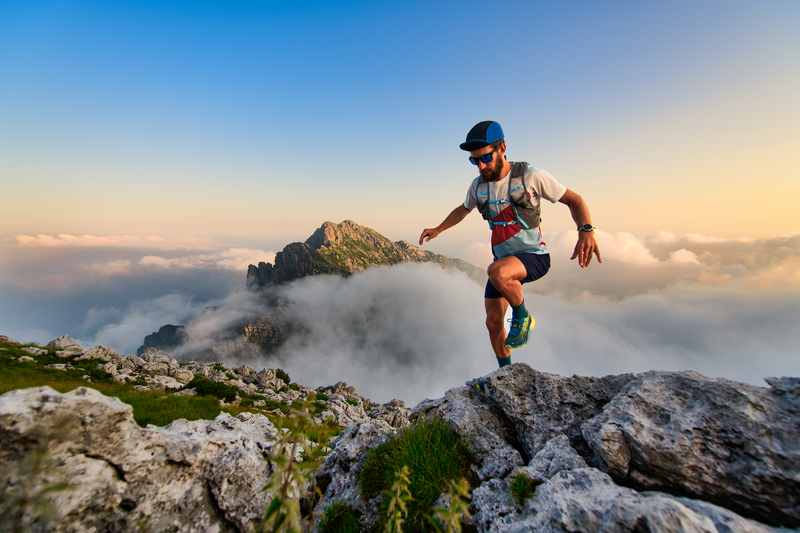
95% of researchers rate our articles as excellent or good
Learn more about the work of our research integrity team to safeguard the quality of each article we publish.
Find out more
PERSPECTIVE article
Front. Aging , 08 March 2023
Sec. Musculoskeletal Aging
Volume 4 - 2023 | https://doi.org/10.3389/fragi.2023.977426
This article is part of the Research Topic Insights in Musculoskeletal Aging 2022 View all 6 articles
Cartilage is a tissue that consist of very few cells embedded in a highly negatively charged extracellular matrix (ECM). This tissue is dealing with several electrical potentials which have been shown to control the production of ECM. Cartilage is present at joints and is constantly prone to degradation. Failing to repair the damage will result in the occurrence of osteoarthritis (OA). This perspective aims to link biophysical insights with biomolecular research in order to provide an alternative view on the possible causes of OA. Firstly, we hypothesize the existence of a threshold potential, which should be reached in order to initiate repair but if not met, unrepaired damage will evolve to OA. Measurements of the magnitude of this threshold electrical potential would be a helpful diagnostic tool. Secondly, since electrical potential alterations can induce chondrocytes to synthesize ECM, a cellular sensor must be present. We here propose an analogy to the hypocalcemia ‘unshielding’ situation to comprehend electrical potential generation and explore possible sensing mechanisms translating the electrical message into cellular responses. A better understanding of the cellular voltage sensors and down-stream signalling mechanisms may lead to the development of novel treatments for cartilage regeneration.
Cartilage is a flexible connective tissue that can be found throughout the animal kingdom in many places of the body where it serves as a shock absorber. Compared to other connective tissues, cartilage has a very slow turnover and extremely slow cell growth and is not easy to repair. Another remarkable feature of cartilage is the stunningly low cell content of <2.5% (Hunziker et al., 2002; Quinn et al., 2005; Quinn et al., 2013) of which both chondrocytes and chondroblasts (the immature cells which can differentiate into chondrocytes), are involved in the maintenance and repair of the extracellular matrix (ECM) of the cartilage. This ECM is composed of proteoglycan and elastic fibers such as different types of collagen and is divided in different zones around the cells (Figure 1; Heinegård, 2009). The proteoglycan is a chain of glycosaminoglycans (GAG) which are covalently attached to various core proteins such as the family of large extracellular chondroitin sulfate proteoglycans like aggrecan or the small leucine-rich core protein family such as decorin, biglycan, fibromodulin, lumican and epiphycan or the basement membrane proteoglycan, perlecan (Knudson and Knudson, 2001). This unit is than linked to hyaluronan via a link protein. Hyaluronic acid and other sulphated GAG (e.g., chondroitin sulphate) contains negative charges that attract high charge density countercharges such as Ca2+ and Mg2+. These negative charges are the so called fixed negative charges (FNC) as opposed to the mobile positive charges of ions dissolved in the extracellular fluid phase (Athanasiou et al., 2017). Under physiological loading conditions, chondrocytes maintain the balance between degradation and synthesis of matrix components. When loading becomes excessive or in case of injury, synthesis will lag in comparison with degradation, causing joint degradation and eventually osteoarthritis (Chen et al., 2013).
FIGURE 1. The molecular organization of cartilage around a chondrocyte. The cartilage matrix is arranged into different zones defined by their distance from the cell. The space immediately around the chondrocyte, that is the zone where molecules interact with cell surface receptors, is called the pericellular matrix (PM); for example, hyaluronan binds the receptor CD44. Next to the PM lies the territorial matrix and at largest distance from the cell is the interterritorial matrix whereby each zone is defined by the types of collagens and the collagen-binding proteins. Abbreviations: CILP, cartilage intermediate layer protein 1; COMP, cartilage oligomeric matrix protein; CS, chondroitin sulfate; KS, keratan sulfate; PRELP, proline-arginine-rich end leucine-rich repeat protein; hyaluronan (HA); CHAD, Chondroadherin; HS-PG, heparan sulphate proteoglycan; MAT, matrilin; NC4, non-collagenous domain 4. Reproduced from Heinegård. (2009) with permission from Wiley & Sons.
Cartilage is an elastic material with a dynamic elastic modulus of ∼2.6 MPa as measured by indentation-type atomic force microscopy on porcine samples, using a micrometer sized tip. By contrast, nanometer sized tips used to explore elasticity at the fine structure level report 100 fold lower values (Stolz et al., 2004). The origin of these mechanical parameters was described in what is known as the “triphasic theory,” through changes in properties in response to deformation and occurrence of stress fields in a biphasic fluid-solid state (the interstitial fluid and the ECM) with a tertiary phase of mobile ions (Na+ and Cl− in most models) under mechanical load. This theory indicated that the Donnan osmotic pressure, the chemical-expansion stress (originating from the repulsive forces of the compressed negative GAG molecules) and the solid matrix elastic stress are the main determinants of overall compression stiffness of cartilage (Lai et al., 1991; Lai et al., 2000; Wan et al., 2004). In another approach, Bushmann and Grodzinsky demonstrated that the pressure build-up in cartilage is mainly due to chemical-expansion stress and is best described by a molecular level Poisson Boltzmann model instead of a macroscopic Donnan model (Buschmann and Grodzinsky, 1995). To complicate things, a positive electrostatic field has been reported to be created outside the cartilage matrix by convection-induced positive charge accumulation at the synovial side of the cartilage-synovial fluid interface (Quenneville and Buschmann, 2005); data on such convection-induced polarization are however limited. By contrast, in vitro experiments on cartilage exposed to mechanical force clearly show the appearance of a well-documented “streaming potential” that can be measured by an extracellular electrode placed in the center of a cartilage disc preparation, relative to a bath electrode positioned at the outer edge (Kim et al., 1995). Ex vivo experiments on isolated porcine cartilage demonstrated the appearance of streaming potentials of up to −5.5 mV (Schmidt-Rohlfing et al., 2002), which correlated with the amount of compression pressure applied, with higher pressures resulting in larger potentials. Similar potentials (−5.3–−6.9 mV) were measured in human lumbar spine discs and were found to be anisotropic in nature and to reflect the degree of cartilage degeneration with larger streaming potential magnitudes being found in less degenerated discs (Gu et al., 1999); these results were confirmed in bovine experiments (Fujisaki et al., 2011). Moreover, the magnitude of the streaming potentials decreased after successive loading cycles measured in human volunteers using electroarthrography (Zhu et al., 2016). Interestingly, these experiments demonstrate that the streaming potential is present in different, articular and fibrous, cartilage types and that its magnitude decreases with degeneration of the cartilage.
Evidence has become available demonstrating that electrical fields may influence chondrocyte function and cartilage properties. In a compression study on bovine cartilage explants it was shown that the synthesis of aggrecan was increased in the regions with the highest interstitial fluid flow (Buschmann et al., 1999). No electrical parameters were recorded in this study, but follow-up work by others later demonstrated that capacitatively coupled electrical signals were able to upregulate chondrocyte matrix genes and products such as Type II collagen and aggrecan in bovine articular chondrocytes (Wang et al., 2004), which was further corroborated in a 2006 study, also on bovine cartilage, where an increase in mRNA for collagen and proteoglycans was observed in response to externally applied electrical fields (Brighton et al., 2006). The team of Alan Grodzinsky had already in 1995 demonstrated an increased proteoglycan production under influence of streaming potentials in bovine explants. The application of 10 mA/cm2 with a 100–1,000 Hz frequency resulted in significantly increased protein synthesis as measured by an increased incorporation of 35S–methionine in articular cartilage explants (MacGinitie et al., 1994). Interestingly, electrical currents had a positive effect on the proliferation of chondrocytes but not on mature hypertonic chondrocytes which are located deeper in the cartilage matrix where fluid currents and shear potentials are less pronounced compared to the cartilage surface (Nogami et al., 1982). Two studies by the team of Maretto Esquisatto demonstrated that a clear and remarkable recovery of artificially induced (by a punch) damaged rat cartilage was observed after 35 days of electrical stimulation, resulting in significantly increased proteoglycan content as compared to non-treated samples (de Campos Ciccone et al., 2013; Zuzzi et al., 2013). Other studies demonstrated enhanced expression levels of type II collagen, aggrecan, proteoglycan and Sox9, driving oscillations in intracellular Ca2+ and ATP concentration (Fitzgerald et al., 2004), and significantly increasing TGF-β1 and BMP2 (Brighton et al., 2006; Kwon et al., 2016), which may be ultimately involved in the repair mechanisms of the cartilage.
The obvious question that follows is how chondrocytes exactly sense the mechanical loading induced electrical signals. Currently, there is indeed a gap between the macroscopic events leading to the appearance of electrical potentials in cartilage and the knowledge of membrane protein channels/sensors on the microscopic level that might be activated by these potentials. Chondrocytes express a substantial array of ion channels, including voltage-gated Na+, K+ and Ca2+ channels (Mobasheri et al., 2012; Mobasheri et al., 2019). However, the resting membrane potential (Vm) of these cells is rather depolarized, ranging from −40 to −10 mV as measured in different organisms (Sugimoto et al., 1996; Clark et al., 2010; Funabashi et al., 2010; Lewis et al., 2011). At such depolarized potentials, voltage-gated Na+ and Ca2+ channels may become inactivated and therefore reside in a closed state (e.g., chondrocytic Cav3.2 channels start to inactivate from −80 mV on; Monteil et al., 2015). Interestingly, voltage sensing is not a prerogative of ion channels as G-protein coupled receptors (GPCRs) may also express voltage sensors (Bezanilla, 2008). E.g., muscarinic receptors display voltage-dependent responses, with depolarizing stimuli enhancing M1-type receptor responses while reducing those of M2 receptors (Ben-Chaim et al., 2006); in chondrocytes, this may lead to increased GAG synthesis (Lind et al., 1998). The ubiquitous P2Y12 purinergic receptor, present in chondrocytes and involved in their migration (Szustak and Gendaszewska-Darmach, 2020) is another example where voltage sensing through GPCRs may impact function (Zhang et al., 2020).
Next to voltage sensing membrane proteins, changes in Vm may have a more direct impact by affecting the driving force for transmembrane transport of electrically charged substances. In fact, any membrane transporter or channel that carries net charge over the plasma membrane, called “electrogenic transport,” will be affected by changes in Vm or by local charge accumulation. This includes transporters like the Na+/K+-ATPase, the plasma membrane Ca2+-ATPase and the Na2+/Ca2+ exchanger, as well as NMDA receptor channels, TRP channels and Ca2+ channels present in chondrocytes (Mobasheri et al., 2019). As such, currents through, e.g., TRPV5 channels may be altered by streaming potentials and thereby affect chondrocyte volume regulation (Lewis et al., 2011). Also TRPV4 channels are involved in chondrocyte Ca2+ signaling and mechanosensing (Agarwal et al., 2021), together with piezo-1 and piezo-2 mechanosensitive channels (Du et al., 2020). The Ca2+-sensing receptor is another well characterized player in bone but also cartilage development and remodeling (reviewed in Goltzman and Hendy, 2015). This G-protein coupled receptor (GPCR) is activated by extracellular Ca2+ elevation and translates to intracellular IP3/Ca2+ signaling. Activation of the Ca2+-sensing receptor is involved in the pathophysiology of osteoarthritis (Burton et al., 2005); however, in the context of ‘Ca2+-unshielding’ discussed below, this receptor would become less activated and thus less likely to be involved in the sensing of streaming potentials.
Of note, it is important to distinguish local effects of mechano-loading induced streaming potentials from Vm changes. Therefore we like to present an educated guess on how macroscopic induced changes in cartilage might affect membrane proteins by comparing the situation with a very well known paradigm of altered charge accumulation in the condition of hypocalcemia. Interestingly, the fixed ECM-linked negative charge accumulation associated with cartilage loading will influence membrane voltage sensors in very much the same way as occurs in hypocalcemia (Figure 2). In hypocalcemia, Ca2+ occupation of fixed negative charges in the glycocalyx decreases, effectively unshielding the outside negativity, which is reminiscent of the situation in cartilage during mechanical compression where mobile positive charges, in particular Ca2+ are expulsed and the negative charges remain unshielded outside the chondrocyte plasma membrane. Due to the membrane capacitance, the de novo appearing outside negativity attracts positive charges inside. Membrane voltage sensors may sense the positive charge accumulation inside and react in very much the same way as if an intracellular depolarizing Vm stimulus would be applied. In muscle, this causes hyperexcitability because voltage sensitive Na+ channels come closer to their activation threshold in hypocalcemia. Likewise, in mechano-loaded chondrocytes, local electrostatic effects that lead to streaming potentials may affect voltage sensors of ion channels and GPCRs, and alter the driving force and activity of electrogenic transports. Moreover, exogenously applied electrical fields may superimpose on these local charge effects thereby reaching a threshold for activating cartilage recovery programs. Of the above mentioned chondrocyte channel family, voltage-gated Ca2+ channels stand out in their ability to induce ECM gene transcription and increased production of ECM components (Xu et al., 2009). These authors observed that electrical stimulation resulted in a 3-4 fold up-regulation of aggrecan and type II collagen mRNA in chondrocytes, which was abolished by voltage-gated Ca2+ channel blockers. Weber and Waldman, (2014) furthermore found a positive correlation between Ca2+ signaling and matrix synthesis under mechanical loading conditions. Chicken chondrocytes express several types of voltage-gated calcium channels, including L-type (Cav1.2 and Cav1.3), R-type (Cav2.3) and T-type (Cav3.1, Cav3.2 and Cav3.3). Most interestingly, L-type Ca2+ channel inhibition with nifedipine was reported to completely abrogate chondrogenesis (Fodor et al., 2013). These data suggest that L-type Ca2+ channels are functional and not completely inactivated in chondrocytes as suggested by their depolarized Vm referred to higher. Thus, Ca2+ channels may play a role in acute or delayed (gene expression-dependent) chondrogenetic responses, pressing for further studies. Here, we propose that the streaming potential and consequent intracellular signaling, including Ca2+ signaling, may tilt the balance towards chondrogenesis and repair.
FIGURE 2. Cartilage compression may generate intracellular accumulation of positive charges by mechanisms that resemble the “unshielding effect” in hypocalcemia. From streaming potentials to functional alterations in chondrocytes and cartilage degeneration. (A). In hypocalcemia, the decreased extracellular Ca2+ concentration exposes fixed negative charges in the glycocalyx surrounding the cells, effectively unshielding the outside negativity. Due to membrane capacitance (Cm), the uncovered negativity attracts positive charges inside the cell. Voltage sensors of plasma membrane ion channels feel this local positive charge accumulation inside and react in very much the same way as if a depolarizing Vm stimulus would be applied. In muscle and nerves, this causes hyperexcitability because the set-point of voltage sensitive Na+ channels is shifted closer to the activation threshold upon hypocalcemia. (B). In cartilage, compression triggers interstitial fluid flow containing anions, e.g., Cl−, bicarbonate, and cations, mainly Na+ and Ca2+. At the center of pressure loading (vertical dotted arrow), this creates a centrifugal movement of Na+ and Ca2+ from the center to peripheral zones (bended arrow) thereby leaving behind fixed negative charges that become unshielded and lead to a local negative streaming potential. Inside the chondrocytes, this creates positive charge accumulation that, as in hypocalcemia, brings voltage sensors closer to their activation threshold. This may affect voltage sensitive ion channels but also GPCRs and electrogenic transports, leading to functional consequences as a result of altered signaling and activation of gene programs. In OA, streaming potentials decrease in magnitude and we hypothesize that the threshold for activating repair signaling is not reached, leading to progressive cartilage degeneration.
It is known that the magnitude of the streaming potentials decreases with the degree of cartilage degradation (Garon et al., 2002; Légaré et al., 2002). Under normal healthy conditions, the streaming potential is apparently able to stimulate the biogenesis of cartilage and counter the degradation by wear and tear in a continuous cycle. One of the current recommendations for people at risk of developing OA is exercise (Roos and Dahlberg, 2005; Racunica et al., 2007; Ravalli et al., 2019; Kong et al., 2022). Exercise may exert this effect by enhancing the magnitude of streaming potentials thereby stimulating chondrocytes to produce new ECM. This raises the question whether there exists a threshold streaming potential which, if not reached, is unable to stimulate cartilage repair. The damage inflicted to the cartilage can result in a decreased number of active chondrocytes, which could explain a diminished repair potential of the damaged matrix. However, microscopic studies performed by Häuselmann and his team (Hunziker et al., 2002; Quinn et al., 2005; Quinn et al., 2013) revealed an extraordinary low cell volume density of only between 1% and 2.5% in articular cartilage, which indicates that most of the damage will be inflicted on the matrix structure thereby decreasing the magnitude of the streaming potential. Interestingly, cell volume measurements in healthy and damaged cartilage did not find a decreased number of cells but rather a decreased volume regulation which resulted in an increased cell volume percentage (Bush and Hall, 2003). Therefore we hypothesize that once the cartilage has reached a critical degree of damage and loss of negatively charged ECM, the potential may dip below a putative threshold and thereby mark the point of no return where the pathology further develops into a full blown OA condition.
In the wake of several papers reporting on streaming potentials, researchers have developed devices to measure this potential and to correlate this to a progressive degradation of the cartilage. The teams of Grodzinsky and recently Buschmann have developed a quantitative electrical impedance analysis method for cartilage degradation by measuring the electrolyte content in several species (canine, human, bovine; US patent 6735468B2; Frank et al., 2011; Changoor et al., 2020). The sensitivity and reliability of such approaches is still limited with substantial variability of the measured potentials. Novel technologies such as nano-device carbon electrodes may however yield more stable less-invasive approaches, allowing continuous monitoring for patients at risk of developing OA (e.g., obese patients). Thus at the engineering front there is definitely a need for standardization and more precise measurements. In terms of theoretical insights there are still many questions open related to the generation of streaming potentials, the contribution of convection-induced polarization effects at the cartilage-synovial fluid interface, and the impact of these complex effects on cellular signaling. Will it be possible to produce a better model that can cope with this complexity and that is backed up by experimental observations, e.g., by measurements on chondrocytes embedded in artificial polymers with an adjustable and known density of fixed negative charges? Can the hypothetical threshold potential proposed here be documented in vivo? Can cartilage biogenesis be stimulated by applying external electrical fields, thereby mimicking the effects of streaming potentials ? Is it possible to overcome the hypothetical threshold potential by an external electrical field ? Can we apply external electrical stimulation combined with rehabilitation to counter the progressive cartilage degradation of patients that have subthreshold potentials ? Alternatively, can we trick the chondrocytes by pharmacological intervention to stimulate the production of ECM by targeting the membrane channel or transport proteins ? Most importantly, to trick the chondrocytes one has to identify the sensor. Here we are favouring a calcium channel as sensor given the effect of Ca2+ on ECM production but this does not exclude that other channel types may be sensitive to the streaming potential. Further research in this exciting field may certainly stimulate new findings and avenues for therapeutic interventions in OA patients.
The original contributions presented in the study are included in the article/Supplementary Material, further inquiries can be directed to the corresponding author.
All authors contributed to the conception of the ideas and hypotheses and provided the final approval of the paper. PV and LL drafted the article and EA and PC revised it critically for important intellectual content.
The authors declare that the research was conducted in the absence of any commercial or financial relationships that could be construed as a potential conflict of interest.
All claims expressed in this article are solely those of the authors and do not necessarily represent those of their affiliated organizations, or those of the publisher, the editors and the reviewers. Any product that may be evaluated in this article, or claim that may be made by its manufacturer, is not guaranteed or endorsed by the publisher.
Agarwal, P., Lee, H. P., Smeriglio, P., Grandi, F., Goodman, S., Chaudhuri, O., et al. (2021). A dysfunctional TRPV4-GSK3β pathway prevents osteoarthritic chondrocytes from sensing changes in extracellular matrix viscoelasticity. Nat. Biomed. Eng. 5, 1472–1484.
Athanasiou, K. A., Darling, E. M., Hu, J. C., DuRaine, G. D., and Reddi, A. H. (2017). Articular cartilage. second edition. Boca Raton: Taylor and Francis CRC Press.
Ben-Chaim, Y., Chanda, B., Dascal, N., Bezanilla, F., Parnas, I., and Parnas, H. (2006). Movement of 'gating charge' is coupled to ligand binding in a G-protein-coupled receptor. Nature 444, 106–109. doi:10.1038/nature05259
Bezanilla, F. (2008). How membrane proteins sense voltage. Nat. Rev. Mol. Cell. Biol. 9, 323–332. doi:10.1038/nrm2376
Brighton, C. T., Wang, W., and Clark, C. C. (2006). Up-regulation of matrix in bovine articular cartilage explants by electric fields. Biochem. Biophys. Res. Commun. 342, 556–561. doi:10.1016/j.bbrc.2006.01.171
Burton, D. W., Foster, M., Johnson, K. A., Hiramoto, M., Deftos, L. J., and Terkeltaub, R. (2005). Chondrocyte calcium-sensing receptor expression is up-regulated in early Guinea pig knee osteoarthritis and modulates PTHrP, MMP-13, and TIMP-3 expression. Osteoarthr. Cartil. 13, 395–404. doi:10.1016/j.joca.2005.01.002
Buschmann, M. D., and Grodzinsky, A. J. (1995). A molecular model of proteoglycan-associated electrostatic forces in cartilage mechanics. J. Biomech. Eng. 117, 179–192. doi:10.1115/1.2796000
Buschmann, M. D., Kim, Y. J., Wong, M., Frank, E., Hunziker, E. B., and Grodzinsky, A. J. (1999). Stimulation of aggrecan synthesis in cartilage explants by cyclic loading is localized to regions of high interstitial fluid flow. Arch. Biochem. Biophys. 366, 1–7. doi:10.1006/abbi.1999.1197
Bush, P. G., and Hall, A. C. (2003). The volume and morphology of chondrocytes within non-degenerate and degenerate human articular cartilage. Osteoarthr. Cartil. 11, 242–251. doi:10.1016/s1063-4584(02)00369-2
Changoor, A., Garon, M., Quenneville, E., Bull, S. B., Gordon, K., Savard, P., et al. (2020) Non-invasive electroarthrography measures load-induced cartilage streaming potentials via electrodes placed on skin surrounding an articular joint. Cartilage. 13, 375–385 .doi:10.1177/1947603520928583
Chen, C., Tambe, D. T., Deng, L., and Yang, L. (2013). Biomechanical properties and mechanobiology of the articular chondrocyte. Am. J. Physiol. Cell. Physiol. 305, C1202–C1208. doi:10.1152/ajpcell.00242.2013
Clark, R. B., Hatano, N., Kondo, C., Belke, D. D., Brown, B. S., Kumar, S., et al. (2010). Voltage-gated K+ currents in mouse articular chondrocytes regulate membrane potential. Channels (Austin) 4, 179–191. doi:10.4161/chan.4.3.11629
de Campos Ciccone, C., Zuzzi, D. C., Neves, L. M., Mendonça, J. S., Joazeiro, P. P., and Esquisatto, M. A. (2013). Effects of microcurrent stimulation on hyaline cartilage repair in immature male rats (Rattus norvegicus). BMC Complem. Altern. Med. 13, 17–25. doi:10.1186/1472-6882-13-17
Du, G., Li, L., Zhang, X., Liu, J., Hao, J., Zhu, J., et al. (2020). Roles of TRPV4 and piezo channels in stretch-evoked Ca2+ response in chondrocytes. Exp. Biol. Med. (Maywood) 245, 180–189. doi:10.1177/1535370219892601
Fitzgerald, J. B., Jin, M., Dean, D., Wood, D. J., Zheng, M. H., and Grodzinsky, A. J. (2004). Mechanical compression of cartilage explants induces multiple time-dependent gene expression patterns and involves intracellular calcium and cyclic AMP. J. Biol. Chem. 279, 19502–19511. doi:10.1074/jbc.M400437200
Fodor, J., Matta, C., Oláh, T., Juhász, T., Takács, R., Tóth, A., et al. (2013). Store-operated calcium entry and calcium influx via voltage-operated calcium channels regulate intracellular calcium oscillations in chondrogenic cells. Cell Calcium 54, 1–16. doi:10.1016/j.ceca.2013.03.003
Frank, E., Evans, R., Lee, C., Treppo, S., Spector, M., and Grodzinsky, A. (2011). Quantitative electrical impedance analysis of cartilage degradation. Biorheology 41, 195–202.
Fujisaki, K., Tadano, S., and Asano, N. (2011). Relationship between streaming potential and compressive stress in bovine intervertebral tissue. J. Biomech. 44, 2477–2481. doi:10.1016/j.jbiomech.2011.06.018
Funabashi, K., Fujii, M., Yamamura, H., Ohya, S., and Imaizumi, Y. (2010). Contribution of chloride channel conductance to the regulation of resting membrane potential in chondrocytes. J. Pharmacol. Sci. 113, 94–99. doi:10.1254/jphs.10026sc
Garon, M., Légaré, A., Guardo, R., Savard, P., Buschmann, M. D., and LegAre, A. (2002). Streaming potentials maps are spatially resolved indicators of amplitude, frequency and ionic strength dependant responses of articular cartilage to load. J. Biomech. 35, 207–216. doi:10.1016/s0021-9290(01)00197-x
Goltzman, D., and Hendy, G. N. (2015). The calcium-sensing receptor in bone--mechanistic and therapeutic insights. Nat. Rev. Endocrinol. 11, 298–307. doi:10.1038/nrendo.2015.30
Gu, W. Y., Mao, X. G., Rawlins, B. A., Iatridis, J. C., Foster, R. J., Sun, D. N., et al. (1999). Streaming potential of human lumbar anulus fibrosus is anisotropic and affected by disc degeneration. J. Biomech. 32, 1177–1182. doi:10.1016/s0021-9290(99)00118-9
Heinegård, D. (2009). Fell-Muir Lecture: Proteoglycans and more-from molecules to biology. Int. J. Exp. Pathol. 90, 575–586. doi:10.1111/j.1365-2613.2009.00695.x
Hunziker, E. B., Quinn, T. M., and Häuselmann, H. J. (2002). Quantitative structural organization of normal adult human articular cartilage. Osteoarthr. Cartil. 10, 564–572. doi:10.1053/joca.2002.0814
Kim, Y. J., Bonassar, L. J., and Grodzinsky, A. J. (1995). The role of cartilage streaming potential, fluid flow and pressure in the stimulation of chondrocyte biosynthesis during dynamic compression. J. Biomech. 28, 1055–1066. doi:10.1016/0021-9290(94)00159-2
Knudson, C. B., and Knudson, W. (2001). Cartilage proteoglycans. Semin. Cell Dev. Biol. 12, 69–78. doi:10.1006/scdb.2000.0243
Kong, H., Wang, X. Q., and Zhang, X. A. (2022). Exercise for osteoarthritis: A literature review of pathology and mechanism. Front. Aging Neurosci. 14, 854026. doi:10.3389/fnagi.2022.854026
Kwon, H. J., Lee, G. S., and Chun, H. (2016). Electrical stimulation drives chondrogenesis of mesenchymal stem cells in the absence of exogenous growth factors. Sci. Rep. 6, 39302. doi:10.1038/srep39302
Lai, W. M., Hou, J. S., and Mow, V. C. (1991). A triphasic theory for the swelling and deformation behaviors of articular cartilage. J. Biomech. Eng. 113, 245–258. doi:10.1115/1.2894880
Lai, W. M., Mow, V. C., Sun, D. D., and Ateshian, G. A. (2000). On the electric potentials inside a charged soft hydrated biological tissue: Streaming potential versus diffusion potential. J. Biomech. Eng. 122, 336–346. doi:10.1115/1.1286316
Légaré, A., Garon, M., Guardo, R., Savard, P., Poole, A. R., and Buschmann, M. D. (2002). Detection and analysis of cartilage degeneration by spatially resolved streaming potentials. J. Orthop. Res. 20, 819–826. doi:10.1016/S0736-0266(02)00002-5
Lewis, R., Asplin, K. E., Bruce, G., Dart, C., Mobasheri, A., and Barrett-Jolley, R. (2011). The role of the membrane potential in chondrocyte volume regulation. J. Cell. Physiol. 226, 2979–2986. doi:10.1002/jcp.22646
Lind, G. J., Chew, S. J., Marzani, D., and Wallman, J. (1998). Muscarinic acetylcholine receptor antagonists inhibit chick scleral chondrocytes. Invest. Ophthalmol. Vis. Sci. 39, 2217–2231.
MacGinitie, L. A., Gluzband, Y. A., and Grodzinsky, A. J. (1994). Electric field stimulation can increase protein synthesis in articular cartilage explants. J. Orthop. Res. 12, 151–160. doi:10.1002/jor.1100120202
Mobasheri, A., Lewis, R., Ferreira-Mendes, A., Rufino, A., Dart, C., and Barrett-Jolley, R. (2012). Potassium channels in articular chondrocytes. Channels (Austin) 6, 416–425. doi:10.4161/chan.22340
Mobasheri, A., Matta, C., Uzielienè, I., Budd, E., Martín-Vasallo, P., and Bernotiene, E. (2019). The chondrocyte channelome: A narrative review. Jt. Bone Spine 86, 29–35. doi:10.1016/j.jbspin.2018.01.012
Monteil, A., Chausson, P., Boutourlinsky, K., Mezghrani, A., Huc-Brandt, S., Blesneac, I., et al. (2015). Inhibition of Cav3.2 T-type calcium channels by its intracellular I-II loop. J. Biol. Chem. 290, 16168–16176. doi:10.1074/jbc.M114.634261
Nogami, H., Aoki, H., Okagawa, T., and Mimatsu, K. (1982). Effects of electric current on chondrogenesis in vitro. Clin. Orthop. Relat. Res. 163, 243–247. doi:10.1097/00003086-198203000-00037
Quenneville, E., and Buschmann, M. D. (2005). A transport model of electrolyte convection through a charged membrane predicts generation of net charge at membrane/electrolyte interfaces. J. Memb. Sci. 265, 60–73. doi:10.1016/j.memsci.2005.04.032
Quinn, T. M., Häuselmann, H. J., Shintani, N., and Hunziker, E. B. (2013). Cell and matrix morphology in articular cartilage from adult human knee and ankle joints suggests depth-associated adaptations to biomechanical and anatomical roles. Osteoarthr. Cartil. 21, 1904–1912. doi:10.1016/j.joca.2013.09.011
Quinn, T. M., Hunziker, E. B., and Häuselmann, H. J. (2005). Variation of cell and matrix morphologies in articular cartilage among locations in the adult human knee. Osteoarthr. Cartil. 13, 672–678. doi:10.1016/j.joca.2005.04.011
Racunica, T. L., Teichtahl, A. J., Wang, Y., Wluka, A. E., English, D. R., Giles, G. G., et al. (2007). Effect of physical activity on articular knee joint structures in community-based adults. Arthritis Rheum. 57, 1261–1268. doi:10.1002/art.22990
Ravalli, S., Castrogiovanni, P., and Musumeci, G. (2019). Exercise as medicine to be prescribed in osteoarthritis. World J. Orthop. 10, 262–267. doi:10.5312/wjo.v10.i7.262
Roos, E. M., and Dahlberg, L. (2005). Positive effects of moderate exercise on glycosaminoglycan content in knee cartilage: A four-month, randomized, controlled trial in patients at risk of osteoarthritis. Arthritis Rheum. 52, 3507–3514. doi:10.1002/art.21415
Schmidt-Rohlfing, B., Schneider, U., Goost, H., and Silny, J. (2002). Mechanically induced electrical potentials of articular cartilage. J. Biomech. 35, 475–482. doi:10.1016/s0021-9290(01)00232-9
Stolz, M., Raiteri, R., Daniels, A. U., VanLandingham, M. R., Baschong, W., and Aebi, U. (2004). Dynamic elastic modulus of porcine articular cartilage determined at two different levels of tissue organization by indentation-type atomic force microscopy. Biophys. J. 86, 3269–3283. doi:10.1016/S0006-3495(04)74375-1
Sugimoto, T., Yoshino, M., Nagao, M., Ishii, S., and Yabu, H. (1996). Voltage-gated ionic channels in cultured rabbit articular chondrocytes. Comp. Biochem. Physiol. C Pharmacol. Toxicol. Endocrinol. 115, 223–232. doi:10.1016/s0742-8413(96)00091-6
Szustak, M., and Gendaszewska-Darmach, E. (2020). Extracellular nucleotides selectively induce migration of chondrocytes and expression of type II collagen. Int. J. Mol. Sci. 21, 5227. doi:10.3390/ijms21155227
Wan, L. Q., Miller, C., Guo, X. E., and Mow, V. C. (2004). Fixed electrical charges and mobile ions affect the measurable mechano-electrochemical properties of charged-hydrated biological tissues: The articular cartilage paradigm. Mech. Chem. Biosyst. 1, 81–99.
Wang, W., Wang, Z., Zhang, G., Clark, C. C., and Brighton, C. T. (2004). Up-regulation of chondrocyte matrix genes and products by electric fields. Clin. Orthop. Relat. Res. 427, 163–173. doi:10.1097/01.blo.0000143837.53434.5c
Weber, J. F., and Waldman, S. D. (2014). Calcium signaling as a novel method to optimize the biosynthetic response of chondrocytes to dynamic mechanical loading. Biomech. Model. Mechanobiol. 13, 1387–1397. doi:10.1007/s10237-014-0580-x
Xu, J., Wang, W., Clark, C. C., and Brighton, C. T. (2009). Signal transduction in electrically stimulated articular chondrocytes involves translocation of extracellular calcium through voltage-gated channels. Osteoarthr. Cart. 17, 397–405. doi:10.1016/j.joca.2008.07.001
Zhang, Q., Liu, B., Li, Y., Yin, L., Younus, M., Jiang, X., et al. (2020). Regulating quantal size of neurotransmitter release through a GPCR voltage sensor. Proc. Natl. Acad. Sci. U. S. A. 117, 26985–26995. doi:10.1073/pnas.2005274117
Zhu, L., Garon, M., Quenneville, E., Buschmann, M. D., and Savard, P. (2016). Decrease of the electrical potentials measured on the surface of the knee and produced by cartilage compression during successive loading cycles. J. Biomech. 49, 3587–3591. 55. doi:10.1016/j.jbiomech.2016.09.008
Keywords: osteoarthiritis, membrane proteins, potentials, voltage sensors, cartilage
Citation: Van Gelder P, Audenaert E, Calders P and Leybaert L (2023) A new look at osteoarthritis: Threshold potentials and an analogy to hypocalcemia. Front. Aging 4:977426. doi: 10.3389/fragi.2023.977426
Received: 26 July 2022; Accepted: 24 February 2023;
Published: 08 March 2023.
Edited by:
Kieran Reid, Brigham and Women’s Hospital and Harvard Medical School, United StatesReviewed by:
Csaba Matta, University of Debrecen, HungaryCopyright © 2023 Van Gelder, Audenaert, Calders and Leybaert. This is an open-access article distributed under the terms of the Creative Commons Attribution License (CC BY). The use, distribution or reproduction in other forums is permitted, provided the original author(s) and the copyright owner(s) are credited and that the original publication in this journal is cited, in accordance with accepted academic practice. No use, distribution or reproduction is permitted which does not comply with these terms.
*Correspondence: L. Leybaert, bHVjLmxleWJhZXJ0QHVnZW50LmJl
Disclaimer: All claims expressed in this article are solely those of the authors and do not necessarily represent those of their affiliated organizations, or those of the publisher, the editors and the reviewers. Any product that may be evaluated in this article or claim that may be made by its manufacturer is not guaranteed or endorsed by the publisher.
Research integrity at Frontiers
Learn more about the work of our research integrity team to safeguard the quality of each article we publish.