- 1Department of Biochemistry and Molecular Biology, Catholic University of Health and Allied Sciences, Mwanza, Tanzania
- 2Department of Clinical Microbiology, Kilimanjaro Christian Medical Centre, Moshi, Tanzania
- 3Department of Microbiology and Immunology, Weill Bugando School of Medicine, Catholic University of Health and Allied Sciences, Mwanza, Tanzania
- 4School of Medicine, University of St Andrews, St Andrews, United Kingdom
Background: Additional antimicrobial resistance to extended-spectrum β-lactamase (ESBL)-producing E. coli exhausts treatment options. We investigated allele distribution and resistance to ciprofloxacin and gentamicin among ESBL-producing E. coli isolates from the urine, stool, animals, and environments of presumptive urinary tract infection (UTI) patients, in order to gain a crucial insight toward devising prevention and control measures and treatment guidelines.
Methods: Archived ESBL-producing E. coli isolates from the urine, stool, animals, and surrounding environments of presumptive UTI patients were retrieved. Antimicrobial susceptibility profiles for ciprofloxacin and gentamicin were done followed by multiplex Polymerase chain reaction (PCR) for blaCTX-M, blaTEM, and blaSHV, to determine ESBL allele distribution. Data were analyzed using STATA version 17.
Results: A total of 472 confirmed ESBL-producing E. coli isolates from Mwanza 243 (51.5%), Kilimanjaro 143 (30.3%), and Mbeya 86 (18.2%) were analyzed. Of these, 75 (15.9%) were from urine, 199 (42.2%) from stool, 58 (12.3%) from rectal/cloaca swabs of animals, and 140 (29.7%) from surrounding environments. Out of the 472 ESBL-producing E. coli, 98.9% (467) had at least one ESBL allele. The most frequent allele was blaCTX-M, which was detected in 88.1% (416/472) of isolates, followed by the blaTEM allele, which was detected in 51.5% (243/472) of isolates. A total of 40.7% (192/472) of isolates harbored dual blaCTX-M + blaTEMalleles and only 0.2% (1/472) of isolates had dual blaCTX-M + blaSHValleles, whereas 2.3% (11/472) of isolates had a combination of all three alleles (blaCTX-M + blaTEM + blaSHV). None of the isolates harbored a combination of blaTEM + blaSHVonly. Resistance to ciprofloxacin and gentamicin was observed in 70.8% (334/472) and 46.0% (217/472) of isolates, respectively. There was a significant difference in the distribution of resistance to ciprofloxacin as well as gentamicin among ESBL-producing E. coli isolated from various sources (p-value < 0.001 and 0.002, respectively).
Conclusion: Almost all ESBL-producing E. coli isolates carry blaCTX-M, blaTEM, and blaSHV either alone or in combination, with the most common allele being blaCTX-M.The resistance to ciprofloxacin and gentamicin, which are frontline antibiotics for UTIs among ESBL-producing E. coli, is high. This implies the need to continually revise the local guidelines used for optimal empirical therapy for UTIs, and for continual research and surveillance using one health approach.
Introduction
Escherichia coli is the main causative pathogen for urinary tract infections (UTIs) and has the greatest potential to acquire extended-spectrum β-lactamases (ESBLs) (Ahmed et al., 2015; Ayoyi et al., 2017; Karikari et al., 2021). The dissemination of ESBL-producing E. coli poses a significant public health threat, as the antibiotic resistance associated with it limits treatment options and challenges health systems (Jasser, 2006).
ESBLs comprise many plasmid-mediated derivatives such as blaTEM, blaOXA, blaSHV, and blaCTX-M (Nicolas-Chanoine et al., 2008; Peirano and Pitout, 2010). However, blaCTX-M has been the predominant ESBL allele worldwide (Cantón and Coque, 2006), including in Tanzania (Mshana et al., 2016; Seni et al., 2016). This group of ESBLs is associated with an extensive pattern of antimicrobial resistance to many antibiotics, including β-lactam agents such as penicillins, cephalosporins, monobactams, and carbapenems (Paterson and Bonomo, 2005; Rogers et al., 2011; Accogli et al., 2014; Cai et al., 2014; Johnson et al., 2015). In addition, over the last two decades ESBL-producing E. coli isolates have demonstrated an increased level of dual resistance to other frontline antibiotics such as aminoglycosides and fluoroquinolones (Meier et al., 2011; Mshana et al., 2011; Rogers et al., 2011). Several surveillance studies across Europe, North America, and South America have reported resistance to these antibiotics, ranging from 20% to 45% among uropathogenic E. coli isolates (Foxaman, 2010; Croxall et al., 2011). As most of these antibiotics are used to treat uncomplicated UTIs and complicated UTIs, which are the leading cause of increased UTI-related hospital visits, this increasing level of antimicrobial resistance of ESBL-producing E. coli to frontline antibiotics is of great concern. It threatens health systems by limiting the therapeutic choices used for treating UTIs and highlights the growing threat of the emergence of pan-drug resistance in ESBL-producing E. coli (Meier et al., 2011). At the time of writing treatment of UTIs is frequently initiated empirically (based on the standard treatment guideline), of which ciprofloxacin and gentamicin are recommended in (The United Republic of Tanzania Ministry of Health and Social Welfare, 2021). Having prior information regarding antimicrobial susceptibility profiles to frontline antimicrobial drugs for common causative pathogens, such as ESBL-producing E. coli in a particular setting, is essential to achieving the most effective empirical therapy as it will provide clinicians with the information required to facilitate the effective treatment and management of UTI patients (Dias Neto et al., 2003; Farajnia et al., 2009).
UTIs are among the most common bacterial infections acquired in community and hospital settings (Foxaman, 2010; Murray et al., 2022), and they are a main cause of hospital admissions that are associated with high morbidity, mortality, and economic costs (Gonzalez and Schaeffer, 1999; Foxman, 2002; Cove Smith and Almond, 2007; Murray et al., 2022). Pathogens causing UTIs can be acquired either endogenously or exogenously (Ayoyi et al., 2017), with about 87.0% of UTIs being endogenously acquired (Nielsen et al., 2014; Tandogdu and Wagenlehner, 2016). In addition, E. coli colonizing the gastrointestinal tract of humans and animals is described as being the main source of UTIs (Monstein et al., 2007; Jakobsen et al., 2012; Nielsen et al., 2014). Previous studies done in Tanzania reported the prevalence of ESBL-producing E. coli colonizing the gastrointestinal tracts of the adult population and animals in the community to be 16.5% and 20.8%, respectively (Mshana et al., 2016; Seni et al., 2016). Furthermore, in Tanzania, the evidence of ESBL-producing E. coli contaminating household latrines has been reported to be at 8.7% (Erb et al., 2018). It is also known that the distribution of ESBL alleles and their antibiotic susceptibility profiles, particularly to non β-lactam antibiotics, differ regionally (Mathai et al., 2001; Farrell et al., 2003). With this note, evaluation of common local ESBL allele distribution of E. coli strains circulating in the community among patients, their domesticated and farm animals, and surrounding environments is crucial in devising strategies to curb the spread of ESBL-producing E. coli. Therefore, this study investigated the allele distribution and antimicrobial resistance patterns of ciprofloxacin and gentamicin among ESBL-producing E. coli isolates from the urine and stool of presumptive UTI patients, their domesticated and farm animals, and their surrounding environments.
Materials and methods
Study design, period, and population
This was a laboratory-based cross-sectional study that utilized a total of 472 ESBL-producing E. coli isolates, which were selected from Gram-negative bacteria archived during the implementation of the Holistic Approach To Unravel Antibacterial resistance (HATUA) project. The HATUA project was conducted in three countries—Kenya, Tanzania, and Uganda—for the period of February 2019 to September 2020 (Asiimwe et al., 2021). In Tanzania, the HATUA project enrolled presumptive UTI patients selected from 10 health facilities in three regions (Mwanza, Mbeya, and Kilimanjaro). The health facilities included Kilimanjaro Christian Medical Center (KCMC), Kibosho District Designated Hospital, and Majengo Health Center for the Kilimanjaro region; Bugando Medical Center, Sekou-Toure Regional Hospital, Nyamagana District Hospital, Sengerema Designated District Hospital, and Makongoro Health Center for the Mwanza region; and Mbeya Regional Referral Hospital and Ifisi Designated District Hospital for the Mbeya region. Out of 472 isolates, 75 were from urine and 199 from stools of these presumptive patients, 58 were from rectal/cloaca swabs of their domesticated and farm animals (dogs, chickens, goats, cows, pigs, ducks, cats, and rabbits), and 140 were from the surrounding environments (bathrooms, toilets, and waste bins or dumping pits) of these presumptive UTI patients.
Data collection
The information related to ESBL-producing E. coli isolates from study participants was retrieved from the pre-existing database of the HATUA project.
Laboratory procedures and methods
Isolates recovery
The isolates were taken from cryovials, containing brain–heart infusion broth with 20% glycerol, stored in –80°C freezers. The isolates were then subcultured on sheep blood agar and incubated aerobically at 37°C for 18 to 24 h.
Antibiotic susceptibility testing
The resistance phenotypes for ESBL-producing E. coli from urine, stool, rectal swabs of animals, and environments were captured from the existing database of the HATUA project. Ciprofloxacin and gentamicin were tested using an agar dilution method for ESBL-producing E. coli originating from the environment and animals only. The agar dilution methods were determined according to established standard operating protocols based on methodology from the (Clinical and Laboratory Standards Institute, 2019). For gentamicin, the stock solution concentration of 40 mg/mL (40 µL) was incorporated into 1,000 mL of Mueller–Hinton media to make a final concentration of 8 µg/mL, while 10 mg/mL (50 µl) of stock solution of ciprofloxacin was incorporated into 1,000 mL of Mueller–Hinton media to make a final concentration of 0.5 µg/mL. The samples were inoculated onto media containing antibiotics and finally incubated at 37°C for 18 to 24 h.
DNA extraction
The boil lysate technique was used to extract bacterial DNA, as previously reported with a slight modification (Minja et al., 2021). Briefly, two colonies of overnight growth of bacteria from Mueller–Hinton agar were suspended into DNAse-free water, and, thereafter, mixed by vortexing and then boiled at 100°C in a water bath for 15 min. Tubes were centrifuged at 12,000 rpm for 10 min. The quality of DNA was determined using gel electrophoresis, whereas quantity was determined using Qubit®. Thereafter, 100 µL of the supernatant (DNA rich) was aliquoted into Eppendorf tubes for storage at –20°C for further PCR amplification and detection of ESBL alleles (blaCTX-M, blaTEM, and blaSHV).
Multiplex PCR amplification for detection of extended-spectrum β-lactamase alleles
A multiplex PCR was performed on a thermal cycler machine (T100™; Bio-Rad, Singapore) to amplify ESBL alleles (blaCTX-M, blaTEM, and blaSHV) using specific primers, as previous reported (Monstein et al., 2007) (Table 1). Briefly, 2 µL (≈ 30 ng) of DNA samples was added into PCR plates containing 12.5 µL of readily reconstituted master-mix (New England Biolabs) with 0.5 µL (500 µg) of each primer and then PCR water was added to make a final volume of 25 µL for the reaction mixture. Amplification conditions included an initial denaturation at 95°C for 15 min followed by 30 cycles of denaturation at 94°C for 30 s, annealing at 60°C for 40 s, and elongation at 72°C for 2 min. Then, a final elongation at 72°C for 10 min completed the process (Monstein et al., 2007).
Gel electrophoresis
The PCR products were visualized under UV illumination via gel electrophoresis using 1.5% ultrapure agarose gel (Thermo Fisher Scientific, UK) with a Tri-acetate-EDTA (TAE) buffer. Staining of the DNA fragments was carried out using Safe-Red dye (Safeview™Classic). The gels were run at 80 V for approximately 45 min. Standard DNA molecular weight markers were used: a 100-bp ladder. The ladder was visualized under UV light.
Quality control
Klebsiella pneumonia ATCC 700603, E. coli ATCC 35218, and a clinical isolate of non-ESBL-producing E. coli were used as control strains. These control strains were used to check the performance of used media and antibiotic discs, as well as multiplex PCR experiments for the amplification and detection of ESBL alleles.
Data management and statistical analysis
Data from isolates, such as identification number, isolate name, source of isolation, antimicrobial resistance pattern, and ESBL allele after PCR, were recorded in the logbook and then entered into the computer using Microsoft Excel® 2018 (Microsoft Corporation, Redmond, WA, USA). Data were imported into STATA software version 17 (StataCorp, College Station, TX, USA) for analysis. Categorical variables were summarized using frequency and proportion (percent). To compare the difference in the proportion of distribution of resistance with ciprofloxacin and gentamicin we used a one-tailed two-sample proportion test. To determine the significance of the difference in the distribution of ciprofloxacin resistance as well as gentamicin resistance across various ESBL alleles and sources of E. coli isolation, we used Pearson’s chi-squared test or Fisher’s exact test where appropriate. In all analyses, the significance level was set at a p-value < 0.05.
Ethics clearance
The study received ethics approval from the University of St Andrews, St Andrews, UK (No. MD14548, 10/09/19); the National Institute for Medical Research, Tanzania (No. 2831, updated 26/07/19); the Mbeya Medical Research and Ethics Committee (No. SZEC-2439/R.A/V.1/30); the Kilimanjaro Christian Medical College, Tanzania (No. 2293, updated 14/08/19); and the CUHAS/BMC Research Ethics and Review Committee (No. CREC/266/2018, updated on 02/2019).
Results
Isolates distribution
A total of 472 confirmed ESBL-producing E. coli isolates were retrieved for this study. These isolates were from Mwanza [243 (51.5%)], Kilimanjaro [143 (30.3%)], and Mbeya [86 (18.2%)]. Of these 472 isolates, 75 (15.9%) were from the urine of presumptive UTI patients, 199 (42.2%) were from the stool of these patients, 140 (29.7%) were from the surrounding environments, and 58 (12.3%) were from the domesticated and farm animals of these patients (Table 2).
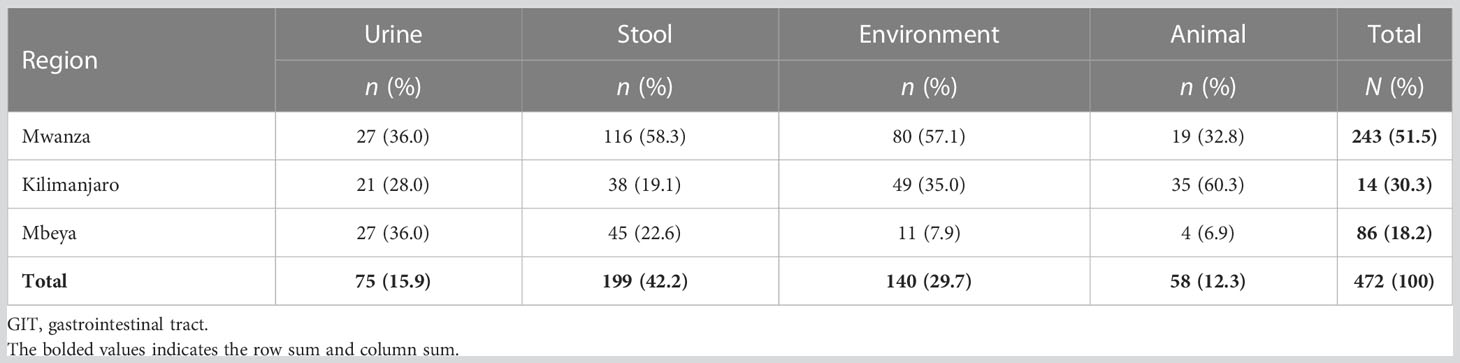
Table 2 Distribution of 472 extended-spectrum β-lactamase (ESBL)-producing Escherichia coli isolates, by region and source.
Distribution of extended-spectrum β-lactamase alleles among extended-spectrum β-lactamase-producing E. coli
Of the 472 phenotypically confirmed ESBL-producing E. coli, 98.9% (467) had at least one ESBL allele, and only five (1.1%) were negative for the tested ESBL alleles (blaCTX-M, blaTEM, and blaSHV). The most predominant allele was blaCTX-M, which was detected in 88.1% (416/472) of isolates, followed by blaTEM and blaSHV alleles, which were detected in 51.5% (243/472) and 4.9% (23/472) of isolates, respectively. A total of 55.7% (263/472) of isolates (212 with blaCTX-M, 40 with blaTEM, and 11 with blaSHV) harbored only one allele. A total of 40.7% (192/472) of isolates harbored blaCTX-M + blaTEMalleles, and only one (0.2%) isolate harbored blaCTX-M + blaSHValleles, whereas 2.3% (11/472) of isolates harbored a combination of all three alleles investigated (blaCTX-M + blaTEM + blaSHV). Of note, none of the isolates harbored a dual combination of blaTEM + blaSHV (Figure 1 and Table 3). All the positive ESBL alleles investigated showed a band on the gel electrophoresis following multiplex PCR amplification for the detection of ESBL alleles (blaCTX-M, blaTEM, and blaSHV) (Figure 2).
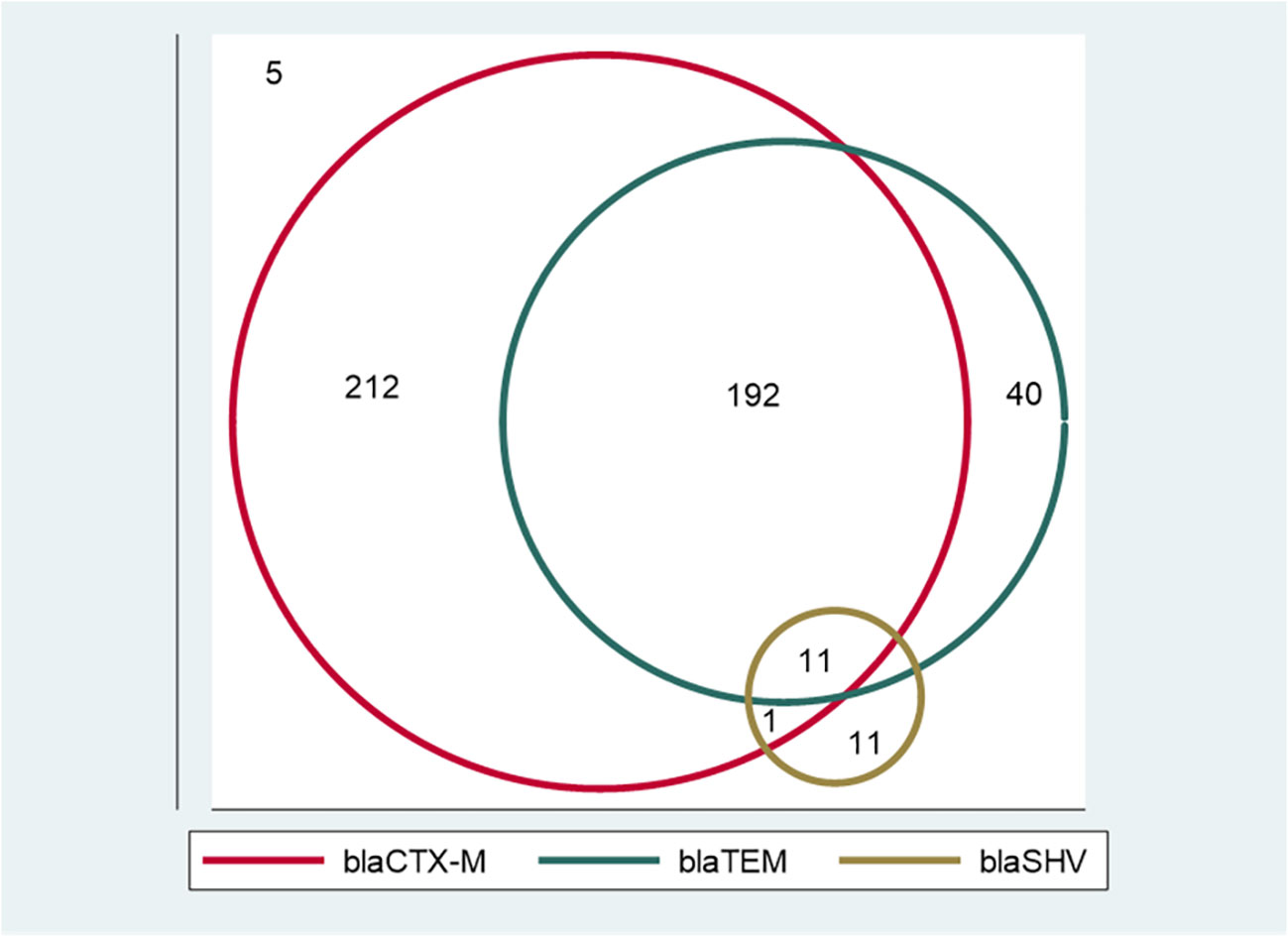
Figure 1 A Venn diagram showing the distribution of extended-spectrum β-lactamase (ESBL) alleles among ESBL-producing E. coli. Isolates with only one ESBL allele: 212 with blaCTX-M, 40 with blaTEM, and 11 with blaSHV. Isolates with dual ESBL alleles: 192 with blaCTX-M + blaTEM, and one with blaCTX-M + blaSHV. There were 11 isolates that had all three alleles (blaCTX-M + blaTEM + blaSHV). None of the isolates harbored a dual combination of blaTEM + blaSHV alleles. Five isolates did not harbor any of the tested ESBL alleles.
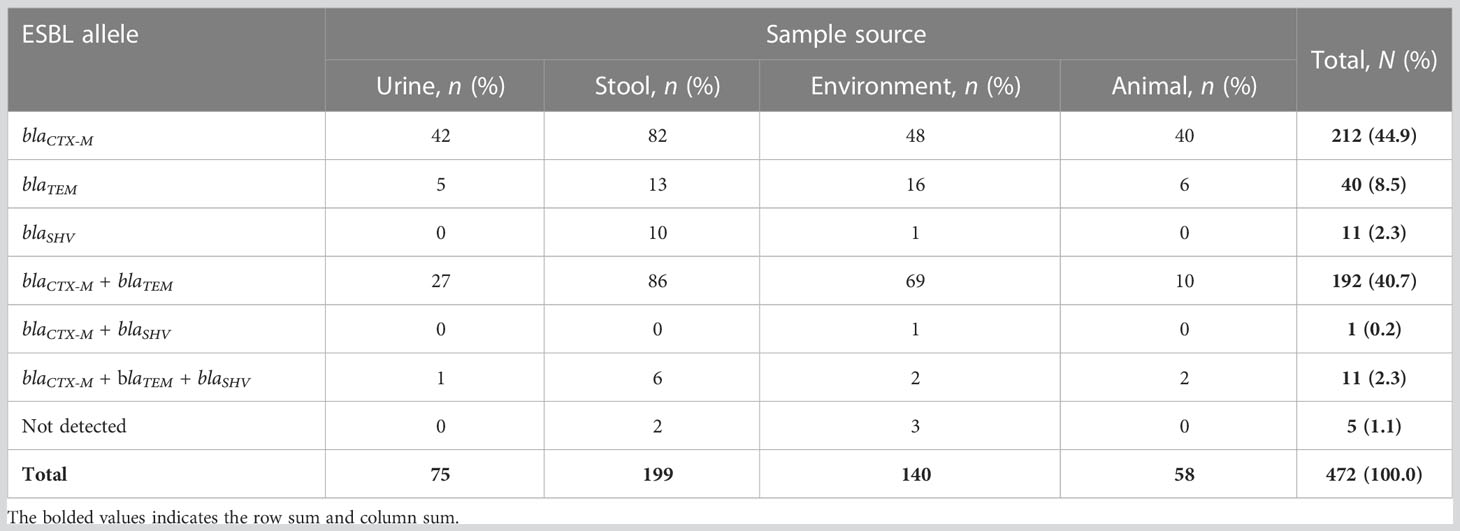
Table 3 Distribution of extended-spectrum β-lactamase (ESBL) alleles, by source of isolation, among 472 study Escherichia coli isolates.
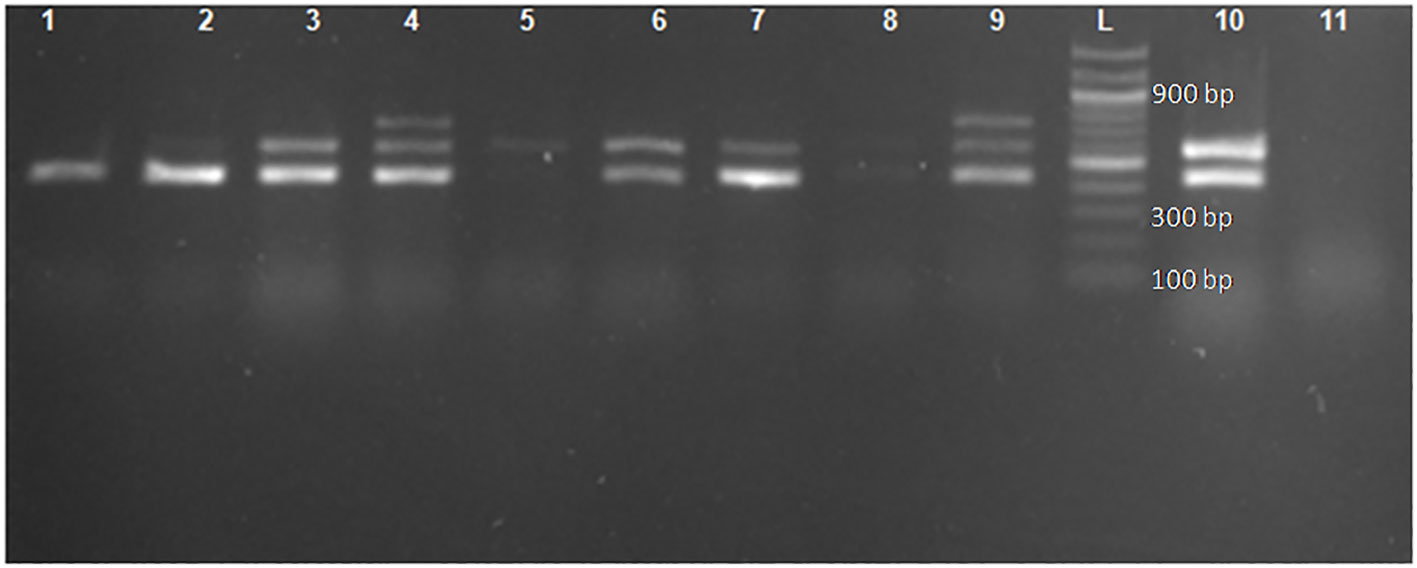
Figure 2 Gel image for the detection of genes encoding blaCTX-M, blaTEM, and blaSHV following multiplex PCR. Lane L: ladder 100 bp (New England Biolabs). Lane 2: the 445-bp PCR product of blaTEM. Lanes 3, 6, 7, and 10: the 445-bp and 593-bp PCR product of blaTEM and blaCTX-M, respectively. Lanes 4 and 9: the 445-bp, 593-bp, and 747-bp PCR products of blaTEM, blaCTX-M, and blaSHV, respectively. Lane 1: positive control blaTEM (E. coli ATCC 35218). Lane 11: negative control (E. coli clinical isolate non-ESBL).
Phenotypic antimicrobial resistance to ciprofloxacin and gentamicin
Out of 472 ESBL-producing E. coli, resistance to ciprofloxacin and gentamicin was observed in 70.8% (334) and 46.0% (217) of isolates, respectively. The resistance to ciprofloxacin was significantly higher than that of gentamicin (p-value < 0.001; two-sample proportion test). Of note, 37.5% (177) of isolates were resistant to both antibiotics, whereas 20.8% (98) were sensitive to both drugs. Resistance to ciprofloxacin and gentamicin was highest for the isolates from urine, that is, 89.3% (67/75) and 56.0% (42/75), respectively. This was followed by the isolates originating from stool (fecal carriage), with 84.4% (168/199) for ciprofloxacin, and 55.0% (77/140) for isolates from the surrounding environments for gentamicin. There was a significant difference in the distribution of resistance to ciprofloxacin as well as to gentamicin across various sources of ESBL-producing E. coli (p-value < 0.001 and 0.002, respectively; Pearson’s chi-squared test). The lowest resistance to ciprofloxacin was observed in ESBL-producing E. coli originating from animals [31.0% (18/58)], whereas the lowest resistance to gentamicin was observed in isolates originating from stool samples [73/199 (36.7%)]. There was no significant difference in the distribution of ciprofloxacin and gentamicin resistance between various ESBL alleles (p-values 0.062 and 0.962, respectively; Fisher’s exact test) (Tables 4, 5). Of note is that more than half [53.3% (40/75)] of isolates from the urine of presumptive UTI patients had dual resistance to ciprofloxacin and gentamicin, and this was significantly more than from other sources (p-value 0.003; Pearson’s chi-squared test) (Table 6).
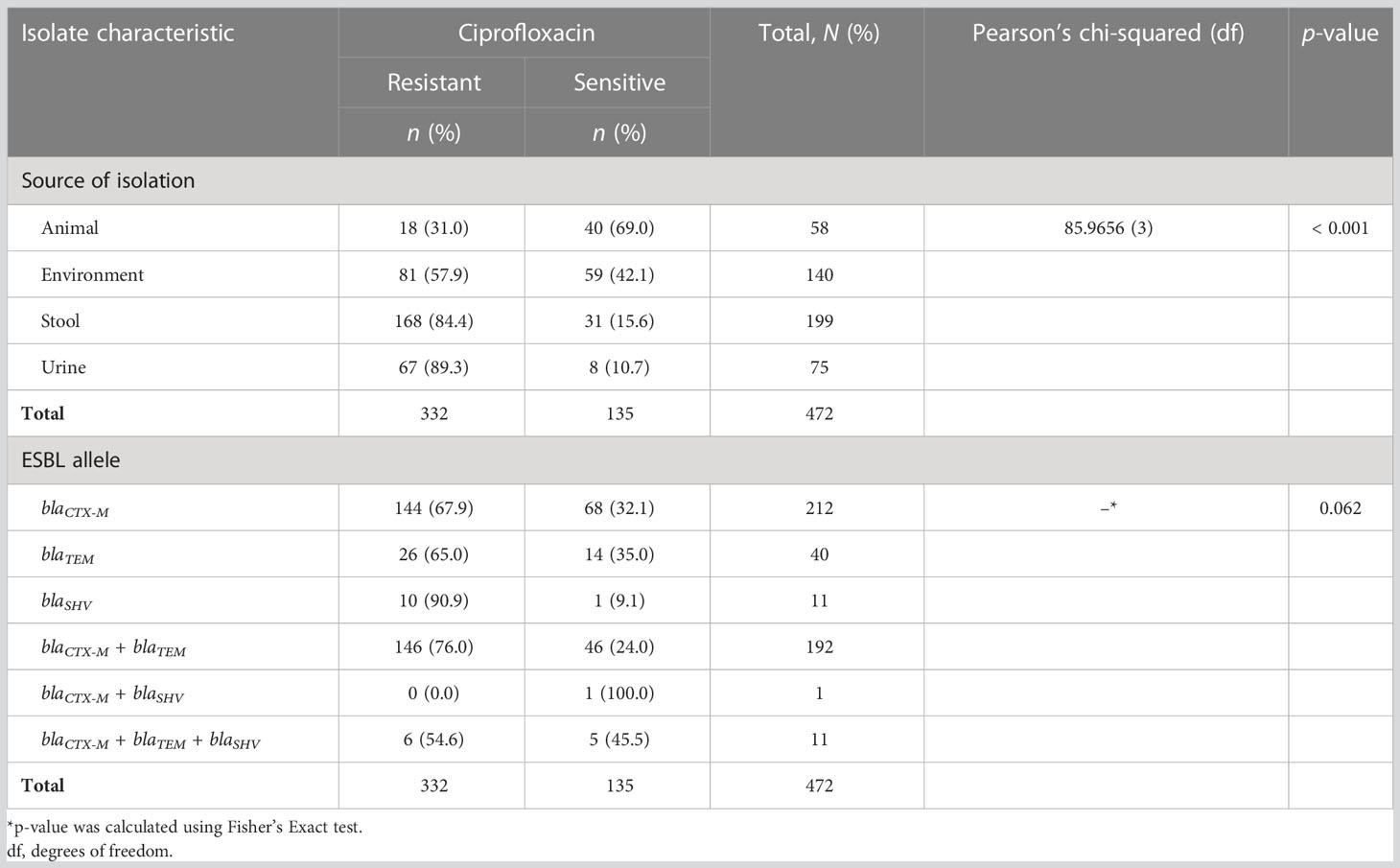
Table 4 Distribution of ciprofloxacin resistance among various sources of isolation and extended-spectrum β-lactamase (ESBL) alleles.
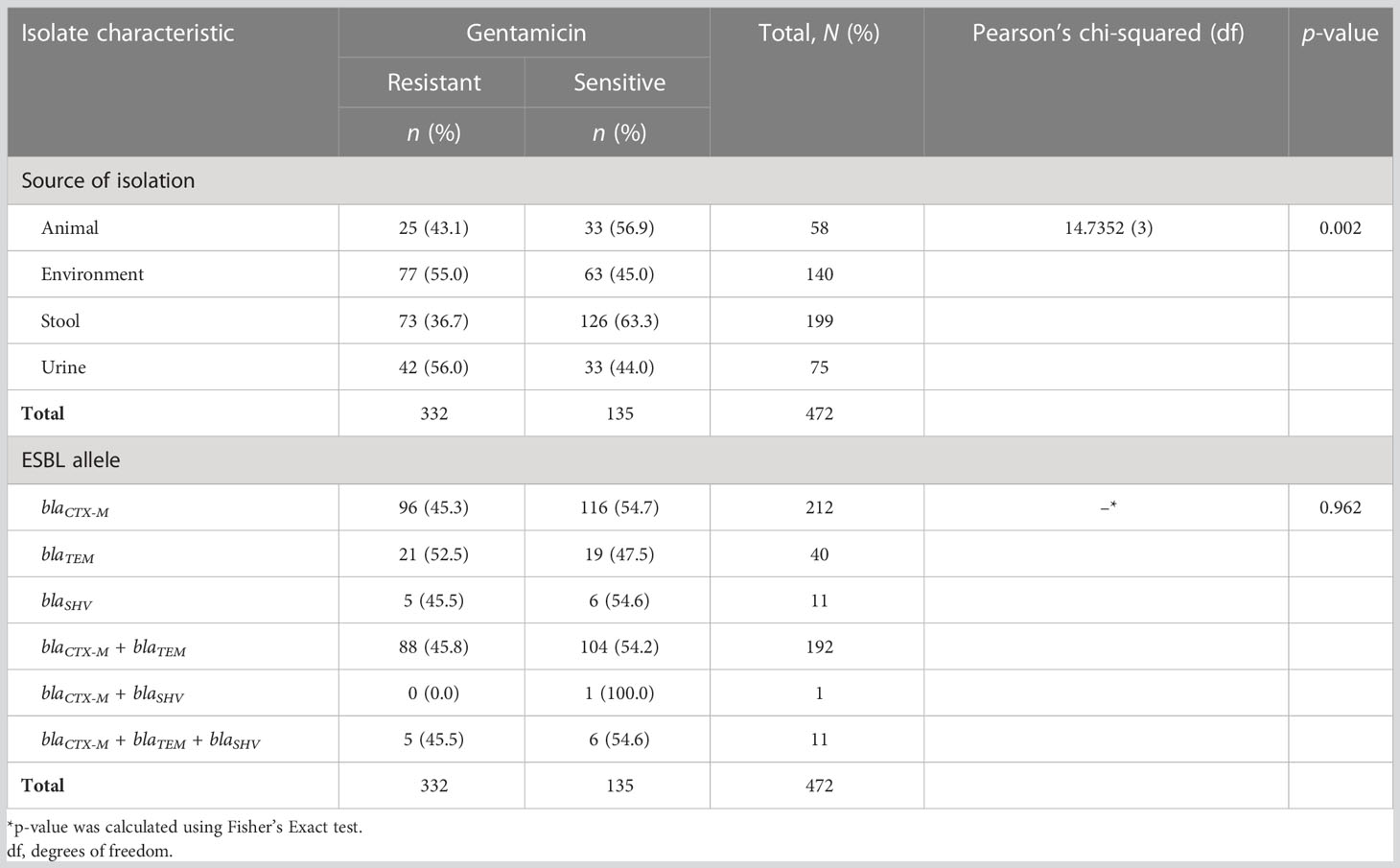
Table 5 Distribution of gentamicin resistance among various sources of isolation and extended-spectrum β-lactamase (ESBL) alleles.
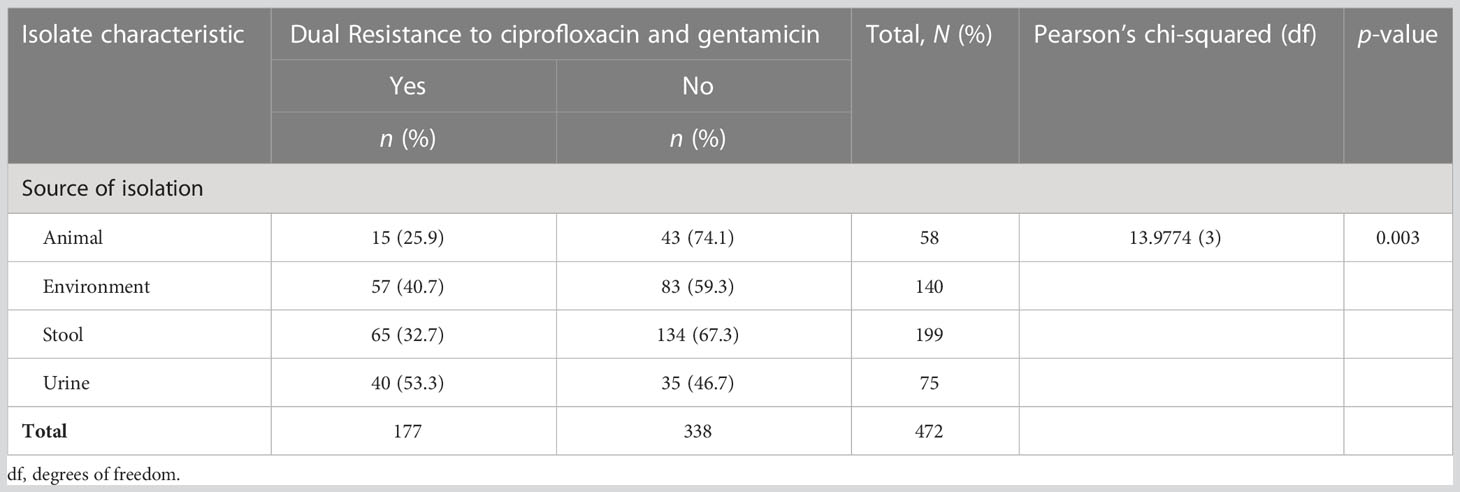
Table 6 Distribution of extended-spectrum β-lactamase (ESBL) isolates with dual resistance to ciprofloxacin and gentamicin by source of isolation.
Discussion
The findings from this study reveal that almost all studied ESBL-producing E. coli from the urine and stool (fecal carriage) of presumptive UTI patients, their domesticated and farm animals, and their surrounding environments possess blaCTX-M, blaTEM, and blaSHV either alone or in combination, with the most common allele being blaCTX-M and the most predominant dual combination of alleles being blaCTX-M + blaTEM. Furthermore, the resistance of ESBL-producing E. coli to frontline antibiotics, ciprofloxacin, and gentamicin, which are currently used to treat UTIs, was high. This emphasizes the need to continually revise the local guidelines used for optimal empirical therapy for UTIs, for proper control methods, and for further research to combat antibiotic resistance.
The findings from this study show that 98.9% of all ESBL-producing E. coli investigated had at least one ESBL allele tested (blaCTX-M, blaTEM, or blaSHV), either as one allele or in a combination of two or three of these ESBL alleles. Only 1.1% of the isolates were negative for the ESBL alleles investigated. These isolates could be more likely harboring other ESBL alleles, such as blaOXA, which was not tested in this study but has been reported elsewhere around the world including Tanzania. Nonetheless, it has been reported to have a very low prevalence in our settings (Marando et al., 2018; Abrar et al., 2019; Onduru et al., 2021). Our finding tallies with the findings from a similar study that reported that 3.4% of ESBL-producing isolates investigated were negative for these ESBL alleles (blaCTX-M, blaTEM, and blaSHV) (Mirkalantari et al., 2020). We investigated three ESBL alleles (blaCTX-M, blaTEM, and blaSHV), as they are the most common ESBL alleles circulating in community and hospital settings in Eastern, Central, and Southern African countries (Onduru et al., 2021). Our findings are in line with previous studies that showed that almost all of the ESBL-producing E. coli is driven by the blaCTX-M, blaTEM, and blaSHValleles (Mshana et al., 2011; Mshana et al., 2016; Moremi et al., 2017; Marando et al., 2018; Mirkalantari et al., 2020; Kimera et al., 2021; Onduru et al., 2021). Our finding that blaCTX-Mis the most common allele followed by blaTEM is supported by many previous and recent studies conducted among isolates from environments, animals, patients, and human carriers, which report the predominance of the blaCTX-Mallele to range from 76.5% to 100% (Moremi et al., 2017; Marando et al., 2018; Abrar et al., 2019; Mirkalantari et al., 2020; Kimera et al., 2021; Onduru et al., 2021). This predominance can be explained by the fact that the conjugative plasmid-carrying blaCTX-Mallele is highly effective at being transferred and has been reported as the most frequently and successfully transferred allele through horizontal gene transfer (Cantón and Coque, 2006; Mshana et al., 2009; Minja et al., 2021). The spread of blaCTX-Mallele is causing rapid, important, and unpredictable changes in the epidemiology of antibiotic resistance.
The most common combination of two alleles was blaCTX-M + blaTEM at 40.7%; the next most common combination was blaCTX-M + blaSHV, with a distant 0.2%. Similarly, the occurrence of dual ESBL alleles in the genes was common elsewhere, with the combination of blaCTX-M + blaTEM alleles being the most common (Sah et al., 2021; Silago et al., 2021). These genes are often present in large plasmids and are capable of conferring resistance to the organisms (Lee et al., 2012). Acquisition and transferability of ESBL genes are of particular importance, as these ESBL-encoding genes are often located in promiscuous plasmids (van Duijkeren et al., 2018). This property of ESBL genes enables their exchange between bacteria, and favors transmission between animals and humans (Brolund and Sandegren, 2016). For this reason, the distribution for all combinations of ESBL alleles was expected. Surprisingly, in our study there was no ESBL-producing E. coli with a combination of blaTEM + blaSHV. Furthermore, we observed only 2.3% of the ESBL-producing E. coli isolates with all three alleles (blaCTX-M, blaTEM, and blaSHV). These findings raise the thought of a genetic preponderance of order and dynamics in the combination, transmission, and acquisition of these genes for ESBL production. Further genome-wide studies are warranted to unravel the plausibility of this hypothesis.
Ciprofloxacin and gentamicin are frontline antimicrobials used to treat UTIs. Extra resistance to frontline non-β-lactam antibiotics, such as ciprofloxacin and gentamicin, to ESBL-producing E. coli, limits the therapeutic options to treat UTIs. Our findings that 70.8% and 46.0% of ESBL-producing E. coli are resistant to ciprofloxacin and gentamicin, respectively, are alarming. This result is similar to studies done among animals, street children, and patients in Dar es Salaam and Mwanza in Tanzania (Seni et al., 2016; Manyahi et al., 2017; Moremi et al., 2017; Kimera et al., 2021). This high prevalence is explained by the fact that these antibiotics are used as frontliners to treat uncomplicated and complicated UTIs, complicated UTIs being the leading causes of UTI-related increases in hospital visits. This increasing level of antimicrobial resistance of ESBL-producing E. coli to frontline antibiotics is of great concern as it highlights the growing threat of the emergence of pan-drug resistance in ESBL-producing E. coli (Meier et al., 2011).
Furthermore, the prevalence of ciprofloxacin and gentamicin resistance among ESBL E. coli is higher than the reported prevalence of ciprofloxacin and gentamicin reported in non-ESBL E. coli in our setting, which ranges from 48.6% to 62.7% and from 14.4% to 17% for ciprofloxacin and gentamicin, respectively (Msanga et al., 2022; Mtemisika et al., 2022). This relatively high resistance rate to fluroquinolones and aminoglycosides to ESBL-producing bacteria compared with non-ESBL-producing bacteria might be due to the co-existence of the ESBL genes with those conferring resistance to fluroquinolones and aminoglycosides in the same large plasmid (Lee et al., 2012). In this study, more than one-third of the isolates were resistant to both ciprofloxacin and gentamicin; this is worrisome as it limits treatment options in the case of acute pyelonephritis and urosepsis, as per standard treatment guidelines in the study setting. This could be due to the presence of the plasmid-mediated genes such as aac (6′)-Ib-cr, which encodes aminoglycoside acetyltransferase that induces resistance against aminoglycosides and fluoroquinolones simultaneously (Rasoulinasab et al., 2021).
The observed levels of high resistance to ciprofloxacin compared with gentamicin could be attributed to the fact that ciprofloxacin is an orally administered antibiotic and is among the more easily obtained over-the-counter antibiotics, whereas gentamicin is administered via injection, which therefore prevents it from being misused by non-health personnel. The treatment of UTIs is frequently initiated empirically; if a patient has ESBL-producing E. coli, ciprofloxacin and gentamicin will be more likely to end up with treatment failure. Further studies to assess other non-beta lactam antibiotics to treat ESBL-producing E. coli are warranted in achieving the most effective empirical therapy, as they will provide clinicians with the information required to facilitate the effective treatment and management of UTI patients (Dias Neto et al., 2003; Farajnia et al., 2009).
We found significant differences in the distribution of ciprofloxacin resistance across various sources of isolation of the ESBL-producing E. coli. The highest resistance to ciprofloxacin was observed from ESBL-producing E. coli isolated from the urine and stool of presumptive UTI patients, as well as from isolates from the environment as compared with the isolates from the animals. Our finding tallies with other studies in Africa that reported ciprofloxacin resistance among ESBL-producing isolates from humans to range from 46.3% to 85.5% (Moyo et al., 2010; Meier et al., 2011; Mirkalantari et al., 2020). This finding could be attributed to the fact that ciprofloxacin is more commonly used as the frontline drug to treat UTIs in humans than in animals. In addition, these presumptive UTI patients could have a reckless habit of contaminating their environment via their urine and stools. On the other hand, we found a significant difference in the distribution of gentamicin resistance across various sources of isolates of ESBL-producing E. coli. The highest level of resistance to gentamicin was observed from ESBL-producing E. coli isolated from the urine of presumptive UTI patients followed by those isolates from the environment, as compared with the isolates from the stool of patients with presumptive UTI (fecal carriage). Studies that compare the significance of the difference in the distribution of gentamicin as well as ciprofloxacin resistance among ESBL-producing E. coli in Africa are limited. However, our prevalence of gentamicin resistance for ESBL-producing E. coli from urine is similar to a study done in Dar Es Salaam, Tanzania (Manyahi et al., 2017). This finding could be attributed to the fact that gentamicin is mainly used for the treatment of UTIs and urosepsis and its use for domesticated and farm animals is less than that for humans; hence, resistance to isolates from stool is minimal.
In conclusion, almost all ESBL-producing E. coli isolates from urine and stool of presumptive patients of UTI, their animals, and their environment harbor blaCTX-M, blaTEM, and blaSHV either alone or in combination, with the most common allele being blaCTX-M. The most common allele combination was blaCTX-M + blaTEM. Higher resistance of ESBL-producing E. coli to current frontline antibiotics (ciprofloxacin and gentamicin) to treat UTIs than in non-ESBL-producing isolates emphasizes the need to continually revise the local guidelines used for optimal empirical therapy for UTIs and it calls for coordinated efforts to address the growing ESBL predicament. Further genome-wide studies are warranted to unravel the genetic dynamics and interplay in the transmission and acquisition of ESBL genes.
Data availability statement
The raw data supporting the conclusions of this article will be made available by the authors, without undue reservation.
Ethics statement
The studies involving human participants were reviewed and approved by the Joint Catholic University of Health and Allied Sciences – Bugando Medical Center Research and Ethics Committee. The patients/participants provided their written informed consent to participate in this study. The animal study was reviewed and approved by the Joint Catholic University of Health and Allied Sciences – Bugando Medical Center Research and Ethics Committee. Written informed consent was obtained from the owners for the participation of their animals in this study.
Author contributions
AM, CM, BK, and SM designed the study. AM, CM, and MM carried out the experiments. BK, CM, and MM analyzed the results. AM, CM, and BK wrote the manuscript. WS, AS, MH, MM, SM, and BK critically reviewed the manuscript. All authors contributed to the article and approved the submitted version.
Funding
This study was funded by the Holistic Approach to Unravel Antibacterial Resistance in East Africa (HATUA) project funded by the National Institute for Health Research, Medical Research Council, and the Department of Health and Social Care, Award (MR/S004785/1). The funders had no role in study design, data collection and analysis, decision to publish, or preparation of the manuscript.
Conflict of interest
The authors BK, WS, and SM declare that they were editorial board members of Frontiers at the time of submission. This had no impact on the peer review process and the final decision.
The remaining authors declare that the research was conducted in the absence of any commercial or financial relationships that could be construed as a potential conflict of interest.
Publisher’s note
All claims expressed in this article are solely those of the authors and do not necessarily represent those of their affiliated organizations, or those of the publisher, the editors and the reviewers. Any product that may be evaluated in this article, or claim that may be made by its manufacturer, is not guaranteed or endorsed by the publisher.
References
Abrar S., Ain N. U., Liaqat H., Hussain S., Rasheed F., Riaz S. (2019). Distribution of blaCTX - M , blaTEM , blaSHV and blaOXA genes in extended-spectrum-β-lactamase-producing clinical isolates: a three-year multi-center study from Lahore, Pakistan. Antimicrob. Resist. Infect. Control. 8, 80. doi: 10.1186/s13756-019-0536-0
Accogli M., Giani T., Monaco M., Giufrè M., García-Fernández A., Conte V., et al. (2014). Emergence of Escherichia coli ST131 sub-clone H30 producing VIM-1 and KPC-3 carbapenemases, Italy. J. Antimicrob. Chemother. 69 (8), 2293–2296. doi: 10.1093/jac/dku132
Ahmed M., Moremi N., Mirambo M. M., Hokororo A., Mushi M. F., Seni J., et al. (2015). Multi-resistant gram negative enteric bacteria causing urinary tract infection among malnourished underfives admitted at a tertiary hospital, northwestern, Tanzania. Ital. J. Pediatrics. 41 (1), 1–5. doi: 10.1186/s13052-015-0151-5
Asiimwe B. B., Kiiru J., Mshana S. E., Neema S., Keenan K., Kesby M., et al. (2021). Protocol for an interdisciplinary cross-sectional study investigating the social, biological and community-level drivers of antimicrobial resistance (AMR)Holistic approach to unravel antibacterial resistance in East Africa (HATUA). BMJ Open 11 (3), e041418. doi: 10.1136/bmjopen-2020-041418
Ayoyi A. O., Kikuvi G., Bii C., Kariuki S. (2017). Prevalence, aetiology and antibiotic sensitivity profile of asymptomatic bacteriuria isolates from pregnant women in selected antenatal clinic from Nairobi, Kenya. Pan Afr. Med. J. 26 (1), 1–12. doi: 10.11604/pamj.2017.26.41.10975
Brolund A., Sandegren L. (2016). Characterization of ESBL disseminating plasmids. Infect. Dis. (Auckl). 48 (1), 18–25. doi: 10.3109/23744235.2015.1062536
Cai J. C., Zhang R., Hu Y. Y., Zhou H. W., Chen G.-X. (2014). Emergence of Escherichia coli sequence type 131 isolates producing KPC-2 carbapenemase in China. Antimicrob. Agents Chemother. 58 (2), 1146–1152. doi: 10.1128/aac.00912-13
Cantón R., Coque T. M. (2006). The CTX-m β-lactamase pandemic. Curr. Opin. Microbiol. 9 (5), 466–475. doi: 10.1016/j.mib.2006.08.011
Clinical and Laboratory Standards Institute (2019). Performance standards for antimicrobial susceptibility testing. 29th edition (Wayne, PA: M100-S29. Clinical and Laboratory Standards Institute).
Cove Smith A., Almond M. (2007). Management of urinary tract infections in the elderly. Trends Urology Gynaecol. Sexual Health 12 (4), 31–34. doi: 10.1002/tre.33
Croxall G., Weston V., Joseph S., Manning G., Cheetham P., Mcnally A. (2011). Increased human pathogenic potential of Escherichia coli from polymicrobial urinary tract infections in comparison to isolates from monomicrobial culture samples. J. Med. Microbiol. 60 (1), 102–109. doi: 10.1099/jmm.0.020602-0
Dias Neto J. A., Silva L. D., Martins A. C., Tiraboschi R. B., Domingos A. L., Suaid H. J., et al. (2003). Prevalence and bacterial susceptibility of hospital acquired urinary tract infection. Acta Cirurgica Brasileira. 18 (5), 36–38. doi: 10.1590/s0102-86502003001200013
Erb S., D’Mello-Guyett L., Malebo H. M., Njee R. M., Matwewe F., Ensink J., et al. (2018). High prevalence of ESBL-producing e. coli in private and shared latrines in an informal urban settlement in dar es salaam, Tanzania. Antimicrob. Res. Infect. Control. 7 (1), 1–6. doi: 10.1186/s13756-017-0292-y
Farajnia S., Alikhani M. Y., Ghotaslou R., Naghili B., Nakhlband A. (2009). Causative agents and antimicrobial susceptibilities of urinary tract infections in the northwest of Iran. Int. J. Infect. Dis. 13 (2), 140–144. doi: 10.1016/j.ijid.2008.04.014
Farrell D., Morrissey I., de Rubeis D., Robbins M., Felmingham D. (2003). A UK multicentre study of the antimicrobial susceptibility of bacterial pathogens causing urinary tract infection. J. Infect. 46 (2), 94–100. doi: 10.1053/jinf.2002.1091
Foxaman B. (2010). The epidemiology of urinary tract infection. Nat. Rev. Urol. 7, 653–660. doi: 10.1038/nrurol.2010.190
Foxman B. (2002). Epidemiology of urinary tract infections: incidence, morbidity, and economic costs. Am. J. Med. 113 (1), 5–13. doi: 10.1016/s0002-9343(02)01054-9
Gonzalez C. M., Schaeffer A. J. (1999). Treatment of urinary tract infection: what’s old, what’s new, and what works. World J. Urol. 17 (6), 372–382. doi: 10.1007/s003450050163
Jakobsen L., Garneau P., Bruant G., Harel J., Olsen S., Porsbo L. J., et al. (2012). Is escherichia coli urinary tract infection a zoonosis? proof of direct link with production animals and meat. Eur. J. Clin. Microbiol. Infect. Dis. 31 (6), 1121–1129. doi: 10.1007/s10096-011-1417-5
Jasser A. (2006). Extended-spectrum β-lactamases (ESBLs): a global problem. Kuwait Med. J. 38 (3), 171–185.
Johnson T. J., Hargreaves M., Shaw K., Snippes P., Lynfield R., Aziz M., et al. (2015). Complete genome sequence of a carbapenem-resistant extraintestinal pathogenic Escherichia coli strain belonging to the sequence type 131 H30R subclade. Genome Announc. 3 (2), e00272-15. doi: 10.1128/genomea.00272-15
Karikari A. B., Saba C. K. S., Yamik D. Y. (2021). Bacterial resistance in urinary tract infections: multidrug resistant ESBL producing gram negative uropathogens from patients. In Urinary Tract Infection and Nephropathy-Insights into Potential Relationship. IntechOpen.
Kimera Z. I., Mgaya F. X., Misinzo G., Mshana S. E., Moremi N., Matee M. I. N. (2021). Multidrug-resistant, including extended-spectrum beta lactamase-producing and quinolone-resistant, escherichia coli isolated from poultry and domestic pigs in da es salaam, Tanzania. Antibiotics (Basel). 10 (4), 406. doi: 10.3390/antibiotics10040406
Lee S. Y., Park Y. J., Yu J. K., Jung S., Kim Y., Jeong S. H., et al. (2012). Prevalence of acquired fosfomycin resistance among extended-spectrum β-lactamase-producing escherichia coli and klebsiella pneumoniae clinical isolates in Korea and IS26-composite transposon surrounding fosA3. J. Antimicrob. Chemother. 67, 2843–2847. doi: 10.1093/jac/dks319
Manyahi J., Moyo S. J., Tellevik M. G., Ndugulile F., Urassa W., Blomberg B., et al. (2017). Detection of CTX-M-15 beta-lactamases in enterobacteriaceae causing hospital-and community-acquired urinary tract infections as early as 2004, in dar es salaam, Tanzania. BMC Infect. Dis. 17 (1), 1–7. doi: 10.1186/s12879-017-2395-8
Marando R., Seni J., Mirambo M. M., Falgenhauer L., Moremi N., Mushi MF., et al. (2018). Predictors of the extended-spectrum-beta lactamases producing enterobacteriaceae neonatal sepsis at a tertiary hospital, Tanzania. Int. J. Med. Microbiol. 308 (7), 803–811. doi: 10.1016/j.ijmm.2018.06.012
Mathai D., Jones R., Pfaller M., America T. S. P. G. N. (2001). Epidemiology and frequency of resistance among pathogens causing urinary tract infections in 1,510 hospitalized patients: a report from the SENTRY antimicrobial surveillance program (North America). Diagn. Microbiol. Infect. Dis. 40 (3), 129–136. doi: 10.1016/s0732-8893(01)00254-1
Meier S., Weber R., Zbinden R., Ruef C., Hasse B. (2011). Extended-spectrum β-lactamase-producing gram-negative pathogens in community-acquired urinary tract infections: an increasing challenge for antimicrobial therapy. Infection 39 (4), 333–340. doi: 10.1007/s15010-011-0132-6
Minja C. A., Shirima G., Mshana S. E. (2021). Conjugative plasmids disseminating CTX-M-15 among human, animals and the environment in mwanza Tanzania: a need to intensify one health approach. Antibiotics 10 (7), 836. doi: 10.3390/antibiotics10070836
Mirkalantari S., Irajian G., Siddig E. E., Fattahi A. (2020). Determination of the frequency of β-lactamase genes (bla SHV, bla TEM, bla CTX-m) and phylogenetic groups among ESBL-producing uropathogenic escherichia coli isolated from outpatients. J. Lab. Med. 44 (1), 27–33. doi: 10.1515/labmed-2018-0136
Monstein H. J., Ostholm-Balkhed A., Nilsson M. V., Nilsson M., Dornbusch K., Nilsson L. E. (2007). Multiplex PCR amplification assay for the detection of blaSHV, blaTEM and blaCTX-m genes in enterobacteriaceae. APMIS 115 (12), 1400–1408. doi: 10.1111/j.1600-0463.2007.00722.x
Moremi N., Claus H., Vogel U., Mshana S. E. (2017). Faecal carriage of CTX-m extended-spectrum beta-lactamase-producing enterobacteriaceae among street children dwelling in mwanza city, Tanzania. PloS One 12 (9), e0184592. doi: 10.1371/journal.pone.0184592
Moyo S. J., Aboud S., Kasubi M., Lyamuya E. F., Maselle S. Y. (2010). Antimicrobial resistance among producers and non-producers of extended spectrum beta-lactamases in urinary isolates at a tertiary hospital in Tanzania. BMC Res. notes. 3 (1), 1–5. doi: 10.1186/1756-0500-3-348
Msanga D. R., Silago V., Massoza T., Kidenya B. R., Balandya E., Mirambo M. M., et al. (2022). High fecal carriage of multidrug resistant bacteria in the community among children in northwestern Tanzania. Pathogens 11 (3), 379. doi: 10.3390/pathogens11030379
Mshana S. E., Falgenhauer L., Mirambo M. M., Mushi M. F., Moremi N., Julius R., et al. (2016). Predictors of bla CTX-M-15 in varieties of Escherichia coli genotypes from humans in community settings in mwanza, Tanzania. BMC Infect. Dis. 16 (1), 1–9. doi: 10.1186/s12879-016-1527-x
Mshana S. E., Hossain H., Hain T., Domann E., Chakraborty T. (2009). Conjugative IncFI plasmids carrying CTX-M-15 among escherichia coli ESBL producing isolates at a university hospital in Germany. BMC Infect. Dis. 9, 97. doi: 10.1186/1471-2334-9-97
Mshana S. E., Imirzalioglu C., Hain T., Domann E., Lyamuya E. F., Chakraborty T. (2011). Multiple ST clonal complexes, with a predominance of ST131, of escherichia coli harbouring blaCTX-M-15 in a tertiary hospital in Tanzania. Clin. Microbiol. Infect. 17 (8), 1279–1282. doi: 10.1111/j.1469-0691.2011.03518.x
Mtemisika C. I., Nyawale H., Benju R. J., Genchwere J. M., Silago V., Mushi M. F., et al. (2022). Epidemiological cut-off values and multidrug resistance of escherichia coli isolated from domesticated poultry and pigs reared in mwanza, Tanzania: a cross-section study. Anim. (Basel). 12 (7), 835. doi: 10.3390/ani12070835
Murray C. J. L., Ikuta K. S., Sharara F., Swetschinski L., Robles Aguilar G., Gray A., et al. (2022). Global burden of bacterial antimicrobial resistance in 2019: a systematic analysis. Lancet 399, 629–655. doi: 10.1016/S0140-6736(21)02724-0
Nicolas-Chanoine M. H., Blanco J., Leflon-Guibout V., Demarty R., Alonso M. P., Caniça M. M., et al. (2008). Intercontinental emergence of Escherichia coli clone O25: H4-ST131 producing CTX-M-15. J. Antimicrob. Chemother. 61 (2), 273–281. doi: 10.1093/jac/dkm464
Nielsen K. L., Dynesen P., Larsen P., Frimodt-Møller N. (2014). Faecal escherichia coli from patients with e. coli urinary tract infection and healthy controls who have never had a urinary tract infection. journal of medical microbiology. J. Med. Microbiol. 63 (4), 582–589. doi: 10.1099/jmm.0.068783-0
Onduru O. G., Mkakosya R. S., Aboud S., Rumisha S. F. (2021). Genetic determinants of resistance among ESBL-producing enterobacteriaceae in community and hospital settings in East, central, and southern Africa: a systematic review and meta-analysis of prevalence. Can. J. Infect. Dis. Med. Microbiol. 2021, 5153237. doi: 10.1155/2021/5153237
Paterson D. L., Bonomo R. A. (2005). Extended-spectrum β-lactamases: a clinical update. Clin. Microbiol. Rev. 18 (4), 657–686. doi: 10.1128/CMR.18.4.657-686.2005
Peirano G., Pitout J. D. D. (2010). Molecular epidemiology of Escherichia coli producing CTX-m [beta]-lactamases: the worldwide emergence of clone ST131 O25: H4. Int. J. Antimicrob. Agents. 35 (4), 316–321. doi: 10.1016/j.ijantimicag.2009.11.003
Rasoulinasab M., Shahcheraghi F., Feizabadi M. M., Nikmanesh B., Hajihasani A., Aslani M. M. (2021). Distribution of ciprofloxacin-resistance genes among ST131 and non-ST131 clones of escherichia coli isolates with ESBL phenotypes isolated from women with urinary tract infection. Iranian J. Microbiol. 13 (3), 294. doi: 10.18502/ijm.v13i3.6389
Rogers B. A., Sidjabat H. E., Paterson D. L. (2011). Escherichia coli O25b-ST131: a pandemic, multiresistant, community-associated strain. J. Antimicrob. Chemother. 66 (1), 1–14. doi: 10.1093/jac/dkq415
Sah R. S. P., Dhungel B., Yadav B. K., Adhikari N., Thapa Shrestha U., Lekhak B., et al. (2021). Detection of TEM and CTX-m genes in escherichia coli isolated from clinical specimens at tertiary care heart hospital, Kathmandu, Nepal. Diseases 9, 15. doi: 10.3390/diseases9010015
Seni J., Falgenhauer L., Simeo N., Mirambo M. M., Imirzalioglu C., Matee M., et al. (2016). Multiple ESBL-producing escherichia coli sequence types carrying quinolone and aminoglycoside resistance genes circulating in companion and domestic farm animals in mwanza, Tanzania, harbor commonly occurring plasmids. Front. Microbiol. 7, 142. doi: 10.3389/fmicb.2016.00142
Silago V., Kovacs D., Samson H., Seni J., Matthews L., Oravcová K., et al. (2021). Existence of multiple ESBL genes among phenotypically confirmed ESBL producing Klebsiella pneumoniae and Escherichia coli concurrently isolated from clinical, colonization and contamination samples from neonatal units at bugando medical center, mwanza, Tanzania. Antibiotics (Basel) 10 (5), 476. doi: 10.3390/antibiotics10050476
Tandogdu Z., Wagenlehner F. M. (2016). Global epidemiology of urinary tract infections. Curr. Opin. Infect. Dis. 29 (1), 73–79. doi: 10.1097/QCO.0000000000000228
The United Republic of Tanzania Ministry of Health and Social Welfare (2021). Standard treatment guidelines and essential medicines list, Sixth Edition. Available at: https://medicine.st-andrews.ac.uk/igh/wp-content/uploads/sites/44/2022/01/STG-NEMLIT-2021.pdf.
Keywords: ESBL-producing E. coli, ESBL allele, non-beta lactam antibiotic, ciprofloxacin, gentamicin
Citation: Mwakyoma AA, Kidenya BR, Minja CA, Mushi MF, Sandeman A, Sabiti W, Holden MTG and Mshana SE (2023) Allele distribution and phenotypic resistance to ciprofloxacin and gentamicin among extended-spectrum β-lactamase-producing Escherichia coli isolated from the urine, stool, animals, and environments of patients with presumptive urinary tract infection in Tanzania. Front. Antibiot. 2:1164016. doi: 10.3389/frabi.2023.1164016
Received: 11 February 2023; Accepted: 16 May 2023;
Published: 05 June 2023.
Edited by:
Suzie Hingley-Wilson, University of Surrey, United KingdomReviewed by:
Mikaeel Young, Baylor University, United StatesAzna Zuberi, Northwestern University, United States
Copyright © 2023 Mwakyoma, Kidenya, Minja, Mushi, Sandeman, Sabiti, Holden and Mshana. This is an open-access article distributed under the terms of the Creative Commons Attribution License (CC BY). The use, distribution or reproduction in other forums is permitted, provided the original author(s) and the copyright owner(s) are credited and that the original publication in this journal is cited, in accordance with accepted academic practice. No use, distribution or reproduction is permitted which does not comply with these terms.
*Correspondence: Benson R. Kidenya, YmVua2lkZW55YUBnbWFpbC5jb20=