- 1Department of Biotechnology, Faculty of Science and Technology, University of Central Punjab, Lahore, Pakistan
- 2National Institute of Biotechnology and Genetic Engineering, Faisalabad, Punjab, Pakistan
Multidrug-resistant organisms are bacteria that are no longer controlled or killed by specific drugs. One of two methods causes bacteria multidrug resistance (MDR); first, these bacteria may disguise multiple cell genes coding for drug resistance to a single treatment on resistance (R) plasmids. Second, increased expression of genes coding for multidrug efflux pumps, which extrude many drugs, can cause MDR. Antibiotic resistance is a big issue since some bacteria may withstand almost all antibiotics. These bacteria can cause serious sickness, making them a public health threat. Methicillin-resistant Staphylococcus aureus (MRSA), vancomycin-resistant Enterococcus (VRE), Multidrug resistant Mycobacterium tuberculosis (TB), and CRE are gut bacteria that resist antibiotics. Antimicrobial resistance is rising worldwide, increasing clinical and community morbidity and mortality. Superbugs have made antibiotic resistance in some environmental niches even harder to control. This study introduces new medicinal plants, gene-editing methods, nanomaterials, and bacterial vaccines that will fight MDR bacteria in the future.
1 Introduction
Antimicrobial resistance poses a critical global health challenge, driven by the emergence of multidrug-resistant organisms, particularly bacteria. These organisms have developed the ability to withstand the effects of antibiotics that were once effective in controlling and killing them. The widespread use of antibiotics in human therapy, agriculture, and aquaculture has contributed to the selection of pathogenic bacteria that are now resistant to multiple drugs (Varela et al., 2021). Bacteria acquire multidrug resistance through two primary mechanisms. First, they can accumulate multiple genes, each conferring resistance to a single drug, within a single cell. This accumulation often takes place on resistance (R) plasmids. Second, multidrug resistance can also occur due to increased expression of genes encoding multidrug efflux pumps, enabling the extrusion of a broad range of drugs (Bush and Bradford, 2019). For instance, Staphylococcus aureus, commonly known as MRSA (Methicillin-Resistant Staphylococcus aureus), and Neisseria gonorrhoeae, the causative agent of gonorrhea are now almost universally resistant to benzylpenicillin, a drug previously effective in controlling these infections (Ahmadi et al., 2022). These bacteria can cause severe diseases and are resistance to nearly all available antibiotics, presenting a significant public health challenge. Examples of these superbugs include methicillin-resistant Staphylococcus aureus (MRSA), vancomycin-resistant Enterococcus (VRE), multidrug-resistant Mycobacterium tuberculosis (TB), and carbapenem-resistant Enterobacteriaceae (CRE) gut bacteria (Mazumdar and Adam, 2021).
The global burden of antimicrobial resistance has led to increased morbidity and mortality in clinical and community settings. The spread of antibiotic resistance to various environmental niches and the emergence of superbugs have complicated effective control strategies. In response to this crisis, international, national, and local approaches have been recommended to control and prevent antimicrobial resistance (El-Halfawy et al., 2020). Key strategies to combat antimicrobial resistance include the rational use of antimicrobials, the regulation of over-the-counter availability of antibiotics, improved hand hygiene, and infection prevention. Addressing this challenge requires a multidisciplinary, collaborative regulatory approach (Liu et al., 2021).
Antimicrobial resistance is now recognized as a global issue by various stakeholders. In 2011, the World Health Organization (WHO) designated it as a major theme, drawing international attention to the need for collective efforts in addressing AMR (Ahmed and Baptiste, 2018). WHO has put forth recommendations that emphasize increased collaboration among governments, non-governmental organizations, professional groups, and international agencies, the establishment of networks for AMR surveillance, international efforts to combat counterfeit antimicrobials, incentives for the development of new drugs and vaccines, and the strengthening of existing programs to contain AMR (Fiore et al., 2019).
This study specifically explores the pathogenic mechanisms of some of the most lethal multidrug-resistant bacteria, including Vancomycin-Resistant Enterococci (VRE), Methicillin-Resistant Staphylococcus aureus (MRSA), Extended-Spectrum β-Lactamase (ESBLs) producing Gram-negative bacteria, Klebsiella pneumoniae carbapenemase (KPC) producing Gram-negative bacteria, and Multidrug-Resistant Gram-Negative Rods (GNR). Researchers are harnessing advancements in science and technology to combat these bacteria using innovative approaches such as newly discovered medicinal plants, gene-editing techniques, novel nanomaterials, and bacterial vaccines. This review provides insights into the mode of action of these techniques in neutralizing bacterial infections, offering valuable guidance for researchers seeking effective strategies to combat multidrug-resistant bacteria in the future. By presenting these critical aspects of antimicrobial resistance in a structured manner, this information aims to enhance reader comprehension and awareness of the global challenge posed by multidrug-resistant organisms.
2 Multi-drug resistant bacteria
2.1 Vancomycin-resistant Enterococci
Enterococci are facultative anaerobes, ovoid-shaped gram-positive bacteria, and commensal inhabitants of the gastrointestinal tract of humans (Fiore et al., 2019). Impulsive applications of antibiotics have prompted the adaptive resistance mechanism in Enterococci and modulated it into an emerging nosocomial pathogen along with urinary tract and bloodstream infections (Said and Abdelmegeed, 2019). The discovery of antibiotics was intended to guarantee defense against pathogenic bacteria, but after some optimistic period, bacteria, for instance, Enterococci, acquired resistance against penicillin and aminoglycosides modified penicillin, streptomycin. Metabolic modifications, hypermutability, and mobile genetic factors in Enterococci acquired genes are involved in the Vancomycin resistance mechanism (Sun et al., 2018). Enterococci have become an opportunistic pathogen with intrinsic and acquired resistance against quinupristin, dalfopristin, tigecycline, and vancomycin antibiotics (Ahmed and Baptiste, 2018).
2.1.1 Pathogenesis of VRE
Enterococci have a diverse resistance mechanism against antibiotic agents, including resistance against antibiotics interfering with cell wall components, protein synthesis, nucleic acid replication, and synthesis (Sianglum et al., 2019). Generally, the Cell wall of Enterococci is synthesized by crosslinking of peptidoglycan’s D-alanine-D-alanine agents, a terminal of pentapeptide precursor. Vancomycin performed its inhibitory activity by binding with the D-alanine-D-alanine of the cell wall and obstructing cell wall synthesis (Turner et al., 2021). However, vancomycin-resistant Enterococci have adapted to bind the vancomycin with D-lactate by altering the pentapeptide precursors of the cell wall. Modification in precursors has reduced the binding affinity of vancomycin to D-alanine by about 1000-fold. The entire mechanism of vancomycin resistance requires seven enzymes: VanH, VanX, VanY, VanZ, VanR, and VanS, taking VanA as a model for altering and eradicating a normal precursor, D-alanine (Figure 1) (Mühlberg et al., 2020).
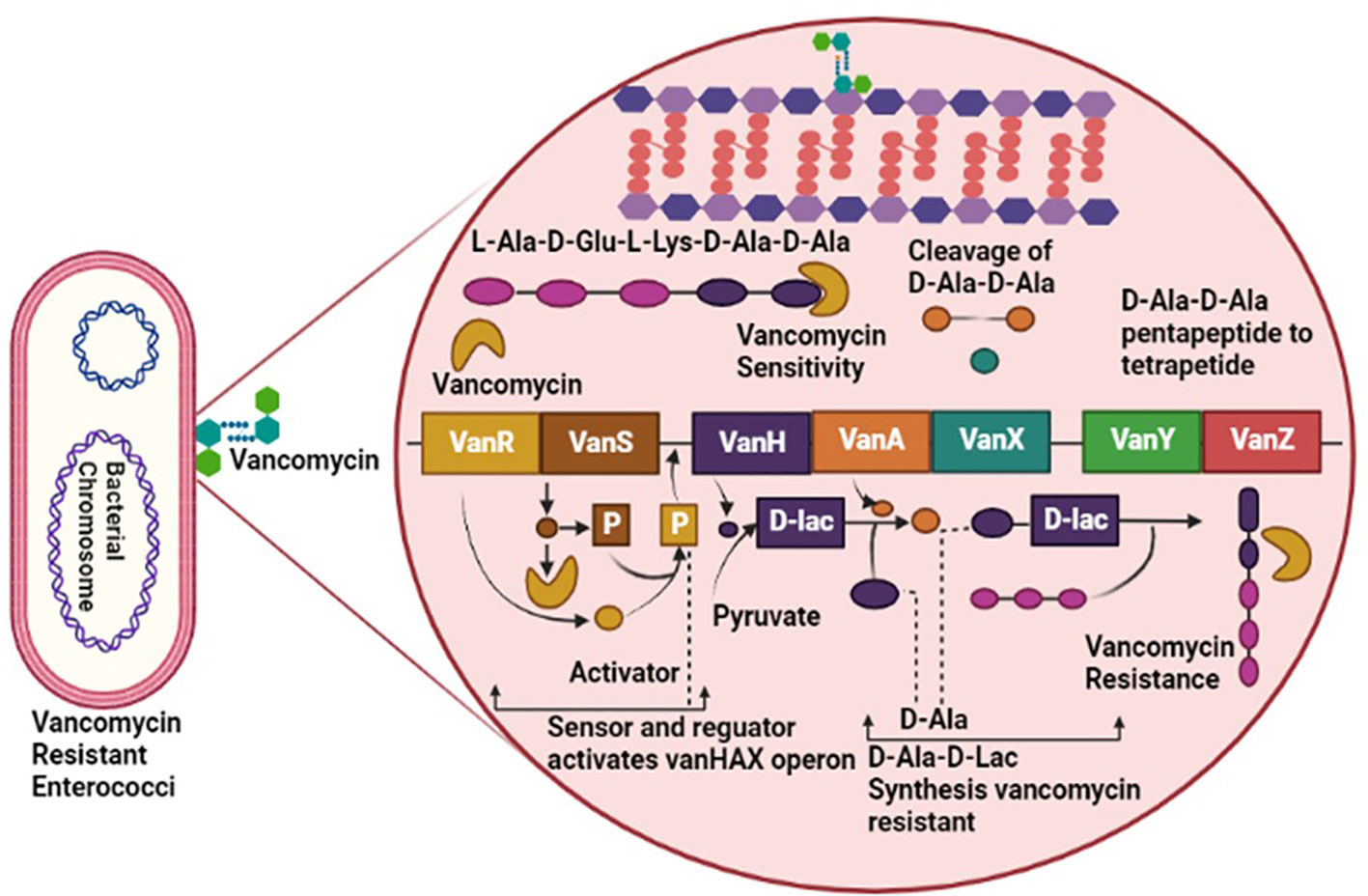
Figure 1 Pathogenesis of Vancomycin-Resistant Enterococci (VRE), involving VanR, VanS, VanH, VanA, VanX, VanY and VanZ virulent factors causing resistance against vancomycin antibiotic.
2.2 Methicillin-resistant Staphylococcus aureus
Staphylococcus aureus is an emerging, facultative gram-positive opportunistic bacteria associated with various community-acquired and nosocomial infections (Waseem et al., 2023). It has also been reported with serious skin infections, pneumonia, and toxic shock syndrome (Faron et al., 2016). Staphylococcus aureus is a versatile pathogen with broad-spectrum hosts from sheep, pigs, and cattle to poultry. Intrinsic and adaptive characteristics of S. aureus have produced resistance and tolerance mechanisms against a range of antibiotics from narrow to broad-spectrum, including Penicillin, Quinolone, Methicillin, and Vancomycin (Gajdács, 2019). This bacterium encodes hydrolyzing enzymes to generate an acquired resistance mechanism against antibiotics and is illustrated as multidrug resistance bacteria (MDR) (Chojnacki et al., 2019).
2.2.1 Pathogenesis of MRSA
Methicillin-resistant Staphylococcus aureus (MRSA), a multi-drug resistant evolving pathogen, has acquired resistance against frequently modified antibiotics. MRSA performed its activity by modifying penicillin-binding proteins (PBP2) (El-Halfawy et al., 2020). Normal PBP2 and bifunctional transglycosylase-transpeptidase are involved in peptidoglycan biosynthesis (cell wall). Here, MRSA produces modified genes that encode PBP2a responsible for the inactivation of transpeptidase (TP) of normal PBP2 and turn off the reach of β-lactams to the serine active site of TP, leading to the non-susceptibility of β-lactams (Dawan and Ahn, 2020).
The Gene involved in the expression of PBP2a is mecA, found in staphylococcal chromosome cassette, regulated by MecIR proteins. These proteins MecIR regulate the expression of the mecA gene for the production of PBP2a on exposure to β-lactams. B-lactams have inherited abilities to counter mecA expression by activating β-lactamase regulators BlaI and BlaR (Mazumdar and Adam, 2021). The resistance mechanism of MRSA could be heterogeneous. Most cells express low resistance and could be homogenous, in which most cells show high resistance but are in the minority. Chromosomal alterations can improve the regulation of the mecA gene to produce additional PBP2a for a high homogenous resistance mechanism in MRSA. Introducing new β-lactams, including fifth-generation cephalosporins and ceftobiprole, may inhibit the PBP2a of methicillin-resistant staphylococcus aureus (Figure 2) (El-Halfawy et al., 2020).
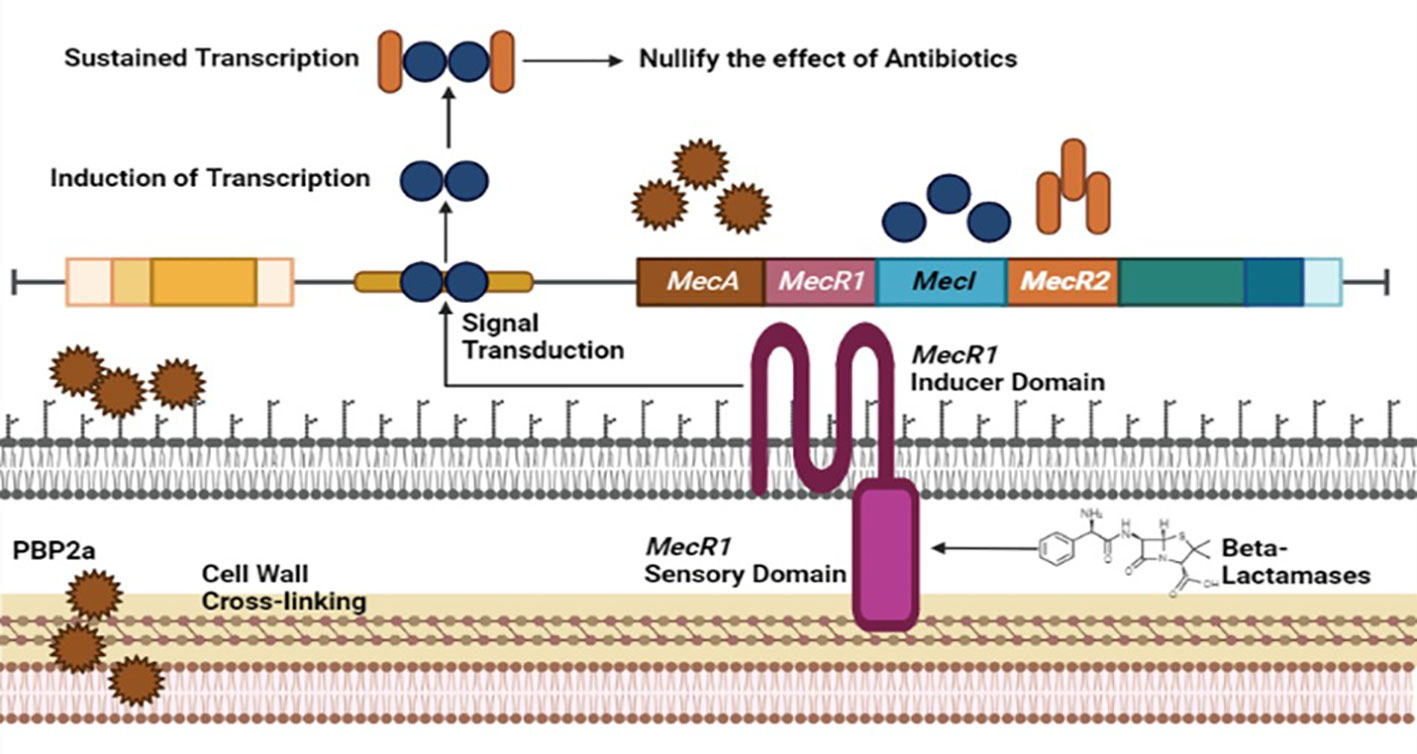
Figure 2 Resistance mechanism of Staphylococcus aureus against Methicilin antibiotic involving MecA, MecR1, MecI and MecR2 genome factors of MRSA.
2.3 Extended-spectrum β-lactamase producing Gram-negative bacteria
Multi-drug resistance in bacteria, facilitated by factors like uncooked food, contact with animals, and antibiotic misuse, poses a global threat to combating microbial infections (Fisher and Mobashery, 2020). Antibiotics are potentially significant in preventing the spread of communicable diseases globally (Khan et al., 2020). Gram-negative bacteria like Escherichia coli, Pseudomonas aeruginosa, Klebsiella pneumoniae, and Acinetobacter spp. are responsible for various infections. They produce β-lactamase enzymes, a major cause of resistance, which can hydrolyze β-lactam antibiotics (Hernando-Amado et al., 2019; Liu et al., 2019). This is a serious threat to underdeveloped countries with less life-saving and diagnostic surveillance having a high burden of microbial infections. Gram-negative bacteria such as Escherichia coli, Pseudomonas aeruginosa, Klebsiella pneumoniae, and Acinetobacter spp. are pathogenic microbes which are responsible for causing abdominal, blood and urinary tract infections (Monserrat-Martinez et al., 2019).
These gram-negative bacteria contain a β-lactamase enzyme that hydrolyzes an integral part of the ring of β-lactam antibiotics. It is a major factor that develops beta-lactam resistance (Portsmouth et al., 2018). Extended spectrum β-lactamases (ESBLs) are a diverse group of enzymes that can hydrolyze penicillin and cephalosporins but not carbapenems and cephamycins. This resistance is a significant concern, especially in underdeveloped countries with limited healthcare resources (Davies and Everett, 2021). These Extended spectrum β-lactamases are inhibited by tazobactam, sulbactam and clavulanic acid (Bush and Bradford, 2019). The most common among the four classes of β-lactamase (A, B, C and D) are CTX-M, TEM, and SHV families (Ali et al., 2018).
2.3.1 Pathogenesis of ESBLs
ESBL production is prevalent in Klebsiella pneumonia and Escherichia coli strains, with over 400 known types of β-lactamases. Class A β-lactamases, like those in the TEM and SHV families, can hydrolyze various antibiotics but are inhibited by clavulanic acid, carbapenems, and cephamycins (Bush, 2018; Hammoudi Halat and Ayoub Moubareck, 2020; Yang et al., 2020; Ejaz et al., 2021). However, these enzymes can be inhibited by using clavulanic acid or by treating the microorganism with carbapenems (imipenem) and cephamycins (cefoxitin) (Ramos et al., 2020). CTX-M β-lactamases are efficient against certain antibiotics and have various subtypes CTX-M, CTX-M-2, CTX-M-3 and CTX-M-14 (Carcione et al., 2021; Castanheira et al., 2021).
Chromosomes of some bacteria and various Enterobacteriaceae encode for class C AmpC β-lactamases. They favor the bacteria by developing resistance against most penicillins, cephalothin, cefoxitin, cefazolin, and different β-lactamase inhibitors (Adel et al., 2021; Farhat et al., 2022). Overexpression of AmpC enzymes leads to cephalosporin resistance in a broad spectrum, for example, ceftriaxone, ceftazidime, and cefotaxime. AmpC-producing bacteria can be treated effectively using Carbapenems, but in some studies, resistance against carbapenem in the microbes has also been reported (Meini et al., 2019; Ali et al., 2020).
OXA-type class D β-lactamases can hydrolyze carbapenems and are plasmid-encoded. Some bacteria, including Pseudomonas aeruginosa, produce uncommon ESBLs like GES, PER, VEB, and IBC β-lactamases (Agyepong, 2017; Govindaswamy et al., 2019). The plasmid of bacteria, mainly P. aeruginosa, also produces uncommon ESBLs such as GES, PER, VEB, and IBC β-lactamases (Sawa et al., 2020). Class B MBLs can become carbapenemases and are transferable among bacteria (Khoshbakht et al., 2014). Figure 3 illustrates the production of ESBLs and the inhibition of β-lactam ring-containing antibiotics by gram-negative bacteria.
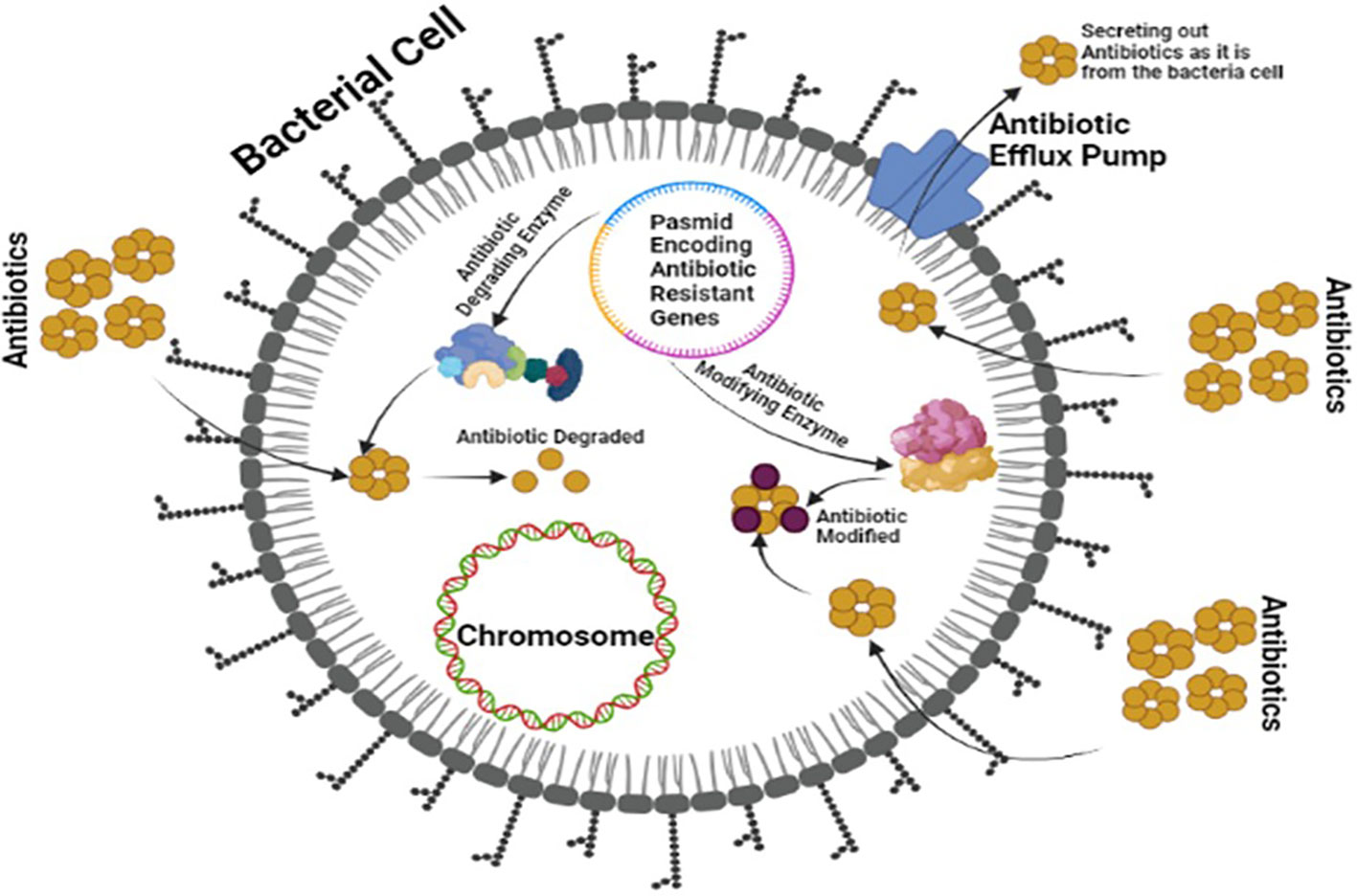
Figure 3 Multi-Drug Resistant Mechanism of ESBLs showing a plasmid encoding antibiotic resistance and modifying enzymes by bacteria against board spectrum antibiotics.
2.4 Klebsiella pneumoniae carbapenemase producing Gram-negatives
Gram-negative bacteria are rapidly becoming resistant to carbapenem worldwide. Specifically, these carbapenemase-producing bacteria mainly originate from healthcare centers. Klebsiella pneumoniae carbapenemase (KPC)-producing Klebsiella pneumonia (KPC-KP) is a widely reported strain causing carbapenem resistance (Berglund et al., 2017). As a result of the rapid increase in resistance against cephalosporin antibiotics within Enterobacteriaceae, some drugs have become commonly to be used worldwide, such as biapenem, meropenem, ertapenem, imipenem, doripenem and panipenem. Carbapenem antibiotics initiate bactericidal activity by binding irreversibly with an amino acid Ser403 present within the active site of the penicillin-binding protein of bacteria (Hussain et al., 2021). This inhibits the bacterial cell wall synthesis, resulting in the bacterial cell being killed. The blaKPC gene responsible for carbapenem resistance is present on mobile genetic elements, facilitating the widespread of carbapenem resistance among intra and interspecies (Mora-Ochomogo and Lohans, 2021). Similarly, the extended-spectrum β-lactamases (ESBLs) and KPCs are efficient enough to hydrolyze β-lactam rings in monobactams, cephalosporins, and penicillins. In vitro studies suggest that KPC enzymes have the highest affinity for ertapenem antimicrobial (Caprari, 2021).
2.4.1 Pathogenesis of antibiotic resistance in carbapenem-resistant Enterobacteriaceae
Carbapenem resistance in Enterobacteriaceae is primarily caused by three mechanisms: carbapenem degradation using the enzyme Carbapenemase, activation of efflux pumps, and alterations in porin proteins (Bianco et al., 2021). Carbapenemase production, along with β-lactamases and porin mutations, leads to resistance. KPC enzymes play a significant role in this resistance, with different subtypes available (Pulzova et al., 2017; Chauzy et al., 2019; Brindangnanam et al., 2022). Bacteria can also use other mechanisms, like efflux pump activation and changes in drug-binding proteins, to resist carbapenems. Plasmid-encoded carbapenemases facilitate resistance spread among bacteria (Seifert et al., 2018; Palacios et al., 2020). In Pseudomonas aeruginosa, efflux pump overexpression and porin loss contribute to carbapenem resistance. Gram-negative bacteria are generally more resistant due to their cell wall, reduced permeability, efflux pumps, and broad-spectrum β-lactamases (Galani et al., 2021; Zhang et al., 2022). Some traditional treatments may be ineffective, highlighting the need for new antimicrobials to combat these resistant infections (Thaden et al., 2017). The pathogenesis of carbapenem-resistant Enterobacteriaceae, which results in multi-drug resistance against carbapenem antibiotics, is depicted in Figure 4.
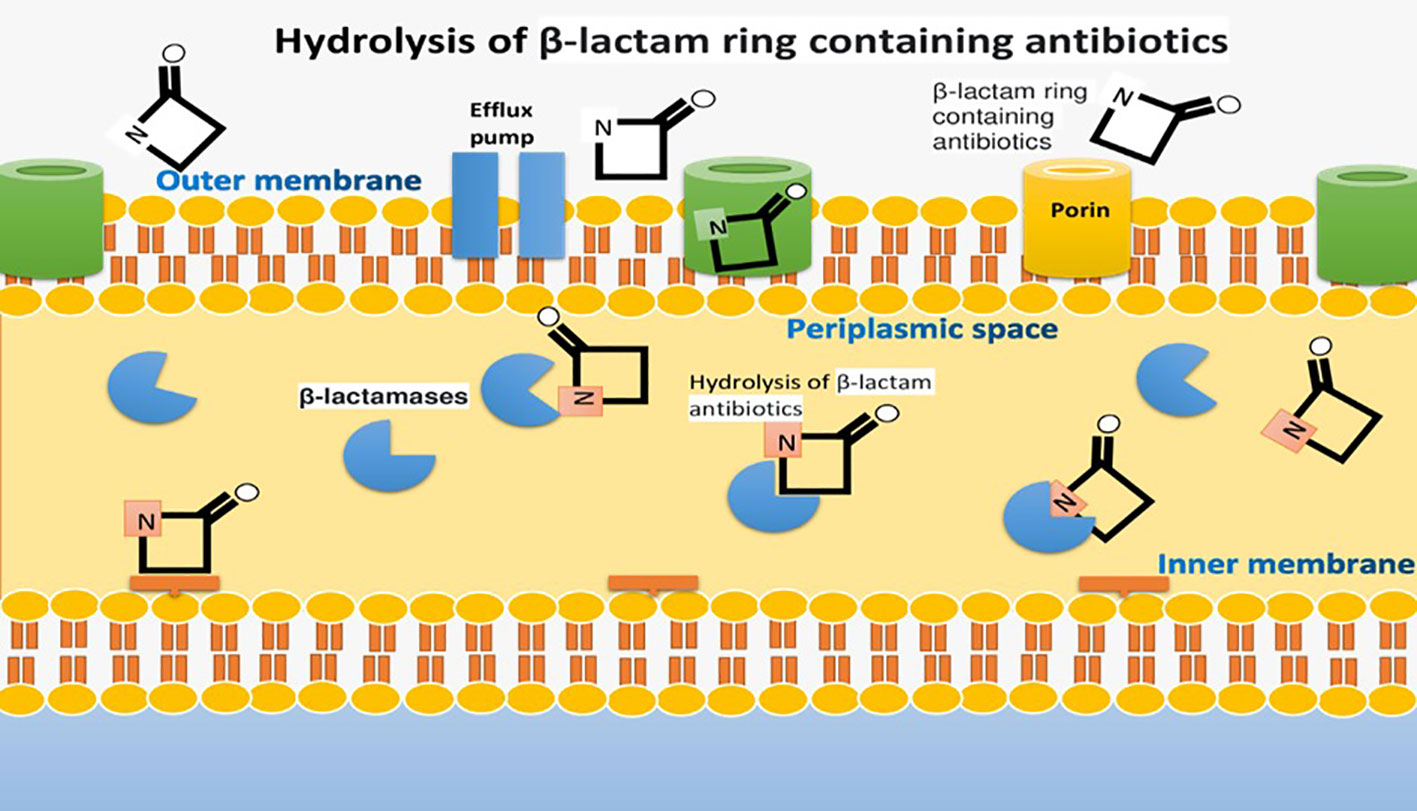
Figure 4 Pathogenesis of Carbapenem-Resistant Enterobacteriaceae causing multi-drug resistance against Carbapenem Antibiotics.
2.5 Multidrug-resistant Gram-negative rods
Gram-negative rod-shaped bacteria, including Escherichia coli, Acinetobacter baumannii, Pseudomonas aeruginosa, and Enterobacteriaceae, are inhabitants of human skin and the GI tracts of humans, animals, sewage, and soil. These are particularly involved in urinary tract infections, community-acquired and nosocomial infections (Zrelovs et al., 2021). Gram-negative bacteria have developed numerous resistance mechanisms against multiple antibiotics owing to genetic variations and inherent and acquired characteristics. Contrary to Gram-positive bacteria, Gram-negative bacteria have a thick outer membrane having porins protecting them from antibiotics by producing antibiotic resistance. Gram-negative bacteria resist several antibiotics, including Carbapenems, piperacillin, ciprofloxacin, broad-spectrum penicillins, and aminoglycosides (Wolfensberger et al., 2019).
2.5.1 Pathogenesis of Multidrug resistant GNR
Gram-negative bacteria exhibit a range of resistance mechanisms; for instance, E. coli is a commensal bacterium of the GI tract that produces virulence factors causing sepsis, abdominal infections, and bacteremia (Kabanangi et al., 2021). MDR E.coli has adapted to extended-spectrum β-lactam producing β-lactamase (ESBL), hydrolyzing the β-lactam antibiotics. Further, MDR E.coli ST131 producing ESBL causing urinary tract infections shows resistance to third-generation cephalosporins and quinolones. Similar to Gram-negative Pseudomonas aeruginosa and Enterobacteriaceae, ESBL and Carbapenemase-producing Enterobacteriaceae (CPE) production by these gram-negative bacteria resist the antibiotic activities of carbapenems, aztreonam, and cephalosporin.
The general mechanism of extended-spectrum beta-lactamase production is diverse, and different strategies are applied (Eichenberger and Thaden, 2019). For instance, hydrolysis of ester-amides bond in antibiotics like cephalosporins, redox reaction of tetracycline by TetX enzyme activity and transferases enzymes inhibit the antibiotic activity by switching it with the acetyl or phosphoryl groups. The thick outer membrane of Pseudomonas aeruginosa and other gram-negative rods protects them from antibiotic activity. Porins in the outer membrane impede antibiotic activity by reducing their numbers or modifications and adaptations, resulting in porins loss and thereby hindering antibiotic activity (Figure 5) (Jean et al., 2022).
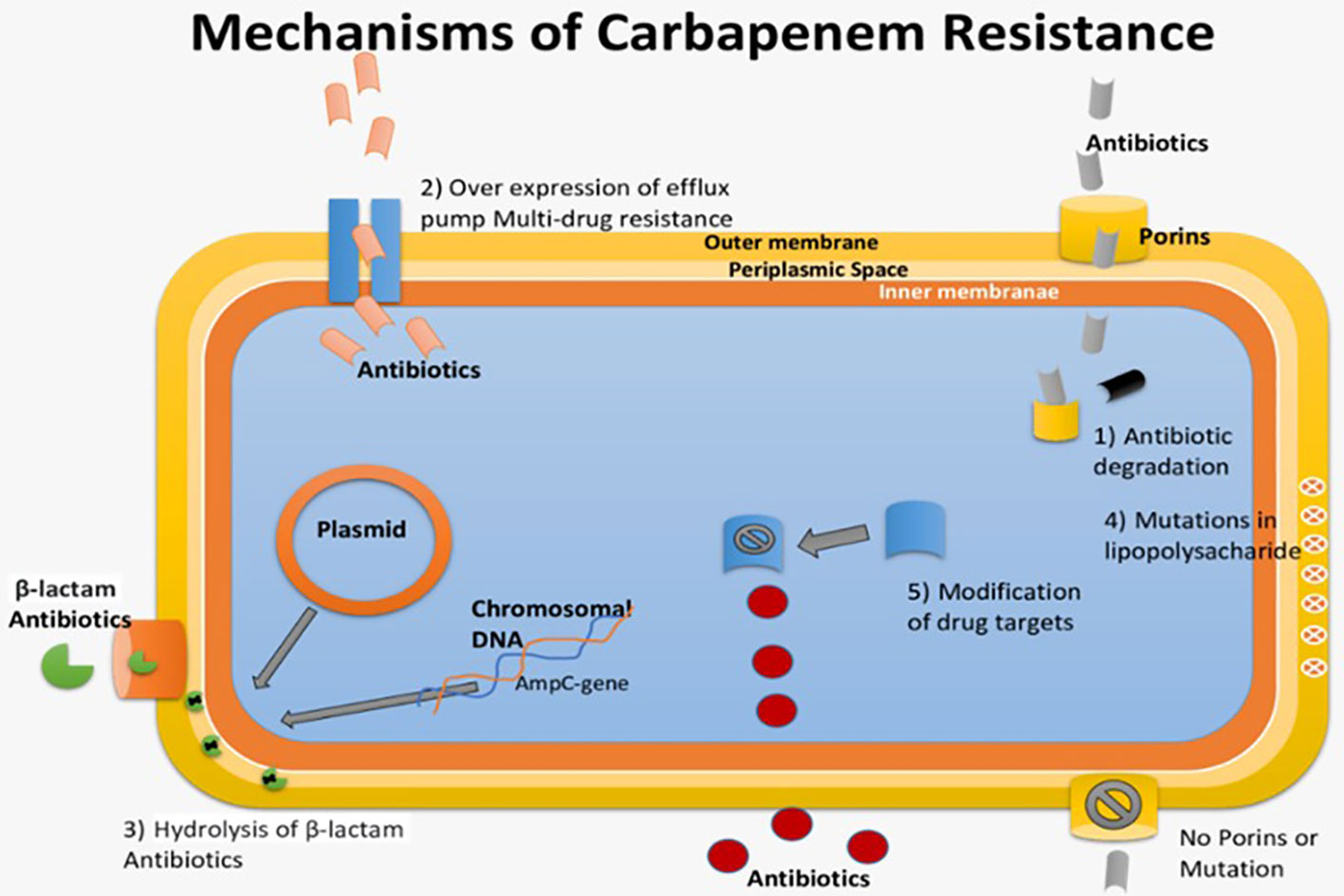
Figure 5 Pathogenesis of Multi-drug resistant GNR causing resistance against antibiotics by gram-positive bacterial species.
3 Advancements to combat multi-drug-resistant bacteria
Microbes possess the ability to neutralize antimicrobial activity through various molecular mechanisms. The antimicrobial agent loses its efficacy as it is deactivated by specific proteins produced by the bacterium. For example, β-lactam antibiotics are hydrolyzed by β-lactamases, with enterobacter spp. producing extended-spectrum β-lactamases (ESBL) that share the same inactivating capability, making them challenging to eliminate (Davies and Everett). Additionally, various enzymes, such as acetyltransferase, phosphotransferase, and adenyltransferase, can deactivate specific antibiotics. In the case of resistance to erythromycin, methylation of an adenine residue in the peptidyltransferase of rRNA 23S reduces its affinity for the antibiotic without affecting protein synthesis. Another notable example is the modification of penicillin-binding proteins (PBPs) by MRSA (Anstey et al., 2019).
To combat these microbial strategies, there is a pressing need for advancements in modern techniques. The development and implementation of cutting-edge technologies are crucial in overcoming microbial resistance. Novel approaches, including gene-editing techniques, innovative nanomaterials, and bacterial vaccines, show promise in the fight against antimicrobial resistance. Moreover, a focus on antibiotic stewardship programs is essential. These programs play a pivotal role in promoting responsible antibiotic use, ensuring judicious prescribing, and mitigating the emergence of resistant strains. Following we discussed some advancements in technology, addressing the ever-evolving challenges posed by microbial resistance to antimicrobial agents.
3.1 Fifth generation antibiotics
Fifth-generation cephalosporins are a class of antibiotics that belong to the cephalosporin family. They are part of the beta-lactam group of antibiotics and have a broad spectrum of activity against various types of bacteria (Rusu and Lungu, 2020). Fifth-generation cephalosporins are among the newest cephalosporins, and they have been developed to address antibiotic resistance and target a wide range of both Gram-positive and Gram-negative bacteria. Fifth-generation cephalosporins are generally effective against a variety of pathogens, including those resistant to earlier generations of cephalosporins and some other classes of antibiotics (Lin and Kück, 2022). They are valuable additions to the armamentarium of antibiotics available to healthcare providers and are particularly useful in the treatment of complicated infections caused by a range of bacteria (Fong, 2023).
3.1.1 Mode of action of antibiotics
Modifications in the host cell surface pose a challenge to the effective penetration of antimicrobials. In Gram-negative bacteria, resistance may arise from alterations in porins or proteins, either through modification or quantitative reduction, which are essential for the entry of many antimicrobial drugs (Dowling et al., 2017). These modifications can impede the incoming flow of various antibiotics by imposing restrictions based on factors such as atomic size, hydrophobicity, and charge, thereby contributing to the intrinsic resistance of numerous microorganisms. An illustrative case of Pseudomonas aeruginosa, showcasing resistance to imipenem due to such surface modifications in Figure 6 (Farhat et al., 2020; Shukla et al., 2020).
Ceftaroline, a cephalosporin antibiotic belonging to the fifth generation, has demonstrated exceptional efficacy in inhibiting the activity of PBP2a, or penicillin-binding protein 2a. This protein plays a pivotal role in conferring resistance to beta-lactam antibiotics in methicillin-resistant Staphylococcus aureus (MRSA) and related Gram-positive bacteria. Ceftaroline’s effectiveness stems from its unique structural features and its ability to overcome resistance mechanisms associated with PBP2a (Dafne et al., 2019). Notably, ceftaroline’s success lies in its structural similarity to the D-Ala-D-Ala terminus of the peptidoglycan pentapeptide chain, serving as the natural substrate for PBPs. The beta-lactam ring of ceftaroline is crucial for its mechanism of action, enabling the antibiotic to mimic the peptidoglycan structure and facilitating its binding to the active site of PBP2a (Zhanel et al., 2009).
Upon binding, ceftaroline forms a stable acyl-enzyme complex with PBP2a, distinguishing it from other beta-lactam antibiotics. This stability is attributed to ceftaroline’s unique structural elements, including a 7α-methoxy group and a 3’ amino group. The resulting stable complex effectively inhibits PBP2a, preventing the enzyme from participating in the cross-linking of the peptidoglycan cell wall. This disruption in peptidoglycan synthesis weakens the bacterial cell wall, leading to cell lysis and the eventual demise of MRSA and susceptible Gram-positive bacteria. Crucially, ceftaroline’s ability to overcome PBP2a-mediated resistance makes it a valuable treatment option for infections caused by MRSA and other resistant Gram-positive bacteria (Morosini et al., 2019). In the ongoing battle against antibiotic-resistant microbes, the development and utilization of fifth-generation antibiotics offer a promising avenue for effective treatment. Continued research and innovation in this field are essential to stay ahead of evolving resistance mechanisms and ensure the availability of potent tools to combat challenging microbial infections.
3.2 Medicinal plants
From ancient times to the modern era, a plethora of plants, herbs, and shrubs have been harnessed for their therapeutic properties in treating diseases and infections caused by pathogens. These botanical wonders exhibit medicinal and antimicrobial attributes, containing phytochemicals endowed with antioxidant, anti-inflammatory, and antibiotic activities. In the face of escalating bacterial resistance to conventional drugs, a phenomenon known as multidrug resistance, the exploration of alternative sources becomes imperative. Six Cameroonian medicinal plants, namely Erigeron floribundus, Nauclea latifolia, Caucalis melanantha, Anthocleista schweinfurthii, Zehneria scobra, and Boehmeria platyphylla have been investigated for their extracts’ efficacy against multidrug-resistant Gram-positive bacteria. Extracts from these plants exhibited a moderate level of antibiotic activity, with 14.3% of 28 bacterial strains demonstrating minimum inhibitory concentration (MIC) values ranging from 128 to 1024 μg/mL (Djeussi et al., 2016). Beyond antibacterial applications, Cameroonian plants have also demonstrated promise in cancer treatment, exerting cytotoxic effects on cancerous cell lines when phytochemicals from fifteen medicinal plants were applied (Kuete et al., 2016).
In a study, Artemisia annua (EtAa, AqAa), Oxalis corniculata (EtOc, AqOc), and A. annua essential oil (EoAa), along with aqueous and ethanolic extracts, showcased antimicrobial activity. This study focused on multidrug resistance in Escherichia coli by determining the minimum bactericidal concentration (MBC) and MIC. Impressively, these extracts inhibited all isolates in 56.7% of cases, presenting a potential alternative to the 13 antibiotics currently employed against EoAa (Golbarg and Mehdipour Moghaddam, 2021). Tuberculosis (TB), a persistent global health concern, has been addressed through the utilization of fifty-two plant species, with Chenopodium ambrosiodes L emerging as the most cited. The historical evolution of TB treatment has witnessed a transition from single-drug therapies to the current approach of multidrug resistance, signifying the adaptation of strategies against the growing drug resistance in the context of tuberculosis (Ahmad et al., 2006; Organization WH, 2013; Nguyen, 2016).
3.2.1 Mode of action of medicinal plants
Medicinal plants harbor phytochemicals with the remarkable ability to suppress and eliminate bacteria through diverse mechanisms. These bioactive compounds target bacterial cells by either breaking down the cell wall or rupturing the cell membrane (Yadav et al., 2023). The process is particularly effective against multidrug-resistant strains, where the interaction with ion channel carrier proteins, specifically efflux pumps, plays a crucial role in acquiring resistance. Upon removal of the cell wall, the bacterial cell becomes vulnerable and succumbs to its demise. Similarly, the rupture of the cell wall exposes the bacterial cell components, leading to the eventual demise of the bacteria (Jorgensen et al., 2017). The intricate mechanism of ion channel efflux, integral to the functionality of resistant drugs, is disrupted by these phytochemicals, rendering them potent agents in combating antibiotic-resistant bacteria (Khameneh et al., 2021). Subsequently, these extracted and refined phytochemicals find application as drugs in medicine, proving effective in suppressing or eradicating bacterial infections (Escherichia coli, Staphylococcus aureus, and Mycobacterium tuberculosis) and offering a promising avenue for addressing antibiotic resistance in a multifaceted manner.
3.3 Bacteriocins
Bacteriocins, bacterial ribosomal products, are antimicrobial peptides that serve as a defense mechanism against pathogenic and deteriorating bacteria. These low molecular weight peptides exhibit diverse inhibitory mechanisms against other bacteria, categorizing them into narrow-spectrum bacteriocins, which hinder the growth of bacteria within the same species, and broad-spectrum bacteriocins, which impede bacteria belonging to different species (Negash and Tsehai, 2020). Both Gram-positive and Gram-negative bacteria produce these antimicrobial peptides. The bacterial cells generating bacteriocins possess a specific immune system to resist their own products. Bacteriocins are encoded by a cluster of genes responsible for their immunity, transport, and production. These genes are typically located adjacently on plasmids or chromosomes, expressing in parallel fashion (O’Connor et al., 2020).
The antimicrobial activity of bacteriocins extends to various bacteria, including food-borne pathogens, making them highly applicable in food preservation. An exemplary case is Nisin, an FDA-approved bacteriocin utilized as a food preservative in over 50 countries worldwide, inhibiting the growth of Listeria and numerous other Gram-positive microbes. The potential of bacteriocins to combat antibiotic-resistant bacteria underscores their importance in clinical applications. For instance, griselimycin, a bacteriocin, demonstrates inhibitory effects against Mycobacterium tuberculosis in vitro and in mice, offering promise as a treatment for multidrug-resistant strains. Furthermore, bacteriocins exhibit efficacy against diverse viruses, such as dengue viruses and HIV (Yang et al., 2014).
3.3.1 Mode of action of bacteriocin
The action of bacteriocins initiates through their interaction with the cell membrane of bacteria. Given the negatively charged nature of the cell membrane, electrostatic interactions occur, leading to both specific and non-specific binding. This interaction facilitates the formation of pores, enabling the insertion of the bacteriocin into the target cell. Subsequently, these pores induce a continuous efflux of cellular components, including ions, amino acids, and ATP, resulting in the leakage of all cellular content and ultimately leading to cell death. Furthermore, this mechanism may induce cell lysis by releasing autolytic enzymes associated with the cell wall lipids of Gram-positive bacteria (van Heel et al., 2017). In certain scenarios, bacteriocins may not exclusively interact with the cell membrane; instead, they can directly interfere with the nucleic acid synthesis mechanism shown in Figure 7 (Acedo et al., 2015).
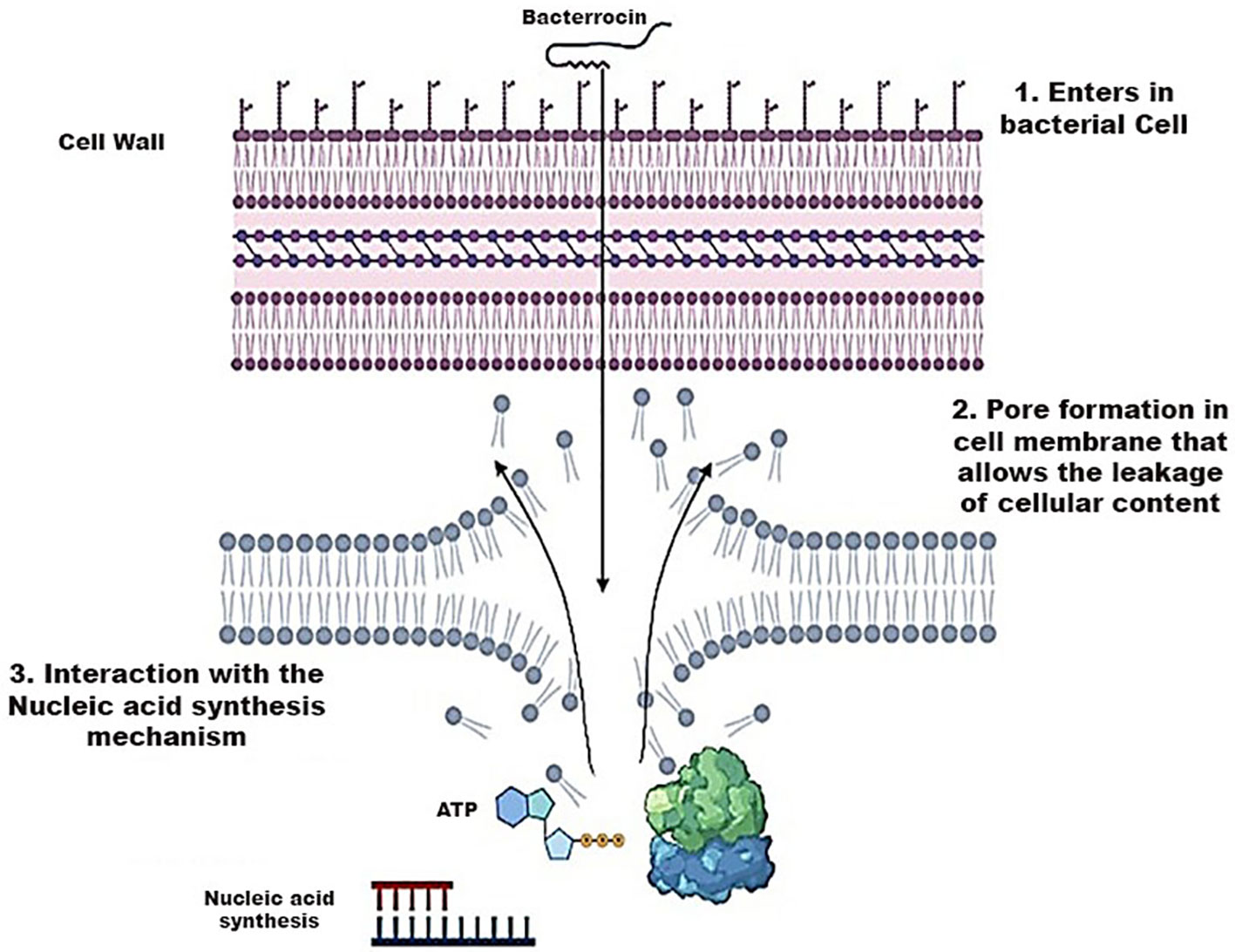
Figure 7 Mechanism of Action of Bacteriocins playing a role in the destruction of bacterial cell wall.
3.4 Bacterial vaccines
Bacterial vaccines, comprised of antigens either synthetically produced or derived from pathogens, play a crucial role in eliciting an immune response within the body. Upon entry, these vaccines stimulate the immune system to generate antibodies specific to the targeted pathogen, thereby preventing its growth upon future exposure. Notably, bacterial vaccines exhibit an extended lifespan, spanning decades, and exert a profound impact on human health (Poolman, 2020). The probability of resistance emergence against these vaccines within the genome is remarkably low. This phenomenon can be attributed to the concise timing of vaccine administration relative to the pathogen’s lifecycle and the diversity and variability of target sites. The latter necessitates a high rate of mutations for the pathogen to acquire resistance against the vaccine. Bacterial vaccines contribute significantly to reducing mortality and illness associated with bacterial diseases, standing as one of the most efficient medical interventions (Bekeredjian-Ding, 2020).
3.4.1 Mode of Action of bacterial vaccines
Upon the entry of a pathogen into the body, antigen-presenting cells undertake the crucial task of processing and presenting these invaders to both B and T cells. The CD4+ T helper cells play a pivotal role by providing growth factors and signals to activate the B and T cells. Subsequently, these activated cells, recognizing the antigens, undergo proliferation, generating effector cells and memory cells. The effector cells, armed with the ability to recognize and target specific antigens, take action in various ways. B cells release antibodies against the identified pathogens, while Cytotoxic T cells execute the targeted destruction of the antigens (Mayer and Impens, 2021). The memory cells, on the other hand, store vital information for sustained immunity against the encountered pathogen and persist longer in diverse host tissues. These memory cells assume responsibility for mounting secondary responses upon reencountering specific pathogens. In the context of vaccines and antigens, the immune response typically engages both B and T cells. When a vaccine contains an antigen, plasma cells are stimulated to produce antibodies that specifically bind to toxins or pathogens. Simultaneously, CD8+ T cells come into play, restricting and diminishing the spread of infection. Figure 8 is a pictorial representation of mode of actions of antibacterial vaccines (Bidmos et al., 2018).
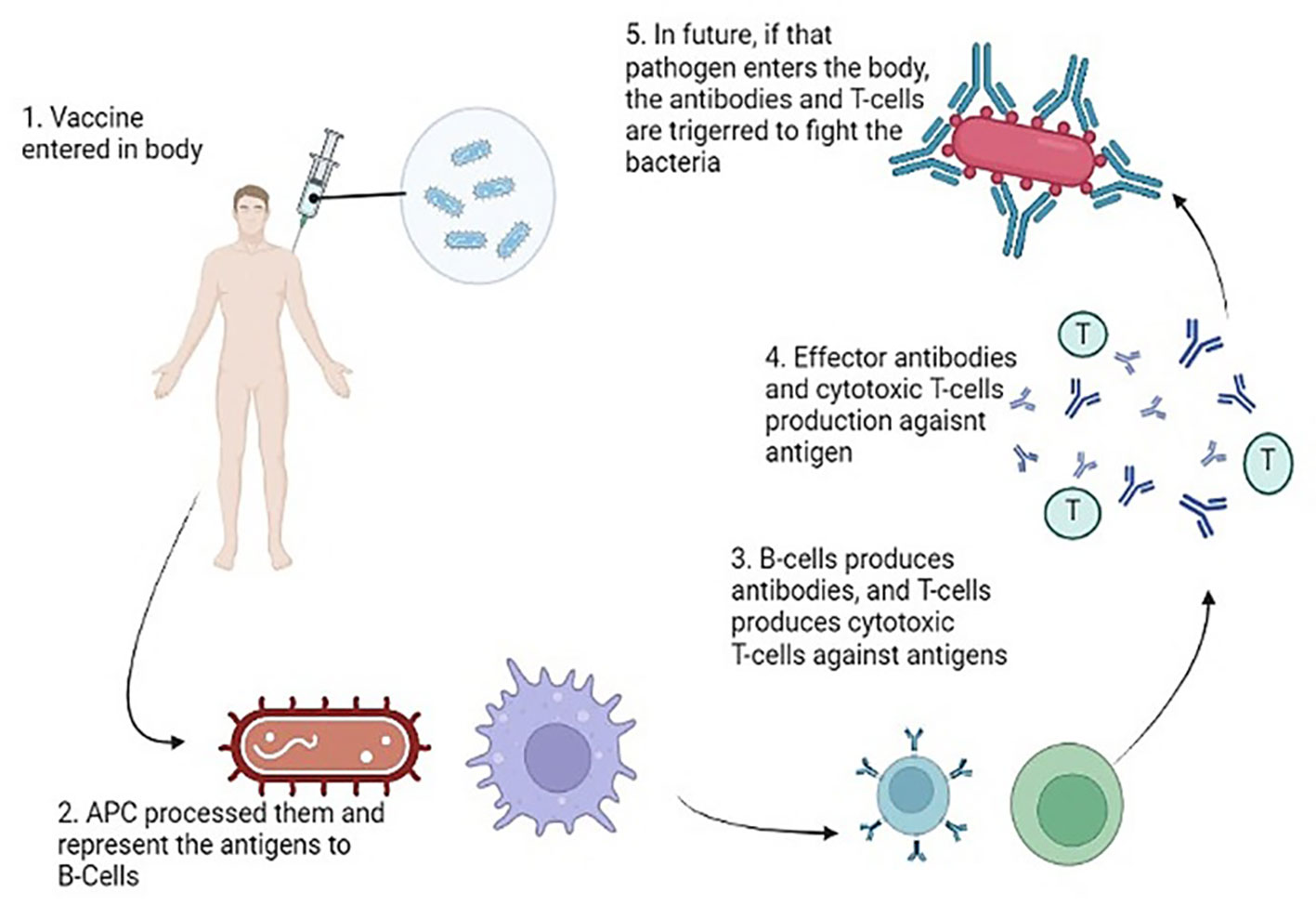
Figure 8 Mode of Action of Vaccines generating an immune response against the virulent components of bacteria.
3.5 Nanomaterials
In the realm of orthopedic surgery, the escalation of microbial resistance to current antibiotic therapies stands as a prominent contributor to implant failure and suboptimal clinical outcomes. However, recent strides in sophisticated antimicrobial nanotechnologies present promising opportunities to effectively combat resistant microbes and preclude the onset of resistance through specialized processes. Nanomaterials, with their programmable physicochemical properties, can be tailored to exhibit bactericidal, antifouling, and immunomodulating capabilities, enabling precise delivery of antibacterial agents to infection sites (Sharmin et al., 2021). Notably, nanoparticles (NPs) have emerged as innovative tools in the fight against severe bacterial diseases, circumventing conventional antimicrobial challenges, including antibiotic resistance (Imani et al., 2020).
3.5.1 Mode of action of nanomaterials
Nanoparticles exhibit distinct physio-chemical characteristics that position them as formidable contenders in combating antibiotic resistance. Leveraging a variety of novel bactericidal pathways, nanomaterials demonstrate the capacity to exert antimicrobial activity through intricate mechanisms. They can adhere to bacterial membranes, inducing damage and facilitating the release of cytoplasmic components. Upon membrane penetration, nanomaterials can further target intracellular elements, including DNA, ribosomes, and enzymes, disrupting normal cellular functions. This interference results in electrolyte imbalance, oxidative stress, and enzyme inhibition, collectively leading to bacterial cell death (Sánchez-López et al., 2020). The core material, shape, size, and surface functionalization of nanomaterials dictate their bactericidal pathways. Early studies focused on modifying intrinsic core materials to create nanomaterials with diverse modes of action. For instance, antimicrobials based on silver nanoparticles utilize free Ag+ ions as the active agent (Wang et al., 2017).
Silver ions damage DNA and impede electron transport across bacterial membranes. Copper nanoparticles generate reactive oxygen species (ROS), disrupting bacterial cells’ amino acid synthesis and DNA maintenance, akin to free Cu2+ ions. Meanwhile, ZnO and TiO2-based nanomaterials induce bacterial cell death by damaging cell membranes and producing ROS (Nisar et al., 2019). The versatility of nanomaterial cores offers a range of antibacterial actions against drug-resistant superbugs. However, unfunctionalized nanomaterials often exhibit limited antibacterial efficacy, restricting their biomedical applications due to low therapeutic indices (i.e., selectivity) against healthy mammalian cells. The surface chemistry of nanomaterials emerges as a pivotal factor in modulating their interactions with bacteria, enhancing broad-spectrum activity while concurrently reducing toxicity toward mammalian cells (Figure 9) (Jeevanandam et al., 2018). To elaborate on the mode of action against antibiotic-resistant bacteria, nanomaterials effectively disrupt key cellular processes in microorganisms, including interference with genetic material (DNA), inhibition of protein synthesis (ribosomes), and disruption of enzymatic activities. The multifaceted mechanisms employed by nanomaterials underscore their potential in addressing the challenge of antibiotic resistance.
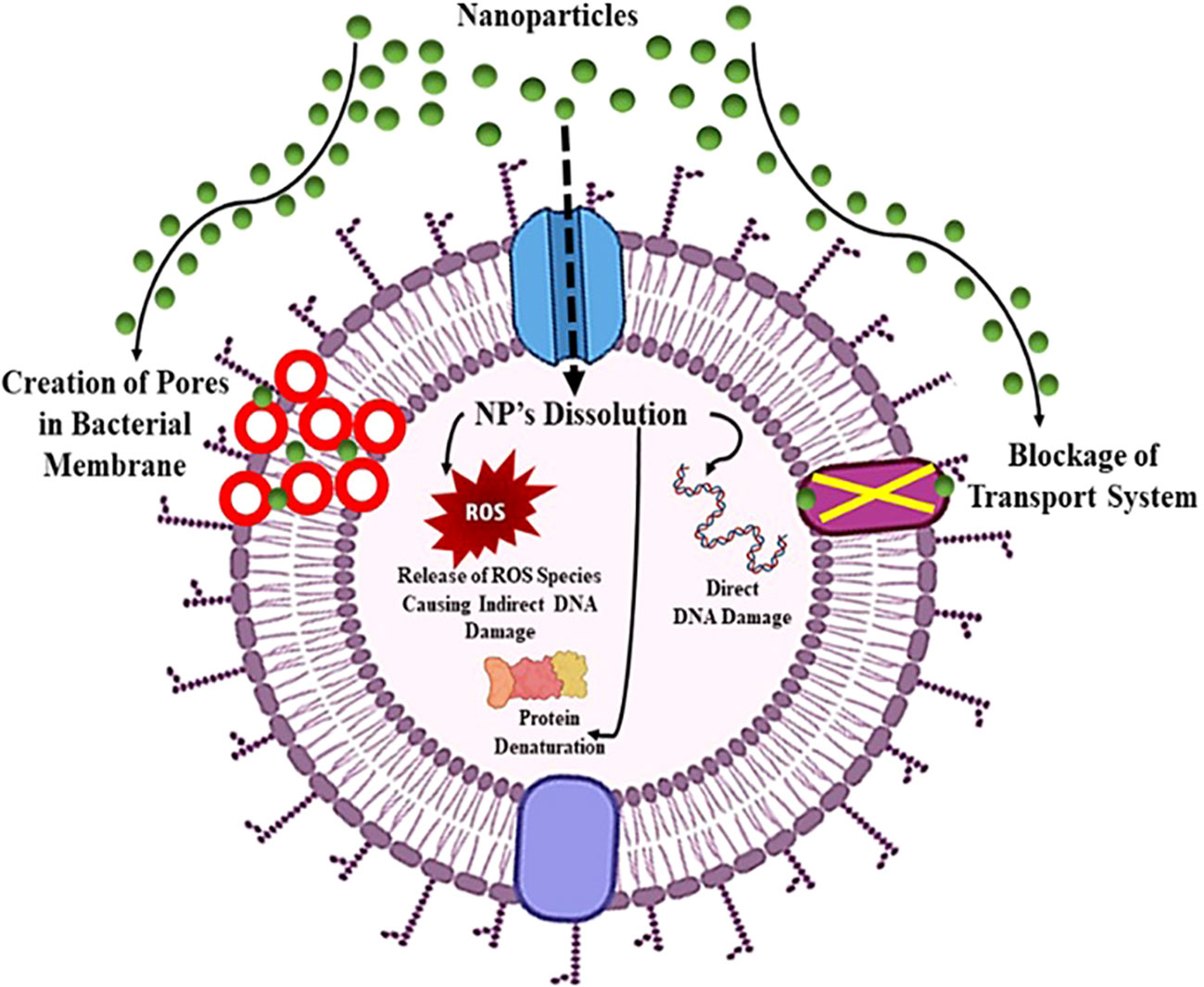
Figure 9 Mechanism of action of nanoparticles showing board spectrum antibacterial properties against pathogenic bacteria.
3.6 Gene editing
Pseudomonas aeruginosa genome editing has been employed to address multidrug resistance through Specific I-F CRISPR-Cas, inducing reverse mutations in the PA154197, an epidemic multidrug-resistant genotype (Makarova et al., 2011). The most used Cas protein is Cas9, with specific gRNA followed by CRISPR to edit the genome (Hartenian and Doench, 2015).
This strategy aims to combat multidrug resistance by developing sensitivity to antibiotics, disrupting the outer membrane’s permeability. The edited P. aeruginosa strain exhibits remarkable susceptibility to antibiotics, offering a potential breakthrough in the battle against multidrug-resistant bacteria. In addition to genome editing, chimeric antigen receptor (CAR-T) cells have been engineered for Allogeneic Combination Immunotherapy to tackle multidrug resistance. Molecular seizer Transcription activator-like effector nucleases (TALEN) were employed to modify CAR-T cells for allogeneic adoptive transfer of multidrug-resistant T cells (Xu et al., 2019). This approach not only reduces alloreactivity but also enables these cells to withstand lymphodepleting regimens, enhancing their therapeutic potential.
Multidrug resistance mechanisms often involve transporter proteins, with ABC transporters playing a key role in mediating resistance (Locher, 2016; Adamska and Falasca, 2018). These transporters, spanning from ABCA to ABCG, facilitate the transport of chemotherapeutics in and out of bacterial cells. Notably, these ABC transporters are predominantly located in the cell membrane of bacteria, serving to transport essential nutrients. P-glycoprotein (P-gp), a transmembrane glycoprotein encoded by ABCB1, is implicated in multidrug resistance (Beis, 2015). In cancerous cells, the knockout of ABCB1 enhances the sensitivity to multiple chemotherapeutic drugs, including alkaloids, taxanes, anthracyclines, and epipodophyllotoxins. Following the knockout, the increased sensitivity of ABCB1 is demonstrated through the accumulation of doxorubicin and rhodamine 123 in tumor cells, presenting a potential strategy for overcoming multidrug resistance in cancer treatment (Yang et al., 2016).
3.6.1 Mode of action of gene editing
Gene editing represents a revolutionary approach in addressing antibiotic-resistant bacteria by targeting specific genetic elements associated with resistance mechanisms. The primary mode of action involves the precise modification or disruption of genes responsible for conferring antibiotic resistance. For instance, the technique can be employed to target genes encoding antibiotic efflux pumps, which play a crucial role in expelling antibiotics from bacterial cells (Mohanty et al., 2021). By disrupting these pumps through gene editing, the bacteria’s ability to pump out antibiotics is compromised, rendering them more susceptible to the drugs. Another target includes genes involved in membrane permeability, a key factor in antibiotic resistance (Delcour, 2009). Gene editing can alter the structure or function of these genes, affecting the permeability of bacterial membranes and enhancing the penetration of antibiotics into the cells. Additionally, modifying genes related to drug targets can prevent bacteria from developing resistance by altering the target site’s structure (Luthra et al., 2018). This precise manipulation at the genetic level offers a nuanced and effective strategy to combat antibiotic resistance, providing hope for the development of novel antibacterial therapies. As advancements in gene editing technologies continue, the potential for tailored and targeted interventions against antibiotic-resistant bacteria becomes increasingly promising. (Figure 10).
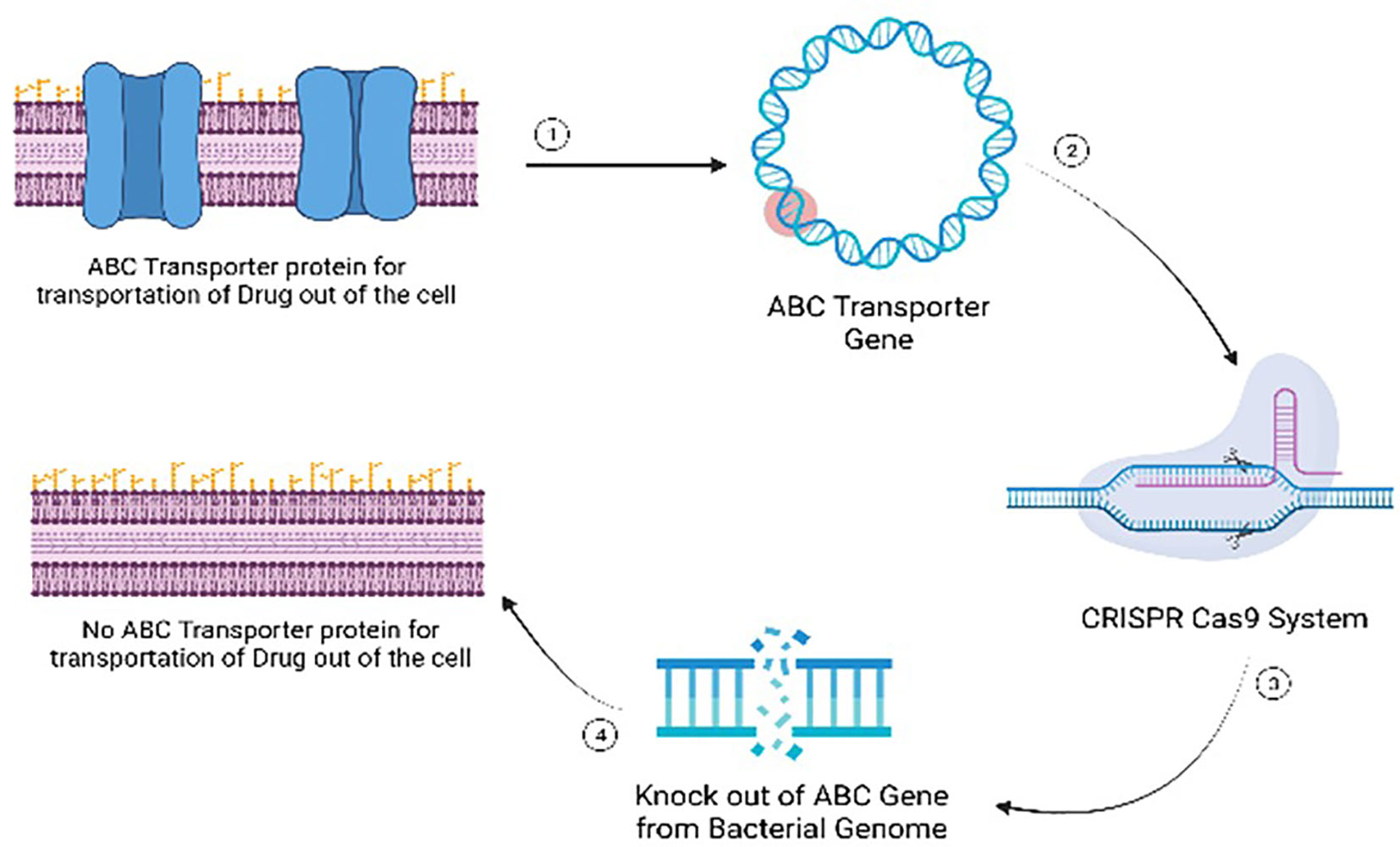
Figure 10 Mode of action of gene editing as an antimicrobial alternative for managing multi-drug resistant microbial infections.
4 Comparison of Methods in the curation of Bacterial infections
The effectiveness of fifth-generation antibiotics, bacteriocins, bacterial vaccines, Medicinal plants, nanomaterials, and gene editing in treating antibiotic-resistant bacteria varies based on their distinct mechanisms and applications. Fifth-generation antibiotics, characterized by improved spectrum coverage and reduced resistance development, have shown efficacy in addressing certain resistant strains. However, their impact is limited as bacteria can still evolve resistance over time (Fair and Tor, 2014). Bacteriocins, naturally occurring antimicrobial peptides produced by bacteria, exhibit promise in targeted bacterial inhibition. Their specificity allows for minimal harm to beneficial bacteria, but their application may be constrained by limited spectra of activity (Li et al., 2021). Medicinal plants offer a rich source of natural compounds with potential antimicrobial properties, providing a diverse array of bioactive molecules to combat antibiotic-resistant bacteria. However, their variable efficacy, dependence on specific plant species, and challenges in standardization present obstacles in harnessing their full potential as consistent and reliable treatments (Mickymaray, 2019). Bacterial vaccines, designed to stimulate the immune system against specific bacterial pathogens, offer a preventive approach to combat antibiotic resistance. While effective in preventing infections, their utility is restricted to specific bacterial strains, and the development of vaccines for all pathogens is challenging (Jansen et al., 2018). Nanomaterials, such as silver nanoparticles or nanotubes, present an innovative avenue by disrupting bacterial membranes and inhibiting growth. However, concerns about potential toxicity and environmental impact necessitate careful consideration (Ahmad et al., 2020). Gene editing techniques, on the other hand, provide a highly tailored and evolving strategy to address antibiotic resistance. By precisely targeting resistance genes, these techniques offer the potential to reverse or mitigate resistance mechanisms (Murugaiyan et al., 2022). The adaptability of gene editing allows for continuous refinement in response to emerging resistance patterns. Despite their promise, challenges include off-target effects and ethical considerations (Han et al., 2020). The comparison among the techniques mentioned above to combat multi-drug resistant bacteria is shown in Table 1.
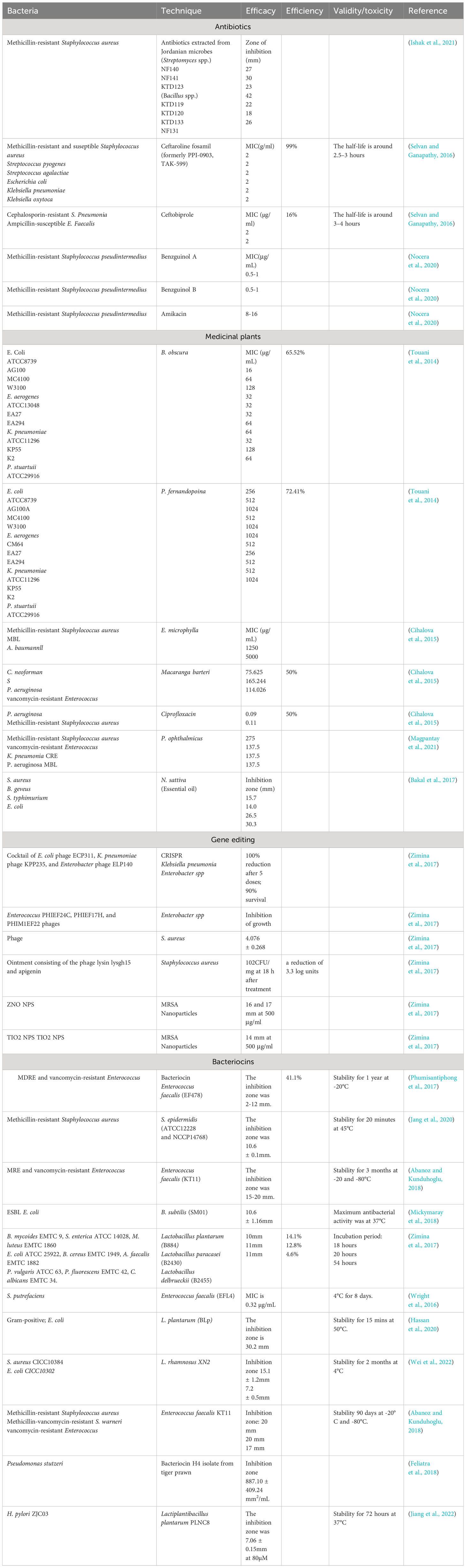
Table 1 Comparison of techniques used to combat bacterial infections via in-vitro biological assays.
5 Antibiotic stewardship advocacy
Antibiotic stewardship advocacy is crucial in addressing antibiotic resistance, emphasizing public education, responsible prescribing practices, and policy initiatives. Engaging healthcare providers through feedback mechanisms and policy initiatives, fostering international cooperation, and exploring innovative alternatives like phage therapy are integral to this multidimensional approach. Ongoing research and innovation remain essential for sustainable antibiotic use and global health (Majumder et al., 2020a; Majumder et al., 2020b). These initiatives encompass the regulation of antibiotic use in agriculture, healthcare settings, and the pharmaceutical industry. Collaborative efforts among healthcare institutions, research organizations, and pharmaceutical companies are highlighted for their role in developing and implementing responsible antibiotic use strategies, conducting research on antibiotic-resistant bacteria, and supporting the development of new antibiotics.
6 Conclusion
Multidrug-resistant organisms are bacteria that have developed resistance to specific treatments and can no longer be controlled or destroyed by them. Multidrug resistance in bacteria can be caused in one of two ways. These bacteria can accumulate many genes within a single cell, each of which codes for drug resistance to a single therapy. This type of accumulation is particularly common on-resistance (R) plasmids. Second, increased expression of genes encoding multidrug efflux pumps, which expel various drugs, can contribute to multidrug resistance. Antibiotic resistance is a big concern, as some bacteria have gained resistance to virtually all antibiotics now in use. These bacteria are a big public health concern because they have the potential to cause serious illness. Antibiotic-resistant gut bacteria include methicillin-resistant Staphylococcus aureus (MRSA), vancomycin-resistant Enterococcus (VRE), and multidrug-resistant Mycobacterium tuberculosis (MDR-TB), and carbapenem-resistant Enterobacteriaceae (CRE). Antimicrobial resistance is on the rise worldwide, and Antibiotic resistance has extended to various environmental niches and the introduction of superbugs has made it even more difficult to implement effective control measures. With advances in science and technology, this review exposes the most cutting-edge technologies, such as newly discovered medicinal plants, unique gene-editing techniques, novel nanomaterials, and bacterial vaccines, that will eventually aid in the fight against MDR bacteria in the future.
Author contributions
MN: Supervision, Writing – original draft, Writing – review & editing. MW: Conceptualization, Methodology, Writing – original draft. IM: Conceptualization, Methodology, Supervision, Writing – original draft, Writing – review & editing. NA: Conceptualization, Methodology, Supervision, Writing – original draft, Writing – review & editing. FA: Writing – original draft. JH: Writing – original draft. HJ: Writing – original draft.
Funding
The author(s) declare that no financial support was received for the research, authorship, and/or publication of this article.
Acknowledgments
We would like to acknowledge that no individuals or organizations were involved in the development, funding, or support of this work. This research was solely conducted by the authors, and no external contributions, financial or otherwise, were received or utilized in the execution of this study.
Conflict of interest
The authors declare that the research was conducted in the absence of any commercial or financial relationships that could be construed as a potential conflict of interest.
Publisher’s note
All claims expressed in this article are solely those of the authors and do not necessarily represent those of their affiliated organizations, or those of the publisher, the editors and the reviewers. Any product that may be evaluated in this article, or claim that may be made by its manufacturer, is not guaranteed or endorsed by the publisher.
References
Abanoz H. S., Kunduhoglu B. (2018). Antimicrobial activity of a bacteriocin produced by Enterococcus faecalis KT11 against some pathogens and antibiotic-resistant bacteria. Korean J. Food Sci. Anim. Resour. 38 (5), 1064. doi: 10.5851/kosfa.2018.e40
Acedo J. Z., van Belkum M. J., Lohans C. T., McKay R. T., Miskolzie M., Vederas J. C. (2015). Solution structure of acidocin B, a circular bacteriocin produced by Lactobacillus acidophilus M46. Appl. Environ. Microbiol. 81 (8), 2910–2918. doi: 10.1128/AEM.04265-14
Adamska A., Falasca M. (2018). ATP-binding cassette transporters in progression and clinical outcome of pancreatic cancer: What is the way forward? World J. Gastroenterol. 24 (29), 3222. doi: 10.3748/wjg.v24.i29.3222
Adel W. A., Ahmed A. M., Hegazy Y., Torky H. A., Shimamoto T. (2021). High prevalence of ESBL and plasmid-mediated quinolone resistance genes in Salmonella enterica isolated from retail meats and slaughterhouses in Egypt. Antibiotics 10 (7), 881. doi: 10.3390/antibiotics10070881
Agyepong N. (2017). Molecular profile of gram-negative ESKAPE pathogens from Komfo Anokye Teaching Hospital in Ghana. Okomfo Anokye Road, Kumasi, Ghana.
Ahmad I., Aqil F., Owais M. (2006). Modern phytomedicine: Turning medicinal plants into drugs (John Wiley & Sons).
Ahmad S. A., Das S. S., Khatoon A., Ansari M. T., Afzal M., Hasnain M. S., et al. (2020). Bactericidal activity of silver nanoparticles: A mechanistic review. Mater. Sci. Energy Technol. 3, 756–769. doi: 10.1016/j.mset.2020.09.002
Ahmadi M., Ranjbar R., Behzadi P., Mohammadian T. (2022). Virulence factors, antibiotic resistance patterns, and molecular types of clinical isolates of Klebsiella Pneumoniae. Expert Rev. Anti-Infective Ther. 20 (3), 463–472. doi: 10.1080/14787210.2022.1990040
Ahmed M. O., Baptiste K. E. (2018). Vancomycin-resistant enterococci: a review of antimicrobial resistance mechanisms and perspectives of human and animal health. Microb. Drug Resist. 24 (5), 590–606. doi: 10.1089/mdr.2017.0147
Ali T., Ali I., Khan N. A., Han B., Gao J. (2018). The growing genetic and functional diversity of extended spectrum beta-lactamases. BioMed. Res. Int. 2018.
Ali A., Kumar R., Khan A., Khan A. U. (2020). Interaction of LysM BON family protein domain with carbapenems: A putative mechanism of carbapenem resistance. Int. J. Biol. Macromol. 160, 212–223. doi: 10.1016/j.ijbiomac.2020.05.172
Anstey K., Anstey J., Hilts-Horeczko A., Doernberg S. B., Chen L.-L., Otani I. M. (2019). Perioperative use and safety of cephalosporin antibiotics in patients with documented penicillin allergy. J. Allergy Clin. Immunol. 143 (2), AB29. doi: 10.1016/j.jaci.2018.12.091
Bakal S. N., Bereswill S., Heimesaat M. M. (2017). Finding novel antibiotic substances from medicinal plants—antimicrobial properties of Nigella sativa directed against multidrug resistant bacteria. Eur. J. Microbiol. Immunol. 7 (1), 92–98. doi: 10.1556/1886.2017.00001
Beis K. (2015). Structural basis for the mechanism of ABC transporters. Biochem. Soc. Trans. 43 (5), 889–893. doi: 10.1042/BST20150047
Bekeredjian-Ding I. (2020). Challenges for clinical development of vaccines for prevention of hospital-acquired bacterial infections. Front. Immunol. 11, 1755. doi: 10.3389/fimmu.2020.01755
Berglund F., Marathe N. P., Österlund T., Bengtsson-Palme J., Kotsakis S., Flach C.-F., et al. (2017). Identification of 76 novel B1 metallo-β-lactamases through large-scale screening of genomic and metagenomic data. Microbiome 5, 1–13. doi: 10.1186/s40168-017-0353-8
Bianco G., Boattini M., Iannaccone M., Bondi A., Ghibaudo D., Zanotto E., et al. (2021). Carbapenemase detection testing in the era of ceftazidime/avibactam-resistant KPC-producing Enterobacterales: A 2-year experience. J. Global Antimicrob. Resist. 24, 411–414. doi: 10.1016/j.jgar.2021.02.008
Bidmos F. A., Siris S., Gladstone C. A., Langford P. R. (2018). Bacterial vaccine antigen discovery in the reverse vaccinology 2.0 Era: Progress and challenges. Front. Immunol. 9, 2315. doi: 10.3389/fimmu.2018.02315
Brindangnanam P., Sawant A. R., Prashanth K., Coumar M. S. (2022). Bacterial effluxome as a barrier against antimicrobial agents: structural biology aspects and drug targeting. Tissue Barriers 10 (4), 2013695. doi: 10.1080/21688370.2021.2013695
Bush K. (2018). Past and present perspectives on β-lactamases. Antimicrob. Agents Chemother. 62 (10). doi: 10.1128/aac.01076-18
Bush K., Bradford PAJNRM. (2019). Interplay between β-lactamases and new β-lactamase inhibitors. Nat. Rev. Microbiol. 17, 5, 295–306. doi: 10.1038/s41579-019-0159-8
Caprari S. (2021). Investigation of the sheltering effect of β-lactam-resistant K. pneumoniae species on twosusceptible E. coli and S. aureus strains (Anglia Ruskin University).
Carcione D., Siracusa C., Sulejmani A., Leoni V., Intra J. (2021). Old and new beta-lactamase inhibitors: Molecular structure, mechanism of action, and clinical Use. Antibiotics 10 (8), 995. doi: 10.3390/antibiotics10080995
Castanheira M., Simner P. J., Bradford P. A. (2021). Extended-spectrum β-lactamases: an update on their characteristics, epidemiology and detection. JAC Antimicrob. Resist. 3 (3), dlab092. doi: 10.1093/jacamr/dlab092
Chauzy A., Gaelzer Silva Torres B., Buyck J., de Jonge B., Adier C., Marchand S., et al. (2019). Semimechanistic pharmacodynamic modeling of aztreonam-avibactam combination to understand its antimicrobial activity against multidrug-resistant gram-negative bacteria. CPT: Pharmacometrics Syst. Pharmacol. 8 (11), 815–824. doi: 10.1002/psp4.12452
Chojnacki M., Philbrick A., Wucher B., Reed J. N., Tomaras A., Dunman P. M., et al. (2019). Development of a broad-spectrum antimicrobial combination for the treatment of Staphylococcus aureus and Pseudomonas aeruginosa corneal infections. Antimicrob. Agents Chemother. 63 (1). doi: 10.1128/aac.01929-18
Cihalova K., Chudobova D., Michalek P., Moulick A., Guran R., Kopel P., et al. (2015). Staphylococcus aureus and MRSA growth and biofilm formation after treatment with antibiotics and SeNPs. Int. J. Mol. Sci. 16 (10), 24656–24672. doi: 10.3390/ijms161024656
Dafne B., Gino M., Stefania S., Floriana C. (2019). Genotypic analysis of Italian MRSA strains exhibiting low-level ceftaroline and ceftobiprole resistance. Diagn. Microbiol. Infect. Dis. 95 (3), 114852. doi: 10.1016/j.diagmicrobio.2019.06.004
Davies D. T., Everett M. (2021). Designing inhibitors of β-lactamase enzymes to overcome carbapenem resistance in Gram-negative bacteria. Accounts Chem. Res. 54 (9), 2055–2064. doi: 10.1021/acs.accounts.0c00863
Dawan J., Ahn J. (2020). Assessment of β-Lactamase Inhibitor Potential of Medicinal Plant Extracts against Antibiotic-resistant Staphylococcus aureus. Korean J. Plant Resour. 33 (6), 578–585. doi: 10.7732/kjpr.2020.33.6.578
Delcour A. H. (2009). Outer membrane permeability and antibiotic resistance. Biochim. Biophys. Acta (BBA) Proteins Proteom. 1794 (5), 808–816. doi: 10.1016/j.bbapap.2008.11.005
Djeussi D. E., Noumedem J. A., Ngadjui B. T., Kuete V. (2016). Antibacterial and antibiotic-modulation activity of six Cameroonian medicinal plants against Gram-negative multi-drug resistant phenotypes. BMC Complement. Altern. Med. 16, 1–9. doi: 10.1186/s12906-016-1105-1
Dowling A., O’dwyer J., Adley C. (2017). Antibiotics: mode of action and mechanisms of resistance. Antimicrob. Res.: Novel Bioknowledge Educ. Programs 1, 536–545.
Eichenberger E. M., Thaden J. T. (2019). Epidemiology and mechanisms of resistance of extensively drug resistant Gram-negative bacteria. Antibiotics 8 (2), 37. doi: 10.3390/antibiotics8020037
Ejaz H., Younas S., Abosalif K. O., Junaid K., Alzahrani B., Alsrhani A., et al. (2021). Molecular analysis of bla SHV, bla TEM, and bla CTX-M in extended-spectrum β-lactamase producing Enterobacteriaceae recovered from fecal specimens of animals. PloS One 16 (1), e0245126. doi: 10.1371/journal.pone.0245126
El-Halfawy O. M., Czarny T. L., Flannagan R. S., Day J., Bozelli J. C., Kuiack R. C., et al. (2020). Discovery of an antivirulence compound that reverses β-lactam resistance in MRSA. Nat. Chem. Biol. 16 (2), 143–149. doi: 10.1038/s41589-019-0401-8
Fair R. J., Tor Y. (2014). Antibiotics and bacterial resistance in the 21st century. Perspectives in medicinal chemistry (Okomfo Anokye Road, Kumasi, Ghana), Vol. 6. PMC. S14459.
Farhat N., Ali A., Bonomo R. A., Khan A. U. (2020). Efflux pumps as interventions to control infection caused by drug-resistance bacteria. Drug Discovery Today 25 (12), 2307–2316. doi: 10.1016/j.drudis.2020.09.028
Farhat N., Gupta D., Ali A., Kumar Y., Akhtar F., Kulanthaivel S., et al. (2022). Broad-Spectrum Inhibitors against Class A, B, and C Type β-Lactamases to Block the Hydrolysis against Antibiotics: Kinetics and Structural Characterization. Microbiol. Spectrum 10 (5), e00450–e00422. doi: 10.1128/spectrum.00450-22
Faron M. L., Ledeboer N. A., Buchan B. W. (2016). Resistance mechanisms, epidemiology, and approaches to screening for vancomycin-resistant Enterococcus in the health care setting. J. Clin. Microbiol. 54 (10), 2436–2447. doi: 10.1128/JCM.00211-16
Feliatra F., Nursyirwani, Tanjung A., Adithiya D., Susanna M., Lukystyowati I. (Eds.) (2018). “The effectiveness of heterotrophic bacteria isolated from Dumai marine waters of Riau, used as antibacterial against pathogens in fish culture,” in IOP Conference Series: Earth and Environmental Science (Iop Conference series, Earth and Environmental Science).
Fiore E., Van Tyne D., Gilmore M. S. (2019). Pathogenicity of enterococci. Microbiol. Spectrum 7 (4), 7.4. 9. doi: 10.1128/9781683670131.ch24
Fisher J. F., Mobashery S. (2020). β-Lactams against the Fortress of the Gram-Positive Staphylococcus aureus Bacterium. Chem. Rev. 121 (6), 3412–3463. doi: 10.1021/acs.chemrev.0c01010
Fong I. (2023). New Cephalosporins: Fifth and Sixth Generations. New Antimicrobials: For the Present and the Future (Springer), 25–38.
Gajdács M. (2019). The continuing threat of methicillin-resistant Staphylococcus aureus. Antibiotics 8 (2), 52. doi: 10.3390/antibiotics8020052
Galani I., Karaiskos I., Giamarellou H. (2021). Multidrug-resistant Klebsiella pneumoniae: mechanisms of resistance including updated data for novel β-lactam-β-lactamase inhibitor combinations. Expert Rev. Anti-Infective Ther. 19 (11), 1457–1468. doi: 10.1080/14787210.2021.1924674
Golbarg H., Mehdipour Moghaddam M. J. (2021). Antibacterial potency of medicinal plants including Artemisia annua and Oxalis corniculata against multi-drug resistance E. coil. BioMed. Res. Int. 2021. doi: 10.1155/2021/9981915
Govindaswamy A., Bajpai V., Khurana S., Aravinda A., Batra P., Malhotra R., et al. (2019). Prevalence and characterization of beta-lactamase-producing Escherichia coli isolates from a tertiary care hospital in India. J. Lab. Physicians 11 (02), 123–127. doi: 10.4103/JLP.JLP_122_18
Hammoudi Halat D., Ayoub Moubareck C. (2020). The current burden of carbapenemases: Review of significant properties and dissemination among gram-negative bacteria. Antibiotics 9 (4), 186. doi: 10.3390/antibiotics9040186
Han H. A., Pang J. K. S., Soh B.-S. (2020). Mitigating off-target effects in CRISPR/Cas9-mediated in vivo gene editing. J. Mol. Med. 98 (5), 615–632. doi: 10.1007/s00109-020-01893-z
Hartenian E., Doench J. G. (2015). Genetic screens and functional genomics using CRISPR/Cas9 technology. FEBS J. 282 (8), 1383–1393. doi: 10.1111/febs.13248
Hassan M. U., Nayab H., Rehman T. U., Williamson M. P., Haq K. U., Shafi N., et al. (2020). Characterisation of bacteriocins produced by Lactobacillus spp. isolated from the traditional Pakistani yoghurt and their antimicrobial activity against common foodborne pathogens. BioMed. Res. Int. 2020. doi: 10.1155/2020/8281623
Hernando-Amado S., Coque T. M., Baquero F., Martínez J. L. (2019). Defining and combating antibiotic resistance from One Health and Global Health perspectives. Nat. Microbiol. 4 (9), 1432–1442. doi: 10.1038/s41564-019-0503-9
Hussain H. I., Aqib A. I., Seleem M. N., Shabbir M. A., Hao H., Iqbal Z., et al. (2021). Genetic basis of molecular mechanisms in β-lactam resistant gram-negative bacteria. Microb. Pathogen. 158, 105040. doi: 10.1016/j.micpath.2021.105040
Imani S. M., Ladouceur L., Marshall T., Maclachlan R., Soleymani L., Didar T. F. (2020). Antimicrobial nanomaterials and coatings: Current mechanisms and future perspectives to control the spread of viruses including SARS-CoV-2. ACS Nano 14 (10), 12341–12369. doi: 10.1021/acsnano.0c05937
Ishak B., Abdul-Jabbar A., Moss G. B., Yilmaz E., von Glinski A., Frieler S., et al. (2021). De novo methicillin-resistant Staphylococcus aureus vs. methicillin-sensitive Staphylococcus aureus infections of the spine, similar clinical outcome, despite more severe presentation in surgical patients. Neurosurg. Rev 44, 2111–2118. doi: 10.1007/s10143-020-01376-2
Jang I.-T., Yang M., Kim H.-J., Park J.-K. (2020). Novel cytoplasmic bacteriocin compounds derived from Staphylococcus epidermidis selectively kill Staphylococcus aureus, including methicillin-resistant Staphylococcus aureus (MRSA). Pathogens 9 (2), 87. doi: 10.3390/pathogens9020087
Jansen K. U., Knirsch C., Anderson A. S. (2018). The role of vaccines in preventing bacterial antimicrobial resistance. Nat. Med. 24 (1), 10–19. doi: 10.1038/nm.4465
Jean S.-S., Lee Y.-L., Liu P.-Y., Lu M.-C., Ko W.-C., Hsueh P.-R. (2022). Multicenter surveillance of antimicrobial susceptibilities and resistance mechanisms among Enterobacterales species and non-fermenting Gram-negative bacteria from different infection sources in Taiwan from 2016 to 2018. J. Microbiol. Immunol. Infect. 55 (3), 463–473. doi: 10.1016/j.jmii.2021.07.015
Jeevanandam J., Barhoum A., Chan Y. S., Dufresne A., Danquah M. K. (2018). Review on nanoparticles and nanostructured materials: history, sources, toxicity and regulations. Beilstein J. Nanotechnol. 9 (1), 1050–1074. doi: 10.3762/bjnano.9.98
Jiang Y., Zheng W., Tran K., Kamilar E., Bariwal J., Ma H., et al. (2022). Hydrophilic nanoparticles that kill bacteria while sparing mammalian cells reveal the antibiotic role of nanostructures. Nat. Commun. 13 (1), 197. doi: 10.1038/s41467-021-27193-9
Jorgensen I., Rayamajhi M., Miao E. A. (2017). Programmed cell death as a defence against infection. Nat. Rev. Immunol. 17 (3), 151–164. doi: 10.1038/nri.2016.147
Kabanangi F., Nkuwi E. J., Manyahi J., Moyo S., Majigo M. (2021). High level of multidrug-resistant gram-negative pathogens causing burn wound infections in hospitalized children in dar es salaam, Tanzania. Int. J. Microbiol. 2021. doi: 10.1155/2021/6644185
Khameneh B., Eskin N. M., Iranshahy M., Fazly Bazzaz B. S. (2021). Phytochemicals: a promising weapon in the arsenal against antibiotic-resistant bacteria. Antibiotics 10 (9), 1044. doi: 10.3390/antibiotics10091044
Khan S. A., Imtiaz M. A., Sayeed M. A., Shaikat A. H., Hassan M. M. (2020). Antimicrobial resistance pattern in domestic animal-wildlife-environmental niche via the food chain to humans with a Bangladesh perspective; a systematic review. BMC Vet. Res. 16, 1–13. doi: 10.1186/s12917-020-02519-9
Khoshbakht R., Shahed A., Aski H. S. (2014). Characterization of extended-spectrum î’-lactamase-producing Escherichia coli strains isolated from dairy products. J. Microbiol. Biotechnol. Food Sci. 3 (4), 333–336.
Kuete V., Djeussi D. E., Mbaveng A. T., Zeino M., Efferth T. (2016). Cytotoxicity of 15 Cameroonian medicinal plants against drug sensitive and multi-drug resistant cancer cells. J. Ethnopharmacol. 186, 196–204. doi: 10.1016/j.jep.2016.04.001
Li W., Separovic F., O’Brien-Simpson N. M., Wade J. D. (2021). Chemically modified and conjugated antimicrobial peptides against superbugs. Chem. Soc. Rev. 50 (8), 4932–4973. doi: 10.1039/D0CS01026J
Lin X., Kück U. (2022). Cephalosporins as key lead generation beta-lactam antibiotics. Appl. Microbiol. Biotechnol. 106 (24), 8007–8020. doi: 10.1007/s00253-022-12272-8
Liu W.-T., Chen E.-Z., Yang L., Peng C., Wang Q., Xu Z., et al. (2021). Emerging resistance mechanisms for 4 types of common anti-MRSA antibiotics in Staphylococcus aureus: A comprehensive review. Microb. Pathogen. 156, 104915. doi: 10.1016/j.micpath.2021.104915
Liu B., Trout R. E. L., Chu G.-H., McGarry D., Jackson R. W., Hamrick J. C., et al. (2019). Discovery of taniborbactam (VNRX-5133): a broad-spectrum serine-and metallo-β-lactamase inhibitor for carbapenem-resistant bacterial infections (ACS Publications).
Locher K. P. (2016). Mechanistic diversity in ATP-binding cassette (ABC) transporters. Nat. Struct. Mol. Biol. 23 (6), 487–493. doi: 10.1038/nsmb.3216
Luthra S., Rominski A., Sander P. (2018). The role of antibiotic-target-modifying and antibiotic-modifying enzymes in Mycobacterium abscessus drug resistance. Front. Microbiol. 9, 2179. doi: 10.3389/fmicb.2018.02179
Magpantay H. D., Malaluan I. N., Manzano J. A. H., Quimque M. T., Pueblos K. R., Moor N., et al. (2021). Antibacterial and COX-2 inhibitory tetrahydrobisbenzylisoquinoline alkaloids from the Philippine medicinal plant Phaeanthus ophthalmicus. Plants 10 (3), 462. doi: 10.3390/plants10030462
Majumder M. A. A., Rahman S., Cohall D., Bharatha A., Singh K., Haque M., et al. (2020a). Antimicrobial stewardship: Fighting antimicrobial resistance and protecting global public health. Infect. Drug Resist. 13 (2020), 4713–4738. doi: 10.2147/IDR.S290835
Majumder M. A. A., Singh K., Hilaire M. G.-S., Rahman S., Sa B., Haque M. (2020b). Tackling antimicrobial resistance by promoting antimicrobial stewardship in medical and allied health professional curricula. Expert Rev. Anti-Infective Ther. 18 (12), 1245–1258. doi: 10.1080/14787210.2020.1796638
Makarova K. S., Haft D. H., Barrangou R., Brouns S. J., Charpentier E., Horvath P., et al. (2011). Evolution and classification of the CRISPR–Cas systems. Nat. Rev. Microbiol. 9 (6), 467–477. doi: 10.1038/nrmicro2577
Mayer R. L., Impens F. (2021). Immunopeptidomics for next-generation bacterial vaccine development. Trends Microbiol. 29 (11), 1034–1045. doi: 10.1016/j.tim.2021.04.010
Mazumdar A., Adam V. (2021). Antimicrobial peptides-An alternative candidates to antibiotics against Staphylococcus aureus and its antibiotic-resistant strains. J. Mol. Clin. Med. 4 (1), 1–17. doi: 10.31083/j.jmcm.2021.01.208
Meini S., Tascini C., Cei M., Sozio E., Rossolini G. M. (2019). AmpC β-lactamase-producing Enterobacterales: what a clinician should know. Infection 47, 363–375. doi: 10.1007/s15010-019-01291-9
Mickymaray S. (2019). Efficacy and mechanism of traditional medicinal plants and bioactive compounds against clinically important pathogens. Antibiotics 8 (4), 257. doi: 10.3390/antibiotics8040257
Mickymaray S., Alturaiki W., Al-Aboody M. S., Mariappan P., Rajenderan V., Alsagaby S. A., et al. (2018). Anti-bacterial efficacy of bacteriocin produced by marine Bacillus subtilis against clinically important extended spectrum beta-lactamase strains and methicillin-resistant Staphylococcus aureus. Int. J. Med. Res. Health Sci. 7 (2), 75–83.
Mohanty H., Pachpute S., Yadav R. P. (2021). Mechanism of drug resistance in bacteria: efflux pump modulation for designing of new antibiotic enhancers. Folia Microbiol. 66 (5), 727–739. doi: 10.1007/s12223-021-00910-z
Monserrat-Martinez A., Gambin Y., Sierecki E. (2019). Thinking outside the bug: molecular targets and strategies to overcome antibiotic resistance. Int. J. Mol. Sci. 20 (6), 1255. doi: 10.3390/ijms20061255
Mora-Ochomogo M., Lohans C. T. (2021). β-Lactam antibiotic targets and resistance mechanisms: from covalent inhibitors to substrates. RSC Medicinal Chem. 12 (10), 1623–1639. doi: 10.1039/D1MD00200G
Morosini M.-I., Díez-Aguilar M., Cantón R. (2019). Mechanisms of action and antimicrobial activity of ceftobiprole. Rev. Española Quimioterapia 32 (Suppl 3), 3.
Mühlberg E., Umstätter F., Kleist C., Domhan C., Mier W., Uhl P. (2020). Renaissance of vancomycin: Approaches for breaking antibiotic resistance in multidrug-resistant bacteria. Can. J. Microbiol. 66 (1), 11–16. doi: 10.1139/cjm-2019-0309
Murugaiyan J., Kumar P. A., Rao G. S., Iskandar K., Hawser S., Hays J. P., et al. (2022). Progress in alternative strategies to combat antimicrobial resistance: Focus on antibiotics. Antibiotics 11 (2), 200. doi: 10.3390/antibiotics11020200
Negash A. W., Tsehai B. A. (2020). Current applications of bacteriocin. Int. J. Microbiol. 2020. doi: 10.1155/2020/4374891
Nguyen L. (2016). Antibiotic resistance mechanisms in M. tuberculosis: an update. Arch. Toxicol. 90, 1585–1604. doi: 10.1007/s00204-016-1727-6
Nisar P., Ali N., Rahman L., Ali M., Shinwari Z. K. (2019). Antimicrobial activities of biologically synthesized metal nanoparticles: an insight into the mechanism of action. JBIC J. Biol. Inorganic Chem. 24, 929–941. doi: 10.1007/s00775-019-01717-7
Nocera F. P., Mancini S., Najar B., Bertelloni F., Pistelli L., De Filippis A., et al. (2020). Antimicrobial activity of some essential oils against methicillin-susceptible and methicillin-resistant Staphylococcus pseudintermedius-associated pyoderma in dogs. Animals 10 (10), 1782. doi: 10.3390/ani10101782
O’Connor P. M., Kuniyoshi T. M., Oliveira R. P., Hill C., Ross R. P., Cotter P. D. (2020). Antimicrobials for food and feed; a bacteriocin perspective. Curr. Opin. Biotechnol. 61, 160–167. doi: 10.1016/j.copbio.2019.12.023
Palacios A. R., Rossi M.-A., Mahler G. S., Vila A. J. (2020). Metallo-β-lactamase inhibitors inspired on snapshots from the catalytic mechanism. Biomolecules 10 (6), 854. doi: 10.3390/biom10060854
Phumisantiphong U., Siripanichgon K., Reamtong O., Diraphat P. (2017). A novel bacteriocin from Enterococcus faecalis 478 exhibits a potent activity against vancomycin-resistant enterococci. PloS One 12 (10), e0186415. doi: 10.1371/journal.pone.0186415
Poolman J. T. (2020). Expanding the role of bacterial vaccines into life-course vaccination strategies and prevention of antimicrobial-resistant infections. NPJ Vaccines 5 (1), 84. doi: 10.1038/s41541-020-00232-0
Portsmouth S., van Veenhuyzen D., Echols R., Machida M., Ferreira J. C. A., Ariyasu M., et al. (2018). Cefiderocol versus imipenem-cilastatin for the treatment of complicated urinary tract infections caused by Gram-negative uropathogens: a phase 2, randomised, double-blind, non-inferiority trial. Lancet Infect. Dis. 18 (12), 1319–1328. doi: 10.1016/S1473-3099(18)30554-1
Pulzova L., Navratilova L., Comor L. (2017). Alterations in outer membrane permeability favor drug-resistant phenotype of Klebsiella pneumoniae. Microb. Drug Resist. 23 (4), 413–420. doi: 10.1089/mdr.2016.0017
Ramos S., Silva V., Dapkevicius M., Caniça M., Tejedor-Junco M. T., Igrejas G., et al. (2020). Escherichia coli as commensal and pathogenic bacteria among food-producing animals: Health implications of extended spectrum β-lactamase (ESBL) production. Animals 10 (12), 2239. doi: 10.3390/ani10122239
Rusu A., Lungu I.-A. (2020). The new fifth-generation cephalosporins–a balance between safety and efficacy. Romanian J. Pharm. PRACTICE| Vol XIII 52 (3). doi: 10.37897/RJPhP.2020.3.2
Said H. S., Abdelmegeed E. S. (2019). Emergence of multidrug resistance and extensive drug resistance among enterococcal clinical isolates in Egypt. Infect. Drug Resist., 1113–1125. doi: 10.2147/IDR.S189341
Sánchez-López E., Gomes D., Esteruelas G., Bonilla L., Lopez-MaChado A. L., Galindo R., et al. (2020). Metal-based nanoparticles as antimicrobial agents: an overview. Nanomaterials 10 (2), 292. doi: 10.3390/nano10020292
Sawa T., Kooguchi K., Moriyama K. (2020). Molecular diversity of extended-spectrum β-lactamases and carbapenemases, and antimicrobial resistance. J. Intensive Care 8, 1–13. doi: 10.1186/s40560-020-0429-6
Seifert H., Stefanik D., Sutcliffe J. A., Higgins P. G. (2018). In-vitro activity of the novel fluorocycline eravacycline against carbapenem non-susceptible Acinetobacter baumannii. Int. J. Antimicrob. Agents 51 (1), 62–64. doi: 10.1016/j.ijantimicag.2017.06.022
Selvan S. R., Ganapathy D. (2016). Efficacy of fifth generation cephalosporins against methicillin-resistant Staphylococcus aureus-A review. Res. J. Pharm. Technol. 9 (10), 1815–1818. doi: 10.5958/0974-360X.2016.00369.3
Sharmin N., Pang C., Sone I., Walsh J. L., Fernández C. G., Sivertsvik M., et al. (2021). Synthesis of sodium alginate–silver nanocomposites using plasma activated water and cold atmospheric plasma treatment. Nanomaterials 11 (9), 2306. doi: 10.3390/nano11092306
Shukla R., Medeiros-Silva J., Parmar A., Vermeulen B. J., Das S., Paioni A. L., et al. (2020). Mode of action of teixobactins in cellular membranes. Nat. Commun. 11 (1), 2848. doi: 10.1038/s41467-020-16600-2
Sianglum W., Muangngam K., Joycharat N., Voravuthikunchai S. P. (2019). Mechanism of action and biofilm inhibitory activity of lupinifolin against multidrug-resistant enterococcal clinical isolates. Microb. Drug Resist. 25 (10), 1391–1400. doi: 10.1089/mdr.2018.0391
Sun Y., Lu H., Zhang X., Wu Q., Bi W., Liu H., et al. (2018). Phenotype and genotype alteration during adaptive evolution of Enterococcus faecalis to antimicrobials. Infect. Genet. Evol. 62, 80–85. doi: 10.1016/j.meegid.2018.03.029
Thaden J. T., Pogue J. M., Kaye K. S. (2017). Role of newer and re-emerging older agents in the treatment of infections caused by carbapenem-resistant Enterobacteriaceae. Virulence 8 (4), 403–416. doi: 10.1080/21505594.2016.1207834
Touani F. K., Seukep A. J., Djeussi D. E., Fankam A. G., Noumedem J. A., Kuete V. (2014). Antibiotic-potentiation activities of four Cameroonian dietary plants against multidrug-resistant Gram-negative bacteria expressing efflux pumps. BMC Complement. Altern. Med. 14 (1), 1–8. doi: 10.1186/1472-6882-14-258
Turner A. M., Lee J. Y., Gorrie C. L., Howden B. P., Carter G. P. (2021). Genomic insights into last-line antimicrobial resistance in multidrug-resistant Staphylococcus and vancomycin-resistant Enterococcus. Front. Microbiol. 12, 637656. doi: 10.3389/fmicb.2021.637656
van Heel A. J., Montalban-Lopez M., Oliveau Q., Kuipers O. P. (2017). Genome-guided identification of novel head-to-tail cyclized antimicrobial peptides, exemplified by the discovery of pumilarin. Microb. Genomics 3 (10).
Varela M. F., Stephen J., Lekshmi M., Ojha M., Wenzel N., Sanford L. M., et al. (2021). Bacterial resistance to antimicrobial agents. Antibiotics 10, 5, 593. doi: 10.3390/antibiotics10050593
Wang L., Hu C., Shao L. (2017). The antimicrobial activity of nanoparticles: present situation and prospects for the future. Int. J. Nanomed., 1227–1249. doi: 10.2147/IJN.S121956
Waseem M., Naveed M., Rehman S. U., Makhdoom S. I., Aziz T., Alharbi M., et al. (2023). Molecular Characterization of spa, hld, fmhA, and l ukD Genes and Computational Modeling the Multidrug Resistance of Staphylococcus Species through Callindra harrisii Silver Nanoparticles. ACS Omega 8 (23), 20920–20936. doi: 10.1021/acsomega.3c01597
Wei X. Y., Xia W., Zhou T. (2022). Antibacterial activity and action mechanism of a novel chitosan oligosaccharide derivative against dominant spoilage bacteria isolated from shrimp Penaeus vannamei. Lett. Appl. Microbiol. 74 (2), 268–276. doi: 10.1111/lam.13596
Wolfensberger A., Kuster S. P., Marchesi M., Zbinden R., Hombach M. (2019). The effect of varying multidrug-resistence (MDR) definitions on rates of MDR gram-negative rods. Antimicrob. Resist. Infect. Control 8 (1), 1–9. doi: 10.1186/s13756-019-0614-3
Wright M. H., Matthews B., Arnold M. S. J., Greene A. C., Cock I. E. (2016). The prevention of fish spoilage by high antioxidant Australian culinary plants: Shewanella putrefaciens growth inhibition. Int. J. Food Sci. Technol. 51 (3), 801–813. doi: 10.1111/ijfs.13026
Xu Z., Li M., Li Y., Cao H., Miao L., Xu Z., et al. (2019). Native CRISPR-Cas-mediated genome editing enables dissecting and sensitizing clinical multidrug-resistant P. aeruginosa. Cell Rep. 29 (6), 1707–17. e3. doi: 10.1016/j.celrep.2019.10.006
Yadav H., Mahalvar A., Pradhan M., Yadav K., Sahu K. K., Yadav R. (2023). Exploring the potential of phytochemicals and nanomaterial: A boon to antimicrobial treatment. Med. Drug Discovery, 100151.
Yang C., Li H., Zhang T., Chu Y., Zuo J., Chen D. (2020). Study on antibiotic susceptibility of Salmonella typhimurium L forms to the third and forth generation cephalosporins. Sci. Rep. 10 (1), 3042.
Yang S.-C., Lin C.-H., Sung C. T., Fang J.-Y. (2014). Antibacterial activities of bacteriocins: application in foods and pharmaceuticals. Front. Microbiol. 5, 241. doi: 10.3389/fmicb.2014.00241
Yang Y., Qiu J.-G., Li Y., Di J.-M., Zhang W.-J., Jiang Q.-W., et al. (2016). Targeting ABCB1-mediated tumor multidrug resistance by CRISPR/Cas9-based genome editing. Am. J. Trans. Res. 8 (9), 3986.
Zhanel G. G., Sniezek G., Schweizer F., Zelenitsky S., Lagacé-Wiens P. R., Rubinstein E., et al. (2009). Ceftaroline: a novel broad-spectrum cephalosporin with activity against meticillin-resistant Staphylococcus aureus. Drugs 69, 809–831. doi: 10.2165/00003495-200969070-00003
Zhang Y., Zhao J., Han J., Fan Y., Xiong Z., Zou X., et al. (2022). Synergistic activity of imipenem in combination with ceftazidime/avibactam or avibactam against non-MBL-producing extensively drug-resistant Pseudomonas aeruginosa. Microbiol. Spectrum 10 (2), e02740–e02721. doi: 10.1128/spectrum.02740-21
Zimina M., Gazieva A., Pozo-Dengra J., Noskova S. Y., Prosekov A. Y. (2017). Determination of the intensity of bacteriocin production by strains of lactic acid bacteria and their effectiveness. Foods Raw Mater. 5 (1), 108–117. doi: 10.21179/2308-4057-2017-1-108-117
Keywords: multi-drug resistant bacteria, staphylococcus aureus, vancomycin-resistant enterococcus, medicinal plants, bacterial vaccines
Citation: Naveed M, Waseem M, Mahkdoom I, Ali N, Asif F, Hassan Ju and Jamil H (2024) Transient comparison of techniques to counter multi-drug resistant bacteria: prime modules in curation of bacterial infections. Front. Antibiot. 2:1309107. doi: 10.3389/frabi.2023.1309107
Received: 07 October 2023; Accepted: 04 December 2023;
Published: 26 January 2024.
Edited by:
Aref Shariati, Iran University of Medical Sciences, IranReviewed by:
Abid Ali, Texas A and M University, United StatesMajid Taati Moghadam, Gilan University of Medical Sciences, Iran
Copyright © 2024 Naveed, Waseem, Mahkdoom, Ali, Asif, Hassan and Jamil. This is an open-access article distributed under the terms of the Creative Commons Attribution License (CC BY). The use, distribution or reproduction in other forums is permitted, provided the original author(s) and the copyright owner(s) are credited and that the original publication in this journal is cited, in accordance with accepted academic practice. No use, distribution or reproduction is permitted which does not comply with these terms.
*Correspondence: Nouman Ali, Tm91bWFuLmFsaS5hbndhcjMzNkBnbWFpbC5jb20=