T. (2014). Mitohormesis. Cell. Metab. 19, 757–766. doi:10.1016/j.cmet.2014.01.011
Keywords: aging, Podospora anserina, biomembranes, phospholipids, membrane trafficking, mitochondria, vacuoles, autophagy
Citation: Osiewacz HD (2024) The impact of biomembranes and their dynamics on organismic aging: insights from a fungal aging model. Front. Aging 5:1356697. doi: 10.3389/fragi.2024.1356697
Received: 16 December 2023; Accepted: 09 January 2024;
Published: 24 January 2024.
Edited by:
John Tower, University of Southern California, United StatesReviewed by:
Christian Q. Scheckhuber, Monterrey Institute of Technology and Higher Education (ITESM), MexicoRyo Higuchi-Sanabria, University of Southern California, United States
Copyright © 2024 Osiewacz. This is an open-access article distributed under the terms of the Creative Commons Attribution License (CC BY). The use, distribution or reproduction in other forums is permitted, provided the original author(s) and the copyright owner(s) are credited and that the original publication in this journal is cited, in accordance with accepted academic practice. No use, distribution or reproduction is permitted which does not comply with these terms.
*Correspondence: Heinz D. Osiewacz, osiewacz@bio.uni-frankfurt.de