- 1Department of Environmental Medicine and Climate Science, Icahn School of Medicine at Mount Sinai, New York, NY, United States
- 2Department of Epidemiology, University of Iowa College of Public Health, Iowa City, IA, United States
- 3Institute for Climate Change, Environmental Health, and Exposomics, Icahn School of Medicine at Mount Sinai, New York, NY, United States
Asthma is a highly prevalent inflammatory condition, significantly affecting nearly six million U.S. children and impacting various facets of their developmental trajectories including neurodevelopment. Evidence supports a link between pediatric environmental exposures in two key areas: asthma and executive function (E.F.). E.F.s are a collective of higher-order cognitive processes facilitating goal-oriented behaviors. Studies also identify asthma-associated E.F. impairments in children. However, limited research has evaluated the inter-relationships among environmental exposures, asthma, and E.F. in children. This review explored relevant research to identify and connect the potential mechanisms and pathways underlying these dynamic associations. The review suggests that the role of the pediatric exposome may function through (1) several underlying biological pathways (i.e., the lung-brain axis, neuroendocrine system, and hypoxia), which could drive asthma and maladaptive E.F. in children and (2) the relationships between the exposome, asthma, and E.F. is a bidirectional linkage. The review reveals essential synergistic links between asthma and E.F. deficits, highlighting the potential role of the pediatric exposome.
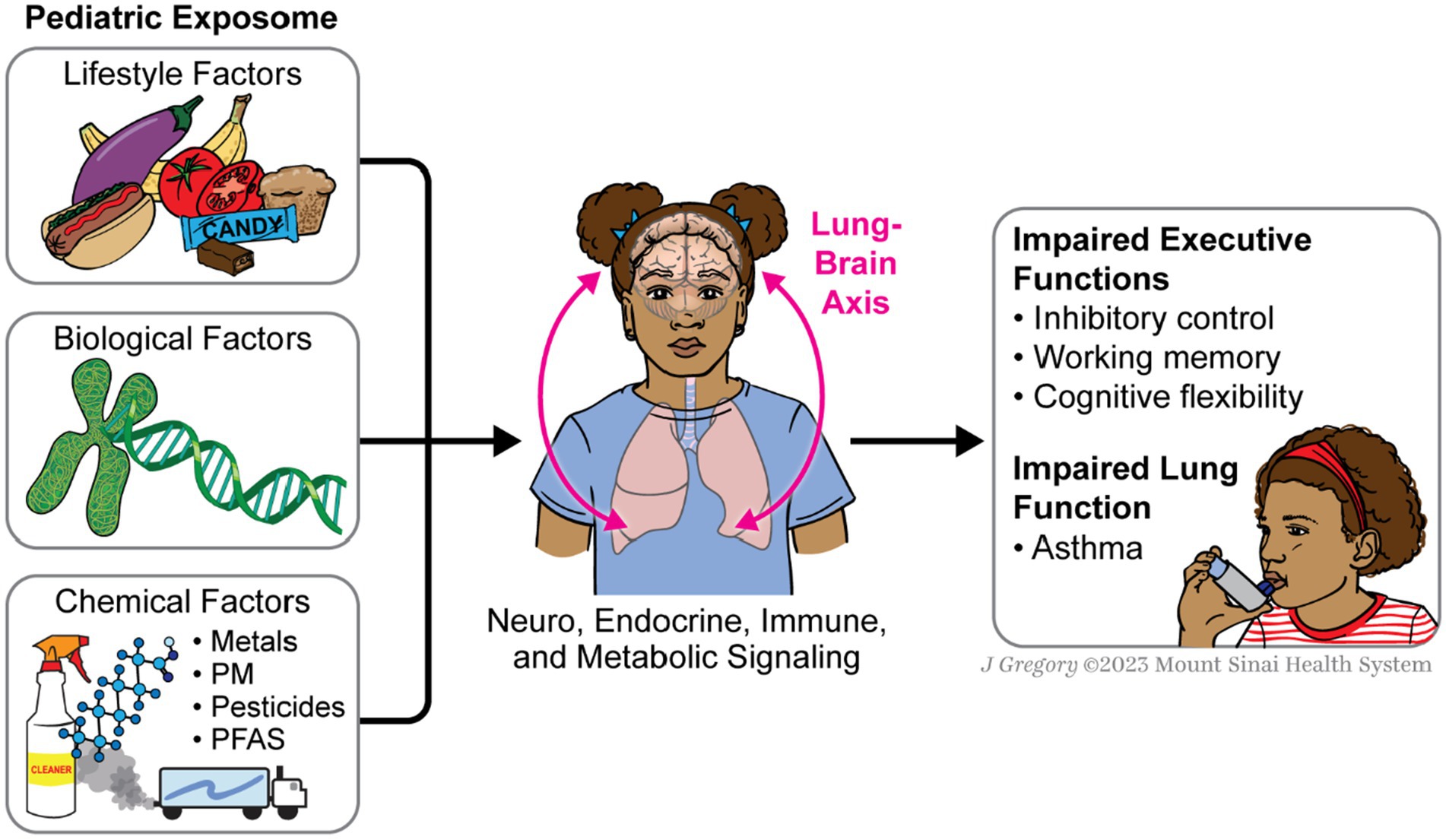
Graphical Abstract. This diagram illustrates the underlying pathways between the exposome, lungs, and E.F. The lungs and the brain are interconnected through neuro, endocrine, and immune signaling pathways. Exposomic factors affect the lungs, which can cause asthma, and this, in turn, affects E.F. through metabolic signaling pathways that send metabolic signals from the lungs to the brain.
Highlights
• Accumulating scientific evidence supports a link between environmental exposures and asthma and executive function, respectively, and a link between asthma and executive function in children.
• There is a gap on the evaluation of the complex synergistic link between environmental chemical exposures, asthma, and E.F. outcomes in children.
• This review explores relevant research to identify and connect the potential mechanisms and pathways underlying these dynamic associations.
• We will focus on the chemical exposure due to its heightened risk for children’s health.
1 Overview of the exposome and pediatric health
The exposome represents a comprehensive assessment of the environment (1) including all the chemical and non-chemical exposures an individual encounters throughout their life course and how these exposures influence human development and health (2, 3). The exposome can be divided into two domains although there is considerable overlap in the 2 domains: (1) external and (2) internal exposome (4, 5). External refers to exposures external to the human body involving chemical, physical, and psychosocial mechanisms such as environmental contaminants (e.g., heavy metals, pesticides, and other persistent organic pollutants) and non-chemical environmental exposures (e.g., diet, stress, climate, infections, among others). Internal refers to the biological processes within an organism involving molecules, internal chemical components (such as endogenous metabolites and exogenous chemicals derived from the environment), and biological reactions to external exposures (2). Comprehensively identifying environmental factors affecting pediatric health and well-being requires understanding the exposome. Investigating the exposome offers an opportunity to explore multiple ecological layers affecting health, including environmental biomarkers, biological mechanistic pathways, and adverse health outcomes, particularly during sensitive and critical developmental stages of life, as it provides researchers and scientists with invaluable insights to explore causal interactions among and between the exposome for formulating effective prevention strategies.
Environmental exposures begin in-utero and can interact with various developmental processes including genetics, epigenetics, and physiological mechanisms starting prenatally through childhood. Specifically, the exposome is critical as the early years of life are highly susceptible to increased physiologic disruptions, increasing the risk for exposure-associated health outcomes that can have long-term effects (6). Evidence has shown that the pediatric exposome involving chemical exposures such as essential and nonessential metals, per-and poly-fluoroalkyl substances (PFAS), pesticides, phthalates, and air pollution has been associated with a range of adverse developmental health outcomes, including respiratory disease and maladaptive cognitive development. Due to children’s distinctive biological composition and behaviors, environmental exposures are more hazardous and detrimental to their health than adults. Childhood is a time of rapid physiologic growth and development, during which various biological systems and organs are at different maturation stages, often with underdeveloped detoxification mechanisms (7, 8). Moreover, children suffer from greater exposure levels as they consume more environmentally contaminated substances relative to their body weight than adults. They also engage in unique behaviors that introduce them to different routes of exposure, including spending more time outdoors (9, 10) and engaging with different consumer products (11, 12). This increased vulnerability is concerning, especially with the rising occurrence of environmental exposure-related diseases like asthma and cognitive impairments in executive functions (E.F.s).
Accumulating scientific evidence supports a link between environmental exposures and (1) asthma and E.F.s and (2) a link between asthma and E.F. in children, but little to no research has evaluated the complex synergistic link between environmental chemical exposures, asthma, and E.F. outcomes in children. This review primarily explores relevant research to identify and connect the potential mechanisms and pathways underlying these dynamic associations. Our focus is directed toward chemical exposure due to its heightened risk for children’s health. Thus, this narrative review will (1) provide a comprehensive review of the literature on the relationships between chemical exposures on (a) respiratory disease and (b) E.F.s; (2) leverage the literature to investigate the (c) link between asthma and E.F. dimensions and (d) the dynamic role of the exposome in the underlying pathways between asthma and E.F.s; and (3) conclude with an discussion on the needs regarding future research in this area.
2 Chemical exposures and respiratory disease in children
Respiratory diseases are associated with significant global mortality and morbidity, especially among infants and young children (13). Asthma is one of the most common chronic diseases in children worldwide (14–17). Globally, approximately 300 million people have asthma, and an estimated 100 million more people, mostly children, will have asthma by 2025 (18, 19). In the U.S., asthma and wheezing disproportionately affect specific groups, including children from urban, low-income, or racial/ethnic minoritized backgrounds. Moreover, socioeconomic status is inversely related to lung function (20). Early-life lung function is also an essential predictor for peak lung function in adults, as well as later-life declines in lung function (21, 22). Lung growth correlates with lung function during childhood and adolescence (23, 24) and predicts later-life respiratory disease (21, 24), early multi-morbidity (25), and premature mortality (25, 26).
Prenatal environmental exposures have been associated with respiratory diseases and can potentially alter lung development and growth in childhood and adulthood (27, 28). Lung development begins in utero through a carefully orchestrated sequence of events vulnerable to disruption by environmental insults leading to alterations in lung development, affecting the structure and function of the respiratory system across the life span. Therefore, identifying early life risk factors that may be amenable to intervention can provide mechanistic information to better elucidate the link between environmental exposures and childhood respiratory disease and inform the timing of public health interventions.
The fetal environment has been shown to be an unrecognized contributor to disease risk (29, 30), including lung disease (31–34). Lung development begins prenatally through a carefully coordinated sequence of events vulnerable to disruption by even relatively low-dose environmental exposures, which can alter the maturation of organ systems later in life (35). During the first half of gestation, the development of bronchi occurs along with the branching of the airways (36). Throughout the second half of gestation, the alveoli develop, and the lungs continue to mature in number, size, and complexity of the alveoli years after birth (37). Classifying modifiable early life risk factors can illuminate the mechanisms linking environmental exposures to childhood respiratory disease, paving the way for targeted interventions (36). To further explore these mechanisms, the following section investigates the associations between specific chemical exposures and respiratory health outcomes among children. This builds on modifiable early life risk factors outlined in this section.
3 Associations between chemical exposures and respiratory health outcomes
3.1 Metals
Metals, specifically manganese (Mn) and lead (Pb), have been associated with adverse respiratory health outcomes and lung function deficits in children (36). Mn and Pb are the most common metals individuals are exposed to. Although not well studied in children, Cd is a well-known respiratory toxicant from occupational exposure. These metals are naturally occurring and have anthropogenic sources (36, 38). There is increasing evidence that suggests that prenatal metal exposures adversely affect the subsequent development of allergic diseases in children (39–43). Prenatal metal mixture exposure was associated with childhood allergic rhinitis (39). Prenatal lead was associated with an increased risk for atopic sensitization to common aeroallergens in children at 5 years of age in Poland. These results suggest that low levels of in-utero lead exposure may be implicated in the process of allergic sensitization (44). In Italian adolescents, personal air manganese levels were associated with greater odds of reported asthma and asthma medication use (45). Exposure to lead has also been associated with lung function deficits in children (46–48). In Mexico City, researchers found associations between prenatal Pb and childhood lung function were modified by disrupted maternal cortisol in pregnancy and child sex (49).
3.2 Per- and polyfluroalkyl substances
Per-and polyfluroalkyl substances (PFAS) are industrial chemicals used in numerous commercial products, which include fabric, cookware, and food container coatings (50). They are also used for aqueous film-forming foams used in firefighting (50–53). PFAS bioaccumulates with the highest concentrations detected in the liver and blood (50). It has been phased out of productivity in the United States, and alternative PFAS has taken its place. When PFAS is inhaled, it targets the lung, and it is known to modify lung surfactant function and proinflammatory responses (50, 54–58). Previous studies have reported associations between exposure to PFAS and asthma, airway hyper-responsiveness (AHR), and inflammation (50, 59–61). There have been inconsistencies (62, 63) in the studies reporting positive associations between exposure to PFAS and asthma-related outcomes in children (64, 65). In Norway, total PFAS serum concentrations were associated with the occurrence of asthma (50, 66) Total perfluorooctane sulfonic acid (PFOS), linear PFOS, and linear perfluorohexane sulfonate (PFHxS) doubled the odds of asthma (50, 66). A correlation was found between recurrent respiratory tract infection and perfluorobutane sulfonic acid (50, 67). PFAS exposure likely alters lung biology influencing asthma, and airway hyper-responsiveness (50).
3.3 Phthalates
Phthalates are a group of ubiquitous endocrine-disrupting chemicals used as plasticizers in various industrial commodities and as additives in cosmetics (68, 69). They are used in cosmetic and personal care products, food packaging, toys, containers, shower curtains, and cleaning and building materials (70, 71). Phthalates with low molecular weight are commonly used in solvents, medications, alcohol denaturants, and fixatives (70, 72). Phthalates can accumulate at measurable concentrations in various environments, which include fresh water, soils, atmosphere, sediments, and landfills (69). Individuals are exposed to phthalates daily through diet, inhalation, breastmilk, and skin contact (69). Phthalate metabolites can also cross the placenta and have been detected in cord blood, amniotic fluid, placental tissue, and neonatal meconium (69, 73). Due to the ubiquitous nature of phthalates in daily life, human exposure to phthalates has become a major concern, specifically among potentially vulnerable populations, including pregnant women and infants (74). There is evidence from both animal (75) and human studies (76, 77) that phthalates affect lung health. In animal studies, in utero exposure to phthalates, specifically to di-(2-ethylhexyl)- phthalate (DEHP), has been shown to impact alveolarization, including lack of formation and maturation of the distal parts of alveoli in the lungs of newborn rats exposed to phthalates prenatally (78). Epidemiological studies have described associations between prenatal phthalate exposure and childhood respiratory, allergic, and atopic diseases (70, 74, 79–83). Jahreis et al. reported that maternal urinary concentrations of butyl benzyl phthalate during pregnancy were associated with increased asthma risk at 6 years of age in Germany (82). Gascon et al. reported that prenatal exposure to bisphenol A (BPA) and high molecular weight phthalates increased the risk of wheezing and asthma outcomes in children from birth to 7 years of age in Catalonia, Spain (81). However, Johnk et al. investigated associations between maternal urinary concentrations of phthalate metabolites and asthma, eczema, and rhinitis in offspring aged 5 years and did not report any significant findings in the Odense Child Cohort, a prospective Danish birth cohort (83).
3.4 Pesticides
Pesticides are a wide group of chemical substances used to kill or control pests and are used for both large and small-scale farming (84, 85). Individuals are exposed to various pesticides simultaneously; thus, the general population, specifically pregnant women and children, may be exposed to pesticide mixtures (86). A few studies have assessed the associations between prenatal pesticide exposure and adverse children’s respiratory health outcomes, including asthma, wheezing, persistent cough, and lung function impairment. However, the results have been inconsistent (87, 88). Researchers have reported that higher prenatal dichlorodiphenyldichloroethylene exposure is associated with an increased risk of wheeze (89, 90). In addition, higher phosphate metabolite exposure in childhood was associated with decreased lung function at seven years of age among the children of farmworkers in California (91). A study in the Netherlands found no association between children living near agricultural fields likely treated with pesticides and asthma/respiratory outcomes (92). While the importance of investigating mixtures of pesticides is emerging (87, 88, 93), there is a dearth of research investigating prenatal pesticide mixtures and asthma and wheeze, and lung function in childhood (94).
3.5 Particulate matter
Particulate matter (PM) is a complex mixture of solids and liquids suspended in the air. The PM can be categorized by its aerodynamic diameter as PM10 (<10 μm, inhalable particulate matter), PM2.5 (<2.5 μm, fine particulate matter), and PM0.1 (<0.1 μm, ultrafine particulate matter) (95). PM2.5 is considered the most harmful and can absorb a variety of toxic and harmful substances (96). Due to its small particle size, it penetrates deep into the lungs, deposits in the terminal bronchioles and alveoli with breath, and enters the circulatory system through the gas-blood barrier (97, 98). Exposure to PM2.5 can lead to systemic adverse effects, specifically on the respiratory and cardiovascular systems (99). The respiratory system is the primary route of toxicity for PM2.5. Individuals are primarily exposed through inhalation (95). Exposure to PM2.5 can cause the development and progression of acute and chronic lung diseases, such as tracheal and pulmonary inflammation (100–102), and asthma (103, 104). Moreover, PM2.5 exposure may increase the susceptibility to respiratory infections (95).
3.6 Summary
Respiratory diseases and respiratory tract symptoms in children are fairly common. Many studies have shown that exposure to chemicals such as pesticides, PFAS, PM, phthalates, and metals can lead to respiratory diseases in children. Most of these studies have reported exposure prenatally as a critical window linked to children developing asthma and wheeze. However, these studies have a few weaknesses, which include sample size or subject selection. Future directions include research on the biological mechanisms of various chemical exposures, individually and as a mixture, and their impact on asthma and wheeze in children. Furthermore, the dissemination of these research findings, and the creation and implementation of effective prevention strategies are essential to increase knowledge of chemical exposures and their impact on adverse respiratory health outcomes in children. In the following section, we delve into children’s E.F.s and their relationship with early-life neurotoxic exposures, comprehensively analyzing the chemistry between these factors.
4 Chemical exposures and executive functioning in children
Executive functions (E.F.s) encompass a group of high-level skills that coordinate sophisticated cognitive and behavioral activities and allow for complex functions such as planning, attention, emotional control, organization, focusing on problem-solving, and shifting viewpoints. E.F. development is vital across childhood and consists of at least three central domains – inhibitory control, working memory, and cognitive flexibility. Accumulating scientific evidence suggests that E.F.s are particularly sensitive to early-life environmental neurotoxicants (e.g., trace metals, PFAS, phthalates, pesticides, and particulate matter) as neurotoxicants can penetrate the blood–brain barriers and accumulate in brain regions (e.g., prefrontal cortex, hippocampus, and basal ganglia) that regulate E.F. functionality.
4.1 Inhibitory control
Inhibitory control is a fundamental E.F. with connectivity within several anatomical brain networks [e.g., caudate nucleus, subthalamic nucleus; (105)], but the prefrontal cortex (PFC) connectivity is essential for regulating and orchestrating successful inhibitory control (106, 107). Inhibitory control enables children to inhibit goal-irrelevant stimuli from interrupting goal-directed behavioral and emotional responses (107, 108), which is critical for cognitive processes relating to attention response, emotional regulation, behavioral control, and social development. Inhibitory control begins to develop rapidly between ages 3 and 6 years (108, 109) and continues until the functional maturation of the frontal lobes, often during late adolescence.
4.2 Working memory
Working memory is a core cognitive feature of the human cognitive system with underpinnings to the dorsolateral PFC, an area in the PFC during child development (110). It is a system with a temporary capacity for simultaneously processing, holding, and manipulating information (108). Working memory development is vital for children as it is responsible for multi-dimensional cognitive activities such as reasoning, decision-making, problem-solving, comprehension, and learning (111) that are central for everyday tasks while involving dual-task performance with several other high-level cognitive faculties (112). Working memory develops during infancy and dramatically increases during middle childhood (ages 4 to 8), with gradual developments during early adolescence.
4.3 Cognitive flexibility
Cognitive flexibility is a core executive dimension that amplifies on the first two E.F. domains, primarily supported by several PFC sub-regions (e.g., orbitofrontal, anterior cingulate) but is also supported by subcortical structures such as the posterior parietal cortex and basal ganglia (113, 114). Cognitive flexibility involves focusing on changing perspectives, shifting attention, creative thinking, and adapting flexibly to changeable environments (108, 115). It aids in managing multiple tasks, fostering innovative, adaptable behavior, and fundamentally promoting a range of life outcomes, including academic achievement, career attainment, and successful social relationships (116). During preschool years, cognitive flexibility matures swiftly and progresses throughout adolescence, paralleling the maturation of neural networks encompassing the PFC, while it only reaches full maturation by the early 20s.
5 Associations between neurotoxicant exposures and executive function development
Accumulating evidence indicates E.F. can be impaired when children are exposed to various environmental neurotoxicants. As previously mentioned, once in the body, early-life neurotoxicants can cause biochemical neurologic damage by readily penetrating the child’s blood–brain barriers, accumulating in supportive brain regions, disrupting neurotransmitter systems and their activities, and altering structural and functional subcortical components that regulate E.F.s (117, 118). In this section, our attention will be directed toward an assortment of neurotoxicants, including metals, PFAS compounds, particulate matter (PM), pesticides, and phthalate metabolites.
5.1 Metals
Environmental epidemiological studies have shown that essential (manganese [Mn], iron [Fe], chromium [Cr], selenium [Se], and zinc [Zn]) metals are critical for child growth and brain development. However, imbalanced metal levels can lead to deficiencies or neurotoxicity, causing maladaptive E.F (119). In addition, any dose exposure to nonessential (arsenic [As], cadmium [Cd], lead [Pb], mercury [Hg], nickel [Ni], and strontium [Sr]) is considered deleterious to E.F. as these metals lack biological significance, with substantial evidence identifying an inverse association between nonessential metals and E.F. performance among children (120–122). Data from children’s studies indicate that prenatal and postnatal under and over-exposure to a panel of essential and nonessential metals can dysregulate E.F. development on an individual exposure (123, 124) and when exposed as mixtures (125–127). For both elements, many studies suggest a sexually dimorphic effect, with E.F. performance differing in the directionality by child sex, depending on the type and dose of metal exposure and time and duration of exposure (128).
5.2 Per- and polyfluroalkyl substances
Evidence from human and non-human animal studies suggests that PFAS dysregulates dopamine metabolism (129) while also affecting thyroid function (122). Both processes may have persistent implications for E.F. development. Animal models have demonstrated an increased risk of neurobehavioral deficits relating to inhibitory control mechanisms (130–135). Children’s studies on the neurotoxicity of certain PFAS compounds are limited and inconsistent. Some research investigating prenatal windows of PFAS exposure reported positive associations with cognitive functions underpinned by E.F., such as reading ability (136), attention (137), and memory capabilities (138). Others reported negative associations with measures of response inhibition, working memory, and global E.F.s (139–143). Research has primarily focused on the prenatal effects of PFAS exposure on children’s E.F. outcomes, while the impact of postnatal windows is sparse and overlooked. Most epidemiologic research has focused on investigating a single PFAS compound on cognitive and behavioral measures in children (137, 144, 145), which does not capture the mixed-exposure contexts in which people encounter PFAS. To our knowledge, only one study has examined the mixture effects of PFAS on E.F., but it was conducted in older adults (146). Lastly, in the literature, evidence suggests that there were no consistent patterns of sexual dimorphism in PFAS associations with E.F (147).
5.3 Phthalates
Human (148) and non-human animal (149–151) studies suggest that phthalate compounds cause dopaminergic dysregulation, a central neurotransmitter system regulating E.F. and reward processing. Animal models of phthalate exposure demonstrate an increased risk of neurobehavioral deficits (152, 153), and memory impairments (154). Human studies have shown that prenatal phthalate exposures are linked to global E.F.s (155) and cognitive constructs related to E.F., such as delayed psychomotor, language development, intellectual developmental (156, 157), and attention deficits (158, 159), as well as overall abnormalities in cognition and neurobehavior. Some studies have also observed sex-specific effects (155, 160, 161). In addition, most epidemiologic research has focused on investigating a single phthalate compound on cognitive measures (152, 159, 162) with some research discovering a link between mixture effects of phthalates and E.F. development (163, 164).
5.4 Pesticides
Pesticide exposures have been linked to E.F. impairment in children (165–167). Several classes (e.g., organophosphorus pesticides [OPPs], carbamate pesticides) of pesticides inherently possess neurotoxic properties by design, acting by directly affecting insects’ nervous systems (168). In children, they have the potential to induce neurological harm (167, 169, 170) even at lower concentrations, manifesting as subtle impacts due to oxidative stress and their influence on fundamental neuronal systems, thereby impairing the dynamics of neurotransmitters involved in synaptic communication (171). Children studies have reported associations between early-life neurotoxic pesticide exposures and poorer E.F. performance in motor inhibition (172, 173), behavioral regulation (173), working memory (173–175), and cognitive flexibility (176, 177). Evidence on the sexually dimorphic effects is limited, yet a few studies report E.F. differences among boys and girls (178–180), highlighting a potentially heightened vulnerability in boys exposed to early-life pesticides.
5.5 Particulate matter
Mounting evidence strongly indicates that environmental ambient PM and pollutants associated with traffic could potentially function as agents of developmental neurotoxicity (181, 182). Moreover, findings from various sources, spanning animal experiments to epidemiological research, suggest that the detrimental impacts of PM on E.F. might initiate during fetal development (183, 184). The developing fetal brain is notably vulnerable to the impacts of PM, as multiple studies have shown that PM exposure increases neuroinflammation and oxidative stress (185) and alters brain morphology (186), further establishing the adverse effects of prenatal air pollution on both neurodevelopment and neurobehavioral functions relating to E.F. in children. Past research has illuminated that exposure to various P.M.s, such as particulate matter 2.5 (PM2.5) and PM10 levels, during distinct sensitive periods like prenatal stages, can amplify the likelihood of maladaptive E.F. trajectories in infants and children (183, 187–190). Limited research has detected sex-specific effects on E.F. outcomes (188), while the majority have not found notable patterns.
5.6 Summary
The implications of these findings are substantial, as childhood is a sensitive and pivotal neurodevelopmental stage. Maladaptive E.F. caused by subclinical neurotoxicity during this stage can manifest later in life and yield enduring ramifications, affecting various aspects of life such as quality of life, educational attainment, social interactions, and professional achievements. The intricacies of the temporal relationships between neurotoxicants and E.F. remain unclear, underscoring the need for more longitudinal designs to unravel these temporal dynamics. Despite this uncertainty, it remains imperative to identify and mitigate risk factors associated with neurotoxic exposures. Developing environmental interventions to reduce or eliminate exposure becomes paramount to enhance E.F. development.
6 Exploring the link between asthma and executive function
Studies have discovered a link between asthma and E.F. decrements in children (191–193) and adults (194, 195). This linkage may be explained by potential disruptions in the neuroendocrine system and chronic to mild intermittent hypoxia (reduced oxygen levels) in children with asthma (192, 194, 196); thus, leading to less efficient E.F. development. Evidence has shown that asthmatic children manifest difficulties in E.F. tasks relating to shifting attention (191, 193) and emotional and behavioral regulation, mainly when the condition is poorly controlled (197, 198). A cross-sectional study comparing asthmatic and healthy adults using diffusion tensor imaging to investigate whole-brain variations in white matter integrity revealed that adults with asthma exhibited several cognitive deficiencies, including E.F. impairments, which strongly correlated with white matter abnormalities (194). These findings reinforce the notion that children with asthma, particularly those with poorly managed treatments, face a heightened risk of E.F. disturbances (199). Consequently, it becomes imperative to proactively evaluate children’s E.F. capabilities rather than solely focusing on their general intellectual aptitude (191).
Some studies suggest that there may be a bidirectional link between asthma and cognitive activities in children and adults (162, 193, 200), but the data are limited. Asthma may affect cognitive function, including E.F., and poor E.F. may be associated with asthma and other comorbid conditions. Further research is needed to better understand the mechanisms underlying this association and to develop targeted interventions that promote optimal executive functioning in individuals with asthma.
7 The role of the exposome in the underlying pathways between asthma and executive function
7.1 Neuroendocrine system and hypoxia
The neuroendocrine system is comprised of nerves and gland cells. The purpose of the system is to create hormones and release them into the bloodstream. These cells receive messages and signals from the nervous system and respond by making and releasing hormones. Glucocorticoids and catecholamines are able to modulate various aspects of the immune system. In addition, the pituitary hormones prolactin and growth hormone are also released, which also can modulate the immune system. Conversely, cytokines and hormones released by an activated immune system influence neural and endocrine processes. For example, the sympathetic nervous system innervates immune organs such as the thymus, bone marrow, spleen, and even lymph nodes (201). We noted earlier that stress hormones released during hypothalamic–pituitary–adrenal (HPA) axis activation can adversely impact immune function. One way they do this is by inhibiting the production of lymphocytes, white blood cells that circulate in the body’s fluids that are important in the immune response. Overall, these hormones control numerous body functions (202). Asthma can impact the central nervous system. It can lead to decreases in the oxygen levels due to brain tissue being sensitive (192). During acute asthma attacks the oxygen saturation can drop to persistently low levels which can influence neural abnormalities (192, 203). A potential cause of cerebral hypoxia is severe asthma attacks. These attacks can lead to the loss of consciousness, cyanosis, anoxic brain damage, or even death (192, 204, 205). Previous studies have reported that baseline arterial hemoglobin oxygen saturation can be found in asthma (192, 206–208). Cognitive dysfunction could potentially be caused by neural changes from hypoxia/ischemia in vulnerable brain regions with high metabolic demands (e.g., neocortex, hippocampus, basal ganglia). Additionally, animal studies have examined the effects of asthma- induced intermittent brain hypoxia on learning and memory show deficits, in addition to impaired long-term potentiation (209). Studies have shown chronic asthma could result in multiple cognitive impairments, specifically learning and memory ability. Lastly, chronic asthma damages synaptic structure and function (192, 194, 209).
7.2 Lung-brain Axis
It has been recently discovered that the brain communicates with various organs in the body through a neuroanatomical communication system. Studies show a dynamic bidirectional signaling pathway between the lung and brain, referred to as the lung-brain axis, identified through sophisticated metabolomics analysis (210). This bidirectional communication involves several biological components, including the central nervous system (CNS), the autonomic nervous system, and the hypothalamus–pituitary–adrenal (HPA) axis, as well as signaling pathways with metabolites, gases, endocrine, and the immune system (211). Activation of the organ-specific immune system in the lung or the brain can alter the functioning of the respective other organs (212). The lung-brain axis has been explored in various pathophysiological conditions, such as inflammatory and immune components, amyloid-β pathology, host–microbe interactions, and ventilator-induced brain injury (213–215). The lung-brain axis is thought to correlate lung microbes to neurodegenerative diseases and changes in behavior (216).
To our knowledge, no human studies have examined chemical exposures’ impact on the lung-brain axis. Much of the research is conducted in rodent models. Evidence suggests that air pollutant exposures in these models lead to serum-borne, cytokine-independent signals that elevate brain proinflammatory conditions connected to the pulmonary response (217). Exposure to zinc oxide nanoparticles may damage the cerebral cortex, possibly via lung-brain axis disruption (218). The lung-brain axis is involved in a process where the pulmonary response to ozone exposures indirectly influences CNS neuroimmune function through circulating and cellular factors (219). Overall, these findings suggest that chemical exposure can impact the lung-brain axis, but the various pathways and mechanisms underlying these effects still need to be fully understood, and further research in humans is needed.
7.3 Limitations
Our review have limitations that warrant attention. A key limitation is the exclusive focus on external chemical exposures within the pediatric exposome, omitting internal exposures (2) such as metabolites, as well as non-chemical factors (220) like infections, lifestyle behaviors, psychosocial stressors, and socioeconomic determinants—all of which are integral to the broader exposome framework. Additionally, this review did not consider biological exposures, such as allergens (221), despite their relevance to pediatric health. To comprehensively understand the pediatric exposome, future research should integrate both internal and non-chemical exposures, capturing the multifactorial nature of environmental influences on child health outcomes.
8 Gaps and future directions
Most evidence linking asthma to E.F. and the influence of the pediatric exposome comes from animal studies. In human studies, the existing data only examine part of the biological pathways between chemical exposures, asthma, and E.F. There are a number of other ways in which E.F. may influence asthma treatment as well. For example, impulsive behavior may increase risk of exposure to asthma triggers such as allergens, and children with poor cognitive flexibility may delay treatment. Few, if any, human studies have explored whether environmental exposure disrupts the lung-brain axis or how exposures impact pathways between neuroendocrine systems and hypoxia, leading to worse asthma outcomes, which could affect E.F. development. As cognitive development is rapid and critical during childhood, further investigation among human studies on the impact of chemical exposure on the biological pathways between asthma and E.F. is necessary. Epidemiological evidence from robustly designed child cohorts, encompassing diverse variables such as sex, age, and longitudinal follow-up, is imperative. This approach will allow for a comprehensive exploration of all three components within each pathway, leading to deeper insights into the dynamic interconnections. We also emphasize the importance of employing advanced statistical methodologies to better understand the dynamics between exposure, asthma, and E.F.s (222). The literature has primarily relied on traditional regression models that examine individual exposures or chemical mixture models, such as Weighted Quantile Sum Regression (223) and Bayesian Kernel Machine Regression (224), which do not fully capture the underlying structural relationships. Therefore, we recommend using a structural equation-modeling framework (225, 226). This approach can effectively capture the complex interrelationships between the chemical exposome, respiratory health, and E.F. dimensions while managing individual variability inherent in studying complex biological and cognitive processes. By adopting this methodology, we can significantly enhance our comprehension of the intricate causal dynamics underlying these relationships (227, 228). Furthermore, there have been extensive advancements in measuring external exposures with personal wearable device (229, 230). This could be used with other omics measurements and linked to pediatric exposome – health in the future. Utilizing technology advancements in epidemiologic research would be an effective way to measure particulate matter, for example, and assess the relationship between executive function and respiratory health outcomes across the lifespan (231). Additionally, researchers collaborating with health care providers to measure the deficits in cognition and their associations with asthma would be useful. This will provide insight into these associations found during primary care and/or asthma specialty clinical visits.
9 Conclusion
This narrative review explored the state of the literature pertaining to the relationship between the pediatric exposome, respiratory health, and E.F. in children. Here, we present scientific evidence that may suggest the role of the exposome through the lung-brain axis, neuroendocrine systems, and hypoxia, which could potentially be the underlying pathways between asthma and E.F. These relationships are not unidirectional but rather bidirectional, as evidence suggests that the lung-brain axis is a two-way communication pathway between the brain and the respiratory system, and the neuroendocrine system is involved through HPA, which integrates cognitive and behavioral responses to early-life exposures. This review implies that human studies in children are needed to understand better the synergistic relationships on the impact of chemical exposure on the biological pathways and interactions between asthma and E.F.
Author contributions
CA: Conceptualization, Funding acquisition, Investigation, Methodology, Project administration, Resources, Validation, Visualization, Writing – original draft, Writing – review & editing. JL: Conceptualization, Formal analysis, Funding acquisition, Investigation, Methodology, Project administration, Resources, Supervision, Validation, Visualization, Writing – original draft, Writing – review & editing. VM: Conceptualization, Resources, Visualization, Writing – review & editing, Writing – original draft. SE: Conceptualization, Resources, Visualization, Writing – review & editing, Writing – original draft. RW: Funding acquisition, Resources, Supervision, Visualization, Writing – review & editing, Writing – original draft. MR: Funding acquisition, Resources, Supervision, Visualization, Writing – review & editing, Writing – original draft.
Funding
The author(s) declare that financial support was received for the research, authorship, and/or publication of this article. This work was supported by the by the National Institute of Child Health and Human Development grant T32HD049311 (Alcala, CS; Lane, JM). The National Institute of Environmental Health Sciences grant, R00ES027496 and R01ES033245 (Rosa MJ, PI); and R01ES014930, R01ES013744, R24ES028522, P30ES023515, R01ES026033, R01MH122447, R01ES029511, and R01ES028927 (Wright, RO, PI).
Conflict of interest
The authors declare that the research was conducted in the absence of any commercial or financial relationships that could be construed as a potential conflict of interest.
Publisher’s note
All claims expressed in this article are solely those of the authors and do not necessarily represent those of their affiliated organizations, or those of the publisher, the editors and the reviewers. Any product that may be evaluated in this article, or claim that may be made by its manufacturer, is not guaranteed or endorsed by the publisher.
References
1. Miller, GW, and Jones, DP. The nature of nurture: refining the definition of the exposome. Toxicol Sci. (2014) 137:1–2. doi: 10.1093/toxsci/kft251
2. Handakas, E, Robinson, O, and Laine, JE. The exposome approach to study children’s health. Curr Opin Environ Sci Health. (2023) 32:100455. doi: 10.1016/j.coesh.2023.100455
3. Vineis, P, Robinson, O, Chadeau-Hyam, M, Dehghan, A, Mudway, I, and Dagnino, S. What is new in the exposome? Environ Int. (2020) 143:105887. doi: 10.1016/j.envint.2020.105887
4. Oyana, TJ, Matthews-Juarez, P, Cormier, SA, Xu, X, and Juarez, PD. Using an external exposome framework to examine pregnancy-related morbidities and mortalities: implications for health disparities research. Int J Environ Res Public Health. (2016) 13:13. doi: 10.3390/ijerph13010013
5. Zhang, H, Lu, H, Yu, L, Yuan, J, Qin, S, Li, C, et al. Effects of gestational exposure to perfluorooctane sulfonate on the lung development of offspring rats. Environ Pollut. (2021) 272:115535. doi: 10.1016/j.envpol.2020.115535
6. Stone, J, Sutrave, P, Gascoigne, E, Givens, MB, Fry, RC, and Manuck, TA. Exposure to toxic metals and per-and polyfluoroalkyl substances and the risk of preeclampsia and preterm birth in the United States: a review. Am J Obstet Gynecol MFM. (2021) 3:100308. doi: 10.1016/j.ajogmf.2021.100308
7. Carroquino, MJ, Posada, M, and Landrigan, P. Environmental toxicology: children at risk. Environ Toxicol. (2012) 239–91. doi: 10.1007/978-1-4614-5764-0_11
8. Larcher, V. Children are not small adults: significance of biological and cognitive development in medical practice. In: Thomas S and Steven E, editors. Handbook of the philosophy of medicine. Dordrecht: Springer (2017). 371–93.
9. Vanos, JK. Children's health and vulnerability in outdoor microclimates: a comprehensive review. Environ Int. (2015) 76:1–15. doi: 10.1016/j.envint.2014.11.016
10. Suwanwaiphatthana, W, Ruangdej, K, and Turner-Henson, A. Outdoor air pollution and children's health. Pediatr Nurs. (2010) 36:25–32.
11. Martínez-Martínez, MI, Alegre-Martínez, A, and Cauli, O. Prenatal exposure to phthalates and its effects upon cognitive and motor functions: a systematic review. Toxicology. (2021) 463:152980. doi: 10.1016/j.tox.2021.152980
12. Xue, J, Zartarian, V, Moya, J, Freeman, N, Beamer, P, Black, K, et al. A meta-analysis of children's hand-to-mouth frequency data for estimating nondietary ingestion exposure. Risk Anal. (2007) 27:411–20. doi: 10.1111/j.1539-6924.2007.00893.x
13. Zar, HJ, and Ferkol, TW. The global burden of respiratory disease-impact on child health. Pediatr Pulmonol. (2014) 49:430–4. doi: 10.1002/ppul.23030
14. Asher, MI, Montefort, S, Björkstén, B, Lai, CK, Strachan, DP, Weiland, SK, et al. Worldwide time trends in the prevalence of symptoms of asthma, allergic rhinoconjunctivitis, and eczema in childhood: ISAAC phases one and three repeat multicountry cross-sectional surveys. Lancet. (2006) 368:733–43. doi: 10.1016/s0140-6736(06)69283-0
15. Moorman, JE, Rudd, RA, Johnson, CA, King, M, Minor, P, Bailey, C, et al. National surveillance for asthma--United States, 1980–2004. MMWR Surveill Summ. (2007) 56:1–54.
16. Weinmayr, G, Weiland, SK, Björkstén, B, Brunekreef, B, Büchele, G, Cookson, WOC, et al. Atopic sensitization and the international variation of asthma symptom prevalence in children. Am J Respir Crit Care Med. (2007) 176:565–74. doi: 10.1164/rccm.200607-994OC
17. Marciniuk, DD, and Schraufnagel, DE. The global impact of respiratory disease European Respiratory Society (2017).
18. Dharmage, SC, Perret, JL, and Custovic, A. Epidemiology of asthma in children and adults. Front Pediatr. (2019) 7:246. doi: 10.3389/fped.2019.00246
19. Global Asthma Network, "The global asthma report 2014," Auckland, New Zealand: Global Asthma Network, vol. 769, pp. 28–36. (2014).
20. Akinbami, LJ, Moorman, JE, Garbe, PL, and Sondik, EJ. Status of childhood asthma in the United States, 1980–2007. Pediatrics. (2009) 123:S131–45. doi: 10.1542/peds.2008-2233C
21. Lange, P, Celli, B, Agustí, A, Boje Jensen, G, Divo, M, Faner, R, et al. Lung-function trajectories leading to chronic obstructive pulmonary disease. N Engl J Med. (2015) 373:111–22. doi: 10.1056/NEJMoa1411532
22. Stern, DA, Morgan, WJ, Wright, AL, Guerra, S, and Martinez, FD. Poor airway function in early infancy and lung function by age 22 years: a non-selective longitudinal cohort study. Lancet. (2007) 370:758–64. doi: 10.1016/s0140-6736(07)61379-8
23. Berry, CE, Billheimer, D, Jenkins, IC, Lu, ZJ, Stern, DA, Gerald, LB, et al. A distinct low lung function trajectory from childhood to the fourth decade of life. Am J Respir Crit Care Med. (2016) 194:607–12. doi: 10.1164/rccm.201604-0753OC
24. Martinez, FD. The origins of asthma and chronic obstructive pulmonary disease in early life. Proc Am Thorac Soc. (2009) 6:272–7. doi: 10.1513/pats.200808-092RM
25. Agusti, A, Noell, G, Brugada, J, and Faner, R. Lung function in early adulthood and health in later life: a transgenerational cohort analysis. Lancet Respir Med. (2017) 5:935–45. doi: 10.1016/S2213-2600(17)30434-4
26. Agusti, A, and Faner, R. Lung function trajectories in health and disease. Lancet Respir Med. (2019) 7:358–64. doi: 10.1016/S2213-2600(18)30529-0
27. Abellan, A, Sunyer, J, Garcia-Esteban, R, Basterrechea, M, Duarte-Salles, T, Ferrero, A, et al. Prenatal exposure to organochlorine compounds and lung function during childhood. Environ Int. (2019) 131:105049. doi: 10.1016/j.envint.2019.105049
28. Kajekar, R. Environmental factors and developmental outcomes in the lung. Pharmacol Ther. (2007) 114:129–45. doi: 10.1016/j.pharmthera.2007.01.011
29. Hanson, M, and Gluckman, P. Developmental origins of noncommunicable disease: population and public health implications. Am J Clin Nutr. (2011) 94:S1754–8. doi: 10.3945/ajcn.110.001206
30. Hanson, MA, Poston, L, and Gluckman, PD. DOHaD – the challenge of translating the science to policy. J Dev Orig Health Dis. (2019) 10:263–7. doi: 10.1017/S2040174419000205
31. Heyob, KM, Mieth, S, Sugar, SS, Graf, AE, Lallier, SW, Britt, RD Jr, et al. Maternal high-fat diet alters lung development and function in the offspring. Am J Physiol Lung Cell Mol Physiol. (2019) 317:L167–74. doi: 10.1152/ajplung.00331.2018
32. Wang, M, Hossain, F, Sulaiman, R, and Ren, X. Exposure to inorganic arsenic and lead and autism spectrum disorder in children: a systematic review and meta-analysis. Chem Res Toxicol. (2019) 32:1904–19. doi: 10.1021/acs.chemrestox.9b00134
33. Melen, E, and Guerra, S. Recent advances in understanding lung function development. F1000Res. (2017) 6:726. doi: 10.12688/f1000research.11185.1
34. Rosa, MJ, Lee, AG, and Wright, RJ. Evidence establishing a link between prenatal and early-life stress and asthma development. Curr Opin Allergy Clin Immunol. (2018) 18:148–58. doi: 10.1097/ACI.0000000000000421
35. Pinkerton, KE, and Joad, JP. Influence of air pollution on respiratory health during perinatal development. Clin Exp Pharmacol Physiol. (2006) 33:269–72. doi: 10.1111/j.1440-1681.2006.04357.x
36. McRae, N, Gennings, C, Rivera Rivera, N, Tamayo-Ortiz, M, Pantic, I, Amarasiriwardena, C, et al. Association between prenatal metal exposure and adverse respiratory symptoms in childhood. Environ Res. (2022) 205:112448. doi: 10.1016/j.envres.2021.112448
37. De Luca, G, Olivieri, F, Melotti, G, Aiello, G, Lubrano, L, and Boner, AL. Fetal and early postnatal life roots of asthma. J Matern Fetal Neonatal Med. (2010) 23:80–3. doi: 10.3109/14767058.2010.509931
38. Lin, P-ID, Cardenas, A, Rifas-Shiman, SL, Hivert, MF, James-Todd, T, Amarasiriwardena, C, et al. Diet and erythrocyte metal concentrations in early pregnancy—cross-sectional analysis in project viva. Am J Clin Nutr. (2021) 114:540–9. doi: 10.1093/ajcn/nqab088
39. Ruan, F, Zhang, J, Liu, J, Sun, X, Li, Y, Xu, S, et al. Association between prenatal exposure to metal mixtures and early childhood allergic diseases. Environ Res. (2022) 206:112615. doi: 10.1016/j.envres.2021.112615
40. Kim, J, Kim, S, Woo, SY, Chung, JY, Hong, YS, Oh, SY, et al. Prenatal exposure to Lead and chromium is associated with IL-13 levels in umbilical cord blood and severity of atopic dermatitis: COCOA study. Immune Netw. (2019) 19:e42. doi: 10.4110/in.2019.19.e42
41. Lee, S, Park, SK, Park, H, Lee, W, Kwon, JH, Hong, YC, et al. Prenatal heavy metal exposures and atopic dermatitis with gender difference in 6-month-old infants using multipollutant analysis. Environ Res. (2021) 195:110865. doi: 10.1016/j.envres.2021.110865
42. Pesce, G, Sesé, L, Calciano, L, Travert, B, Dessimond, B, Maesano, CN, et al. Foetal exposure to heavy metals and risk of atopic diseases in early childhood. Pediatr Allergy Immunol. (2021) 32:242–50. doi: 10.1111/pai.13397
43. Tsai, T-L, Lei, W-T, Kuo, C-C, Sun, H-L, Su, P-H, and Wang, S-L. Maternal and childhood exposure to inorganic arsenic and airway allergy–a 15-year birth cohort follow-up study. Environ Int. (2021) 146:106243. doi: 10.1016/j.envint.2020.106243
44. Jedrychowski, W, Perera, F, Maugeri, U, Miller, RL, Rembiasz, M, Flak, E, et al. Intrauterine exposure to lead may enhance sensitization to common inhalant allergens in early childhood: a prospective prebirth cohort study. Environ Res. (2011) 111:119–24. doi: 10.1016/j.envres.2010.11.002
45. Rosa, MJ, Benedetti, C, Peli, M, Donna, F, Nazzaro, M, Fedrighi, C, et al. Association between personal exposure to ambient metals and respiratory disease in Italian adolescents: a cross-sectional study. BMC Pulm Med. (2016) 16:1–9. doi: 10.1186/s12890-016-0173-9
46. Little, BB, Ignasiak, Z, Sławinska, T, Posłuszny, P, Malina, R, and Wiegman, DL. Blood lead levels, pulmonary function and agility in polish schoolchildren. Ann Hum Biol. (2017) 44:723–8. doi: 10.1080/03014460.2017.1387284
47. Madrigal, JM, Persky, V, Pappalardo, A, and Argos, M. Association of heavy metals with measures of pulmonary function in children and youth: results from the National Health and nutrition examination survey (NHANES). Environ Int. (2018) 121:871–8. doi: 10.1016/j.envint.2018.09.045
48. Zheng, G, Xu, X, Li, B, Wu, K, Yekeen, TA, and Huo, X. Association between lung function in school children and exposure to three transition metals from an e-waste recycling area. J Expo Sci Environ Epidemiol. (2013) 23:67–72. doi: 10.1038/jes.2012.84
49. Rosa, MJ, Tamayo-Ortiz, M, Mercado Garcia, A, Rivera Rivera, NY, Bush, D, Lee, AG, et al. Prenatal lead exposure and childhood lung function: influence of maternal cortisol and child sex. Environ Res. (2022) 205:112447. doi: 10.1016/j.envres.2021.112447
50. Dragon, J, Hoaglund, M, Badireddy, AR, Nielsen, G, Schlezinger, J, and Shukla, A. Perfluoroalkyl substances (PFAS) affect inflammation in lung cells and tissues. Int J Mol Sci. (2023) 24:8539. doi: 10.3390/ijms24108539
51. Zhu, W, Khan, K, Roakes, H, Maker, E, Underwood, KL, Zemba, S, et al. Vermont-wide assessment of anthropogenic background concentrations of perfluoroalkyl substances in surface soils. J Hazard Mater. (2022) 438:129479. doi: 10.1016/j.jhazmat.2022.129479
52. Kurwadkar, S, Dane, J, Kanel, SR, Nadagouda, MN, Cawdrey, RW, Ambade, B, et al. Per-and polyfluoroalkyl substances in water and wastewater: a critical review of their global occurrence and distribution. Sci Total Environ. (2022) 809:151003. doi: 10.1016/j.scitotenv.2021.151003
53. Sznajder-Katarzyńska, K, Surma, M, and Cieślik, I. A review of perfluoroalkyl acids (PFAAs) in terms of sources, applications, human exposure, dietary intake, toxicity, legal regulation, and methods of determination. J Chem. (2019) 2019:1–20. doi: 10.1155/2019/2717528
54. Ahmad, S, Wen, Y, and Irudayaraj, JMK. PFOA induces alteration in DNA methylation regulators and SARS-CoV-2 targets Ace2 and Tmprss2 in mouse lung tissues. Toxicol Rep. (2021) 8:1892–8. doi: 10.1016/j.toxrep.2021.11.014
55. Sørli, JB, Låg, M, Ekeren, L, Perez-Gil, J, Haug, LS, da Silva, E, et al. Per-and polyfluoroalkyl substances (PFASs) modify lung surfactant function and pro-inflammatory responses in human bronchial epithelial cells. Toxicol In Vitro. (2020) 62:104656. doi: 10.1016/j.tiv.2019.104656
56. Welch, BM, Keil, AP, Buckley, JP, Calafat, AM, Christenbury, KE, Engel, SM, et al. Associations between prenatal urinary biomarkers of phthalate exposure and preterm birth: a pooled study of 16 US cohorts. JAMA Pediatr. (2022) 176:895–905. doi: 10.1001/jamapediatrics.2022.2252
57. Zhou, X, Dong, T, Fan, Z, Peng, Y, Zhou, R, Wang, X, et al. Perfluorodecanoic acid stimulates NLRP3 inflammasome assembly in gastric cells. Sci Rep. (2017) 7:45468. doi: 10.1038/srep45468
58. Shao, W, Xu, J, Xu, C, Weng, Z, Liu, Q, Zhang, X, et al. Early-life perfluorooctanoic acid exposure induces obesity in male offspring and the intervention role of chlorogenic acid. Environ Pollut. (2021) 272:115974. doi: 10.1016/j.envpol.2020.115974
59. Dong, GH, Tung, KY, Tsai, CH, Liu, MM, Wang, D, Liu, W, et al. Serum polyfluoroalkyl concentrations, asthma outcomes, and immunological markers in a case-control study of Taiwanese children. Environ Health Perspect. (2013) 121:507–13. doi: 10.1289/ehp.1205351
60. Chang, HY, Suh, DI, Yang, SI, Kang, MJ, Lee, SY, Lee, E, et al. Prenatal maternal distress affects atopic dermatitis in offspring mediated by oxidative stress. J Allergy Clin Immunol. (2016) 138:468–475.e5. doi: 10.1016/j.jaci.2016.01.020
61. Ryu, MH, Jha, A, Ojo, OO, Mahood, TH, Basu, S, Detillieux, KA, et al. Chronic exposure to perfluorinated compounds: impact on airway hyperresponsiveness and inflammation. Am J Physiol Lung Cell Mol Physiol. (2014) 307:L765–74. doi: 10.1152/ajplung.00100.2014
62. Begley, TH, White, K, Honigfort, P, Twaroski, ML, Neches, R, and Walker, RA. Perfluorochemicals: potential sources of and migration from food packaging. Food Addit Contam. (2005) 22:1023–31. doi: 10.1080/02652030500183474
63. Smit, LA, Lenters, V, Høyer, BB, Lindh, CH, Pedersen, HS, Liermontova, I, et al. Prenatal exposure to environmental chemical contaminants and asthma and eczema in school-age children. Allergy. (2015) 70:653–60. doi: 10.1111/all.12605
64. Schecter, A, Colacino, J, Haffner, D, Patel, K, Opel, M, Päpke, O, et al. Perfluorinated compounds, polychlorinated biphenyls, and organochlorine pesticide contamination in composite food samples from Dallas, Texas, USA. Environ Health Perspect. (2010) 118:796–802. doi: 10.1289/ehp.0901347
65. Impinen, A, Nygaard, UC, Lødrup Carlsen, KC, Mowinckel, P, Carlsen, KH, Haug, LS, et al. Prenatal exposure to perfluoralkyl substances (PFASs) associated with respiratory tract infections but not allergy- and asthma-related health outcomes in childhood. Environ Res. (2018) 160:518–23. doi: 10.1016/j.envres.2017.10.012
66. Averina, M, Brox, J, Huber, S, Furberg, AS, and Sørensen, M. Serum perfluoroalkyl substances (PFAS) and risk of asthma and various allergies in adolescents. The Tromsø study fit futures in northern Norway. Environ Res. (2019) 169:114–21. doi: 10.1016/j.envres.2018.11.005
67. Huang, H, Yu, K, Zeng, X, Chen, Q, Liu, Q, Zhao, Y, et al. Association between prenatal exposure to perfluoroalkyl substances and respiratory tract infections in preschool children. Environ Res. (2020) 191:110156. doi: 10.1016/j.envres.2020.110156
68. Katsikantami, I, Sifakis, S, Tzatzarakis, MN, Vakonaki, E, Kalantzi, OI, Tsatsakis, AM, et al. A global assessment of phthalates burden and related links to health effects. Environ Int. (2016) 97:212–36. doi: 10.1016/j.envint.2016.09.013
69. Qian, Y, Shao, H, Ying, X, Huang, W, and Hua, Y. The endocrine disruption of prenatal phthalate exposure in mother and offspring. Front Public Health. (2020) 8:366. doi: 10.3389/fpubh.2020.00366
70. Jurewicz, J, and Hanke, W. Exposure to phthalates: reproductive outcome and children health. A review of epidemiological studies. Int J Occup Med Environ Health. (2011) 24:115–41. doi: 10.2478/s13382-011-0022-2
71. Wu, H, Kupsco, AJ, Deierlein, AL, Just, AC, Calafat, AM, Oken, E, et al. Trends and patterns of phthalates and phthalate alternatives exposure in pregnant women from Mexico City during 2007–2010. Environ Sci Technol. (2020) 54:1740–9. doi: 10.1021/acs.est.9b05836
72. Lyche, JL, Gutleb, AC, Bergman, Å, Eriksen, GS, Murk, ATJ, Ropstad, E, et al. Reproductive and developmental toxicity of phthalates. J Toxicol Environ Health B Crit Rev. (2009) 12:225–49. doi: 10.1080/10937400903094091
73. Mose, T, Mortensen, GK, Hedegaard, M, and Knudsen, LE. phthalate monoesters in perfusate from a dual placenta perfusion system, the placenta tissue and umbilical cord blood. Reprod Toxicol. (2007) 23:83–91. doi: 10.1016/j.reprotox.2006.08.006
74. Preece, AS, Knutz, M, Lindh, CH, Bornehag, CG, and Shu, H. Prenatal phthalate exposure and early childhood wheeze in the SELMA study. J Expo Sci Environ Epidemiol. (2022) 32:303–11. doi: 10.1038/s41370-021-00382-w
75. Seymore, TN, Rivera-Núñez, Z, Stapleton, PA, Adibi, JJ, and Barrett, ES. Phthalate exposures and placental health in animal models and humans: a systematic review. Toxicol Sci. (2022) 188:153–79. doi: 10.1093/toxsci/kfac060
76. Vernet, C, Pin, I, Giorgis-Allemand, L, Philippat, C, Benmerad, M, Quentin, J, et al. In utero exposure to select phenols and phthalates and respiratory health in five-year-old boys: a prospective study. Environ Health Perspect. (2017) 125:097006. doi: 10.1289/EHP1015
77. Berger, K, Eskenazi, B, Balmes, J, Kogut, K, Holland, N, Calafat, AM, et al. Prenatal high molecular weight phthalates and bisphenol a, and childhood respiratory and allergic outcomes. Pediatr Allergy Immunol. (2019) 30:36–46. doi: 10.1111/pai.12992
78. Rosicarelli, B, and Stefanini, S. DEHP effects on histology and cell proliferation in lung of newborn rats. Histochem Cell Biol. (2009) 131:491–500. doi: 10.1007/s00418-008-0550-4
79. Adgent, MA, Carroll, KN, Hazlehurst, MF, Loftus, CT, Szpiro, AA, Karr, CJ, et al. A combined cohort analysis of prenatal exposure to phthalate mixtures and childhood asthma. Environ Int. (2020) 143:105970. doi: 10.1016/j.envint.2020.105970
80. Ku, HY, Su, PH, Wen, HJ, Sun, HL, Wang, CJ, Chen, HY, et al. Prenatal and postnatal exposure to phthalate esters and asthma: a 9-year follow-up study of a taiwanese birth cohort. PLoS One. (2015) 10:e0123309. doi: 10.1371/journal.pone.0123309
81. Gascon, M, Casas, M, Morales, E, Valvi, D, Ballesteros-Gómez, A, Luque, N, et al. Prenatal exposure to bisphenol a and phthalates and childhood respiratory tract infections and allergy. J Allergy Clin Immunol. (2015) 135:370–8. doi: 10.1016/j.jaci.2014.09.030
82. Jahreis, S, Trump, S, Bauer, M, Bauer, T, Thürmann, L, Feltens, R, et al. Maternal phthalate exposure promotes allergic airway inflammation over 2 generations through epigenetic modifications. J Allergy Clin Immunol. (2018) 141:741–53. doi: 10.1016/j.jaci.2017.03.017
83. Jøhnk, C, Høst, A, Husby, S, Schoeters, G, Timmermann, CAG, Kyhl, HB, et al. Maternal phthalate exposure and asthma, rhinitis and eczema in 552 children aged 5 years; a prospective cohort study. Environ Health. (2020) 19:1–10. doi: 10.1186/s12940-020-00586-x
84. Carvalho, FP. Pesticides, environment, and food safety. Food Energy Secur. (2017) 6:48–60. doi: 10.1002/fes3.108
85. Roberts, JR, Karr, CJ, Council on Environmental HealthPaulson, JA, Brock-Utne, AC, Brumberg, HL, et al. Pesticide exposure in children. Pediatrics. (2012) 130:e1765–88. doi: 10.1542/peds.2012-2758
86. Mora, AM, Hoppin, JA, Córdoba, L, Cano, JC, Soto-Martínez, M, Eskenazi, B, et al. Prenatal pesticide exposure and respiratory health outcomes in the first year of life: results from the infants' environmental health (ISA) study. Int J Hyg Environ Health. (2020) 225:113474. doi: 10.1016/j.ijheh.2020.113474
87. Buralli, RJ, Dultra, AF, and Ribeiro, H. Respiratory and allergic effects in children exposed to pesticides-a systematic review. Int J Environ Res Public Health. (2020) 17:2740. doi: 10.3390/ijerph17082740
88. Gilden, RCC, Harris, RL, Friedmann, EJ, Han, M, Hackney, AJ, Olorunyemi, E, et al. Systematic review: Association of Pesticide Exposure and Child Wheeze and asthma. Curr Pediatr Rev. (2023) 19:169–78. doi: 10.2174/1573396318666220510124457
89. Gascon, M, Vrijheid, M, Martínez, D, Ballester, F, Basterrechea, M, Blarduni, E, et al. Pre-natal exposure to dichlorodiphenyldichloroethylene and infant lower respiratory tract infections and wheeze. Eur Respir J. (2012) 39:1188–96. doi: 10.1183/09031936.00011711
90. Sunyer, J, Torrent, M, Muñoz-Ortiz, L, Ribas-Fitó, N, Carrizo, D, Grimalt, J, et al. Prenatal dichlorodiphenyldichloroethylene (DDE) and asthma in children. Environ Health Perspect. (2005) 113:1787–90. doi: 10.1289/ehp.8127
91. Raanan, R, Balmes, JR, Harley, KG, Gunier, RB, Magzamen, S, Bradman, A, et al. Decreased lung function in 7-year-old children with early-life organophosphate exposure. Thorax. (2016) 71:148–53. doi: 10.1136/thoraxjnl-2014-206622
92. Bukalasa, JS, Brunekreef, B, Brouwer, M, Koppelman, GH, Wijga, AH, Huss, A, et al. Associations of residential exposure to agricultural pesticides with asthma prevalence in adolescence: the PIAMA birth cohort. Environ Int. (2018) 121:435–42. doi: 10.1016/j.envint.2018.09.029
93. Hyland, C, Bradshaw, PT, Gunier, RB, Mora, AM, Kogut, K, Deardorff, J, et al. Associations between pesticide mixtures applied near home during pregnancy and early childhood with adolescent behavioral and emotional problems in the CHAMACOS study. Environ Epidemiol. (2021) 5:e150. doi: 10.1097/ee9.0000000000000150
94. Díaz-Criollo, S, Palma, M, Monroy-García, AA, Idrovo, AJ, Combariza, D, and Varona-Uribe, ME. Chronic pesticide mixture exposure including paraquat and respiratory outcomes among Colombian farmers. Ind Health. (2020) 58:15–21. doi: 10.2486/indhealth.2018-0111
95. Yang, L, Li, C, and Tang, X. The impact of PM2. 5 on the host defense of respiratory system. Front Cell Dev Biol. (2020) 8:91. doi: 10.3389/fcell.2020.00091
96. Hsu, C-Y, Chiang, H-C, Lin, S-L, Chen, M-J, Lin, T-Y, and Chen, Y-C. Elemental characterization and source apportionment of PM10 and PM2. 5 in the western coastal area of Central Taiwan. Sci Total Environ. (2016) 541:1139–50. doi: 10.1016/j.scitotenv.2015.09.122
97. Pinkerton, KE, Green, FH, Saiki, C, Vallyathan, V, Plopper, CG, Gopal, V, et al. Distribution of particulate matter and tissue remodeling in the human lung. Environ Health Perspect. (2000) 108:1063–9. doi: 10.1289/ehp.001081063
98. Schulze, F, Gao, X, Virzonis, D, Damiati, S, Schneider, MR, and Kodzius, R. Air quality effects on human health and approaches for its assessment through microfluidic chips. Genes. (2017) 8:244. doi: 10.3390/genes8100244
99. Xing, Y-F, Xu, Y-H, Shi, M-H, and Lian, Y-X. The impact of PM2. 5 on the human respiratory system. J Thorac Dis. (2016) 8:E69–74. doi: 10.3978/j.issn.2072-1439.2016.01.19
100. Kampa, M, and Castanas, E. Human health effects of air pollution. Environ Pollut. (2008) 151:362–7. doi: 10.1016/j.envpol.2007.06.012
101. Jedrychowski, WA, Perera, FP, Spengler, JD, Mroz, E, Stigter, L, Flak, E, et al. Intrauterine exposure to fine particulate matter as a risk factor for increased susceptibility to acute broncho-pulmonary infections in early childhood. Int J Hyg Environ Health. (2013) 216:395–401. doi: 10.1016/j.ijheh.2012.12.014
102. Ge, E, Lai, K, Xiao, X, Luo, M, Fang, Z, Zeng, Y, et al. Differential effects of size-specific particulate matter on emergency department visits for respiratory and cardiovascular diseases in Guangzhou, China. Environ Pollut. (2018) 243:336–45. doi: 10.1016/j.envpol.2018.08.068
103. Habre, R, Moshier, E, Castro, W, Nath, A, Grunin, A, Rohr, A, et al. The effects of PM2. 5 and its components from indoor and outdoor sources on cough and wheeze symptoms in asthmatic children. J Expo Sci Environ Epidemiol. (2014) 24:380–7. doi: 10.1038/jes.2014.21
104. Zheng, X-Y, Ding, H, Jiang, LN, Chen, SW, Zheng, JP, Qiu, M, et al. Association between air pollutants and asthma emergency room visits and hospital admissions in time series studies: a systematic review and meta-analysis. PLoS One. (2015) 10:e0138146. doi: 10.1371/journal.pone.0138146
105. Wiecki, TV, and Frank, MJ. A computational model of inhibitory control in frontal cortex and basal ganglia. Psychol Rev. (2013) 120:329–55. doi: 10.1037/a0031542
106. Depue, BE, Orr, J, Smolker, H, Naaz, F, and Banich, M. The organization of right prefrontal networks reveals common mechanisms of inhibitory regulation across cognitive, emotional, and motor processes. Cereb Cortex. (2016) 26:1634–46. doi: 10.1093/cercor/bhu324
107. Durston, S, Thomas, KM, Yang, Y, Uluğ, AM, Zimmerman, RD, and Casey, B. A neural basis for the development of inhibitory control. Dev Sci. (2002) 5:F9–F16. doi: 10.1111/1467-7687.00235
108. Diamond, A. Executive functions. Annu Rev Psychol. (2013) 64:135–68. doi: 10.1146/annurev-psych-113011-143750
109. Cragg, L. The development of stimulus and response interference control in midchildhood. Dev Psychol. (2016) 52:242–52. doi: 10.1037/dev0000074
110. Moriguchi, Y, and Hiraki, K. Prefrontal cortex and executive function in young children: a review of NIRS studies. Front Hum Neurosci. (2013) 7:867. doi: 10.3389/fnhum.2013.00867
111. Cowan, N. Working memory underpins cognitive development, learning, and education. Educ Psychol Rev. (2014) 26:197–223. doi: 10.1007/s10648-013-9246-y
112. Hocking, DR, Fritsche, S, Farhat, H, Atkinson, A, Bendak, H, and Menant, J. Working memory is a core executive function supporting dual-task locomotor performance across childhood and adolescence. J Exp Child Psychol. (2020) 197:104869. doi: 10.1016/j.jecp.2020.104869
113. Buttelmann, F, and Karbach, J. Development and plasticity of cognitive flexibility in early and middle childhood. Front Psychol. (2017) 8:1040. doi: 10.3389/fpsyg.2017.01040
114. van Schouwenburg, MR, Onnink, AMH, ter Huurne, N, Kan, CC, Zwiers, MP, Hoogman, M, et al. Cognitive flexibility depends on white matter microstructure of the basal ganglia. Neuropsychologia. (2014) 53:171–7. doi: 10.1016/j.neuropsychologia.2013.11.015
115. Cañas, J, Quesada, JF, Antolí, A, and Fajardo, I. Cognitive flexibility and adaptability to environmental changes in dynamic complex problem-solving tasks. Ergonomics. (2003) 46:482–501. doi: 10.1080/0014013031000061640
116. Diamond, A, and Lee, K. Interventions shown to aid executive function development in children 4 to 12 years old. Science. (2011) 333:959–64. doi: 10.1126/science.1204529
117. Bai, Y, Henry, J, Campana, O, and Wlodkowic, D. Emerging prospects of integrated bioanalytical systems in neuro-behavioral toxicology. Sci Total Environ. (2021) 756:143922. doi: 10.1016/j.scitotenv.2020.143922
118. Kori, RK, Singh, MK, Jain, AK, and Yadav, RS. Neurochemical and behavioral dysfunctions in pesticide exposed farm workers: a clinical outcome. Indian J Clin Biochem. (2018) 33:372–81. doi: 10.1007/s12291-018-0791-5
119. Irizar, A, Molinuevo, A, Andiarena, A, Jimeno-Romero, A, San Román, A, Broberg, K, et al. Prenatal manganese serum levels and neurodevelopment at 4 years of age. Environ Res. (2021) 197:111172. doi: 10.1016/j.envres.2021.111172
120. Kponee-Shovein, KZ, Weisskopf, MG, Grashow, R, Rotem, RS, Coull, BA, Schnaas, L, et al. Estimating the causal effect of prenatal lead exposure on prepulse inhibition deficits in children and adolescents. Neurotoxicology. (2020) 78:116–26. doi: 10.1016/j.neuro.2020.02.013
121. Polanska, K, Hanke, W, Pawlas, N, Wesolowska, E, Jankowska, A, Jagodic, M, et al. Sex-dependent impact of low-level lead exposure during prenatal period on child psychomotor functions. Int J Environ Res Public Health. (2018) 15:2263. doi: 10.3390/ijerph15102263
122. Kim, JH, Lee, A, Kim, SK, Moon, HB, Park, J, Choi, K, et al. Lead and mercury levels in repeatedly collected urine samples of young children: a longitudinal biomonitoring study. Environ Res. (2020) 189:109901. doi: 10.1016/j.envres.2020.109901
123. Rafiee, A, Delgado-Saborit, JM, Sly, PD, Quémerais, B, Hashemi, F, Akbari, S, et al. Environmental chronic exposure to metals and effects on attention and executive function in the general population. Sci Total Environ. (2020) 705:135911. doi: 10.1016/j.scitotenv.2019.135911
124. Soetrisno, FN, and Delgado-Saborit, JM. Chronic exposure to heavy metals from informal e-waste recycling plants and children's attention, executive function and academic performance. Sci Total Environ. (2020) 717:137099. doi: 10.1016/j.scitotenv.2020.137099
125. Merced-Nieves, FM, Arora, M, Wright, RO, and Curtin, P. Metal mixtures and neurodevelopment: recent findings and emerging principles. Curr Opin Toxicol. (2021) 26:28–32. doi: 10.1016/j.cotox.2021.03.005
126. Sanders, AP, Claus Henn, B, and Wright, RO. Perinatal and childhood exposure to cadmium, manganese, and metal mixtures and effects on cognition and behavior: a review of recent literature. Curr Environ Health Rep. (2015) 2:284–94. doi: 10.1007/s40572-015-0058-8
127. Sun, Y, Wang, Y, Fang, J, du, Y, Wang, M, Liu, X, et al. Exposure to metal mixtures may decrease children’s cognitive flexibility via gut microbiota. Environ Technol Innov. (2023) 29:103012. doi: 10.1016/j.eti.2023.103012
128. Lane, JM, Liu, SH, Pantic, I, Martinez-Medina, S, Téllez-Rojo, MM, Amarasiriwardena, C, et al. Sex-specific association between prenatal manganese exposure and working memory in school-aged children in Mexico city: an exploratory multi-media approach. Environ Pollut. (2023) 333:121965. doi: 10.1016/j.envpol.2023.121965
129. Grønnestad, R, Johanson, SM, Müller, MHB, Schlenk, D, Tanabe, P, Krøkje, Å, et al. Effects of an environmentally relevant PFAS mixture on dopamine and steroid hormone levels in exposed mice. Toxicol Appl Pharmacol. (2021) 428:115670. doi: 10.1016/j.taap.2021.115670
130. Foguth, RM, Flynn, RW, de Perre, C, Iacchetta, M, Lee, LS, Sepúlveda, MS, et al. Developmental exposure to perfluorooctane sulfonate (PFOS) and perfluorooctanoic acid (PFOA) selectively decreases brain dopamine levels in northern leopard frogs. Toxicol Appl Pharmacol. (2019) 377:114623. doi: 10.1016/j.taap.2019.114623
131. Chen, J, das, SR, la du, J, Corvi, MM, Bai, C, Chen, Y, et al. Chronic PFOS exposures induce life stage–specific behavioral deficits in adult zebrafish and produce malformation and behavioral deficits in F1 offspring. Environ Toxicol Chem. (2013) 32:201–6. doi: 10.1002/etc.2031
132. Wang, Y, Liu, W, Zhang, Q, Zhao, H, and Quan, X. Effects of developmental perfluorooctane sulfonate exposure on spatial learning and memory ability of rats and mechanism associated with synaptic plasticity. Food Chem Toxicol. (2015) 76:70–6. doi: 10.1016/j.fct.2014.12.008
133. Onishchenko, N, Fischer, C, Wan Ibrahim, WN, Negri, S, Spulber, S, Cottica, D, et al. Prenatal exposure to PFOS or PFOA alters motor function in mice in a sex-related manner. Neurotox Res. (2011) 19:452–61. doi: 10.1007/s12640-010-9200-4
134. Gaballah, S, Swank, A, Sobus, JR, Howey, XM, Schmid, J, Catron, T, et al. Evaluation of developmental toxicity, developmental neurotoxicity, and tissue dose in zebrafish exposed to GenX and other PFAS. Environ Health Perspect. (2020) 128:047005. doi: 10.1289/EHP5843
135. Johansson, N, Fredriksson, A, and Eriksson, P. Neonatal exposure to perfluorooctane sulfonate (PFOS) and perfluorooctanoic acid (PFOA) causes neurobehavioural defects in adult mice. Neurotoxicology. (2008) 29:160–9. doi: 10.1016/j.neuro.2007.10.008
136. Zhang, H, Yolton, K, Webster, GM, Ye, X, Calafat, AM, Dietrich, KN, et al. Prenatal and childhood perfluoroalkyl substances exposures and children's reading skills at ages 5 and 8 years. Environ Int. (2018) 111:224–31. doi: 10.1016/j.envint.2017.11.031
137. Hoffman, K, Webster, TF, Weisskopf, MG, Weinberg, J, and Vieira, VM. Exposure to polyfluoroalkyl chemicals and attention deficit/hyperactivity disorder in US children 12–15 years of age. Environ Health Perspect. (2010) 118:1762–7. doi: 10.1289/ehp.1001898
138. Vuong, AM, Yolton, K, Xie, C, Dietrich, KN, Braun, JM, Webster, GM, et al. Prenatal and childhood exposure to poly-and perfluoroalkyl substances (PFAS) and cognitive development in children at age 8 years. Environ Res. (2019) 172:242–8. doi: 10.1016/j.envres.2019.02.025
139. Skogheim, TS, Villanger, GD, Weyde, KVF, Engel, SM, Surén, P, Øie, MG, et al. Prenatal exposure to perfluoroalkyl substances and associations with symptoms of attention-deficit/hyperactivity disorder and cognitive functions in preschool children. Int J Hyg Environ Health. (2020) 223:80–92. doi: 10.1016/j.ijheh.2019.10.003
140. Vuong, AM, Yolton, K, Wang, Z, Xie, C, Webster, GM, Ye, X, et al. Childhood perfluoroalkyl substance exposure and executive function in children at 8 years. Environ Int. (2018) 119:212–9. doi: 10.1016/j.envint.2018.06.028
141. Carrizosa, C, Murcia, M, Ballesteros, V, Costa, O, Manzano-Salgado, CB, Ibarluzea, J, et al. Prenatal perfluoroalkyl substance exposure and neuropsychological development throughout childhood: the INMA project. J Hazard Mater. (2021) 416:125185. doi: 10.1016/j.jhazmat.2021.125185
142. Gump, BB, Wu, Q, Dumas, AK, and Kannan, K. Perfluorochemical (PFC) exposure in children: associations with impaired response inhibition. Environ Sci Technol. (2011) 45:8151–9. doi: 10.1021/es103712g
143. Oh, J, Schmidt, RJ, Tancredi, D, Calafat, AM, Roa, DL, Hertz-Picciotto, I, et al. Prenatal exposure to per-and polyfluoroalkyl substances and cognitive development in infancy and toddlerhood. Environ Res. (2021) 196:110939. doi: 10.1016/j.envres.2021.110939
144. Lien, G-W, Huang, CC, Shiu, JS, Chen, MH, Hsieh, WS, Guo, YL, et al. Perfluoroalkyl substances in cord blood and attention deficit/hyperactivity disorder symptoms in seven-year-old children. Chemosphere. (2016) 156:118–27. doi: 10.1016/j.chemosphere.2016.04.102
145. Wang, F, Zou, Y, Shen, Y, Zhong, Y, Lv, Y, Huang, D, et al. Synergistic impaired effect between smoking and manganese dust exposure on pulmonary ventilation function in Guangxi manganese-exposed workers healthy cohort (GXMEWHC). PLoS One. (2015) 10:e0116558. doi: 10.1371/journal.pone.0116558
146. Weng, X, Liang, H, Tan, Y, Chen, J, Fei, Q, Liu, S, et al. Mixed effects of perfluoroalkyl and polyfluoroalkyl substances exposure on cognitive function among people over 60 years old from NHANES. Environ Sci Pollut Res. (2022) 29:32093–104. doi: 10.1007/s11356-021-17789-5
147. Harris, MH, Oken, E, Rifas-Shiman, SL, Calafat, AM, Bellinger, DC, Webster, TF, et al. Prenatal and childhood exposure to per-and polyfluoroalkyl substances (PFAS) and child executive function and behavioral problems. Environ Res. (2021) 202:111621. doi: 10.1016/j.envres.2021.111621
148. Hu, J, Wu, C, Zheng, T, Zhang, B, Xia, W, Peng, Y, et al. Critical windows for associations between manganese exposure during pregnancy and size at birth: a longitudinal cohort study in Wuhan, China. Environ Health Perspect. (2018) 126:127006. doi: 10.1289/EHP3423
149. Holahan, MR, Smith, CA, Luu, BE, and Storey, KB. Preadolescent phthalate (DEHP) exposure is associated with elevated locomotor activity and reward-related behavior and a reduced number of tyrosine hydroxylase positive neurons in post-adolescent male and female rats. Toxicol Sci. (2018) 165:512–30. doi: 10.1093/toxsci/kfy171
150. Huang, W, Xiao, J, Shi, X, Zheng, S, Li, H, Liu, C, et al. Effects of di-(2-ethylhexyl) phthalate (DEHP) on behavior and dopamine signaling in zebrafish (Danio rerio). Environ Toxicol Pharmacol. (2022) 93:103885. doi: 10.1016/j.etap.2022.103885
151. Tran, CM, Do, TN, and Kim, K-T. Comparative analysis of neurotoxicity of six phthalates in zebrafish embryos. Toxics. (2021) 9:5. doi: 10.3390/toxics9010005
152. Farzanehfar, V, Naderi, N, Kobarfard, F, and Faizi, M. Determination of dibutyl phthalate neurobehavioral toxicity in mice. Food Chem Toxicol. (2016) 94:221–6. doi: 10.1016/j.fct.2016.05.006
153. Smith, CA. The evaluation of neurodevelopmental and Behavioural correlates of acute postnatal exposure to di (2-ethylhexyl) phthalate in rats Carleton University (2014).
154. Li, Y, Zhuang, M, Li, T, and Shi, N. Neurobehavioral toxicity study of dibutyl phthalate on rats following in utero and lactational exposure. J Appl Toxicol. (2009) 29:603–11. doi: 10.1002/jat.1447
155. Engel, SM, Miodovnik, A, Canfield, RL, Zhu, C, Silva, MJ, Calafat, AM, et al. Prenatal phthalate exposure is associated with childhood behavior and executive functioning. Environ Health Perspect. (2010) 118:565–71. doi: 10.1289/ehp.0901470
156. Factor-Litvak, P, Insel, B, Calafat, AM, Liu, X, Perera, F, Rauh, VA, et al. Persistent associations between maternal prenatal exposure to phthalates on child IQ at age 7 years. PLoS One. (2014) 9:e114003. doi: 10.1371/journal.pone.0114003
157. Cho, S-C, Bhang, SY, Hong, YC, Shin, MS, Kim, BN, Kim, JW, et al. Relationship between environmental phthalate exposure and the intelligence of school-age children. Environ Health Perspect. (2010) 118:1027–32. doi: 10.1289/ehp.0901376
158. Engel, SM, Villanger, GD, Nethery, RC, Thomsen, C, Sakhi, AK, Drover, SSM, et al. Prenatal phthalates, maternal thyroid function, and risk of attention-deficit hyperactivity disorder in the Norwegian mother and child cohort. Environ Health Perspect. (2018) 126:057004. doi: 10.1289/EHP2358
159. Kobrosly, RW, Evans, S, Miodovnik, A, Barrett, ES, Thurston, SW, Calafat, AM, et al. Prenatal phthalate exposures and neurobehavioral development scores in boys and girls at 6–10 years of age. Environ Health Perspect. (2014) 122:521–8. doi: 10.1289/ehp.1307063
160. Polanska, K, Ligocka, D, Sobala, W, and Hanke, W. Phthalate exposure and child development: the polish mother and child cohort study. Early Hum Dev. (2014) 90:477–85. doi: 10.1016/j.earlhumdev.2014.06.006
161. Zhang, Y, Chen, B, Zheng, L, and Wu, X. Study on the level of phthalates in human biological samples. Zhonghua Yu Fang Yi Xue Za Zhi. (2003) 37:429–34.
162. Park, S, Zimmerman, E, Huerta-Montañez, G, Rosario-Pabón, Z, Vélez-Vega, CM, Cordero, JF, et al. Gestational exposure to phthalates and phthalate replacements in relation to neurodevelopmental delays in early childhood. Toxics. (2023) 11:65. doi: 10.3390/toxics11010065
163. Dewey, D, Martin, JW, MacDonald, A, Kinniburgh, DW, Letourneau, N, Giesbrecht, GF, et al. Sex-specific associations between maternal phthalate exposure and neurodevelopmental outcomes in children at 2 years of age in the APrON cohort. Neurotoxicology. (2023) 98:48–60. doi: 10.1016/j.neuro.2023.07.005
164. Loftus, CT, Bush, NR, Day, DB, Ni, Y, Tylavsky, FA, Karr, CJ, et al. Exposure to prenatal phthalate mixtures and neurodevelopment in the conditions affecting neurocognitive development and learning in early childhood (CANDLE) study. Environ Int. (2021) 150:106409. doi: 10.1016/j.envint.2021.106409
165. Carrillo, G, Mehta, RK, and Johnson, NM. Neurocognitive effects of pesticides in children. Pediatr Neurotoxicol. (2016) 127–41. doi: 10.1007/978-3-319-32358-9_7
166. Korrick, SA, and Sagiv, SK. Polychlorinated biphenyls (PCBs), organochlorine pesticides, and neurodevelopment. Curr Opin Pediatr. (2008) 20:198–204. doi: 10.1097/MOP.0b013e3282f6a4e9
167. Thistle, JE, Ramos, A, Roell, KR, Choi, G, Manley, CK, Hall, AM, et al. Prenatal organophosphorus pesticide exposure and executive function in preschool-aged children in the Norwegian mother, father and child cohort study (MoBa). Environ Res. (2022) 212:113555. doi: 10.1016/j.envres.2022.113555
168. Bellinger, DC. An overview of environmental chemical exposures and neurodevelopmental impairments in children. Pediatr Med. (2018) 1:1–13.
169. Lucero, B, and Muñoz-Quezada, MT. Neurobehavioral, neuromotor, and neurocognitive effects in agricultural workers and their children exposed to pyrethroid pesticides: a review. Front Hum Neurosci. (2021) 15:648171. doi: 10.3389/fnhum.2021.648171
170. Zhang, Q, Chen, XZ, Huang, X, Wang, M, and Wu, J. The association between prenatal exposure to phthalates and cognition and neurobehavior of children-evidence from birth cohorts. Neurotoxicology. (2019) 73:199–212. doi: 10.1016/j.neuro.2019.04.007
171. Terry, AV Jr. Functional consequences of repeated organophosphate exposure: potential non-cholinergic mechanisms. Pharmacol Ther. (2012) 134:355–65. doi: 10.1016/j.pharmthera.2012.03.001
172. Binter, AC, Bannier, E, Saint-Amour, D, Simon, G, Barillot, C, Monfort, C, et al. Exposure of pregnant women to organophosphate insecticides and child motor inhibition at the age of 10-12 years evaluated by fMRI. Environ Res. (2020) 188:109859. doi: 10.1016/j.envres.2020.109859
173. Sagiv, SK, Kogut, K, Harley, K, Bradman, A, Morga, N, and Eskenazi, B. Gestational exposure to organophosphate pesticides and longitudinally assessed behaviors related to attention-deficit/hyperactivity disorder and executive function. Am J Epidemiol. (2021) 190:2420–31. doi: 10.1093/aje/kwab173
174. Cartier, C, Warembourg, C, le Maner-Idrissi, G, Lacroix, A, Rouget, F, Monfort, C, et al. Organophosphate insecticide metabolites in prenatal and childhood urine samples and intelligence scores at 6 years of age: results from the mother–child PELAGIE cohort (France). Environ Health Perspect. (2016) 124:674–80. doi: 10.1289/ehp.1409472
175. Ribas-Fitó, N, Torrent, M, Carrizo, D, Muñoz-Ortiz, L, Júlvez, J, Grimalt, JO, et al. In utero exposure to background concentrations of DDT and cognitive functioning among preschoolers. Am J Epidemiol. (2006) 164:955–62. doi: 10.1093/aje/kwj299
176. Sánchez Lizardi, P, O'Rourke, MK, and Morris, RJ. The effects of organophosphate pesticide exposure on Hispanic children's cognitive and behavioral functioning. J Pediatr Psychol. (2008) 33:91–101. doi: 10.1093/jpepsy/jsm047
177. Rodríguez, D, Barg, G, Queirolo, EI, Olson, JR, Mañay, N, and Kordas, K. Pyrethroid and Chlorpyrifos pesticide exposure, general intellectual abilities, and executive functions of school children from Montevideo, Uruguay. Int J Environ Res Public Health. (2023) 20:5288. doi: 10.3390/ijerph20075288
178. Comfort, N, and Re, DB. Sex-specific neurotoxic effects of organophosphate pesticides across the life course. Curr Environ Health Rep. (2017) 4:392–404. doi: 10.1007/s40572-017-0171-y
179. Horton, MK, Kahn, LG, Perera, F, Barr, DB, and Rauh, V. Does the home environment and the sex of the child modify the adverse effects of prenatal exposure to chlorpyrifos on child working memory? Neurotoxicol Teratol. (2012) 34:534–41. doi: 10.1016/j.ntt.2012.07.004
180. Andersen, HR, Debes, F, Wohlfahrt-Veje, C, Murata, K, and Grandjean, P. Occupational pesticide exposure in early pregnancy associated with sex-specific neurobehavioral deficits in the children at school age. Neurotoxicol Teratol. (2015) 47:1–9. doi: 10.1016/j.ntt.2014.10.006
181. Genc, S, Zadeoglulari, Z, Fuss, SH, and Genc, K. The adverse effects of air pollution on the nervous system. J Toxicol. (2012) 2012:782462. doi: 10.1155/2012/782462
182. Costa, LG, Cole, TB, Dao, K, Chang, Y-C, and Garrick, JM. Developmental impact of air pollution on brain function. Neurochem Int. (2019) 131:104580. doi: 10.1016/j.neuint.2019.104580
183. Calderón-Garcidueñas, L, Serrano-Sierra, A, Torres-Jardón, R, Zhu, H, Yuan, Y, Smith, D, et al. The impact of environmental metals in young urbanites' brains. Exp Toxicol Pathol. (2013) 65:503–11. doi: 10.1016/j.etp.2012.02.006
184. Zanchi, AC, Fagundes, LS, Barbosa, F Jr, Bernardi, R, Rhoden, CR, Saldiva, PHN, et al. Pre and post-natal exposure to ambient level of air pollution impairs memory of rats: the role of oxidative stress. Inhal Toxicol. (2010) 22:910–8. doi: 10.3109/08958378.2010.494313
185. Morgan, ZE, Bailey, MJ, Trifonova, DI, Naik, NC, Patterson, WB, Lurmann, FW, et al. Prenatal exposure to ambient air pollution is associated with neurodevelopmental outcomes at 2 years of age. Environ Health. (2023) 22:11. doi: 10.1186/s12940-022-00951-y
186. Lubczyńska, MJ, Muetzel, RL, el Marroun, H, Hoek, G, Kooter, IM, Thomson, EM, et al. Air pollution exposure during pregnancy and childhood and brain morphology in preadolescents. Environ Res. (2021) 198:110446. doi: 10.1016/j.envres.2020.110446
187. Calderón-Garcidueñas, L, Kulesza, RJ, Doty, RL, D'Angiulli, A, and Torres-Jardón, R. Megacities air pollution problems: Mexico City metropolitan area critical issues on the central nervous system pediatric impact. Environ Res. (2015) 137:157–69. doi: 10.1016/j.envres.2014.12.012
188. Chiu, Y-HM, Hsu, HHL, Coull, BA, Bellinger, DC, Kloog, I, Schwartz, J, et al. Prenatal particulate air pollution and neurodevelopment in urban children: examining sensitive windows and sex-specific associations. Environ Int. (2016) 87:56–65. doi: 10.1016/j.envint.2015.11.010
189. Guxens, M, Garcia-Esteban, R, Giorgis-Allemand, L, Forns, J, Badaloni, C, Ballester, F, et al. Air pollution during pregnancy and childhood cognitive and psychomotor development: six European birth cohorts. Epidemiology. (2014) 25:636–47. doi: 10.1097/EDE.0000000000000133
190. Harris, MH, Gold, DR, Rifas-Shiman, SL, Melly, SJ, Zanobetti, A, Coull, BA, et al. Prenatal and childhood traffic-related air pollution exposure and childhood executive function and behavior. Neurotoxicol Teratol. (2016) 57:60–70. doi: 10.1016/j.ntt.2016.06.008
191. Fryt, J, Pilecka, W, and Smolen, T. Does asthma disturb executive functions and self-regulation in children. Pol J Appl Psychol. (2013) 12:169–85.
192. Irani, F, Barbone, JM, Beausoleil, J, and Gerald, L. Is asthma associated with cognitive impairments? A meta-analytic review. J Clin Exp Neuropsychol. (2017) 39:965–78. doi: 10.1080/13803395.2017.1288802
193. Taha, H. Poor executive functions among children with moderate-into-severe asthma: evidence from WCST performance. Front Psychol. (2017) 8:793. doi: 10.3389/fpsyg.2017.00793
194. Bian, R, Zhang, Y, Yang, Y, Yin, Y, Zhao, X, Chen, H, et al. White matter integrity disruptions correlate with cognitive impairments in asthma. J Magn Reson Imaging. (2018) 48:748–56. doi: 10.1002/jmri.25946
195. Ray, M, Sano, M, Wisnivesky, JP, Wolf, MS, and Federman, AD. Asthma control and cognitive function in a cohort of elderly adults. J Am Geriatr Soc. (2015) 63:684–91. doi: 10.1111/jgs.13350
196. Bass, JL, Corwin, M, Gozal, D, Moore, C, Nishida, H, Parker, S, et al. The effect of chronic or intermittent hypoxia on cognition in childhood: a review of the evidence. Pediatrics. (2004) 114:805–16. doi: 10.1542/peds.2004-0227
197. McQuaid, EL, Weiss-Laxer, N, Kopel, SJ, Koinis Mitchell, D, Nassau, JH, Wamboldt, MZ, et al. Pediatric asthma and problems in attention, concentration, and impulsivity: disruption of the family management system. Fam Syst Health. (2008) 26:16–29. doi: 10.1037/1091-7527.26.1.16
198. Goldbeck, L, Koffmane, K, Lecheler, J, Thiessen, K, and Fegert, JM. Disease severity, mental health, and quality of life of children and adolescents with asthma. Pediatr Pulmonol. (2007) 42:15–22. doi: 10.1002/ppul.20509
199. Kroll, JL, and Ritz, T. Asthma, the central nervous system, and neurocognition: current findings, potential mechanisms, and treatment implications. Neurosci Biobehav Rev. (2023) 146:105063. doi: 10.1016/j.neubiorev.2023.105063
200. Liu, X, Plana-Ripoll, O, McGrath, JJ, Petersen, LV, Dharmage, SC, and Momen, NC. Bidirectional associations between asthma and types of mental disorders. J Allergy Clin Immunol Pract. (2023) 11:799–808.e14. doi: 10.1016/j.jaip.2022.11.027
201. Maier, SF, Watkins, LR, and Fleshner, M. Psychoneuroimmunology. The interface between behavior, brain, and immunity. Am Psychol. (1994) 49:1004–17. doi: 10.1037/0003-066X.49.12.1004
202. Toni, R. The neuroendocrine system: organization and homeostatic role. J Endocrinol Investig. (2004) 27:35–47.
203. Brown, ES, Vera, E, Frol, AB, Woolston, DJ, and Johnson, B. Effects of chronic prednisone therapy on mood and memory. J Affect Disord. (2007) 99:279–83. doi: 10.1016/j.jad.2006.09.004
204. Newcomb, RW, and Akhter, J. Respiratory failure from asthma: a marker for children with high morbidity and mortality. Am J Dis Child. (1988) 142:1041–4. doi: 10.1001/archpedi.1988.02150100035020
205. Brannan, JD, and Lougheed, MD. Airway hyperresponsiveness in asthma: mechanisms, clinical significance, and treatment. Front Physiol. (2012) 3:460. doi: 10.3389/fphys.2012.00460
206. Carruthers, D, and Harrison, B. Arterial blood gas analysis or oxygen saturation in the assessment of acute asthma? Thorax. (1995) 50:186–8. doi: 10.1136/thx.50.2.186
207. Moss, M, Franks, M, Briggs, P, Kennedy, D, and Scholey, A. Compromised arterial oxygen saturation in elderly asthma sufferers results in selective cognitive impairment. J Clin Exp Neuropsychol. (2005) 27:139–50. doi: 10.1080/13803390490515450
208. Rudolf, M, Riordan, J, Grant, B, Maberly, D, and Saunders, KB. Arterial blood gas tensions in acute severe asthma. Eur J Clin Investig. (1980) 10:55–62. doi: 10.1111/j.1365-2362.1980.tb00010.x
209. Guo, R-B, Sun, PL, Zhao, AP, Gu, J, Ding, X, Qi, J, et al. Chronic asthma results in cognitive dysfunction in immature mice. Exp Neurol. (2013) 247:209–17. doi: 10.1016/j.expneurol.2013.04.008
210. Mao, Y, Bajinka, O, Tang, Z, Qiu, X, and Tan, Y. Lung–brain axis: metabolomics and pathological changes in lungs and brain of respiratory syncytial virus-infected mice. J Med Virol. (2022) 94:5885–93. doi: 10.1002/jmv.28061
211. Li, C, Chen, W, Lin, F, Li, W, Wang, P, Liao, G, et al. Functional two-way crosstalk between brain and lung: the brain–lung axis. Cell Mol Neurobiol. (2023) 43:991–1003. doi: 10.1007/s10571-022-01238-z
212. Rummel, C, Del Rey, A, Bähr, L, Krüger, K, and Peters, E. 1st European Psychoneuroimmunology Network (EPN) Autumn School: lung-brain Axis in health and disease. Neuroimmunomodulation. (2022) 29:3–8. doi: 10.1159/000526565
213. Azzoni, R, and Marsland, BJ. The lung-brain axis: a new frontier in host-microbe interactions. Immunity. (2022) 55:589–91. doi: 10.1016/j.immuni.2022.03.015
214. Greve, HJ, Dunbar, AL, Lombo, CG, Ahmed, C, Thang, M, Messenger, EJ, et al. The bidirectional lung brain-axis of amyloid-β pathology: ozone dysregulates the peri-plaque microenvironment. Brain. (2023) 146:991–1005. doi: 10.1093/brain/awac113
215. Witzenrath, M, and Kuebler, WM. The Lung–Brain Axis in ventilator-induced brain injury: enter IL-6. Am J Respir Cell Mol Biol. (2021) 65:339–40. doi: 10.1165/rcmb.2021-0233ED
216. Bajinka, O, Simbilyabo, L, Tan, Y, Jabang, J, and Saleem, SA. Lung-brain axis. Crit Rev Microbiol. (2022) 48:257–69. doi: 10.1080/1040841x.2021.1960483
217. Mumaw, CL, Levesque, S, McGraw, C, Robertson, S, Lucas, S, Stafflinger, JE, et al. Microglial priming through the lung-brain axis: the role of air pollution-induced circulating factors. FASEB J. (2016) 30:1880–91. doi: 10.1096/fj.201500047
218. Zhang, L, Zhang, Y, Jiang, X, Mao, L, Xia, Y, Fan, Y, et al. Disruption of the lung-gut-brain axis is responsible for cortex damage induced by pulmonary exposure to zinc oxide nanoparticles. Toxicology. (2023) 485:153390. doi: 10.1016/j.tox.2022.153390
219. Erickson, MA, Jude, J, Zhao, H, Rhea, EM, Salameh, TS, Jester, W, et al. Serum amyloid a: an ozone-induced circulating factor with potentially important functions in the lung-brain axis. FASEB J. (2017) 31:3950–65. doi: 10.1096/fj.201600857RRR
220. Nilsen, FM, Ruiz, JDC, and Tulve, NS. A meta-analysis of stressors from the Total environment associated with Children's general cognitive ability. Int J Environ Res Public Health. (2020) 17:5451. doi: 10.3390/ijerph17155451
221. Murrison, LB, Brandt, EB, Myers, JB, and Hershey, GKK. Environmental exposures and mechanisms in allergy and asthma development. J Clin Invest. (2019) 129:1504–15. doi: 10.1172/jci124612
222. Santos, S, Maitre, L, Warembourg, C, Agier, L, Richiardi, L, Basagaña, X, et al. Applying the exposome concept in birth cohort research: a review of statistical approaches. Eur J Epidemiol. (2020) 35:193–204. doi: 10.1007/s10654-020-00625-4
223. Tanner, EM, Bornehag, CG, and Gennings, C. Repeated holdout validation for weighted quantile sum regression. MethodsX. (2019) 6:2855–60. doi: 10.1016/j.mex.2019.11.008
224. Bobb, JF, Valeri, L, Claus Henn, B, Christiani, DC, Wright, RO, Mazumdar, M, et al. Bayesian kernel machine regression for estimating the health effects of multi-pollutant mixtures. Biostatistics. (2015) 16:493–508. doi: 10.1093/biostatistics/kxu058
225. Stein, CM, Morris, NJ, and Nock, NL. Structural equation modeling. Methods Mol Biol. (2012) 850:495–512. doi: 10.1007/978-1-61779-555-8_27
226. Sánchez, BN, Budtz-Jørgensen, E, Ryan, LM, and Hu, H. Structural equation models: a review with applications to environmental epidemiology. J Am Stat Assoc. (2005) 100:1443–55. doi: 10.1198/016214505000001005
227. Budtz-Jørgensen, E, Keiding, N, Grandjean, P, and Weihe, P. Estimation of health effects of prenatal methylmercury exposure using structural equation models. Environ Health. (2002) 1:1–22. doi: 10.1186/1476-069X-1-2
228. Beran, TN, and Violato, C. Structural equation modeling in medical research: a primer. BMC Res Notes. (2010) 3:1–10. doi: 10.1186/1756-0500-3-267
229. Shan, G, Li, X, and Huang, W. AI-enabled wearable and flexible electronics for assessing full personal exposures. Innovation (Camb). (2020) 1:100031. doi: 10.1016/j.xinn.2020.100031
230. Koelmel, JP, Lin, EZ, Guo, P, Zhou, J, He, J, Chen, A, et al. Exploring the external exposome using wearable passive samplers – the China BAPE study. Environ Pollut. (2021) 270:116228. doi: 10.1016/j.envpol.2020.116228
Keywords: pediatric environmental health, asthma, executive function, respiratory health, exposome
Citation: Alcala CS, Lane JM, Midya V, Eggers S, Wright RO and Rosa MJ (2024) Exploring the link between the pediatric exposome, respiratory health, and executive function in children: a narrative review. Front. Public Health. 12:1383851. doi: 10.3389/fpubh.2024.1383851
Edited by:
Fenna Sille, Johns Hopkins University, United StatesReviewed by:
Arlette Setiawan, Padjadjaran University, IndonesiaChao Jiang, Zhejiang University, China
Copyright © 2024 Alcala, Lane, Midya, Eggers, Wright and Rosa. This is an open-access article distributed under the terms of the Creative Commons Attribution License (CC BY). The use, distribution or reproduction in other forums is permitted, provided the original author(s) and the copyright owner(s) are credited and that the original publication in this journal is cited, in accordance with accepted academic practice. No use, distribution or reproduction is permitted which does not comply with these terms.
*Correspondence: Cecilia S. Alcala, Y2VjaWxpYS5hbGNhbGFAbXNzbS5lZHU=
†These authors have contributed equally to this work