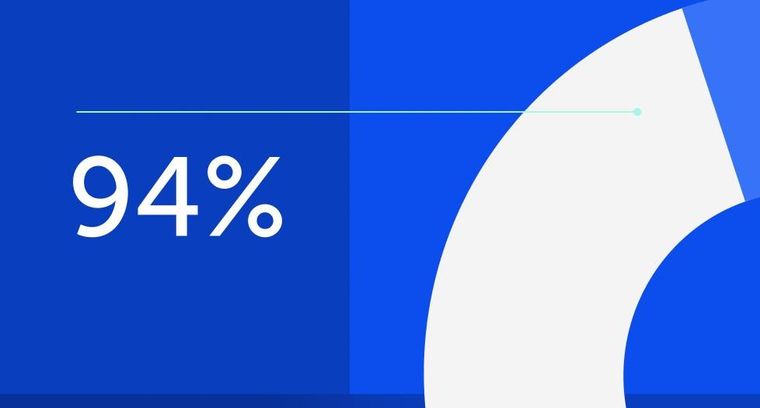
94% of researchers rate our articles as excellent or good
Learn more about the work of our research integrity team to safeguard the quality of each article we publish.
Find out more
ORIGINAL RESEARCH article
Front. Public Health, 08 March 2024
Sec. Environmental Health and Exposome
Volume 12 - 2024 | https://doi.org/10.3389/fpubh.2024.1340261
Organophosphate flame retardants (OPFRs) are emerging environmental pollutants that can be detected in water, dust, and biological organisms. Certain OPFRs can disrupt lipid metabolism in animal models and cell lines. However, the effects of OPFRs on human lipid metabolism remain unclear. We included 1,580 participants (≥20 years) from the 2013–2014 National Health and Nutrition Examination Survey (NHANES) to explore the relationship between OPFR exposure and lipid metabolism biomarkers. After adjusting for confounding factors, results showed that one-unit increases in the log levels of diphenyl phosphate (DPhP) (regression coefficient = −5.755; S.E. = 2.289; p = 0.023) and log bis-(1-chloro-2-propyl) phosphate (BCPP) (regression coefficient = −4.637; S.E. = 2.019; p = 0.036) were negatively associated with the levels of total cholesterol (TC) in all participants. One-unit increases in the levels of DPhP (regression coefficient = −2.292; S.E. = 0.802; p = 0.012), log bis (1,3-dichloro-2-propyl) phosphate (BDCPP) (regression coefficient = −2.046; S.E. = 0.825; p = 0.026), and log bis-2-chloroethyl phosphate (BCEP) (regression coefficient = −2.604; S.E. = 0.704; p = 0.002) were negatively associated with the levels of high-density lipoprotein cholesterol (HDL-C). With increasing quartiles of urine BDCPP levels, the mean TC levels significantly decreased in all participants (p value for trend = 0.028), and quartile increases in the levels of DPhP (p value for trend = 0.01), BDCPP (p value for trend = 0.001), and BCEP (p value for trend<0.001) were negatively corelated with HDL-C, with approximately 5.9, 9.9, and 12.5% differences between the upper and lower quartiles. In conclusion, DPhP, BDCPP, and BCEP were negatively related to HDL-C concentration, whereas DPhP and BCPP levels were negatively associated with TC level. Thus, exposure to OPFRs may interfere with lipid metabolism.
Cardiovascular disease (CVD) is a major leading cause of morbidity and mortality worldwide, and dyslipidemia is an established risk factor for CVD (1). Dyslipidemia is characterized by elevated serum total cholesterol (TC), low-density lipoprotein cholesterol (LDL-C), or triglyceride (TG) levels (2) and reduced serum high-density lipoprotein cholesterol (HDL-C) concentrations. Data from the 2007–2018 National Health and Nutrition Examination Survey (NHANES) showed that the prevalence rates of hypercholesterolemia (TC values ≥ 240 mg/dL) and hypertriglyceridemia (TG levels ≥ 200 mg/dL) were 11.5 and 10.4%, respectively (3). Dyslipidemia can originate from familial disorders (primary) or an alternative underlying etiology, such as metabolic disorder (diabetes, hypothyroidism), medications, unhealthy diet, and poor lifestyle regimen (4).
Organophosphate flame retardants (OPFRs) are ubiquitous in various environmental media because they are physically rather than chemically bound to a material, allowing these compounds to be easily released into the environment (5). Few toxicologic studies have demonstrated that OPFR exposure might interfere with lipid metabolism. TCP exposure might disturb the homeostasis and fluidity of lipid in in cerebrum, spinal cord and sciatic nerve (6). Evidence showed that the meta-isomer of TCP could alter hepatocytes lipid metabolism of seabream through interacting between liver X receptor α and proliferator-activated receptors (PPARs) proteins or modulating the expression levels of micro ribonucleic acids (7). Furthermore, TCP exposure could lead to increased lipid content and alter the fatty acid profile in human hepatocarcinoma (HepG2) cells through activation the pregnane X receptor pathway along with the deficient FA β-oxidation and enhanced lipogenesis (8). Triphenyl phosphate (TPhP, parent compound of diphenyl phosphate) inhibits specific liver carboxylesterases (CEs), altering hepatic lipid metabolism, inducing serum hypertriglyceridemia, and increasing very-low-density lipoprotein (VLDL) and LDL masses in mice (9). TPhP treatment significantly increases blood TC and TG concentrations and induces large lipid droplets in the livers of zebrafish possibly by inhibiting cholesterol utilization and liver lipid transfer (10). Lipid metabolism pathways, such as the fatty acid elongation pathway, are also significantly affected by TPhP exposure (10). TG levels increase and cholesterol levels significantly increase in hepatocytes exposed to high concentrations of tri-m-cresyl phosphate, one of the major isomers of commercial tricresyl phosphate (TCP), in gilthead sea bream (7). Le et al. (11) found that aryl-OPFRs (TPhP and TCP) and chlorinated-OPFRs, such as tris (1,3-dichloro-2-propyl) phosphate (TDCPP), cause lipid accumulation in mouse hepatic cells, accompanied with reduced mitochondrial (mito)-networks/cell, biased mitoATP/glcoATP rate, and expanded mito-area/cell.
Due to dyslipidemia being one of the major risk factors for cardiovascular diseases, toxicological studies have also indicated that exposure to OPFRs may interfere with lipid metabolism. OPFRs have been identified in various environments, including air, dust, water sources, soil, and sediments. Furthermore, traces of OPFRs have been detected in human samples and biotic organisms (12). However, the relationship between OPFRs exposure and lipid metabolism in humans remains unclear. Our study aims to investigate these associations through the analysis of the National Health and Nutrition Examination Survey (NHANES) database.
This study utilized the dataset from the 2013–2014 NHANES dataset in the United States. NHANES is a comprehensive, nationwide, population-based survey initiated in 1999 to evaluate the health and nutritional status of the U.S. population. The 2013–2014 NHANES was in review approved by the US National Center for Health Statistics Research Ethics Review Board (Continuation of Protocol #2011–17), and informed consent was obtained from all participants. The dataset and detailed survey protocols are provided on the NHANES website (13). In the 2013–2014 NHANES, one-third of the participants aged ≥6 years were randomly selected for the measurement of OPFR profiles in stored spot urine samples, and lipid profiles were examined in those who were ≥ 6 years of age and provided serum specimens. In our study, adult participants (≥ 20 years of age) of the 2013–2014 NHANES who had available urinary OPFR profiles and serum lipid data were enrolled for the analysis (n = 1,580, Figure 1). We selected the 2013–2014 NHANES data as it includes measurements of both Organophosphate Flame Retardants (OPFRs) and indicators of lipid metabolism.
The analytical procedure for the urinary OPFR profiles in the 2013–2014 NHANES has been described previously and is available on the NHANES website (13–15). Briefly, a 400 μL urine specimen was utilized for analysis in this study. Analyte extraction involved enzymatic hydrolysis of urinary conjugation followed by solid-phase extraction (SPE) using a 60 mg Strata XAW polymeric sorbent (Phenomenex, Torrance, CA, United States) with 1.5 mL liquid space. The target analytes in the extracts were separated using reversed-phase high-performance liquid chromatography (Agilent, 1,290, Agilent Technologies, Santa Clara, CA, United States) and quantified using isotope dilution-electrospray ionization tandem mass spectrometry (AB Sciex 5,500 Qtrap mass spectrometer, Applied Biosystems, Foster City, CA, United States). In the 2013–2014 NHANES, the following eight OPFR metabolites were measured in the eligible urine samples as exposure surrogates: bis (1-chloro-2-propyl) phosphate (BCPP), bis (1,3-dichloro-2-propyl) phosphate (BDCPP), bis (2-chloroethyl) phosphate (BCEP), diphenyl phosphate (DPhP), di-n-butyl phosphate (DnBP), di-p-cresyl phosphate, di-o-cresyl phosphate, and dibenzyl phosphate. We selected these indicators because previous NHANES data indicated detection rates of BCPP, BDCPP, BCEP, DnBP, and DPhP were above 60%, while other monitored OPFRs in NHANES, such as di-p-cresylphosphate (DpCP), di-o-cresylphosphate (DoCP), dibenzyl phosphate (DBzP), and 2,3,4,5-tetrabromobenzoic acid (TBBA), had detection rates below 20% (15). Therefore, this study investigates the relationship between BCPP, BDCPP, BCEP, DnBP, DPhP, and lipid metabolism. The limits of detection (LODs) were 0.10, 0.11, 0.08, and 0.16 μg/L for BCPP, BDCPP, BCEP, and DPhP, respectively, and 0.05 μg/L for other OPFR metabolites. Urinary metabolites of OPFRs with a detection rate of 50% or higher were considered for statistical analyses. For non-detected samples, a value of LOD/√2 was assigned to estimate urinary concentration during analysis.
At each study site, trained personnel followed the standardized protocol outlined on the NHANES website to collect blood specimens. Detailed procedures for specimen collection are provided in the NHANES Laboratory Procedures Manual (13). The data on TC, LDL-C, HDL-C, and TG were included for analysis in the present study.
In accordance with the NHANES protocols, sociodemographic profiles were collected during household interviews by well-trained interviewers using standardized questionnaires and a computer-assisted personal interview system. Body measurement data were recorded by trained health technicians in NHANES mobile examination centers following standardized procedures. Other procedure details are provided on the NHANES website (13). The age, sex, ethnicity, household income, smoking status, alcohol consumption, and body mass index (BMI) of all participants were recorded.
Categorical variables are presented as numbers (n) with percentages, and continuous variables are presented as medians with interquartile ranges. To identify baseline covariates associated with lipid profiles and further subgroup analysis, we stratified the study population into subgroups according to age (20–50 vs. >50 years), sex, ethnicity, household income (<4,500 vs. ≥4,500 USD/year), smoking status, alcohol consumption (<12 vs. ≥12 drink/year), and BMI (<25 vs. 25–30 vs. ≥30 kg/m2). The lipid profiles were compared between subgroups via the Mann–Whitney U test (for two subgroups) or Kruskal–Wallis H-test (for three or more subgroups). Baseline covariates with a p-value of <0.05 in univariate analyses were included in further multivariate analyses for adjustment. We conducted multiple linear regression analyses in the complex samples to explore the relationships between urinary OPFR metabolite concentrations and lipid profiles. The analyses were adjusted for baseline covariates, and sampling weights were applied in accordance with the National Center for Health Statistics Analytic Guidelines. Due to the non-normal distribution, urinary OPFR metabolite concentrations were subjected to logarithmic transformation and quartile stratification before the linear regression analysis. Statistical significance was set at a p < 0.05. Statistical Product and Service Solutions (version 22.0; IBM, Armonk, NY, United States) was used for all analyses.
The serum lipid profiles among different subgroups are listed in Table 1. The median (25, 75 percentile) values of serum TG, TC, LDL-C, and HDL-C among the participants were 118 (80, 190) mg/dL, 187 (160, 215) mg/dL, 107 (87, 132) mg/dL, and 50 (41, 61) mg/dL, respectively. Male (p < 0.001), older adults (>50 years, p = 0.001), less household income (<4,500 U.S. dollar, p = 0.043), and higher BMI (≥30, <0.001) participants had higher levels of TG. Male, older persons, less household income (<4,500 U.S. dollar), and higher BMI (≧25) participants had higher levels of TC. Male, older adults, less household income (<4,500 U.S. dollar), and higher BMI (≥25) participants had lower levels of HDL-C. The concentrations of OPFRs for each subgroup were shown in Supplementary Table S1.
The adjusted regression coefficients (S.E.) for the differences in TG, TC, LDL-C, and HDL-C relative to a one-unit increase in log-transformed DPhP are summarized in Table 2. A one-unit increase in the log DPhP level was negatively associated with TC (regression coefficient = −5.755; S.E. = 2.289; p = 0.023) and HDL-C (regression coefficient = −2.292; S.E. = 0.802; p = 0.012) in all participants. Subgroup analysis showed that the effects on TC levels (regression coefficient = −9.552; S.E. = 3.506; p = 0.016) and HDL-C (regression coefficient = −2.411; S.E. = 1.067; p = 0.039) were more prominent in the female group, whereas the effects on HDL-C levels (regression coefficient = −9.552; S.E. = 3.506; p = 0.016) were more prominent in the younger group (≤50 years).
Table 2. Adjusted regression coefficients (S.E.) for differences in lipid profile relative to a one-unit increase in log10-transformed diphenyl phosphate (DPhP), with results weighted for sampling strategy.
The adjusted regression coefficients (S.E.) for the differences in TG, TC, LDL-C, and HDL-C relative to a one-unit increase in log-transformed BDCPP are summarized in Table 3. We found that a one-unit increase in the log BDCPP level was negatively associated with the levels of HDL-C (regression coefficient = −2.046; S.E. = 0.825; p = 0.026). Subgroup analysis showed that the effects on TC levels (regression coefficient = −8.559; S.E. = 2.616; p = 0.005) were more prominent in the female group, whereas the effects on HDL-C levels were more prominent in the male (regression coefficient = −2.455; S.E. = 1.044; p = 0.033), older participant (>50 years, regression coefficient = −3.596; S.E. = 1.108; p = 0.005), and non-Hispanic White (>50 years, regression coefficient = −2.334; S.E. = 0.894; p = 0.02) groups.
Table 3. Adjusted regression coefficients (S.E.) for differences in TG, cholesterol, LDL, and HDL relative to a one-unit increase in log10-transformed biomarkers of bis(1,3-dichloro-2-propyl) phosphate (BDCPP), with results weighted for sampling strategy.
The adjusted regression coefficients (S.E.) for the differences in TG, TC, LDL-C, and HDL-C relative to a one-unit increase in the log-transformed BCPP level are summarized in Table 4. A one-unit increase in the log BCPP level was negatively associated with TC levels (regression coefficient = −4.637; S.E. = 2.019; p = 0.036). Subgroup analysis also showed the negative association of BCPP level with the levels of TG (regression coefficient = −13.286; S.E. = 5.626; p = 0.032) and TC (regression coefficient = −9.410; S.E. = 3.448; p = 0.016) in the female group.
Table 4. Adjusted regression coefficients (S.E.) for differences in TG, cholesterol, LDL, and HDL relative to a one-unit increase in log10-transformed biomarkers of bis-(1-chloro-2-propyl) phosphate (BCPP), with results weighted for sampling strategy.
The adjusted regression coefficients (S.E.) for the differences in TG, TC, LDL-C, and HDL-C relative to a one-unit increase in the log-transformed BCEP level are summarized in Table 5. A one-unit increase in the log BCEP level was negatively associated with the levels of HDL-C (regression coefficient = −2.604; S.E. = 0.704; p = 0.002), especially among the groups of female (regression coefficient = −2.959; S.E. = 1.003; p = 0.01), BMI less than 25 (regression coefficient = −4.449; S.E. = 1.259; p = 0.003), non-smoker (regression coefficient = −3.209; S.E. = 1.315; p = 0.028), less alcohol consumption (<12 drinks per year, regression coefficient = −3.423; S.E. = 0.881; p = 0.001), household income more than 4,500 USD (regression coefficient = −3.485; S.E. = 0.806; p = 0.001), and non-Hispanic white (regression coefficient = −3.307; S.E. = 0.850; p = 0.001).
Table 5. Adjusted regression coefficients (S.E.) for differences in TG, cholesterol, LDL, and HDL relative to a one-unit increase in log10-transformed biomarkers of bis-2-chloroethyl phosphate (BCEP), with results weighted for sampling strategy.
The adjusted regression coefficients (S.E.) for the differences in TG, TC, LDL-C, and HDL-C levels relative to a one-unit increase in the log-transformed DnBP level (μg/L) are summarized in Table 6. The association between a one-unit increase in the log DnBP level and TG, TC, LDL-C, and HDL-C levels did not achieve statistical significance in the overall group.
Table 6. Adjusted regression coefficients (S.E.) for differences in triglycerides, cholesterol, HDL-cholesterol, and LDL-cholesterol relative to a one-unit increase in log10-transformed di-n-butyl phosphate (DnBP), with results weighted for sampling strategy.
After adjusting for potential covariates in multiple regression analysis, the correlations between the quartiles of each OPFR and TC, as well as HDL-C in all participants and different sexes are listed in Figure 2. With increasing quartiles of urine BDCPP levels, the mean TC levels significantly decreased in all participants (p value for trend = 0.028) and the female group (p value for trend<0.001), whereas the mean differences in TC levels between the upper and lower quartiles of BDCPP in all participants and the female group were 3.4 and 5.8%, respectively. Furthermore, the quartile increase in urine BCEP level was negatively related to TC levels (p value for trend = 0.016) in the female group, with approximately 5.6% difference between the upper and lower quartiles. Quartile increases in the levels of DPhP (p value for trend = 0.01), BDCPP (p value for trend = 0.001), and BCEP (p value for trend<0.001) were negatively corelated with HDL-C, with approximately 5.9, 9.9, and 12.5% differences between the upper and lower quartiles. Conversely, we also observed gender differences in the impact of OPFRs on HDL-C. In males, DPhP (p value for trend = 0.017) and BDCPP (p value for trend = 0.019) levels were negatively correlated with HDL-C levels, with a decrease of approximately 10.4 and 9.3%, respectively, in the highest quartile of HDL-C compared with the lowest quartile. In contrast to the male group, the female group showed negative correlations of DPhP (p value for trend = 0.025), BDCPP (p value for trend = 0.009), and BCEP (p value for trend = 0.01) with HDL-C. The highest quartile of DPhP, BDCPP, and BCEP levels in the females was associated with approximately 5.2, 7.0, and 12.1% reductions in HDL-C, respectively, compared with the lowest quartile.
Figure 2. Mean and SE of cholesterol and HDL, across quartiles of OPFRs in linear regression models, with results weighted for sampling strategy. (A,B) Diphenyl phosphate (DPhP), (C,D) bis(1,3-dichloro-2-propyl) phosphate (BDCPP), (E,F) bis(1-chloro-2-propyl) phosphate (BCPP), (G,H) bis(2-chloroethyl) phosphate (BCEP), and (I,J) di-n-butyl phosphate (DnBP). HDL, high-density lipoprotein; SE, standard error.
In the present study, we observed a statistically significant association between OPFRs and lipid profiles. After adjusting for confounding factors, the DPhP level was negatively associated with TC and HDL-C levels, the BDCPP level was negatively associated with HDL-C levels, the BCPP level was negatively associated with TC levels, and the BCEP level was negatively associated with HDL-C levels. Furthermore, quartile increases in the levels of DPhP, BDCPP, and BCEP were negatively correlated with HDL-C, with approximately 5.9, 9.9, and 12.5% differences between the upper and lower quartiles.
Dyslipidemia is one of major risk factors for cardiovascular and cerebrovascular diseases, leading to an increased risk of atherosclerotic cardiovascular disease (16). Among lipid metabolites, HDL-C has been found to be associated with mortality. Li et al. followed 7,766 older adults individuals aged ≥65 years, and found that the group with HDL-C < 61 mg/dL had higher rates of all-cause mortality and cardiovascular-related mortality (17). Another research also indicated that for each 1 mg/dL increase in HDL-C, there is a 3.7 to 4.7% decrease in the rate of cardiovascular mortality (18). In our study, we observed a negative correlation between the levels of DPhP, BDCPP, and BCEP with HDL-C. This may suggest that populations with higher levels of DPhP, BDCPP, and BCEP could potentially have an increased risk of cardiovascular diseases. Further research is needed to clarify this association.
Recently, a few studies have investigated the effects of exposure to OPFRs on fatty acid metabolism. Hu et al. (19) found that the exposure of RAW264.7 macrophage cells to TPhP increases endoplasmic reticulum (ER) stress and inflammation, which further downregulate and decrease fatty acid saturation. Lpcat3 is one of the factors that regulate carbohydrate metabolism and adipocyte differentiation. Another study using Alpha mouse liver 12 cells found that exposure to two aryl-OPFRs (TCP and TPhP) and three chlorinated OPFRs (TDCPP, TCPP, and TCEP) causes intracellular lipid accumulation at relatively low concentrations (<10 μmol/L) for TCP, TPHP, and TDCPP. They also observed intracellular lipid accumulation at concentrations >10 μmol/L for TCPP and TCEP. This study also found that OPFRs increase oxidative stress and alter mitochondrial membrane potential in liver cells, thereby interfering with ATP metabolism and causing lipid accumulation (11). Meanwhile, CEs are responsible for hydrolyzing xenobiotic or endogenous compounds that contain ester, thioester, or amide groups (20). In the liver, CEs are responsible for metabolizing TGs and fatty acids in lipid droplets and resynthesizing them into VLDL in the ER, which is then released into the bloodstream, further affecting the metabolism of carbohydrates and esters and promoting insulin resistance (21). In a previous study, exposure to TPhP inhibits CE activity in the liver of mice, resulting in increased concentrations of LDL-C and VLDL in the serum (9). The reason for the inhibition of CEs may be that OPFRs irreversibly bind to the activation site of CEs, thereby inhibiting their function. Another study using Atlantic cod liver found that exposure to TCPP, 2-ethyldiphenyl phosphate, or their mixture downregulates the expression of genes involved in cholesterol synthesis and affects subsequent lipid metabolism (22). Cholesterol is a precursor for steroid hormones, such as follicle-stimulating hormone, luteinizing hormone, total testosterone, and total estradiol, and interference of cholesterol synthesis might lead to endocrine disruption. However, limited studies focused on the relationship between OPFR exposure and human lipid metabolism. The results of the present study showed that DPhP, BDCPP, and BCEP were negatively related to HDL-C, whereas DPhP and BCPP were negatively associated with TC. In addition, OPFRs exerted differential effects on lipid metabolism interference in males and females. Specifically, the negative correlation of DPhP, BDCPP, and BCEP with TC was more pronounced in females than in males. However, the association between OPFRs exposure and HDL-C showed less gender difference. Further investigation is warranted to clarify the reasons for this gender difference.
OPFRs are low-cost and effective flame retardants widely used in various consumer products, building materials, textiles, and electronics. They have been used to replace polybrominated diphenyl ethers (PBDEs) owing to the persistence, bioaccumulation, and toxicity of the latter. By 2011, OPFRs accounted for 20% of the global flame retardant market (23). They are also used as plasticizers for epoxy resins, coatings, engineering thermoplastics, and floor polishes. The consumption of OPFRs reached 83,000 tons in Europe and 72,000 tons in the United States in 2007, and the usage has grown at a rate of 3.7% annually from 2007 to 2012 (24). However, OPFRs are physically rather than chemically bound to the products, allowing them to easily detach from the products during use and enter the surrounding environment through volatilization, dissolution, deposition, and infiltration. OPFRs can be detected in various environmental and biological matrices, such as air (25), soil (26), water (27), fish (28), and even breast milk (29). Humans can be exposed to OPFRs through skin contact, inhalation, and ingestion (30). OPFRs with low logarithmic octanol-air coefficient values (Log Koa) mainly exist in the gas phase; hence, the contribution of these compounds in the air is greater than that in the dust. Exposure to volatile OPFRs such as TCPP and TCEP usually occurs through air inhalation. By contrast, OPFRs with high Log Koa are primarily in the particulate phase and settled on dust. Therefore, dust ingestion is the important exposure pathway for less volatile OPRFs, such as tris (2-butoxyethyl) phosphate (TBEP), TCP, and TPhP. A study from Vietnam reported that the total estimated daily intakes of ΣOPFRs via dermal absorption, air inhalation, and dust ingestion for toddlers and adults under medial exposure are 160 ngkg−1 day−1 and 36.7 gkg−1 day−1, respectively (31). The value is approximately 4–5 times greater in toddlers than in adults. Dermal absorption is the major exposure pathway for toddlers and adults (accounting for 45.1 and 49.5% ΣOPFRs, respectively), followed by air inhalation (contributing to 40 and 46.5% ΣOPFRs, respectively). Human OPFR exposure could be estimated by studying the concentration of OPFRs and their metabolites in bio-samples, such as urine, serum, semen, breast milk, and hair. Urine is a commonly used biomatrix because its collection is easy and noninvasive. OPFR metabolites in urine are linked to external OPFR exposure; for instance, the urinary concentration of DPhP, a metabolite of TPhP, has been associated with TPhP in handwipes and dust (32). Data extracted from the 2013–2014 NHANES showed that BDCIPP and DPhP were present in approximately 92% of the participants, BCEP in 89%, DnBP in 81%, and BCPP in 61% of the US general population (15). Among the OPFRs studied, DPhP had the highest concentration range (<0.16–193 μg/L), followed by BDCPP (<0.11–169 μg/L) and BCEP (<0.08–110 μg/L). Bis (1-chloro-2-propyl) 1-hydroxy-2-propyl phosphate (a metabolite of TCPP) and DPHP were the most frequently detected compounds in urine (detection frequency > 98%) and the most abundant compounds in urine, accounting for 46% (median level 720 pg./mL) and 39% (medial level 610 pg./mL) of ΣOPFRs, respectively, in one study from Norway (12). The detection frequencies in urine were greater than 90% for DPhP, DnBP (metabolites of tri-n-butyl phosphate), bis-(2-butoxyethyl) phosphate (a metabolite of TBEP), and dicresyl phosphate (a metabolite of TCP), with relatively low detection frequencies of BDCPP (76%) and BCEP (71%) in southern China (33). Among all OPRFs investigated in the present study, DPhP (0.55 ng/mL) exhibited the highest mean level, followed by BCEP (0.72 ng/mL), DnBP (0.29 ng/mL), and BCPP (0.094 ng/mL). The overall urinary detection rate of OPFRs was 98.8% in a chronic kidney disease population in Taiwan (34). In the present study, the detection rate and median level were 78.31% and 0.134 μg/g creatinine (Cr) for DPhP, 78.31% and 0.212 μg/g Cr for TBEP, 64.46% and 0.025 μg/g Cr, and 59.64% and 0.186 μg/g Cr for BBOEP, respectively. Universal exposure to OPFRs was proved by growing evidence, and the disturbance of OPFR exposure on lipid metabolism might result in more and more adverse health impacts.
Dyslipidemia is an important risk factor for atherosclerotic CVD and ischemic cerebrovascular accident (CVA). Insulin resistance, which is associated with metabolic syndrome, increases plasma TG and LDL-C levels and reduces HDL-C levels, thereby increasing the risk for atherosclerotic CVD, CVA, and peripheral artery disease. High-density lipoproteins are involved in delaying the formation of atherosclerotic lesions through several mechanisms, such as removal of cholesterol from macrophages within the arterial wall and transportation to the liver for excretion (35, 36). Observational studies found that a 1 mg/dL (0.026 mmol/L) increase in HDL-C is associated with a 3% risk reduction of coronary heart disease in women and 2% risk reduction in men, irrespective of age, body mass index, smoking habit, blood pressure, and LDL-C level (16). In a nationwide, community-based, prospective cohort study in the US, the risk for all-cause mortality was significantly higher in the group with HDL-C concentrations <61 mg/dL than in the group with HDL-C concentrations ranging from 61 to 87 mg/dL among older adults (aged ≥65 years). Repeatedly measured low HDL-C levels (defined as <40 mg/dL for men and < 50 mg/dL for women) have been associated with the risk of thyroid cancer, and this correlation is stronger in metabolically unhealthy Korean persons (37). Data from a large German primary care provider database showed that low HDL-C concentrations (<40 mg/dL) are positively associated and elevated TC levels (>200 mg/dL) are negatively associated with cancer, irrespective of diabetes, obesity, age, and sex. By contrast, serum levels of TG and LDL pose no impact on cancer risk (38). In the present study, exposure to DPhP, BDCPP, and TCEP were negatively associated with HDL-C. However, whether these negative associations result in adverse health outcome merits further investigation.
The majority of total cellular cholesterol is localized on the plasma membranes and interacts with the adjacent lipids to regulate the rigidity, fluidity, and permeability of the cell membrane. Cholesterol could bind to numerous transmembrane proteins and either maintain or alter their conformation. It can also interact with several transport proteins that facilitate cholesterol trafficking and regulate the subcellular distribution. In addition to their roles in membrane structure and function, derivatives of cholesterol are engaged in various biological processes, such as steroid hormone generation and bile acid production. The homeostasis of cholesterol is determined by de novo biosynthesis, uptake, export, and storage (39). Negative associations of DPhP and BCPP levels with TC levels were disclosed in our study. TBEP exposure in Tm3 Leydig cells increases oxidative stress, decreases cell viability, disrupts hormone generation (40), and induces abnormal sperm morphology and testicular histopathology in male rats (41). Moreover, TPhP and TDCPP can cause endocrine disruption, alter thyroid hormone levels (42), and decrease semen quality in men (43). DPhP downregulates the expression of genes involved in lipid/cholesterol and glucose/fatty acid metabolism (44). An animal study revealed that exposure to DPHP causes metabolic disturbance in the organism possibly because of its interfering effects on estrogen and mineralocorticoids (45). Thyroid hormone is an important regulator of serum cholesterol levels and hepatic cholesterol metabolism, including synthesis, endocytosis by the (LDL)-receptor, and peripheral uptake and hepatic excretion by reverse cholesterol transport. The disruption of cholesterol metabolism by OPFRs might further interfere with thyroid hormone synthesis.
There are several limitations about our study. First, the composition and concentration of different OPFRs might be varied in different regions, hence, the results might not be applied to other countries. Second, several kinds of chemicals such as phthalates and polybrominated diphenylethers are co-existing in the environment. These chemicals might interfere with OPRFs which lead to different impacts on human health. The interactions between different environmental toxicants and its effects on human health could not be further clarified in our study. Third, the mechanisms of lipid metabolism might vary between different persons biochemically, therefore, the disturbance from OPFRs on lipid metabolism might also be different. The concomitant medical illness and medications might also exert different degrees of influence of lipid metabolism which could not be delineated in our study.
DPhP, BDCPP, and TCEP levels were negatively related to the concentrations of HDL-C, whereas DPhP and BCPP levels were negatively associated with the levels of total cholesterol. Furthermore, the mean differences in TC levels between the upper and lower quartiles of BDCPP in all participants and the female group were 3.4 and 5.8%, respectively. Conversely, quartile increases in DPhP, BDCPP, and BCEP levels were negatively corelated with HDL-C levels, with approximately 5.9, 9.9, and 12.5% differences between the upper and lower quartiles. The findings of the current study may suggest that exposure to OPFRs could potentially interfere with lipid metabolism and have associated health effects.
The original contributions presented in the study are included in the article/supplementary material, further inquiries can be directed to the corresponding author.
The studies involving humans were approved by the 2013–2014 NHANES and by the US National Center for Health Statistics Research Ethics Review Board (Continuation of Protocol #2011-17), and informed consent was obtained from all participants. The studies were conducted in accordance with the local legislation and institutional requirements. The human samples used in this study were acquired from another research group. Written informed consent for participation was not required from the participants or the participants’ legal guardians/next of kin in accordance with the national legislation and institutional requirements.
F-JC: Writing – original draft, Writing – review & editing, Conceptualization, Methodology, Formal analysis, Validation. K-FT: Formal analysis, Resources, Software, Validation, Writing – original draft. K-CH: Data curation, Investigation, Writing – review & editing. C-TK: Project administration, Resources, Supervision, Writing – review & editing. W-TH: Resources, Writing – review & editing. H-LY: Validation, Writing – review & editing. S-HL: Software, Visualization, Writing – review & editing. C-CW: Data curation, Writing – review & editing. W-CL: Investigation, Validation, Writing – review & editing. H-YP: Conceptualization, Data curation, Investigation, Supervision, Validation, Visualization, Writing – original draft, Writing – review & editing.
The author(s) declare that no financial support was received for the research, authorship, and/or publication of this article.
The authors declare that the research was conducted in the absence of any commercial or financial relationships that could be construed as a potential conflict of interest.
All claims expressed in this article are solely those of the authors and do not necessarily represent those of their affiliated organizations, or those of the publisher, the editors and the reviewers. Any product that may be evaluated in this article, or claim that may be made by its manufacturer, is not guaranteed or endorsed by the publisher.
The Supplementary material for this article can be found online at: https://www.frontiersin.org/articles/10.3389/fpubh.2024.1340261/full#supplementary-material
1. Go, AS, Mozaffarian, D, Roger, VL, Benjamin, EJ, Berry, JD, Borden, WB, et al. Executive summary: heart disease and stroke statistics--2013 update: a report from the American Heart Association. Circulation (2013) 127:143–52. doi: 10.1161/CIR.0b013e318282ab8f
2. Jacobs, I, Nadkarni, V, Bahr, J, Berg, RA, Billi, JE, Bossaert, L, et al. Cardiac arrest and cardiopulmonary resuscitation outcome reports: update and simplification of the Utstein templates for resuscitation registries. A statement for healthcare professionals from a task force of the international liaison committee on resuscitation (American Heart Association, European Resuscitation Council, Australian Resuscitation Council, New Zealand Resuscitation Council, Heart and Stroke Foundation of Canada, InterAmerican Heart Foundation, Resuscitation Council of Southern Africa). Resuscitation (2004) 63:233–49. doi: 10.1016/j.resuscitation.2004.09.008
3. Li, Z, Zhu, G, Chen, G, Luo, M, Liu, X, Chen, Z, et al. Distribution of lipid levels and prevalence of hyperlipidemia: data from the NHANES 2007-2018. Lipids Health Dis (2022) 21:111. doi: 10.1186/s12944-022-01721-y
4. Stewart, J, McCallin, T, Martinez, J, Chacko, S, and Yusuf, S. Hyperlipidemia. Pediatr Rev (2020) 41:393–402. doi: 10.1542/pir.2019-0053
5. Yan, Z, Jin, X, Liu, D, Hong, Y, Liao, W, Feng, C, et al. The potential connections of adverse outcome pathways with the hazard identifications of typical organophosphate esters based on toxicity mechanisms. Chemosphere (2021) 266:128989. doi: 10.1016/j.chemosphere.2020.128989
6. Jokanovic, M, Kosanovic, M, Brkic, D, and Vukomanovic, P. Organophosphate induced delayed polyneuropathy in man: an overview. Clin Neurol Neurosurg (2011) 113:7–10. doi: 10.1016/j.clineuro.2010.08.015
7. Cocci, P, Mosconi, G, and Palermo, FA. Changes in expression of microRNA potentially targeting key regulators of lipid metabolism in primary gilthead sea bream hepatocytes exposed to phthalates or flame retardants. Aquat Toxicol (2019) 209:81–90. doi: 10.1016/j.aquatox.2019.02.002
8. Kojima, H, Takeuchi, S, Itoh, T, Iida, M, Kobayashi, S, and Yoshida, T. In vitro endocrine disruption potential of organophosphate flame retardants via human nuclear receptors. Toxicology (2013) 314:76–83. doi: 10.1016/j.tox.2013.09.004
9. Morris, PJ, Medina-Cleghorn, D, Heslin, A, King, SM, Orr, J, Mulvihill, MM, et al. Organophosphorus flame retardants inhibit specific liver carboxylesterases and cause serum hypertriglyceridemia. ACS Chem Biol (2014) 9:1097–103. doi: 10.1021/cb500014r
10. Du, Z, Zhang, Y, Wang, G, Peng, J, Wang, Z, and Gao, S. TPhP exposure disturbs carbohydrate metabolism, lipid metabolism, and the DNA damage repair system in zebrafish liver. Sci Rep (2016) 6:21827. doi: 10.1038/srep21827
11. Le, Y, Shen, H, Yang, Z, Lu, D, and Wang, C. Comprehensive analysis of organophosphorus flame retardant-induced mitochondrial abnormalities: potential role in lipid accumulation. Environ Pollut (2021) 274:116541. doi: 10.1016/j.envpol.2021.116541
12. Xu, F, Giovanoulis, G, van Waes, S, Padilla-Sanchez, JA, Papadopoulou, E, Magnér, J, et al. Comprehensive study of human external exposure to organophosphate flame retardants via air, dust, and hand wipes: the importance of sampling and assessment strategy. Environ Sci Technol (2016) 50:7752–60. doi: 10.1021/acs.est.6b00246
13. US National Center for Health Statistics. Available at: https://www.cdc.gov/nchs/nhanes/index.htm (Accessed May 20).
14. Jayatilaka, NK, Restrepo, P, Williams, L, Ospina, M, Valentin-Blasini, L, and Calafat, AM. Quantification of three chlorinated dialkyl phosphates, diphenyl phosphate, 2,3,4,5-tetrabromobenzoic acid, and four other organophosphates in human urine by solid phase extraction-high performance liquid chromatography-tandem mass spectrometry. Anal Bioanal Chem (2017) 409:1323–32. doi: 10.1007/s00216-016-0061-4
15. Ospina, M, Jayatilaka, NK, Wong, LY, Restrepo, P, and Calafat, AM. Exposure to organophosphate flame retardant chemicals in the U.S. general population: data from the 2013-2014 National Health and nutrition examination survey. Environ Int (2018) 110:32–41. doi: 10.1016/j.envint.2017.10.001
16. Casula, M, Colpani, O, Xie, S, Catapano, AL, and Baragetti, A. HDL in atherosclerotic cardiovascular disease: in search of a role. Cells (2021) 10:1869. doi: 10.3390/cells10081869
17. Li, ZH, Lv, YB, Zhong, WF, Gao, X, Byers Kraus, V, Zou, MC, et al. High-density lipoprotein cholesterol and all-cause and cause-specific mortality among the elderly. J Clin Endocrinol Metab (2019) 104:3370–8. doi: 10.1210/jc.2018-02511
18. Gordon, DJ, Probstfield, JL, Garrison, RJ, Neaton, JD, Castelli, WP, Knoke, JD, et al. High-density lipoprotein cholesterol and cardiovascular disease. Four prospective American studies. Circulation (1989) 79:8–15. doi: 10.1161/01.cir.79.1.8
19. Hu, W, Kang, Q, Zhang, C, Ma, H, Xu, C, Wan, Y, et al. Triphenyl phosphate modulated saturation of phospholipids: induction of endoplasmic reticulum stress and inflammation. Environ Pollut (2020) 263:114474. doi: 10.1016/j.envpol.2020.114474
20. Hosokawa, M. Structure and catalytic properties of carboxylesterase isozymes involved in metabolic activation of prodrugs. Molecules (2008) 13:412–31. doi: 10.3390/molecules13020412
21. Lian, J, Nelson, R, and Lehner, R. Carboxylesterases in lipid metabolism: from mouse to human. Protein Cell (2018) 9:178–95. doi: 10.1007/s13238-017-0437-z
22. Aluru, N, Hallanger, IG, McMonagle, H, and Harju, M. Hepatic gene expression profiling of Atlantic cod (Gadus morhua) liver after exposure to organophosphate flame retardants revealed altered cholesterol biosynthesis and lipid metabolism. Environ Toxicol Chem (2021) 40:1639–48. doi: 10.1002/etc.5014
23. Bollmann, UE, Moller, A, Xie, Z, Ebinghaus, R, and Einax, JW. Occurrence and fate of organophosphorus flame retardants and plasticizers in coastal and marine surface waters. Water Res (2012) 46:531–8. doi: 10.1016/j.watres.2011.11.028
24. Fromme, H, Lahrz, T, Kraft, M, Fembacher, L, Mach, C, Dietrich, S, et al. Organophosphate flame retardants and plasticizers in the air and dust in German daycare centers and human biomonitoring in visiting children (LUPE 3). Environ Int (2014) 71:158–63. doi: 10.1016/j.envint.2014.06.016
25. Wong, F, de Wit, CA, and Newton, SR. Concentrations and variability of organophosphate esters, halogenated flame retardants, and polybrominated diphenyl ethers in indoor and outdoor air in Stockholm, Sweden. Environ Pollut (2018) 240:514–22. doi: 10.1016/j.envpol.2018.04.086
26. Wang, Y, Sun, H, Zhu, H, Yao, Y, Chen, H, Ren, C, et al. Occurrence and distribution of organophosphate flame retardants (OPFRs) in soil and outdoor settled dust from a multi-waste recycling area in China. Sci Total Environ (2018) 625:1056–64. doi: 10.1016/j.scitotenv.2018.01.013
27. Hao, C, Helm, PA, Morse, D, and Reiner, EJ. Liquid chromatography-tandem mass spectrometry direct injection analysis of organophosphorus flame retardants in Ontario surface water and wastewater effluent. Chemosphere (2018) 191:288–95. doi: 10.1016/j.chemosphere.2017.10.060
28. Choo, G, Cho, HS, Park, K, Lee, JW, Kim, P, and Oh, JE. Tissue-specific distribution and bioaccumulation potential of organophosphate flame retardants in crucian carp. Environ Pollut (2018) 239:161–8. doi: 10.1016/j.envpol.2018.03.104
29. Sundkvist, AM, Olofsson, U, and Haglund, P. Organophosphorus flame retardants and plasticizers in marine and fresh water biota and in human milk. J Environ Monit (2010) 12:943–51. doi: 10.1039/b921910b
30. He, C, English, K, Baduel, C, Thai, P, Jagals, P, Ware, RS, et al. Concentrations of organophosphate flame retardants and plasticizers in urine from young children in Queensland, Australia and associations with environmental and behavioural factors. Environ Res (2018) 164:262–70. doi: 10.1016/j.envres.2018.02.040
31. Hoang, MTT, Le, GT, Kiwao, K, Duong, HT, Nguyen, TQ, Phan, TQ, et al. Occurrence and risk of human exposure to organophosphate flame retardants in indoor air and dust in Hanoi, Vietnam. Chemosphere (2023) 328:138597. doi: 10.1016/j.chemosphere.2023.138597
32. Hoffman, K, Garantziotis, S, Birnbaum, LS, and Stapleton, HM. Monitoring indoor exposure to organophosphate flame retardants: hand wipes and house dust. Environ Health Perspect (2015) 123:160–5. doi: 10.1289/ehp.1408669
33. Lu, SY, Li, YX, Zhang, T, Cai, D, Ruan, JJ, Huang, MZ, et al. Effect of E-waste recycling on urinary metabolites of organophosphate flame retardants and plasticizers and their association with oxidative stress. Environ Sci Technol (2017) 51:2427–37. doi: 10.1021/acs.est.6b05462
34. Tsai, KF, Cheng, FJ, Huang, WT, Kung, CT, Lee, CT, Cheng, BC, et al. The associations between renal disease severity and exposure to organophosphate flame retardants in patients with chronic kidney disease. Environ Int (2022) 170:107573. doi: 10.1016/j.envint.2022.107573
35. Rye, KA, Bursill, CA, Lambert, G, Tabet, F, and Barter, PJ. The metabolism and anti-atherogenic properties of HDL. J Lipid Res (2009) 50:S195–200. doi: 10.1194/jlr.R800034-JLR200
36. Cuchel, M, and Rader, DJ. Macrophage reverse cholesterol transport: key to the regression of atherosclerosis? Circulation (2006) 113:2548–55. doi: 10.1161/CIRCULATIONAHA.104.475715
37. Kim, J, Kim, MK, Baek, KH, Song, KH, Han, K, and Kwon, HS. Repeated low high-density lipoprotein cholesterol and the risk of thyroid cancer: a Nationwide population-based study in Korea. Endocrinol Metab (Seoul) (2022) 37:303–11. doi: 10.3803/EnM.2021.1332
38. Loosen, SH, Kostev, K, Luedde, M, Luedde, T, and Roderburg, C. Low blood levels of high-density lipoprotein (HDL) cholesterol are positively associated with cancer. J Cancer Res Clin Oncol (2022) 148:3039–46. doi: 10.1007/s00432-021-03867-1
39. Luo, J, Yang, H, and Song, BL. Mechanisms and regulation of cholesterol homeostasis. Nat Rev Mol Cell Biol (2020) 21:225–45. doi: 10.1038/s41580-019-0190-7
40. Jin, Y, Chen, G, and Fu, Z. Effects of TBEP on the induction of oxidative stress and endocrine disruption in Tm3 Leydig cells. Environ Toxicol (2016) 31:1276–86. doi: 10.1002/tox.22137
41. Pan, HY, Cheng, FJ, Huang, KC, Kung, CT, Huang, WT, You, HL, et al. Exposure to tris(2-butoxyethyl) phosphate induces abnormal sperm morphology and testicular histopathology in male rats. Ecotoxicol Environ Saf (2022) 241:113718. doi: 10.1016/j.ecoenv.2022.113718
42. Wei, GL, Li, DQ, Zhuo, MN, Liao, YS, Xie, ZY, Guo, TL, et al. Organophosphorus flame retardants and plasticizers: sources, occurrence, toxicity and human exposure. Environ Pollut (2015) 196:29–46. doi: 10.1016/j.envpol.2014.09.012
43. Hoffman, K, Lorenzo, A, Butt, CM, Adair, L, Herring, AH, Stapleton, HM, et al. Predictors of urinary flame retardant concentration among pregnant women. Environ Int (2017) 98:96–101. doi: 10.1016/j.envint.2016.10.007
44. Su, G, Crump, D, Letcher, RJ, and Kennedy, SW. Rapid in vitro metabolism of the flame retardant triphenyl phosphate and effects on cytotoxicity and mRNA expression in chicken embryonic hepatocytes. Environ Sci Technol (2014) 48:13511–9. doi: 10.1021/es5039547
45. Zhang, Q, Yu, C, Fu, L, Gu, S, and Wang, C. New insights in the endocrine disrupting effects of three primary metabolites of organophosphate flame retardants. Environ Sci Technol (2020) 54:4465–74. doi: 10.1021/acs.est.9b07874
Keywords: organophosphate flame retardants, lipid metabolism, triglycerides, cholesterol, HDL
Citation: Cheng F-J, Tsai K-F, Huang K-C, Kung C-T, Huang W-T, You H-L, Li S-H, Wang C-C, Lee W-C and Pan H-Y (2024) Association between organophosphate flame retardant exposure and lipid metabolism: data from the 2013–2014 National Health and Nutrition Examination Survey. Front. Public Health. 12:1340261. doi: 10.3389/fpubh.2024.1340261
Received: 17 November 2023; Accepted: 06 February 2024;
Published: 08 March 2024.
Edited by:
Ella Atlas, Health Canada, CanadaReviewed by:
Sandeep Kondakala, National Center for Toxicological Research (FDA), United StatesCopyright © 2024 Cheng, Tsai, Huang, Kung, Huang, You, Li, Wang, Lee and Pan. This is an open-access article distributed under the terms of the Creative Commons Attribution License (CC BY). The use, distribution or reproduction in other forums is permitted, provided the original author(s) and the copyright owner(s) are credited and that the original publication in this journal is cited, in accordance with accepted academic practice. No use, distribution or reproduction is permitted which does not comply with these terms.
*Correspondence: Hsiu-Yung Pan, Z2V0dGluZ2ZhdDcyMEBnbWFpbC5jb20=
Disclaimer: All claims expressed in this article are solely those of the authors and do not necessarily represent those of their affiliated organizations, or those of the publisher, the editors and the reviewers. Any product that may be evaluated in this article or claim that may be made by its manufacturer is not guaranteed or endorsed by the publisher.
Research integrity at Frontiers
Learn more about the work of our research integrity team to safeguard the quality of each article we publish.