- University of South Florida, Department of Cell Biology, Microbiology, and Molecular Biology, Tampa, FL, United States
The decline of proteostasis is a hallmark of aging that is, in part, affected by the dysregulation of the heat shock response (HSR), a highly conserved cellular response to proteotoxic stress in the cell. The heat shock transcription factor HSF-1 is well-studied as a key regulator of proteostasis, but mechanisms that could be used to modulate HSF-1 function to enhance proteostasis during aging are largely unknown. In this study, we examined lysine acetyltransferase regulation of the HSR and HSF-1 in C. elegans. We performed an RNA interference screen of lysine acetyltransferases and examined mRNA expression of the heat-shock inducible gene hsp-16.2, a widely used marker for HSR activation. From this screen, we identified one acetyltransferase, CBP-1, the C. elegans homolog of mammalian CREB-binding protein CBP/p300, as a negative regulator of the HSR. We found that while knockdown of CBP-1 decreases the overall lifespan of the worm, it also enhances heat shock protein production upon heat shock and increases thermotolerance of the worm in an HSF-1 dependent manner. Similarly, we examined a hallmark of HSF-1 activation, the formation of nuclear stress bodies (nSBs). In analyzing the recovery rate of nSBs, we found that knockdown of CBP-1 enhanced the recovery and resolution of nSBs after stress. Collectively, our studies demonstrate a role of CBP-1 as a negative regulator of HSF-1 activity and its physiological effects at the organismal level upon stress.
Introduction
One of the hallmarks of aging is the dysregulation of protein homeostasis, or proteostasis, in the cell which is critical for cell survival and function (López-Otín et al., 2013). The heat shock response (HSR) is a key player in the proteostasis network, known to play a role in a variety of physiological processes, including development, reproduction, aging, and age-related diseases (Labbadia and Morimoto, 2015; Joutsen and Sistonen, 2019). The HSR is a highly conserved cellular response that maintains proteostasis in the cell through the activation of heat shock transcription factor 1 (HSF1). HSF1 is the master regulator of the HSR and when activated, drives the expression of heat shock proteins (HSPs) that act as molecular chaperones to restore proteostasis after stress (Hartl et al., 2011). HSF1 has been implicated in a wide array of age-related diseases, including neurodegenerative diseases, metabolic diseases, and cancer (Powers et al., 2009; Gomez-Pastor et al., 2017). Understanding HSF1 regulation with aging may be useful when developing therapeutic strategies for such diseases.
The transcriptional activity of HSF1 is stress-inducible and highly regulated (Westerheide et al., 2012). Human HSF1 is regulated in part through post-translational modifications. These modifications include acetylation, phosphorylation, and sumoylation, and they function in both the activation and attenuation of HSF1 (Gomez-Pastor et al., 2017). Studies have shown multiple site-specific, reversible acetylation modifications on HSF1 that affect its overall protein stability and attenuation (Westerheide et al., 2009; Raychaudhuri et al., 2014; Zelin and Freeman, 2015). Under non-stress conditions, HSF1 levels are stabilized by the acetylation of Lys208 and Lys298 by the lysine acetyltransferase (KAT) CBP/p300 (Raychaudhuri et al., 2014). During stress, CBP/p300 also mediates the acetylation of Lys80 of HSF1, which aids in inhibiting HSF1 binding to DNA to attenuate the HSR (Westerheide et al., 2009; Raychaudhuri et al., 2014). There are also multiple other known sites of HSF1 acetylation where the effect of acetylation has yet to be elucidated.
The activity of HSF-1, the C. elegans homolog of HSF1, is highly conserved and makes C. elegans a useful model organism to study the regulation of HSF-1 during stress and aging. HSF-1 has been shown to interact with CREB binding protein CBP-1, the C. elegans homolog of CBP/p300, in lifespan regulation (Zhang et al., 2009); however, the mechanism by which CBP-1 regulates HSF-1 has not been examined.
CBP-1 in C. elegans functions as a chromatin remodeler and lysine acetyltransferase that can regulate transcription (Victor et al., 2002). CBP-1 is an essential protein for embryonic development, cell differentiation, and aging, and knockdown of cbp-1 significantly reduces lifespan (Shi and Mello 1998; Victor et al., 2002; Zhang et al., 2009; Cai et al., 2019). CBP-1 also acts in multiple stress response pathways, including the mitochondrial unfolded protein response and the oxidative stress response (Ganner et al., 2019; Li et al., 2021). Although CBP-1 interactions with HSF-1 have been described, its role, as well as the potential role of other lysine acetyltransferases, in regulating HSF-1 and the HSR are still unknown.
In this study, we utilized C. elegans to screen for lysine acetyltransferase modulators of the HSR. We identified a role of CBP-1 in negatively regulating the HSR in C. elegans, confirming the importance of this regulator in this stress response pathway. Collectively, our studies demonstrate the ability of CBP-1 to regulate the HSR in an HSF-1 dependent-manner and highlight its effects on stress resistance and longevity.
Methods
C. elegans Strains and Maintenance
The following strains were used in this study: N2 (Bristol), PS3551–hsf-1 (sy441), MH2430—cbp-1 (ku258), SDW015–hsf-1 (asd002 (hsf-1:GFP + unc-119 (+)), and SDW173. The MH2430 strain was outcrossed three times to the N2 wildtype strain to generate the SDW173 strain. Strains were maintained at 20°C on standard NGM plates seeded with Escherichia coli OP50-1. The synchronous population of nematodes was obtained by bleach synchronization and plated for 19 h on NGM plates without food.
RNA Interference
Synchronous L1 nematodes were grown on standard NGM plates seeded with OP50-1 bacteria for 19 h to prevent potential RNAi-mediated effects on early development. Worms were transferred onto standard NGM plates supplemented with 25 μg/ml carbenicillin and 1 mM isopropyl-beta-D-thiogalactopyranoside (IPTG) and seeded with either HT115 bacteria containing an empty vector (EV/L4440 control) or with sequence-verified gene-specific RNAi strains isolated from the Ahringer RNAi library (J. Ahringer, University of Cambridge, Cambridge, U.K.), as previously described (Kamath et al., 2003). To induce dsRNA production, HT115 bacteria were supplemented with 1 mM IPTG shaking at 37°C for 1 h before seeding.
RNA Isolation and Quantitative PCR
RNA was extracted with TRIzol® reagent (Ambion®, cat# 15,596–026) by standard protocol. RNA was reverse-transcribed using a High Capacity cDNA Reverse Transcription Kit (Applied Biosystems, cat# 4,368,814). cDNA was diluted to 100 ng/μl to be used as a template for qRT–PCR performed with the StepOne Plus Real-time PCR system (Applied Biosystems, cat # 4,376,600) using iTaq™ Universal SYBR® Green Supermix (Bio-Rad, cat# 1,725,121) according to the manufacturer’s instructions. Expression levels were analyzed via qPCR using the ΔΔCt method. The housekeeping gene cdc-42 (R07G3.1) was used for normalization. Results show averages of independent biological triplicates performed in technical triplicates. Statistical analysis was performed with GraphPad (GraphPad Software, https://www.graphpad.com) using an unpaired Student’s t-test.
Primers used: hsp16.2 (Y46H3A.3) Fwd: ACGCCAATTTGCTCCAGTCT, Rvs: TGATGGCAAACTTTTGATCATTGT; hsp-70 (C12C8.1) Fwd: TTCAATGGGAAGGACCTCAACT, Rvs: GGCTGCACCAAAGGCTACTG; cdc-42 (R07G3.1) Fwd: CTTCTGAGTATGTGCCGACAGTCT, Rvs: GGCTCGCCACCGATCAT; hsp16.48 (T27E4.3) Fwd: TTGGAGAAATGCTGATCACAACTC, Rvs: TTTTTAGTTCTCTTCCATCCAATTCA; F44E5.5 Fwd: CTTCATGCAAAGCTATTGGTATCG, Rvs: CTTCCGAGTTGGCGAGGAT; cbp-1 (R10E11.1) Fwd: GCAGCGAAAACGGAGGAA, Rvs: GCATGGAACAAATGTGGAGTCTT; hsf-1 (Y53C10A.12) Fwd: TGCAGCCAGGATTGTCGA, Rvs: GGCGGCGCAAAAGTCTATT; hat-1 (M03C11.4) Fwd: ACGGACTTGCTGTCGTTAAA, Rvs: CCGAAGATTGTCTCCTCATCTC; kat-1 (T02G5.8) Fwd: CCACATCTGCTGCACTATCA, Rvs: GCAGTTACCGAAGAGAGAGAAG; taf-1 (W04A8.7) Fwd: TACGAGGCCACAGCTTATTG, Rvs: CGCTTCTCCTCCTTATACTGTTC; mys-1 (VC5.4) Fwd: CGAGCTGCAAATGGTTCAATTA, Rvs: GTAGCTCACACGACGCTAAA; mys-2 (K03D10.3) Fwd: GGAGCGAAAGAGCTCATGTATT; Rvs: GCTCGACTACCACTTCGTTTAC; mec-17 (F57H12.7) Fwd: GGTCATCAGAGCAAGGGAAA, Rvs: TTGGCCTGATGAGCTCTATTG; atat-2 (W06B11.1) Fwd: GTTCAGCTGTGTCCAGTCAT, Rvs: TAACATATCCTGGCGCATCAA; T02G5.4 Fwd: GCAGTTACCGAAGAGAGAGAAG, Rvs: CCACATCTGCTGCACTATCA; hsp-1 (F26D10.3) Fwd: TCAAGAGAAACACCACCATCC, Rvs: GGCACGTTCTCCTTCGTAAA; hsp-90 (C47E8.5) Fwd: AGTACTGCGTCCAACAACTC, Rvs: TCTTCTCCTCCTCGGTTTCT.
Thermotolerance Analysis
Thermotolerance was tested by exposing Day 1 animals to 37°C heat shock for 3.5 h and then determining survival 48 h later by assessing response to a gentle touch. For each trial, ∼ 100 randomly selected individual animals were assessed for the fraction alive (live/total). Thermotolerance assay data reflects three biologically independent trials. Data was plotted as the % survival using GraphPad Prism (GraphPad Software, https://www.graphpad.com) and was analyzed with a two-tailed t test.
Lifespan Analysis
All lifespan assays were performed at 20°C with approximately 100 worms per condition in biological triplicate. Animals were transferred to fresh plates daily for 5 days to avoid progeny contamination. Adult worms were scored approximately every day and counted as dead when no response was observed by poking with a platinum wire. The average survivability of three replicates was plotted using OASIS 2 (https://sbi.postech.ac.kr/oasis2/) (Han et al., 2016), and statistical analysis was done by Log-rank (Mantel-Cox) Test.
Fluorescent Microscopy and Nuclear Stress Body Assessment
Fluorescent images were obtained using a Keyence BZ-X fluorescent microscope. Animals were picked free of bacteria and anesthetized with 10 mM levamisole. Nuclear stress body formation was quantified by assessing for the presence of nuclear foci containing HSF-1:GFP in hypodermal cells. The heat shock conditions for nuclear stress body assessment were 15 min in a 33°C water bath on plates wrapped in parafilm. After anesthetizing and placing the cover slip on top of the worms, they were imaged within 10–15 min to avoid the formation of nuclear stress bodies which may be due to hypoxia or other cytotoxic stress (n = ∼ 10 worms per condition). Quantification was performed in GraphPad Prism (GraphPad Software, www.graphpad.com) and significance was determined using 2-way ANOVA.
Statistical Analyses
Statistical analyses were carried out with GraphPad Software (GraphPad Software, La Jolla, CA, United States, https://www.graphpad.com) unless otherwise stated. All error bars are representative of standard deviation between independent biological replicates, as indicated.
Results
Targeted-RNAi Screen for Lysine Acetyltransferase Modulators of the HSR Identifies CBP-1
To identify lysine acetyltransferases that may regulate the HSR, we performed an RNA interference (RNAi) screen by targeting ∼ 75% of all putative lysine acetyltransferases (KATs) in C. elegans using RNAi knockdown (Figure 1A) C. elegans KATs were identified by searching the C. elegans protein database for proteins containing conserved acetyltransferase domains to those of known human KATs (Sheikh and Akhtar, 2019). We utilized quantitative RT-PCR to assess the heat shock inducibility of hsp-16.2 mRNA expression, a widely used marker of HSR activity, after KAT RNAi knockdown (Golden et al., 2020). We induced RNAi 19 h after the L1 stage until Day 1 of adulthood, when we extracted RNA before and immediately after a 1-h heat shock at 33°C to assess hsp-16.2 expression (Figure 1B). Our KAT and hsf-1 RNAi conditions suppress expression of their corresponding genes by about 50% (Supplementary Figure S1). From this screen, we found no significant changes in hsp-16.2 expression in non-heat shock conditions after KAT RNAi knockdown (Figure 1C). After heat shock, only RNAi for cbp-1 (R10E11.1), the homolog of human CBP/p300 CREB binding protein, significantly altered the mRNA expression of hsp16.2 expression, resulting in an increase in expression of approximately 2-fold (Figure 1C).
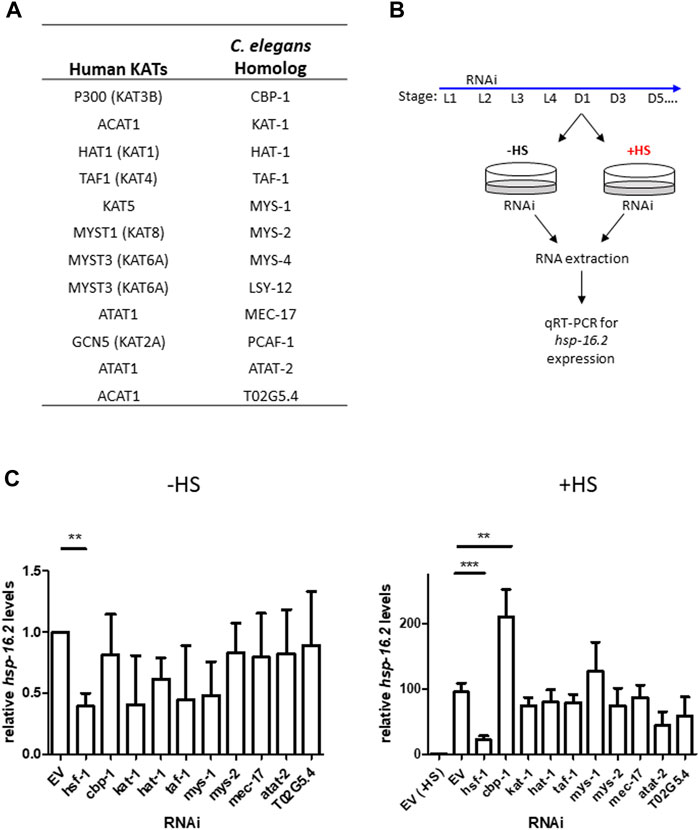
FIGURE 1. Targeted-RNAi screen for lysine acetyltransferase modulators of the HSR identifies CBP-1. (A) Table of all putative lysine acetyltransferases (KATs) and their human homologs. (B) Schematic of the targeted RNAi screen for lysine acetyltransferase modulators of the HSR. ∼ 75% of worm KATs were screened by examining endogenous hsp-16.2 gene expression, a heat-inducible heat shock protein gene, before and after heat shock using qRT-PCR. (C) N2 (wildtype) animals were fed empty vector (EV) control or KAT RNAi from 19 h after L1 larval stage to Day 1 of adulthood. RNA was extracted from Day 1 animals before and immediately after a 1-h heat shock at 33°C and hsp-16.2 levels were quantified by qRT-PCR using the ΔΔCt method (n = 3 biologically independent samples). The housekeeping gene cdc-42 (R07G3.1) was used for normalization. Statistical analysis was determined by conducting a One-Way ANOVA using GraphPad Prism (GraphPad Software, www.graphpad.com) followed by a Tukey post hoc test comparison of all columns. *p-value < 0.05, **p-value < 0.01, ***p-value < 0.001.
CBP-1 Regulates the Expression of Multiple HSF-1 Target Genes in an HSF-1-Dependent Manner
To further examine the role of CBP-1 on the HSR, we examined how knockdown of cbp-1 affects various heat shock inducible HSF-1 target genes using qRT-PCR. The target genes tested, including hsp-16.2, hsp.16.48, hsp-70, and F44E5.5 (an inducible hsp-70 family gene), are highly inducible upon heat shock. We found that while cbp-1 RNAi didn’t significantly change the expression of the hsp target genes tested under basal conditions (Supplementary Figure S2A), cbp-1 RNAi did significantly increase the expression of these genes after heat shock as compared to EV control (Figure 2A). We also found that cbp-1 knockdown does not affect hsf-1 expression, or vice versa (Supplementary Figure S1A). HSF-1 also regulates the expression of a distinct subset of developmental genes independent of the HSR, including hsp-1 and hsp-90 (Li et al., 2016). We found that cbp-1 knockdown does not affect expression of these HSF-1 target genes (Supplementary Figure S2B). Thus, CBP-1 is negatively regulating HSP but not HSF-1 expression after heat stress.
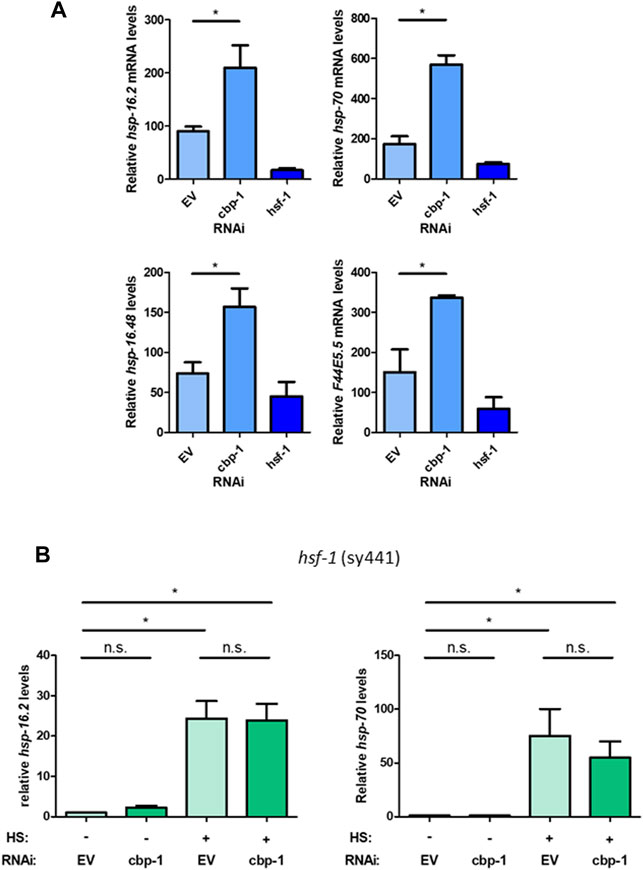
FIGURE 2. CBP-1 regulates the expression of multiple HSF-1 target genes in an HSF-1-dependent manner. (A) RNA was extracted from N2 (wildtype) worms on Day 1 of adulthood with a 1-h heat shock at 33°C that were fed empty vector (EV), cbp-1, or hsf-1 RNAi beginning 19 h after L1. Heat shock inducible heat shock protein gene expression levels for hsp-16.2, hsp-16.48, F44E5.5, and hsp-70 were quantified by qRT-PCR. (B) RNA was extracted from an HSF-1 deletion worm strain (PS3551) on Day 1 of adulthood with and without a 1-h heat shock at 33°C with animals fed EV or cbp-1 RNAi. PS3551 animals were fed empty vector (EV) control or cbp-1 RNAi from 19 h after L1 larval stage to Day 1 of adulthood. Heat shock inducible hsp-16.2 and hsp-70 levels were quantified by qRT-PCR. Statistical analysis was determined by determined by conducting a One-Way ANOVA using GraphPad Prism (GraphPad Software, www.graphpad.com) followed by a Tukey post hoc test comparison of all columns. *p-value < 0.05.
To determine whether this negative regulation of CBP-1 is mediated directly through HSF-1, we used the PS3551 worm strain containing a non-functional HSF-1 (Zhou et al., 2018). This mutant contains a point mutation in the HR-C domain of HSF-1 that causes the expression of a truncated non-functional HSF-1 mutant. We then measured the expression of hsp-16.2 and hsp-70 (C12C8.1) in response to cbp-1 RNAi (beginning 19 h after L1) before and after heat shock in this mutant to determine dependence on HSF-1. While we found that knockdown of cbp-1 increased hsp-16.2 expression after heat shock with wildtype HSF-1 (Figure 1A), that increase is abolished with a nonfunctional HSF-1. Knockdown of cbp-1 does not significantly increase hsp-16.2 or hsp-70 (C12C8.1) expression after heat shock compared to control (Figure 2B). We conclude that CBP-1 depends on HSF-1 in order to regulate hsp gene expression.
Effect of cbp-1 and hsf-1 RNAi on Thermotolerance and Lifespan
We next wanted to test whether CBP-1 also regulates other physiological readouts of the HSR. To test for resistance to heat stress, we examined thermotolerance, a physiological effect known to be modulated by HSF-1. To test this, we fed the worms cbp-1, hsf-1, or control RNAi from 19 h after L1 until Day 1 of adulthood prior to subjecting the worms to a lethal heat shock of 37°C for 3.5 h. After a 48-h recovery, the worms were scored alive/dead after a gentle touch. Compared to empty vector control RNAi-treated worms, hsf-1 RNAi-treated worms had significantly reduced survival after heat shock ( ∼ 20% decrease), as expected (Figure 3A). However, knockdown of cbp-1 increased the % survival of the worms by ∼ 40% (Figure 3A). Thus, knockdown of cbp-1 increases thermotolerance.
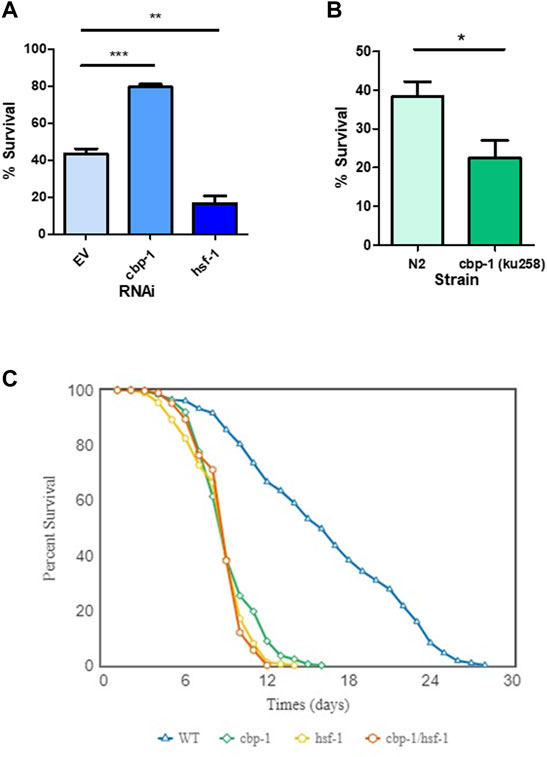
FIGURE 3. Effect of CBP-1 on thermotolerance and lifespan. (A) In order to assess CBP-1 activity on resistance to heat stress, N2 worms were given a lethal heat shock of 3.5 h at 37°C on Day 1 of adulthood and then ∼ 100 animals were randomly scored for survival after a 48-h recovery. Percent survival was determined for worms fed with empty vector (EV), cbp-1, and hsf-1 RNAi. Data was plotted as % survival using GraphPad Prism (GraphPad Software, www.graphpad.com) and was analyzed with a two-tailed t test. *p-value < 0.05, **p-value < 0.01, ***p-value < 0.001. (B) Thermotolerance was assessed in N2 and SDW173, a strain containing a gain-of-function allele of cbp-1 that increases acetyltransferase activity (MH2430 strain outcrossed 3x to N2). Data was plotted as % survival using GraphPad Prism (GraphPad Software, www.graphpad.com) and was analyzed with a two-tailed t test. *p-value < 0.05. (C) Lifespan analysis was performed in wild type N2 animals fed with EV, cbp-1 RNAi, hsf-1 RNAi, or cbp-1/hsf-1 double RNAi throughout the lifespan. Worms were scored approximately every day for survival. The average survivability of three replicates was plotted using OASIS 2 (https://sbi.postech.ac.kr/oasis2/) (Han et al., 2016), and statistical analysis was done by Log-rank (Mantel-Cox) Test (Shown in Supplementary Table S1).
We then performed a complimentary experiment, assessing how an increase in CBP-1 activity would affect thermotolerance. We utilized the MH3420 strain that contains two point mutations in cbp-1, resulting in a gain-of-function allele with increased KAT activity (Eastburn and Han, 2005). This strain was outcrossed three times with our laboratory N2 strain to create the SDW173 strain. N2 and SDW173 worms were synchronized and grown to Day 1 of adulthood then subjected to a lethal heat shock of 37°C for 3.5 h. We found that SDW173 worms had an ∼20% decrease in percent survival as compared to N2 wildtype worms (Figure 3B). This suggests that an increase in CBP-1 acetyltransferase activity decreases thermotolerance. In summary, cbp-1 RNAi activates thermotolerance, while a cbp-1 gain-of-function mutant inhibits thermotolerance.
Previous studies have found that cbp-1 RNAi reduces the lifespan of the worm (Zhang et al., 2009; Cai et al., 2019). We wanted to test whether this effect depends on HSF-1. Worms were fed with EV control, cbp-1, hsf-1, or cbp-1/hsf-1 double RNAi from 19 h after L1 throughout their lifespans. The worms were scored approximately every day starting at day 1 of adulthood for survival, and dead worms were scored when non-responsive to poking with a platinum wire. We found that cbp-1 RNAi decreased lifespan to a significantly lesser extent than hsf-1 RNAi (Figure 3C). However, the cbp-1/hsf-1 double RNAi did not further reduce lifespan (Figure 3C).
cbp-1 RNAi Increases Recovery Rate of Nuclear Stress Bodies After Heat Shock
Upon activation of the HSR, HSF-1 undergoes localization changes and forms nuclear stress bodies (nSBs), a hallmark of HSF-1 activation (Deonarine et al., 2021). nSBs require HSF-1 binding and are a sign of activation of the HSR (Morton and Lamitina, 2013). After HSR activation, nSBs form within 5 min of stress detection and gradually dissolve until HSF-1 is diffuse in the nucleus (Figure 4A) (Deonarine et al., 2021). We examined the effect of cbp-1 RNAi on the rate of nSB recovery after stress. We used the SDW015 (HSF-1:GFP) strain to visualize nSB formation before and after a 15-min heat shock at 33°C on Day 2 of adulthood. We utilized fluorescence microscopy to image hypodermal cells of the worm in 30-min increments until the majority of cells containing nSBs had diffuse HSF-1:GFP expression in the nuclei (Figure 4A). After heat shock, control worms took an average of 1.7 h to recover and resolve their nSBs, whereas worms fed cbp-1 RNAi took an average of 1.1 h to resolve nSBs (Figure 4B). Thus, knockdown of cbp-1 significantly increased the rate of recovery of nSBs after heat shock.
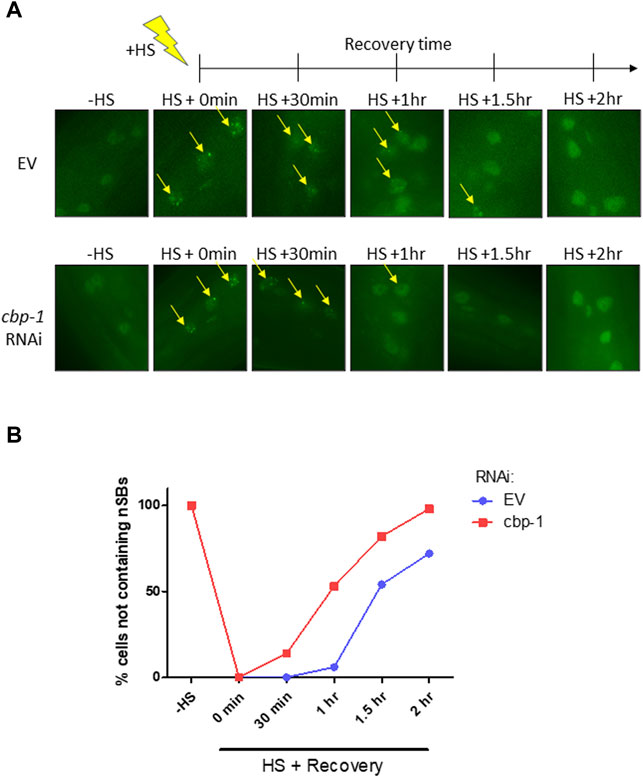
FIGURE 4. cbp-1 RNAi increases recovery rate of nuclear stress bodies (nSBs) after heat shock. (A) Fluorescent images of HSF-1:GFP in the SDW015 strain were examined before heat shock and in 30-min increments after a 15-min heat shock at 33°C for 2 h at 20°C until the majority of hypodermal cells containing nuclear stress bodies (nSBs) had been resolved. Images represent fluorescent nSB formation in hypodermal cells under control and cbp-1 RNAi conditions at each time point in Day 2 adult worms. Yellow arrows indicate hypodermal cell nuclei containing nSB foci. (B) The percentage of hypodermal cells not containing nSBs were quantitated following fluorescent imaging of worms fed empty vector (EV) control and cbp-1 RNAi (n = 10 worms). Significance was determined using 2-way ANOVA. Column *p-value = 0.0497; row **p-value = 0.0022.
Discussion
Given the importance of post-translational modifications on HSF-1 regulation and function, we sought to elucidate the effect of lysine acetyltransferase regulation on the HSR. Here, we conducted a targeted RNAi screen for lysine acetyltransferase regulation of hsp-16.2 expression levels and identified CBP-1 as a regulator of hsp expression. In addition, cbp-1 knockdown also increased the expression of multiple other heat shock genes, increased thermotolerance, and improved the recovery rate of nuclear stress bodies after heat stress. Overall, these studies suggest a role for CBP-1 in negatively regulating HSF-1 activity during stress.
CBP-1 is widely known to be a transcriptional coactivator that aids in transcription (Victor et al., 2002). We further examined various other heat-shock inducible hsp expression and found that cbp-1 knockdown increases the expression of multiple hsps (hsp-16.2, hsp.16.48, hsp-70, and F44E5.5) after heat shock. This type of negative transcriptional regulation is a novel role for CBP-1 in C. elegans. However, human CBP/p300 has some characterized instances in which it negatively regulates transcription through effects on transcription factors. For example, acetylation of the elongation factor AFF1 by CBP/p300 inhibits transcription during genotoxic stress (Kumari et al., 2019).
CBP-1 is an essential protein that regulates multiple physiological processes including cell differentiation, embryonic development, metabolism, lifespan, and aging (Shi and Mello, 1998; Zhang et al., 2009; Vora et al., 2013; Cai et al., 2019). Specifically, CBP-1 interacts with DAF-16 and HSF-1 to regulate lifespan during caloric restriction (Zhang et al., 2009). Similarly, our results suggest that CBP-1 and HSF-1 work in the same pathway to regulate lifespan. While our work suggests that CBP-1 negatively regulates the HSR, our lifespan results suggest that it positively affects lifespan and aging. This was unexpected, as activating the HSR generally protects from aging (Hsu et al., 2003; Morley and Morimoto, 2004). Since CBP-1 plays a positive role in a variety of physiological pathways that control aging (Zhang et al., 2009; Cai et al., 2019; Ganner et al., 2019; Zhou et al., 2019; Li et al., 2021) it may be that the effects on these pathways are overriding the effects of CBP on HSF-1 and HSP expression in terms of life span regulation. It will be informative in future work to determine how the regulation of HSF-1 activity by CBP-1 can benefit aging and potentially lifespan in the worm.
While our data indicates that CBP-1 regulates HSF-1 activity, the mechanism of this regulation is still unknown. CBP-1 could directly acetylate HSF-1 as one potential mechanism. Mammalian CBP/p300 acetylates HSF-1 under both stress and non-stress conditions to both stabilize HSF-1 and attenuate HSF-1 from the DNA (Westerheide et al., 2009; Raychaudhuri et al., 2014). CBP/p300 acetylation of HSF1 could be conserved in C. elegans and function to attenuate HSF-1 activity. While human CBP/p300 also acts as a scaffolding protein to recruit the transcriptional machinery (Holmqvist and Mannervik, 2013), it is likely that worm CBP-1 regulation of the HSR requires CBP-1 acetyltransferase activity since we found that the SDW173 mutant containing CBP-1 with hyperactive KAT activity decreased thermotolerance. There is also the possibility of CBP-1 regulating HSF-1 through an indirect regulator, as CBP-1 also binds to a multitude of other factors including the stress-responsive factors SKN-1 and DAF-16 (Nasrin et al., 2000; Ganner et al., 2019). Future work to uncover the mechanism behind HSF-1 regulation will allow for a more detailed analysis of the role of CBP-1 during stress and aging.
A hallmark of HSF1 activation in both mammalian cells and C. elegans is the formation and resolution of nuclear stress bodies (nSBs) containing HSF1 (Jolly et al., 1999; Jolly et al., 2004; Biamonti and Vourc’h, 2010; Morton and Lamitina, 2013; Deonarine et al., 2021). In mammalian cells, the resolution of nSBs correlates with HSF1 activity, transcription of hsp target genes, and cell survival at the single cell level (Gaglia et al., 2020). A recent mammalian study discovered the existence of both HSF1 nSBs at non-hsp gene loci and smaller HSF1 condensates at transcriptionally active hsp gene loci upon heat shock (Zhang et al., 2022). In this study, HSF1 in the condensates was found to colocalize with transcription apparatus factors, including RNA Pol II, BRD4, MED1, and CYCT1 (Zhang et al., 2022). It is thus possible that upon heat shock, HSF1 in nSBs sequesters transcription factors to halt general transcription, while HSF1 in the smaller condensates functions to induce the transcription of hsp target genes. HSF1 nSBs that are not able to resolve may go on to transition from liquid to gel phase condensates, which could then permanently hinder HSF1 activity and the HSR. Our study did not analyze small HSF-1 condensates, and it is not yet known if these are present in the worm. However, we found that cbp-1 knockdown promotes the recovery of large HSF-1 nSBs after heat shock. Thus, CBP-1 may facilitate the stabilization of HSF-1 nSBs, which could promote the gel phase state upon prolonged stress, leading to ultimate HSF-1 inactivation. We hypothesize that decreasing CBP-1 levels through RNAi could thus enhance the HSR. We do not know how CBP-1 may stabilize HSF-1 nSBs. It could be through the acetylation of HSF-1 or another protein, through providing a scaffolding, or through an alternative mechanism. It will be interesting to investigate these possibilities in future work.
CBP-1 also functions as a regulator of multiple other stress response pathways. Interestingly, CBP-1 positively regulates SKN-1/Nrf activation and the oxidative stress response, where it regulates SKN-1 activity and protein abundance (Ganner et al., 2019). CBP-1 is also an essential regulator of the mitochondrial unfolded protein response (Li et al., 2021). Collectively, our studies identify a new role of CBP-1 in HSR regulation in C. elegans and its physiological effects at the organismal level. CBP-1 negatively regulates HSP expression, nuclear stress body recovery, and thermotolerance ability of the worm. Future work is needed to determine the precise mechanism of action of CBP-1 in the HSR pathway and any potential acetylation changes of HSF-1 that are induced by CBP-1.
Data Availability Statement
The original contributions presented in the study are included in the article/Supplementary Material. Further inquiries can be directed to the corresponding author.
Author Contributions
LB and SW designed the study. LB performed the experiments and the data analyses. LB and SW contributed to figure design and wrote the manuscript.
Funding
This work was funded by NIH grant AG052149. All strains were provided by the CGC, which is funded by NIH Office of Research Infrastructure Programs (P40 OD010440).
Conflict of Interest
The authors declare that the research was conducted in the absence of any commercial or financial relationships that could be construed as a potential conflict of interest.
Publisher’s Note
All claims expressed in this article are solely those of the authors and do not necessarily represent those of their affiliated organizations, or those of the publisher, the editors and the reviewers. Any product that may be evaluated in this article, or claim that may be made by its manufacturer, is not guaranteed or endorsed by the publisher.
Acknowledgments
The authors acknowledge Andrew Deonarine, Doreen I. Lugano, Younghoon Kee, and Eric Guisbert for their helpful advice.
Supplementary Material
The Supplementary Material for this article can be found online at: https://www.frontiersin.org/articles/10.3389/fragi.2022.861761/full#supplementary-material
Supplementary Figure S1 | Validation of KAT and hsf-1 RNAi. (A) RNA was extracted from wildtype (N2) worms on Day 1 of adulthood that were fed empty vector (EV), cbp-1, or hsf-1 RNAi beginning 19 hrs after L1. cbp-1 and hsf-1 levels were quantified by qRT-PCR (n = 3 biologically independent samples). (B) RNA was extracted from N2 worms on Day 1 of adulthood that were fed EV or KAT RNAi beginning 19 hrs after L1. Validation of KAT knockdown was examined by quantifying KAT levels by qRT-PCR (n = 3 biologically independent samples). Statistical analysis determined by determined by conducting a One-Way ANOVA using GraphPad Prism (GraphPad Software, www.graphpad.com) followed by a Tukey post hoc test comparison of all columns. *p-value < 0.05, **p-value < 0.01, ***p-value < 0.001.
Supplementary Figure S2 | cbp-1 RNAi does not change the expression of HSF-1 target genes under basal conditions. (A) RNA was extracted from N2 (wildtype) worms on Day 1 of adulthood under basal conditions at 20°C that were fed empty vector (EV), cbp-1, or hsf-1 RNAi beginning 19 hrs after L1. Heat shock inducible heat shock protein gene expression levels for hsp-16.2, hsp-16.48, F44E5.5, and hsp-70 were quantified by qRT-PCR. (B) RNA was extracted from N2 worms on Day 1 of adulthood under basal conditions that were fed EV or cbp-1 RNAi beginning 19 hrs after L1. Developmental HSF-1 target genes independent of the heat shock response, hsp-1 and hsp-90, were quantified by qRT-PCR. Statistical analysis was determined by determined by conducting a One-Way ANOVA using GraphPad Prism (GraphPad Software, www.graphpad.com) followed by a Tukey post hoc test comparison of all columns. *p-value < 0.05.
Supplementary Table S1 | Lifespan analysis. Analysis of lifespan of EV, cbp-1, hsf-1, and cbp-1/hsf-1 RNAi including mean lifespan and total/censored/scored number of worms per trial (done in biological triplicate). Survivability was plotted using OASIS 2 (https://sbi.postech.ac.kr/oasis2/) (Han et al. 2016), and statistical analysis was done by Log-rank (Mantel-Cox) Test.
References
Biamonti, G., and Vourc'h, C. (2010). Nuclear Stress Bodies. Cold Spring Harbor. Perspect. Biol. 2 (6), a000695. doi:10.1101/cshperspect.a000695
Cai, H., Dhondt, I., Vandemeulebroucke, L., Vlaeminck, C., Rasulova, M., and Braeckman, B. P. (2019). CBP-1 Acts in GABAergic Neurons to Double Life Span in Axenically Cultured Caenorhabditis elegans. Journals Gerontol. Ser. A Biol. Sci. Med. Sci. 74 (8), 1198–1205. doi:10.1093/gerona/glx206
Deonarine, A., Walker, M. W. G., and Westerheide, S. D. (2021). HSF-1 Displays Nuclear Stress Body Formation in Multiple Tissues in Caenorhabditis elegans upon Stress and Following the Transition to Adulthood. Cell Stress Chaperones 26 (2), 417–431. doi:10.1007/s12192-020-01188-9
Eastburn, D. J., and Han, M. (2005). A Gain-Of-Function Allele of Cbp-1 , the Caenorhabditis elegans Ortholog of the Mammalian CBP/p300 Gene, Causes an Increase in Histone Acetyltransferase Activity and Antagonism of Activated Ras. Mol. Cel Biol. 25 (21), 9427–9434. doi:10.1128/mcb.25.21.9427-9434.2005
Gaglia, G., Rashid, R., Yapp, C., Joshi, G. N., Li, C. G., Lindquist, S. L., et al. (2020). HSF1 Phase Transition Mediates Stress Adaptation and Cell Fate Decisions. Nat. Cel Biol. 22 (2), 151–158. doi:10.1038/s41556-019-0458-3
Ganner, A., Gerber, J., Ziegler, A.-K., Li, Y., Kandzia, J., Matulenski, T., et al. (2019). CBP-1/p300 Acetyltransferase Regulates SKN-1/Nrf Cellular Levels, Nuclear Localization, and Activity in C. elegans. Exp. Gerontol. 126, 110690. doi:10.1016/j.exger.2019.110690
Golden, N. L., Plagens, R. N., Kim Guisbert, K. S., and Guisbert, E. (2020). Standardized Methods for Measuring Induction of the Heat Shock Response in Caenorhabditis elegans. J. Vis. Exp. 161. doi:10.3791/61030
Gomez-Pastor, R., Burchfiel, E. T., and Thiele, D. J. (2017). Regulation of Heat Shock Transcription Factors and Their Roles in Physiology and Disease. Nat. Rev. Mol. Cel Biol. 19, 4–19. doi:10.1038/nrm.2017.73
Han, S. K., Lee, D., Lee, H., Kim, D., Son, H. G., Yang, J. S., et al. (2016). OASIS 2: Online Application for Survival Analysis 2 with Features for the Analysis of Maximal Lifespan and Healthspan in Aging Research. Oncotarget 7, 56147–56152. doi:10.18632/oncotarget.11269
Hartl, F. U., Bracher, A., and Hayer-Hartl, M. (2011). Molecular Chaperones in Protein Folding and Proteostasis. Nature 475 (7356), 324–332. doi:10.1038/nature10317
Holmqvist, P.-H., and Mannervik, M. (2013). Genomic Occupancy of the Transcriptional Co-activators P300 and CBP. Transcription 4 (1), 18–23. doi:10.4161/trns.22601
Hsu, A.-L., Murphy, C. T., and Kenyon, C. (2003). Regulation of Aging and Age-Related Disease by DAF-16 and Heat-Shock Factor. Science 300 (5622), 1142–1145. doi:10.1126/science.1083701
Jolly, C., Usson, Y., and Morimoto, R. I. (1999). Rapid and Reversible Relocalization of Heat Shock Factor 1 within Seconds to Nuclear Stress Granules. Proc. Natl. Acad. Sci. U.S.A. 96 (12), 6769–6774. doi:10.1073/pnas.96.12.6769
Jolly, C., Metz, A., Govin, J., Vigneron, M., Turner, B. M., Khochbin, S., et al. (2004). Stress-induced Transcription of Satellite III Repeats. J. Cel. Biol. 164 (1), 25–33. doi:10.1083/jcb.200306104
Joutsen, J., and Sistonen, L. (2019). Tailoring of Proteostasis Networks with Heat Shock Factors. Cold Spring Harb. Perspect. Biol. 11 (4), a034066. doi:10.1101/cshperspect.a034066
Kamath, R. S., Fraser, A. G., Dong, Y., Poulin, G., Durbin, R., Gotta, M., et al. (2003). Systematic Functional Analysis of the Caenorhabditis elegans Genome Using RNAi. Nature 421 (6920), 231–237. doi:10.1038/nature01278
Kumari, N., Hassan, M. A., Lu, X., Roeder, R. G., and Biswas, D. (2019). AFF1 Acetylation by P300 Temporally Inhibits Transcription during Genotoxic Stress Response. Proc. Natl. Acad. Sci. U.S.A. 116 (44), 22140–22151. doi:10.1073/pnas.1907097116
Labbadia, J., and Morimoto, R. I. (2015). The Biology of Proteostasis in Aging and Disease. Annu. Rev. Biochem. 84, 435–464. doi:10.1146/annurev-biochem-060614-033955
Li, J., Chauve, L., Phelps, G., Brielmann, R. M., and Morimoto, R. I. (2016). E2F Coregulates an Essential HSF Developmental Program that Is Distinct from the Heat-Shock Response. Genes Dev. 30 (18), 2062–2075. doi:10.1101/gad.283317.116
Li, T. Y., Sleiman, M. B., Li, H., Gao, A. W., Mottis, A., Bachmann, A. M., et al. (2021). The Transcriptional Coactivator CBP/p300 Is an Evolutionarily Conserved Node that Promotes Longevity in Response to Mitochondrial Stress. Nat. Aging 1 (2), 165–178. doi:10.1038/s43587-020-00025-z
López-Otín, C., Blasco, M. A., Partridge, L., Serrano, M., and Kroemer, G. (2013). The Hallmarks of Aging. Cell 153 (6), 1194–1217. doi:10.1016/j.cell.2013.05.039
Morley, J. F., and Morimoto, R. I. (2004). Regulation of Longevity inCaenorhabditis Elegansby Heat Shock Factor and Molecular Chaperones. MBoC 15 (2), 657–664. doi:10.1091/mbc.e03-07-0532
Morton, E. A., and Lamitina, T. (2013). Caenorhabditis elegans HSF-1 is an Essential Nuclear Protein that Forms Stress Granule-like Structures Following Heat Shock. Aging Cell 12 (1), 112–120. doi:10.1111/acel.12024
Nasrin, N., Ogg, S., Cahill, C. M., Biggs, W., Nui, S., Dore, J., et al. (2000). DAF-16 Recruits the CREB-Binding Protein Coactivator Complex to the Insulin-like Growth Factor Binding Protein 1 Promoter in HepG2 Cells. Proc. Natl. Acad. Sci. U.S.A. 97 (19), 10412–10417. doi:10.1073/pnas.190326997
Powers, E. T., Morimoto, R. I., Dillin, A., Kelly, J. W., and Balch, W. E. (2009). Biological and Chemical Approaches to Diseases of Proteostasis Deficiency. Annu. Rev. Biochem. 78, 959–991. doi:10.1146/annurev.biochem.052308.114844
Raychaudhuri, S., Loew, C., Körner, R., Pinkert, S., Theis, M., Hayer-Hartl, M., et al. (2014). Interplay of Acetyltransferase EP300 and the Proteasome System in Regulating Heat Shock Transcription Factor 1. Cell 156 (5), 975–985. doi:10.1016/j.cell.2014.01.055
Sheikh, B. N., and Akhtar, A. (2019). The many Lives of KATs - Detectors, Integrators and Modulators of the Cellular Environment. Nat. Rev. Genet. 20 (1), 7–23. doi:10.1038/s41576-018-0072-4
Shi, Y., and Mello, C. (1998). A CBP/p300 Homolog Specifies Multiple Differentiation Pathways in Caenorhabditis elegans. Genes Dev. 12 (7), 943–955. doi:10.1101/gad.12.7.943
Victor, M., Bei, Y., Gay, F., Calvo, D., Mello, C., and Shi, Y. (2002). HAT Activity Is Essential for CBP‐1‐dependent Transcription and Differentiation inCaenorhabditis Elegans. EMBO Rep. 3 (1), 50–55. doi:10.1093/embo-reports/kvf006
Vora, M., Shah, M., Ostafi, S., Onken, B., Xue, J., Ni, J. Z., et al. (2013). Deletion of microRNA-80 Activates Dietary Restriction to Extend C. elegans Healthspan and Lifespan. Plos Genet. 9 (8), e1003737. doi:10.1371/journal.pgen.1003737
Westerheide, S. D., Anckar, J., Stevens, S. M., Sistonen, L., and Morimoto, R. I. (2009). Stress-inducible Regulation of Heat Shock Factor 1 by the Deacetylase SIRT1. Science 323 (5917), 1063–1066. doi:10.1126/science.1165946
Westerheide, S. D., Raynes, R., Powell, C., Xue, B., and Uversky, V. N. (2012). HSF Transcription Factor Family, Heat Shock Response, and Protein Intrinsic Disorder. Curr. Protein Pept. Sci. 13 (1), 86–103. doi:10.2174/138920312799277956
Zelin, E., and Freeman, B. C. (2015). Lysine Deacetylases Regulate the Heat Shock Response Including the Age-Associated Impairment of HSF1. J. Mol. Biol. 427 (7), 1644–1654. doi:10.1016/j.jmb.2015.02.010
Zhang, M., Poplawski, M., Yen, K., Cheng, H., Bloss, E., Zhu, X., et al. (2009). Role of CBP and SATB-1 in Aging, Dietary Restriction, and Insulin-like Signaling. Plos Biol. 7 (11), e1000245. doi:10.1371/journal.pbio.1000245
Zhang, H., Shao, S., Zeng, Y., Wang, X., Qin, Y., Ren, Q., et al. (2022). Reversible Phase Separation of HSF1 Is Required for an Acute Transcriptional Response during Heat Shock. Nat. Cel Biol. 24 (3), 340–352. doi:10.1038/s41556-022-00846-7
Zhou, J.-B., Zheng, Y.-L., Zeng, Y.-X., Wang, J.-W., Pei, Z., and Pang, J.-Y. (2018). Marine Derived Xyloketal Derivatives Exhibit Anti-stress and Anti-ageing Effects through HSF Pathway in Caenorhabditis elegans. Eur. J. Med. Chem. 148, 63–72. doi:10.1016/j.ejmech.2018.02.028
Keywords: HSF-1, CBP-1, heat shock response, aging, C. elegans
Citation: Barrett LN and Westerheide SD (2022) The CBP-1/p300 Lysine Acetyltransferase Regulates the Heat Shock Response in C. elegans. Front. Aging 3:861761. doi: 10.3389/fragi.2022.861761
Received: 25 January 2022; Accepted: 01 April 2022;
Published: 27 April 2022.
Edited by:
Cindy Voisine, Northeastern Illinois University, United StatesReviewed by:
Jian Li, Oklahoma Medical Research Foundation, United StatesEric Guisbert, Florida Institute of Technology, United States
Copyright © 2022 Barrett and Westerheide. This is an open-access article distributed under the terms of the Creative Commons Attribution License (CC BY). The use, distribution or reproduction in other forums is permitted, provided the original author(s) and the copyright owner(s) are credited and that the original publication in this journal is cited, in accordance with accepted academic practice. No use, distribution or reproduction is permitted which does not comply with these terms.
*Correspondence: Sandy D. Westerheide, c3dlc3RlcmhlaWRlQHVzZi5lZHU=