- 1Faculty of Dentistry, University of Toronto, Toronto, ON, Canada
- 2Eastman Dental Institute, University College London, London, United Kingdom
- 3L’Oréal Research and Innovation, Aulnay-sous-Bois, France
- 4Faculty of Medicine, School of Dentistry, Pontificia Universidad Catolica de Chile, Santiago, Chile
- 5Schools of Engineering, Medicine, and Biological Sciences, Institute for Biological and Medical Engineering, Pontificia Universidad Católica de, Santiago, Chile
- 6Physics Department, Lancaster University, Lancaster, United Kingdom
The skin is the largest organ in the body and is essential for protecting us from environmental stressors such as UV radiation, pollution, and pathogens. As we age, our skin undergoes complex changes that can affect its function, appearance, and health. These changes result from intrinsic (chronological) and extrinsic (environmental) factors that can cause damage to the skin’s cells and extracellular matrix. As higher-resolution microscopical techniques, such as Atomic Force Microscopy (AFM), are being deployed to support histology, it is possible to explore the biophysical properties of the dermal scaffold’s constituents, such as the collagen network. In this study, we demonstrate the use of our AFM-based quantitative nanohistology, performed directly on unfixed cryosections of 30 donors (female, Caucasian), to differentiate between dermal collagen from different age groups and anatomical sites. The initial 420 (10 × 10 μm2) Atomic Force Microscopy images were segmented into 42,000 (1 × 1 μm2) images before being classified according to four pre-defined empirical collagen structural biomarkers to quantify the structural heterogeneity of the dermal collagen. These markers include interfibrillar gap formation, undefined collagen structure, and registered or unregistered dense collagen fibrillar network with evident D-banding. The structural analysis was also complemented by extensive nanoindentation (∼1,000 curves) performed on individual fibrils from each section, yielding 30,000 indentation curves for this study. Principal Component Analysis was used to reduce the complexity of high-dimensional datasets. The % prevalence of the empirical collagen structural biomarkers between the papillary and reticular dermis for each section proves determinant in differentiating between the donors as a function of their age or the anatomical site (cheek or breast). A case of abnormal biological aging validated our markers and nanohistology approach. This case also highlighted the difference between chronological and biological aging regarding dermal collagen phenotyping. However, quantifying the impact of chronic and pathological conditions on the structure and function of collagen at the sub-micron level remains challenging and lengthy. By employing tools such as the Atomic Force Microscope as presented here, it is possible to start evaluating the complexity of the dermal matrix at the nanoscale and start identifying relevant collagen morphology which could be used toward histopathology standards.
Introduction
The skin is a complex and multi-layered connective tissue that defines the external appearance of all individuals. Specific pathologies or the unavoidable aging process directly impact this appearance (Khavkin and Ellis, 2011). Genetic influences (Venkatesh et al., 2019) and internal factors such as hormones (Tobin, 2017) or metabolic substances (Khavkin and Ellis, 2011) govern the intrinsic aging of the skin. It illustrates the naturally occurring skin modifications with age, leading to wrinkles and skin dryness (Swift et al., 2021). The wrinkle formation in human skin has been associated with marked decreases in skin elasticity (Fenske and Lober, 1986). Extrinsic skin aging arrives earlier and is due, for example, to exposure to sunlight (Fisher et al., 2002), pollution (Salsberg et al., 2019), or lifestyle choices such as a lack of balanced nutrition (Bonté et al., 2019). More generally, in aging skin, cell replacement is continuously declining (Franco et al., 2022), the barrier function and mechanical protection are compromised (Wong et al., 2016), wound healing and immune responses are delayed (Chambers and Vukmanovic-Stejic, 2020), thermoregulation is impaired, and sweat and sebum productions are decreased (Terao and Katayama, 2016).
The aging of the dermis is a complex process with several changes occurring to both collagen and non-collagenous components of the dermal Extracellular Matrix (ECM). Both collagen and elastin undergo enzymatic and non-enzymatic crosslinks in the ECM as a function of aging (Monnier et al., 2005; Saito and Marumo, 2010). Lysyl oxidase (LOX) is the primary enzyme that crosslinks collagen during the final step of its biosynthesis to stabilize the supramolecular assembly of collagen molecules into fibrils (Bailey, 2001). However, the proportion of LOX-derived crosslinks reduces with age (Szauter et al., 2005), while the proportion of non-enzymatic (glycation) crosslinks increases with age. Glycation is the reaction of carbonyl groups of reducing sugars with free amino groups of lipids and proteins to form a Schiff base, which then undergoes a time-dependent rearrangement to form a reasonably stable Amadori product. These structures are still reactive and convert to stable substances called Advanced Glycation End-products (AGEs). The low turnover of collagen (as found in the skin) causes AGEs to accumulate within the collagen fibrils in our tissues and organs during normal aging or some pathological conditions such as diabetes (Snedeker and Gautieri, 2014). In diabetic conditions, glycation is expected to proceed faster due to an increase in available free sugars that are available to react with collagen residues. While these crosslinks’ biochemical and mechanical impact on collagen has been extensively studied in tissue development, repair, and diseases (Tanzer, 1973; Singh et al., 2001; Szauter et al., 2005; Cox et al., 2013; Stammers et al., 2020), few studies have explored their association with dermal collagen fibril organization (Fang et al., 2012; Strange et al., 2017; Penuela et al., 2018). An early study by Achterberg et al. (2014) investigated how the nanoscale mechanical properties of the extracellular matrix regulate dermal fibroblast function by systematically exploring the mechanical and structural dermis properties (human) as a function of age (26–55). Alongside presenting the first ultra-topography images of native skin by Atomic Force Microscopy (AFM) (Binnig et al., 1986), they found that the dermis elasticity ranged from 0.1 to 10 kPa in hydrated sections. These results were further explored by Ahmed et al. who proposed the first phenotypic markers for collagen aging in the reticular dermis, also measured by AFM. In addition, Ahmed explored the relationship between advanced glycation products and the elasticity of individual collagen fibrils within the reticular dermis of young and older individuals. Thus, despite being one of the most imaged proteins by AFM, collagen remains largely uncharacterized at the fibrils scale within the dermis, especially as a function of aging. Here, we present the first large cohort study (30 donors) in which we explore the use of AFM-based quantitative nanohistology to quantify the biophysical properties of dermal collagen at the nanoscale and to demonstrate how the variations in these properties can be used to reveal the skin’s biological age.
Materials and methods
Human skin histological sections
In this study, cryo-preserved historical skin samples (30 Caucasian female donors, 18–75 years, cosmetic surgery procedures) collected under informed consent were analyzed as part of this explorative study. All the samples were anonymized, and the donors’ age, biological sex, and ethnicity were made available for this study. The sample cohort was split into three groups according to both the donors’ age and anatomical site: Breast Young Skin (BYS, N = 11, age range: 18–29 year-old, median: 22.0 ± 4.2 years), Breast Old Skin (BOS, N = 9, age range: 49–78 year-old, median: 69.0 ± 13.2 years), and Cheek Old Skin (COS, N = 10, age range: 56–80 year-old, median: 65.0 ± 7.7 years). Samples of skin obtained from these donors were split in two for processing. For histology, the skin samples were fixed in neutral formalin and then embedded in paraffin before being sectioned (5 um). The sections were stained using Hematoxylin and Eosin Stain (HES), Orcein, and Sirius Red (SR). For quantitative nanohistology (AFM), the skin samples were not fixed but cryo-embedded in optimum cutting temperature media before being cryo-sectioned (8–10 µm thickness). All sections were physisorbed directly on individual glass slides.
Histological imaging
A Leica (Wetzlar, Germany) light microscope (LM) was used for histology imaging of the fixed and stained sections. This microscope was equipped with two crossed-light polarizers (90°) to allow for polarization (darkfield) LM and with an 8-megapixel digital camera (EOS Rebel 100, Canon). Complete histological sections were digitized using the auto-stitch function in Image-Pro Plus software (Meyer Instr. Inc., Houston, United States).
Quantitative nanohistological imaging
Topological images (10 × 10 μm2) of the tissue were acquired on the unfixed sections by Atomic Force Microscopes (AFM Nanowizard I and III, Bruker-JPK, Berlin–Germany) operated in contact mode (MSNL cantilevers (Nom. tip radius: 2 nm, triangular geometry, Bruker, Santa Barbara) operated in ambient conditions at a scanning rate of 1.0 Hz or above. To avoid bias in selecting the area to be imaged, we used a random walk approach to land the probe in each dermal layer by using the AFM sample holders’ x-y translation screws to move the sample (within each dermal layer). Images were then acquired and optimized where the probe came into contact with the sample regardless of the topology seen in the image acquired. A total of 14 images were acquired for each donor (papillary dermis 7; reticular dermis: 7 on a minimum of 2 sections per donor), leading to a dataset of 420 (10 × 10 μm2) images. Following their acquisition, each AFM image was plane-fitted before being segmented into 100 x (1 × 1 μm2) images using ImageJ, creating a final topology dataset of 42,000 (1 × 1 μm2) images to be analyzed.
Quantitative nanomechanical analysis
The mechanical properties of collagen fibrils of the histological sections were acquired by a Nanowizard I AFM (Bruker-JPK, Berlin–Germany) operated in the force-distance mode in ambient conditions. For these measurements, RFESPA cantilevers (Nom Tip Radius: 8nm, rectangular geometry, Bruker, Santa Barbara) with a spring constant k = 3 N/m were employed. The selection of the sample area to be indented was also performed using the same random-walk approach as previously mentioned. First, a low-resolution image (128 × 128 px over 10 × 10 μm2) was performed to ensure that collagen fibrils with defined D-banding periodicity could be observed. Then, indentation sites were manually selected directly on distinct collagen fibrils’ D-banding (overlap region). All indentations were carried out at 1Hz, with a maximum indentation load not exceeding 350 nN (yielding an average indentation depth of d = 25 ± 5 nm). For each histological section, a minimum of 500 individual collagen fibril measurements were carried out in the papillary and reticular dermis, resulting in a mechanical dataset of 30,000 indentations for the 30 samples. The diameter of each indented fibrils was not measured. All force-distance curves were processed by the JPK Data Processing Software v.5.1.8 using the Sneddon/Hertz model (Harding and Sneddon, 1945). The resultant distribution of Elastic (Young’s) Moduli was plotted as histograms with a fixed bin size (B = 500 MPa) to calculate the median Elastic (Young’s) Moduli for the respective dermal layers.
Data management and statistical analyses
All data were processed using Origin Pro (Origin Lab Corporation, Northampton, United States). In this study, each histological block was treated as an individual variable, and data obtained from serial histological sections from the same block were pooled together. Four calibrated analysts were used to quantify the prevalence of the four structural biomarkers in each 42,000 1 × 1 μm2 image. Analysts’ calibration was performed by agreeing on the assignment of marker 1-2-3-4 on 100 AFM 1 × 1 μm2 images. For the remainder of the analysis, all analysts were blinded and did not know the sample groups donors’ assignment until the study was analyzed statistically. The Young’s modulus of fibrils was compared using a two-sided hypothesis Mann-Whitney U test with a significance level α = 0.001. In addition, 2D Principal component analyses (Jolliffe and Cadima, 2016) (PCA) were performed on the dataset using Origin Pro (Origin Lab Corporation, Northampton, United States). The relative position of the variables on the PCA score plot, with their coordinates equal to correlations with the first two principal components, was used to assess the level of discrimination between the variables. Finally, a Partial least Squares Discriminant Analysis (PLS-DA) was performed using SIMCA® 16.0 multivariate Data Analysis Software (Sartorius Stedim Data Analytics AB, Sweden) with the binary age class as the response variable.
Results and discussion
Histology of an aging dermal section
Histological analysis is routinely used to assess structural changes in tissues, including skin, to prognose or diagnose pathologies such as aging. Figure 1 presents the histology of representative sections from our three groups. The young group (Breast Young Skin–BYS) represented by the photo-protected breast skin specimen presents distinct epidermis (Ep), papillary (PD), and reticular (RD) dermal layers (Figure 1A). The dermo-epidermal junction is non-uniform and presents well-defined rete ridges (arrow). Structurally, the collagen in both dermal layers is very dense, and there is little evidence of elastin (Figures 1B-i, iv, vii). In the intrinsically aged skin (Breast Old Skin: BOS) specimen, one observes a thinning of the epidermis and papillary dermis (Figures 1B-ii). The dermo-epidermal junction presents a flattening aspect resulting from the disappearance of the rete ridges. The dermis has an atrophic aspect with a loss of cells and extracellular matrix. As a result, the dermal collagen becomes sparser (Figure 1B-v). Finally, the BOS histology shows evidence of loss of oxytalan fibers but not of solar elastosis/elastin deposition (Figure 1B-viii).
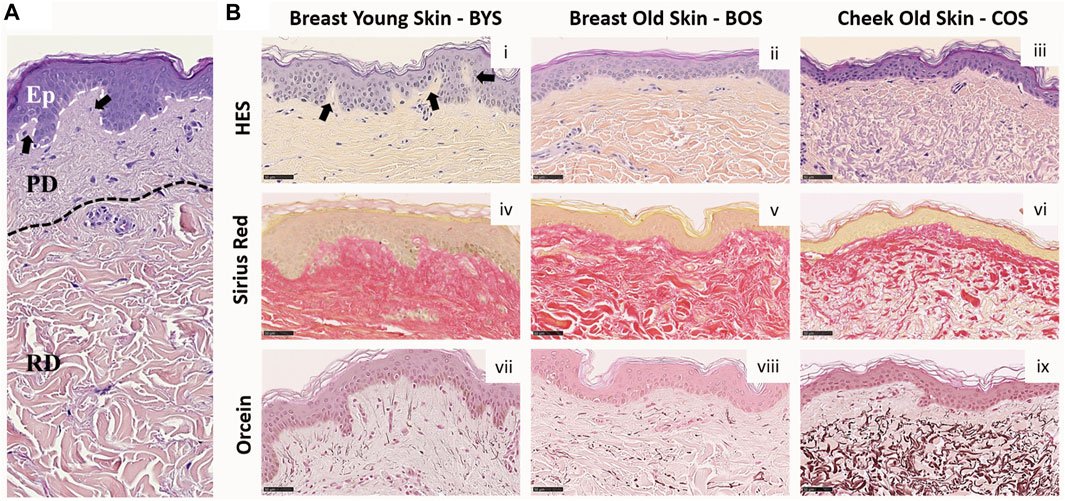
FIGURE 1. Histology of Normal Human Skin: (A) Normal human skin from a young donor (22 yo). The dotted lines separate the epidermis (Ep), papillary (PD), and reticular (RD) dermis. (B) Histological colorations of human skin from breast young (i, iv, and vii) and old (ii, v, and viii) skin (respectively 22 and 74 yo) and cheek old (iii, vi, and ix) skin (75 yo). Different types of colorations were performed: Hematoxylin Eosin Saffron–HES (i–iii), Sirius red (iv–vi), and Orcein (vii–ix). Scale Bar = 50 µm. Arrows highlight the rete ridges.
In contrast, the primary histological fingerprint for extrinsically aged skin (Cheek Old Skin: COS) is the abundance of abnormally structured elastin accumulated as elastotic material. This accumulation can be found in the reticular dermis, as shown in Figure 1B-ix. In addition, the extrinsically aged skin specimen presents a flattening of the dermis and a loss of rete ridges found for the intrinsically aged skin (Figure 1B-iii). The ratio of elastin to collagen has also increased (Figures 1B-vi, ix), suggesting that the collagen is being replaced by elastin, which explains the altered functional and biomechanical properties of this skin (Uitto, 2008). The histological presentation of these groups: young photo-protected skin and intrinsically and extrinsically aged skin, are well known and are routinely used to define the pathological age of skin (Khavkin and Ellis, 2011).
Defining nanoscale structural biomarkers for (type I) collagen
Type I collagen fibril topology is one of the most recognized protein structures due to its highly conserved annular-banding periodicity along the long axis of the fibrils, namely, the D-banding periodicity (Petruska and Hodge, 1964; Chernoff and Chernoff, 1992; Stylianou, 2022). In our quantitative nanohistology approach, we have used AFM to image collagen structure on the skin groups’ histological sections. Figure 2 presents our approach to correlating AFM images site with a polarized image of SR-stained skin sections. We observe the presence of long fibrils with a defined D-banding periodicity in all the images recorded. Fibrils can appear as thick bundles, homogeneous sheets, or interwoven scaffolds. Our original skin study defined several potential structural biomarkers for aging in collagen (Ahmed et al., 2017). Four were present across the three skin groups from a list of 7 candidates’ structural (or topological) collagen biomarkers, as presented in Figure 2B. Marker 1 is defined as the presence of interfibrillar gaps, suggesting a loosening of the dense collagen sheet structure, leading to the loss in fibril registration with one another (Figure 2B-i). Marker 2 characterizes areas where the fibrillar collagen structure is not readily observable and could be associated with cell processes or other extracellular matrix components (Figure 2B-ii). Marker 3 characterizes a well-defined collagen matrix, with the fibrillar D-banding apparent (Figure 2B-iii). However, the collagen fibrils in those areas are disorganized and lack registration between them. In other studies, we found collagen fibrils morphology prevalent in the fibrotic area (Strange et al., 2017). Finally, Marker 4 characterizes a well-defined collagen matrix, with the fibrillar D-banding apparent and the fibrils aligned, forming dense collagen sheets (Figure 2B-iv). The prevalence of each of these structural (or topological) collagen biomarkers in the papillary and reticular dermis by segmenting each 10 × 10 μm2 AFM image into a 100 sub-image 1 × 1 μm2 as presented in Figure 3A. Each sub-image was evaluated independently (1 chosen marker per 1 × 1 μm2 image). In addition to these structural biomarkers, we added the median value of the collagen fibrils Young’s modulus for both the papillary and reticular dermis, as presented in Figure 3B. We have already demonstrated the variation of Young’s modulus of collagen fibrils (dry) as a function of the aging process (Wenger et al., 2007; Ahmed et al., 2017).
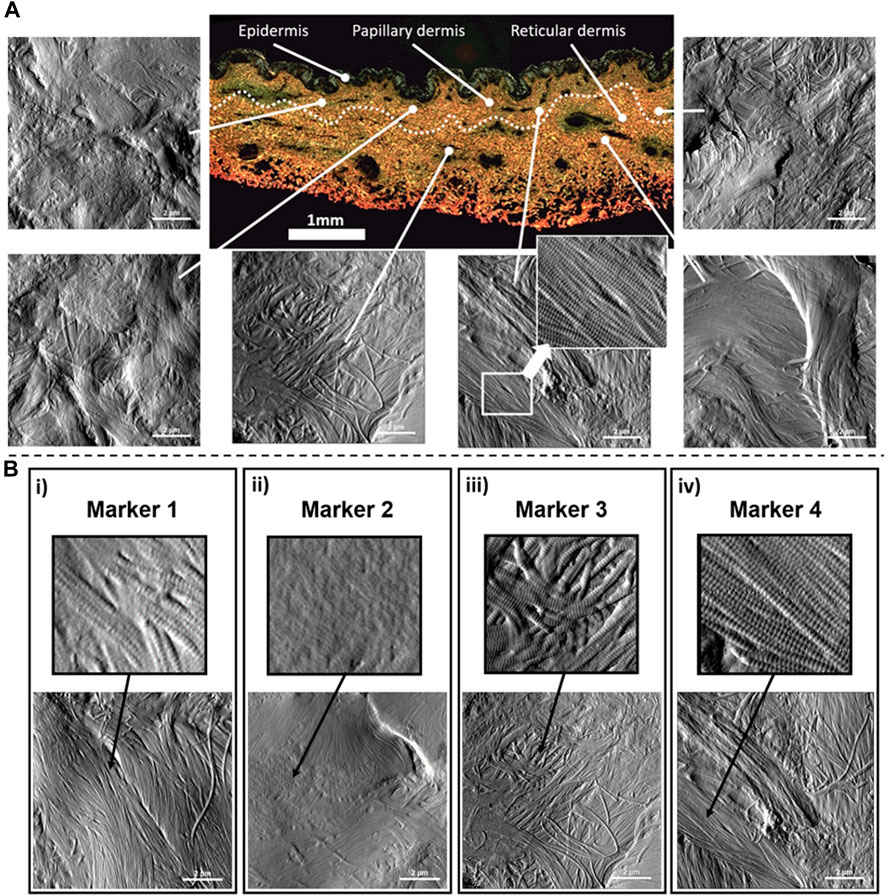
FIGURE 2. Quantitative Nanohistology of dermal collagen performed on histological sections: (A) Representative localized AFM topological images (10 × 10 μm2) obtained from both the papillary and reticular dermal layers obtained directly on a polarised Sirius Red Stain histological section. The boundary between papillary and reticular dermal layers, denoted as a dashed line, was empirically set 500 μm below the epidermal junction. (B) Structural nanoscale biomarkers for (type I) collagen and their presentation on AFM topological images: i) Marker 1 is defined as the presence of interfibrillar gaps (or holes); ii) Marker 2 characterizes areas where the fibrillar collagen structure is not readily observable and could be associated with cell processes or other extracellular matrix components; iii) Marker 3 characterizes a well-defined collagen matrix, with the fibrillar D-banding apparent on the collagen fibrils, but presenting a disorganized and lack registration between the collagen fibrils; iv) Marker 4 characterizes a well-defined collagen matrix, with the fibrillar D-banding apparent on the fibrils and forming a dense sheet of aligned collagen fibrils.
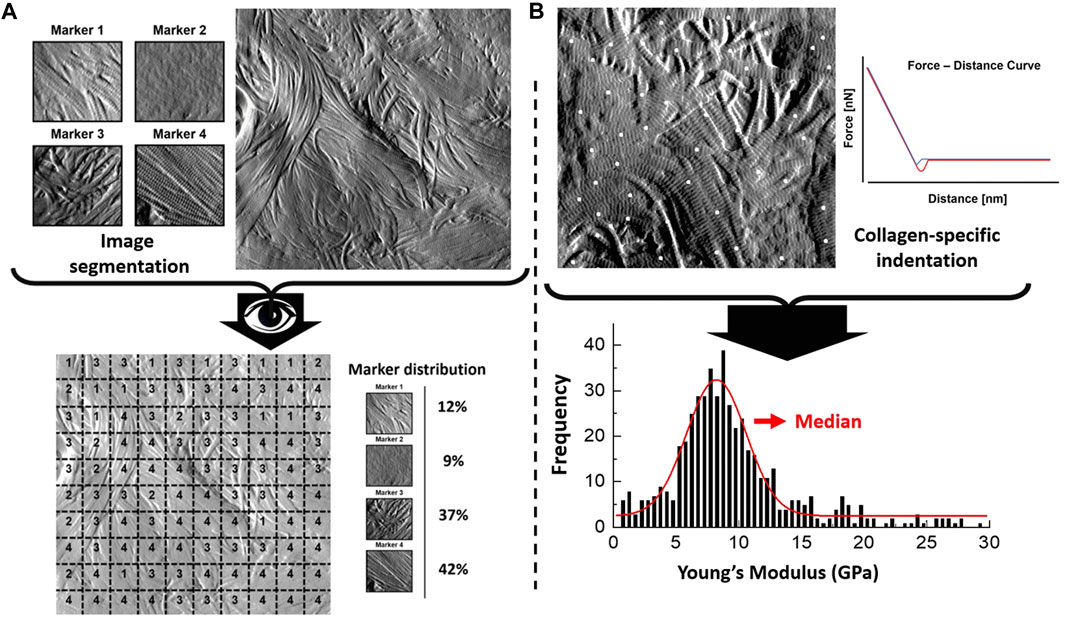
FIGURE 3. Quantitative Nanohistology image analysis and mechanical data analysis: (A) AFM-based image visual segmentation approach presenting the four collagen morphological markers and a representative AFM topological image pre and post-visual segmentation, and the resultant marker distribution. The segmented AFM topological image is analyzed by overlaying a 10 × 10 macro pixel grid and assigning a unique marker in each of the grid areas; (B) specific collagen fibrils AFM-based nanoindentation presenting localized indentation points performed on fibrils (white dots) to generate the frequency distribution of the Young’s Moduli which is in turn fitted to extract the median Young’s Moduli (compressive) of the fibrils indented in the AFM image.
Deciphering the structure of collagen in dermal layers
Dermal collagen phenotype varies among individuals based on biological sex, ethnic origins, and lifestyles (Fenske and Lober, 1986), (Farage et al., 2008). Therefore, to analyze collagen fibril phenotype, one cannot assume that the properties of the dermal collagen for all the individual donors within a given group are similar; thus, markers cannot be represented through a common median value per dermal layer and donor group.
We explored whether the relative structural variations in the collagen matrices between the papillary and reticular dermis could be used to differentiate between the three groups. To do so, we referenced the variations in individual markers in the reticular dermis to those found for the papillary dermis and normalized these variations using Eq. 1 in Figure 4A. The results are plotted in Figure 4A, presenting the %variation of each marker becoming more prevalent towards either the papillary or reticular dermis. Both markers 1 and 2 show an increased prevalence in the papillary dermis of the BYS group. While marker 2 does not readily inform us of the collagen phenotype, marker 1 suggests that the collagen matrix in the papillary dermis of young photo-protected skin exhibits structural loosening due to the increased presence of interfibrillar gaps (or holes) when compared to the reticular dermis. This finding would also suggest that the collagen matrix aging would occur in the papillary dermis before progressing to the reticular dermis. This outcome supports the recent finding by Lynch et al., who established that the mechanical property of the papillary dermis decreases before that of the reticular dermis with age (Lynch et al., 2022). Their study suggested that with aging, the earliest microstructural and mechanical changes occur in the topmost layers of the dermis/skin and then propagate deeper, providing an opportunity for topical preventive treatments acting at the level of the papillary dermis. An earlier study focusing solely on phenotyping the reticular dermis collagen suggested marker 3 is the hallmark of collagen aging. In our present study, marker 3 does not appear more prevalent in either dermal layer, regardless of age. This result does not contradict our previous study but implies that at the nanoscale, one cannot differentiate the two dermal layers solely based on marker 3. Marker 4 shows an increased prevalence in the reticular dermis of the BOS group, suggesting that intrinsic aging promotes the formation of localized, well-aligned collagen fibril bundles. Using our nanohistology approach, we can assert that the reticular collagen is more aligned than the papillary collagen in the sparser region of dermal collagen. This increase in fibril alignment can be directly associated with interfibrillar crosslinks, such as those mediated by the formation of advanced glycation end-products (Perrone et al., 2020). Finally, no clear trends exist in the % variation of any of the markers associated with the COS group. The lack of a specific structural collagen marker to describe the matrix associated with extrinsically aged skin is unsurprising due to the reduced collagen content favoring elastin fiber formation.
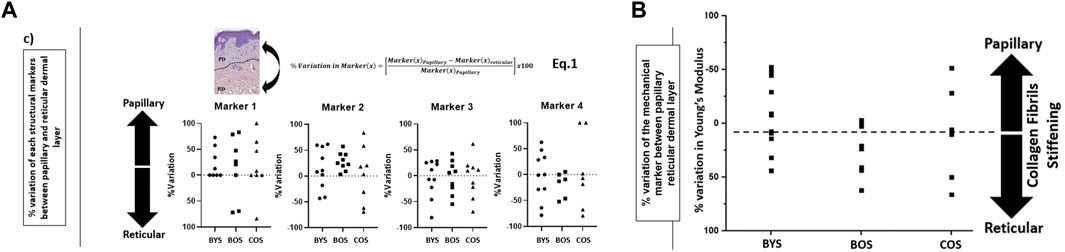
FIGURE 4. Evaluation of the prevalence of structural biomarkers for (type I) collagen as a function of dermal layers and skin groups: (A) %variation in the prevalence of each nanoscale structural biomarkers for (type I) collagen between papillary and reticular dermis (defined in Eq. 1) as a function of the skin groups; (B) variation in the prevalence of the mechanical biomarkers for (type I) collagen between papillary and reticular dermis (as defined in Eq. 1) for each skin group. The direction of the %variation indicates a greater marker prevalence in the papillary or reticular dermis.
Collagen elasticity as a viable marker for age and anatomical differentiation
In a similar approach for the morphological assessment, we calculated the %variation of Young’s moduli between the papillary and reticular dermis, as presented in Figure 4B. This result shows intrinsic aging promotes reticular collagen stiffening over papillary collagen within the intrinsically aged group (BYS compared to BOS). On the other hand, the extrinsically aged group (COS) did not show any trend toward stiffening of the papillary or reticular dermis. This heterogeneity in the mechanical response corroborates our finding in the nanohistology analysis, for which we could not use our pre-defined histomorphological markers. Numerous studies have explored the variations in the collagen mechanical properties at various scales as a function of aging and focused on calculating the nominal values for the elastic modulus of dermal collagen fibrils or scaffolds. (Crichton et al., 2011; Achterberg et al., 2014; Ahmed et al., 2017; Strange et al., 2017; Penuela et al., 2018). Unfortunately, the range of published elastic modulus values for dermal collagen fibrils varies across several orders ranging from a few 100 kPa to a few GPa (Ahmed et al., 2017). This wide range in the elastic modulus values is directly linked to the histological section conditioning, indentation size, inconsistent indentation load, limited sample size, and collagen hydration level. Unfortunately, comparing study outcomes regarding collagen elasticity measurement is challenging due to the wide variations in the techniques used.
Discrimination between chronological and biological aging
To explore from a multivariate perspective the correlation between the structural (marker 1-2-3-4) and mechanical (elasticity) markers regarding the chronological age of the donors, both unsupervised (regardless of the initially assigned group) PCA and supervised (with the age group as response variable) PLS-DA analyses have been carried out. Figure 5A presents the PCA score plot associated with the %variation of the markers across all donors. The size of the vectors associated with the % variation in markers 3, 4, and elasticity confirm that these three markers are the more potent contributor to the principal components, thus, more prominent differentiators of the entire dataset. On the other hand, the size of the vector associated with marker 1 confirms marker 1 has a limited impact on the dataset. Therefore, we supplemented our PCA analysis with a PLS-DA using the donors’ age binary class as the response variable rather than the markers (Figure 5B). The Biplot chart allows individuals and descriptors (% variation of all markers) to be simultaneously represented and allows us to interpret the individuals in terms of descriptors.
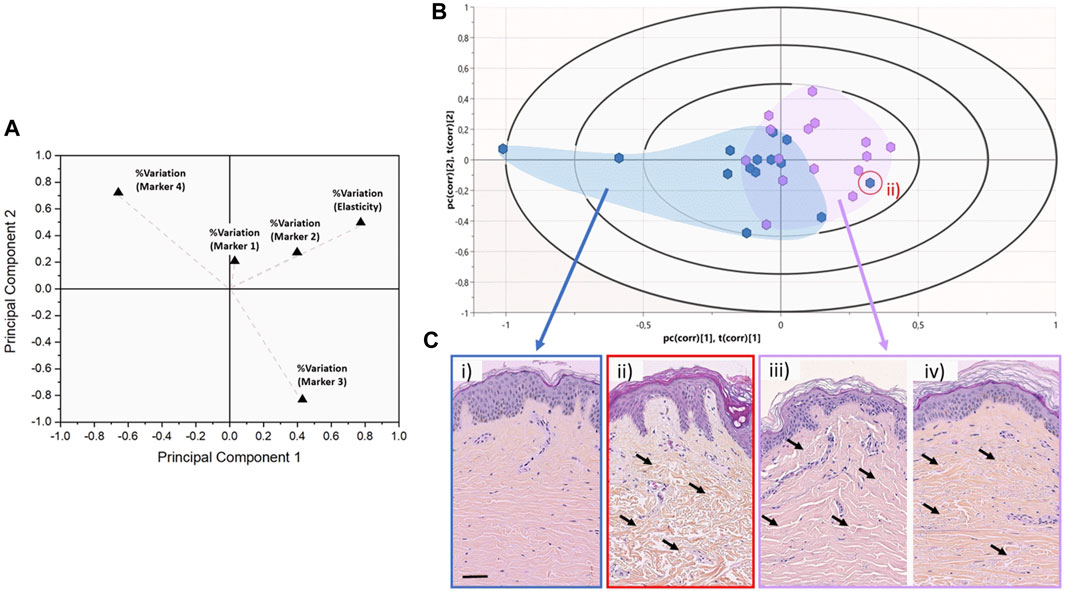
FIGURE 5. Discrimination between chronological and biological aging using multivariate analyses. (A) 2-D PCA score plot associated with the %variation of the markers across the entire dataset for all donors. Variables are presented as vectors extending away from the origin to assess their contribution towards the two principal components. (B) Graphical biplot of the PLS-DA performed on the entire dataset using the donors’ age binary class as the response variable. The blue cluster regroups donors belonging to the young group (<30 years old—median 22.0 ± 4.2 years old), whereas the purple cluster regroups donors belonging to the older group (>50 years old - median 66.0 ± 10.4 years old). The red circled data point highlights an outlier belonging to the young group. (C) representative HES Histology of Normal Human Skin from either the young or the older group, as presented in Figure 1. The red circle histological image refers to the outlier (young) case in (B), which presents an old skin phenotype. The black arrows highlight the variations in the dermal layer morphology which can be described as atrophic and sparse in the case of ii), when compared to iii) and iv).
In this biplot, we can observe that the distribution of donors tends to form 2 clusters: all the young donors (<30y.o) are clustered on the left-hand side of the biplot (with two exceptions), whereas the older donors (>50 y.o) tend to cluster on the right-hand side of the biplot. Significant overlap exists between the two sub-populations around the central axis of the PLS-DA. This overlap can be associated with the well-known disparity between the chronological age of the donors and their biological age (Rittie and Fisher, 2015). Although a 20-year gap exists between our younger and old groups, one cannot assume that the chronological age of the donor is the same as their biological age. This is proven by a young donor case on the aged group’s outer edge (red circle). Figure 5C-ii presents the histological images of this young donor (22y.o). Despite the dermo-epidermal junction being non-uniform and presenting well-defined rete ridges, the underlying dermal collagen presents significant signs of aging-induced damage, as found in older donors (Figure 5C-iii, iv). The dermal collagen is heavily atrophic and sparse for this young donor, likely associated with significant Sun damage. The quantitative nanohistological assessment performed in this study could classify this donor’s collagen phenotype amongst the old group. This 22-year-old donor has the dermal fingerprint of a chronologically old donor, demonstrating the disparity between chronological and biological age again.
Significance
Exploring variations in the phenotypic properties of human largest and most accessible organs has been the subject of much research. However, quantifying the impact of chronic and pathological conditions on the structure and function of collagen at the sub-micron level remains challenging. This is due to a) a lack of current techniques to assess these properties systematically at the nanoscale and b) a lack of histopathology standards (at that scale) in the literature. By employing tools such as the Atomic Force Microscope (Kiio and Park, 2020), we can start cataloging the complexity of the dermal matrix at the nanoscale. All the studies to date present a snapshot of the dermal matrix morphology at the nanoscale, but there is a significant lack of extensive studies. This lack of cohort study poses a challenge for the dermo-cosmetic and dermo-pharmaceutical fields as our study demonstrates an extensive and diverse collagen phenotype across the donors’ ages with unique properties for everyone. Such diversity is especially true for the photo-exposed skin with the most heterogeneous biophysical properties.
On the contrary, the photo-protected skin remains more biomechanically homogeneous and presents some evident structural characteristics. Our study suggests that the relative difference in the collagen biophysical properties between the papillary and reticular dermis can be the most effective discriminator to differentiate between chronological and biological aging and the anatomical site. Furthermore, this differential phenotypic assessment of collagen at the nanoscale can be used to assess subtle variations in the collagen properties in dermal localized pathological or genetic conditions (Arseni et al., 2018), such as Scleroderma or Ehlers-Danlos Syndrome (Angwin et al., 2020), for example. With the advent of artificial intelligence to support image processing and data analysis, we anticipate that such studies’ time-consuming (and therefore resource-costly) nature would be significantly reduced. By implementing Convolutional Neural Network (CNN) approaches37 to identify pathology-related morphological collagen fingerprints, it will be possible to create CNN models to predict either early signs or the severity of collagen-based conditions (Arseni et al., 2018).
Conclusion
Our study presents a significant milestone in understanding the complex nature of connective tissue, such as skin, as a function of aging. Here, we demonstrate that we can differentiate between individuals based on their biological age by considering individual skin samples as unique statistical entities with defined structural and mechanical variations in the reticular and papillary dermal collagen. Here, we used an AFM to image and quantify the collagen in the dermis before manually analyzing all the images. Using this approach supported by PCA, we demonstrated how the relative variations in structure and mechanics of the dermal collagen could be used to determine individuals’ biological age. Our approach requires advanced knowledge of collagen morphological variations at the nanoscale, for which no established standards exist. Yet, accessing the collagen structure at that scale is fundamental to help define candidate histological markers. As such, we are on course to create a new field of histology: quantitative nanohistology, to probe, quantify and explore connective tissues’ structural and functional properties at the sub-micron level.
Data availability statement
The data will be made available upon reasonable request to the corresponding author.
Ethics statement
The studies involving human participants were reviewed and approved by L’Oreal Research & Innovation. The patients/participants provided their written informed consent to participate in this study.
Author contributions
SH contributed to the conception, study design, data interpretation, and curation drafted and critically revised the manuscript; AS, AM, and SS contributed to the data acquisition, and pilot data analysis (not shown here) and critically revised the manuscript; PB contributed to the statistical design and interpretation, drafted and critically revised the manuscript; SA, MV, and HM-P contributed to the study design, data interpretation, incl. statistical analyses and critically revised the manuscript, MG and AP contributed to the conception and critically revised the manuscript; HP and LB contributed to the conception, study design (both experimental and statistical), data acquisition and analysis, drafted and critically revised the manuscript. All authors contributed to the article and approved the submitted version.
Acknowledgments
We would like to thank the London Centre for Nanotechnology (London United Kingdom) for granting access to the AFM Facility and UCL Consultants for their support.
Conflict of interest
The authors declare that the research was conducted in the absence of any commercial or financial relationships that could be construed as a potential conflict of interest.
Publisher’s note
All claims expressed in this article are solely those of the authors and do not necessarily represent those of their affiliated organizations, or those of the publisher, the editors and the reviewers. Any product that may be evaluated in this article, or claim that may be made by its manufacturer, is not guaranteed or endorsed by the publisher.
References
Achterberg, V. F., Buscemi, L., Diekmann, H., Smith-Clerc, J., Schwengler, H., Meister, J. J., et al. (2014). The nano-scale mechanical properties of the extracellular matrix regulate dermal fibroblast function. J. Invest. Dermatol 134, 1862–1872. doi:10.1038/jid.2014.90
Ahmed, T., Nash, A., Clark, K. E., Ghibaudo, M., de Leeuw, N. H., Potter, A., et al. (2017). Combining nano-physical and computational investigations to understand the nature of "aging" in dermal collagen. Int. J. Nanomedicine 12, 3303–3314. doi:10.2147/IJN.S121400
Angwin, C., Ghali, N., Baker, D., Brady, A. F., Pope, F. M., Vandersteen, A., et al. (2020). Electron microscopy in the diagnosis of ehlers-danlos syndromes: Correlation with clinical and genetic investigations. Br. J. Dermatol 182, 698–707. doi:10.1111/bjd.18165
Arseni, L., Lombardi, A., and Orioli, D. (2018). From structure to phenotype: Impact of collagen alterations on human health. Int. J. Mol. Sci. 19, 1407. doi:10.3390/ijms19051407
Bailey, A. J. (2001). Molecular mechanisms of ageing in connective tissues. Mech. ageing Dev. 122, 735–755. doi:10.1016/s0047-6374(01)00225-1
Binnig, G., Quate, C. F., and Gerber, C. (1986). Atomic force microscope. Phys. Rev. Lett. 56, 930–933. doi:10.1103/PhysRevLett.56.930
Bonté, F., Girard, D., Archambault, J. C., and Desmoulière, A. (2019). “Skin changes during ageing,” in Biochemistry and cell biology of ageing: Part II clinical science. Editors J. Robin Harris,, and V. I. Korolchuk (Singapore: Springer), 249–280.
Chambers, E. S., and Vukmanovic-Stejic, M. (2020). Skin barrier immunity and ageing. Immunology 160, 116–125. doi:10.1111/imm.13152
Chernoff, E. A. G., and Chernoff, D. A. (1992). Atomic force microscope images of collagen fibers. J. Vac. Sci. Technol. A 10, 596–599. doi:10.1116/1.577736
Cox, T. R., Bird, D., Baker, A. M., Barker, H. E., Ho, M. W. Y., Lang, G., et al. (2013). LOX-mediated collagen crosslinking is responsible for fibrosis-enhanced metastasis. Cancer Res. 73, 1721–1732. doi:10.1158/0008-5472.CAN-12-2233
Crichton, M. L., Donose, B. C., Chen, X., Raphael, A. P., Huang, H., and Kendall, M. A. F. (2011). The viscoelastic, hyperelastic and scale dependent behaviour of freshly excised individual skin layers. Biomaterials 32, 4670–4681. doi:10.1016/j.biomaterials.2011.03.012
Fang, M., Goldstein, E. L., Turner, A. S., Les, C. M., Orr, B. G., Fisher, G. J., et al. (2012). Type I collagen D-spacing in fibril bundles of dermis, tendon, and bone: Bridging between nano- and micro-level tissue hierarchy. ACS Nano 6, 9503–9514. doi:10.1021/nn302483x
Farage, M. A., Miller, K. W., Elsner, P., and Maibach, H. I. (2008). Intrinsic and extrinsic factors in skin ageing: A review. Int. J. Cosmet. Sci. 30, 87–95. doi:10.1111/j.1468-2494.2007.00415.x
Fenske, N. A., and Lober, C. W. (1986). Structural and functional changes of normal aging skin. J. Am. Acad. Dermatol 15, 571–585. doi:10.1016/s0190-9622(86)70208-9
Fisher, G. J., Kang, S., Varani, J., Bata-Csorgo, Z., Wan, Y., Datta, S., et al. (2002). Mechanisms of photoaging and chronological skin aging. Arch. Dermatol 138, 1462–1470. doi:10.1001/archderm.138.11.1462
Franco, A. C., Aveleira, C., and Cavadas, C. (2022). Skin senescence: Mechanisms and impact on whole-body aging. Trends Mol. Med. 28, 97–109. doi:10.1016/j.molmed.2021.12.003
Harding, J., and Sneddon, I. (1945). The elastic stresses produced by the indentation of the plane surface of a semi-infinite elastic solid by a rigid punch. Math. Proc. Camb. Philosophical Soc. 41, 16–26. doi:10.1017/S0305004100022325
Jolliffe, I. T., and Cadima, J. (2016). Principal component analysis: A review and recent developments. Philos. Trans. A Math. Phys. Eng. Sci. 374, 20150202. doi:10.1098/rsta.2015.0202
Khavkin, J., and Ellis, D. A. (2011). Aging skin: Histology, physiology, and pathology. Facial Plast. Surg. Clin. North Am. 19, 229–234. doi:10.1016/j.fsc.2011.04.003
Kiio, T. M., and Park, S. (2020). Nano-scientific application of atomic force microscopy in pathology: From molecules to tissues. Int. J. Med. Sci. 17, 844–858. doi:10.7150/ijms.41805
Lynch, B., Pageon, H., Le Blay, H., Brizion, S., Bastien, P., Bornschlögl, T., et al. (2022). A mechanistic view on the aging human skin through ex vivo layer-by-layer analysis of mechanics and microstructure of facial and mammary dermis. Sci. Rep. 12, 849. doi:10.1038/s41598-022-04767-1
Monnier, V. M., Mustata, G. T., Biemel, K. L., Reihl, O., Lederer, M. O., Zhenyu, D., et al. (2005). Cross-Linking of the extracellular matrix by the maillard reaction in aging and diabetes: An update on “a puzzle nearing resolution”. Ann. N. Y. Acad. Sci. 1043, 533–544. doi:10.1196/annals.1333.061
Penuela, L., Negro, C., Massa, M., Repaci, E., Cozzani, E., Parodi, A., et al. (2018). Atomic force microscopy for biomechanical and structural analysis of human dermis: A complementary tool for medical diagnosis and therapy monitoring. Exp. Dermatol 27, 150–155. doi:10.1111/exd.13468
Perrone, A., Giovino, A., Benny, J., and Martinelli, F. (2020). Advanced glycation end products (AGEs): Biochemistry, signaling, analytical methods, and epigenetic effects. Oxid. Med. Cell. Longev. 2020, 3818196. doi:10.1155/2020/3818196
Petruska, J. A., and Hodge, A. J. (1964). A subunit model for the tropocollagen macromolecule. Proc. Natl. Acad. Sci. U. S. A. 51, 871–876. doi:10.1073/pnas.51.5.871
Rittie, L., and Fisher, G. J. (2015). Natural and sun-induced aging of human skin. Cold Spring Harb. Perspect. Med. 5, a015370. doi:10.1101/cshperspect.a015370
Saito, M., and Marumo, K. (2010). Collagen cross-links as a determinant of bone quality: A possible explanation for bone fragility in aging, osteoporosis, and diabetes mellitus. Osteoporos. Int. 21, 195–214. doi:10.1007/s00198-009-1066-z
Salsberg, J., Andriessen, A., Abdulla, S., Ahluwalia, R., Beecker, J., Sander, M., et al. (2019). A review of protection against exposome factors impacting facial skin barrier function with 89% mineralizing thermal water. J. Cosmet. Dermatol 18, 815–820. doi:10.1111/jocd.12927
Singh, R., Barden, A., Mori, T., and Beilin, L. (2001). Advanced glycation end-products: A review. Diabetologia 44, 129–146. doi:10.1007/s001250051591
Snedeker, J. G., and Gautieri, A. (2014). The role of collagen crosslinks in ageing and diabetes - the good, the bad, and the ugly. Muscles Ligaments Tendons J. 4, 303–308. doi:10.32098/mltj.03.2014.07
Stammers, M., Ivanova, I. M., Niewczas, I. S., Segonds-Pichon, A., Streeter, M., Spiegel, D. A., et al. (2020). Age-related changes in the physical properties, cross-linking, and glycation of collagen from mouse tail tendon. J. Biol. Chem. 295, 10562–10571. doi:10.1074/jbc.RA119.011031
Strange, A. P., Aguayo, S., Ahmed, T., Mordan, N., Stratton, R., Porter, S. R., et al. (2017). Quantitative nanohistological investigation of scleroderma: An atomic force microscopy-based approach to disease characterization. Int. J. Nanomedicine 12, 411–420. doi:10.2147/IJN.S118690
Stylianou, A. (2022). Assessing collagen D-band periodicity with atomic force microscopy. Mater. (Basel) 15, 1608. doi:10.3390/ma15041608
Swift, A., Liew, S., Weinkle, S., Garcia, J. K., and Silberberg, M. B. (2021). The facial aging process from the "inside out. Aesthet. Surg. J. 41, 1107–1119. doi:10.1093/asj/sjaa339
Szauter, K. M., Cao, T., Boyd, C. D., and Csiszar, K. (2005). Lysyl oxidase in development, aging and pathologies of the skin. Pathol. Biol. Paris. 53, 448–456. doi:10.1016/j.patbio.2004.12.033
Tanzer, M. L. (1973). Cross-linking of collagen. Science 180, 561–566. doi:10.1126/science.180.4086.561
Terao, M., and Katayama, I. (2016). Local cortisol/corticosterone activation in skin physiology and pathology. J. Dermatol Sci. 84, 11–16. doi:10.1016/j.jdermsci.2016.06.014
Tobin, D. J. (2017). Introduction to skin aging. J. Tissue Viability 26, 37–46. doi:10.1016/j.jtv.2016.03.002
Uitto, J. (2008). The role of elastin and collagen in cutaneous aging: Intrinsic aging versus photoexposure. J. Drugs Dermatol 7, s12–s16.
Venkatesh, S., Maymone, M. B. C., and Vashi, N. A. (2019). Aging in skin of color. Clin. Dermatol 37, 351–357. doi:10.1016/j.clindermatol.2019.04.010
Wenger, M. P., Bozec, L., Horton, M. A., and Mesquida, P. (2007). Mechanical properties of collagen fibrils. Biophys. J. 93, 1255–1263. doi:10.1529/biophysj.106.103192
Keywords: collagen, aging, atomic force microscopy, nanomechanics, histology, dermis, skin, statistical methods
Citation: Huang S, Strange A, Maeva A, Siddiqui S, Bastien P, Aguayo S, Vaez M, Montagu-Pollock H, Ghibaudo M, Potter A, Pageon H and Bozec L (2023) Quantitative nanohistology of aging dermal collagen. Front. Aging 4:1178566. doi: 10.3389/fragi.2023.1178566
Received: 03 March 2023; Accepted: 16 May 2023;
Published: 31 May 2023.
Edited by:
Augusto Schneider, Federal University of Pelotas, BrazilReviewed by:
Michael John Sherratt, The University of Manchester, United KingdomVenu Varanasi, University of Texas at Arlington, United States
Copyright © 2023 Huang, Strange, Maeva, Siddiqui, Bastien, Aguayo, Vaez, Montagu-Pollock, Ghibaudo, Potter, Pageon and Bozec. This is an open-access article distributed under the terms of the Creative Commons Attribution License (CC BY). The use, distribution or reproduction in other forums is permitted, provided the original author(s) and the copyright owner(s) are credited and that the original publication in this journal is cited, in accordance with accepted academic practice. No use, distribution or reproduction is permitted which does not comply with these terms.
*Correspondence: Laurent Bozec, bC5ib3plY0B1dG9yb250by5jYQ==
‡ORCID:Sebastian Aguayo, orcid.org/0000-0003-0900-1993; Mina Vaez, orcid.org/0000-0003-1707-3356; Laurent Bozec, orcid.org/0000-0001-6322-7817
†These authors share senior authorship