- Department of Biology, Clarkson University, Potsdam, NY, United States
Degeneration of the intervertebral disc (IVD) is a normal part of aging. Due to the spine's declining function and the development of pain, it may affect one's physical health, mental health, and socioeconomic status. Most of the intervertebral disc degeneration (IVDD) therapies today focus on the symptoms of low back pain rather than the underlying etiology or mechanical function of the disc. The deteriorated disc is typically not restored by conservative or surgical therapies that largely focus on correcting symptoms and structural abnormalities. To enhance the clinical outcome and the quality of life of a patient, several therapeutic modalities have been created. In this review, we discuss genetic and environmental causes of IVDD and describe promising modern endogenous and exogenous therapeutic approaches including their applicability and relevance to the degeneration process.
Introduction
Low back pain (LBP) is one of the most common health concerns in the world. It affects a significant part of the population and, in the United States, has the highest health-related economic cost of up to 560–630 billion dollars per year (1–3). It is estimated that between 70 and 85% of the population will experience LBP at some point in their lives and that it can already limit activities in those under the age of 45, posing a significant socioeconomic impact by accounting for over 100 million lost workdays annually in the USA alone (4, 5). LBP presents as one of the most frequent causes of disability among young adults (6, 7). Although the reasons for most cases of LBP are unknown, intervertebral disc degeneration (IVDD) is regarded as the most common factor (1, 8). IVDD is not limited to humans (9). The organ affected in IVDD is the intervertebral disc (IVD). The IVD is a semi-movable joint and a cushion of fibrocartilage between the vertebrae. It is comprised of a central nucleus pulposus (NP) surrounded by an inner and outer annulus fibrosus (AF) and is sandwiched between the cartilaginous endplates (CEP) as seen in Figure 1A (10–15). The AF is made up of concentric lamellae, which are densely interwoven collagen bundles that run obliquely between adjacent vertebral bodies (14, 16). The NP, on the other hand, has a loose collagen network and is highly hydrated (11, 14, 16). Compared to other tissue types, NP cells are present at a low cell-density (3,000 cells/mm3) sequestered in an abundance of extracellular matrix (ECM) while the cell density in the AF is ~3 × higher (17–19). The strong, fibrous collagen framework of the disc holds cells and proteoglycans (PG) in the matrix in place while securing the disc to the vertebral bodies (20, 21). Collagen II represents ~20% of the NP dry weight, while PG, especially the big aggregating PG aggrecan (ACAN), make up ~50% of the NP dry weight (22), the latter providing the osmotic swelling pressure that maintains disc height and turgor amidst heavy compressive loads or impacts.
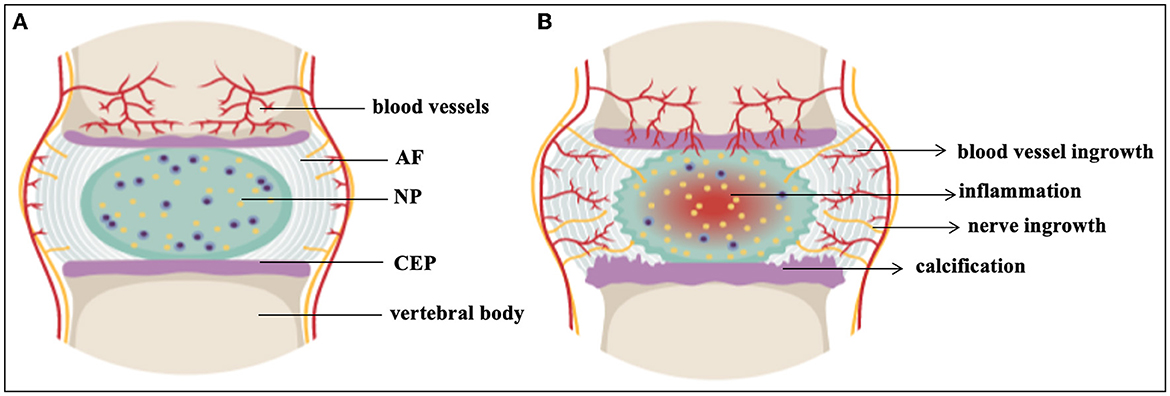
Figure 1. The intervertebral disc. (A) Healthy IVD (B) Degenerated IVD. AF, annulus fibrosus; CEP, cartilaginous end plates; NP, nucleus pulposus. This figure created in the Mind the Graph platform (www.mindthegraph.com).
During embryogenesis carefully orchestrated events give rise to the notochord (NC) a crucial structure during early chordate development that is filled with relatively large, vacuolized NC cells. NC cells take on an important function in maintaining hydro-pressure against external forces through the production of polar macromolecules (23, 24). While largely considered conserved, precise events of early notochord generation might vary between different chordate species (25). Genetic engineering in mouse demonstrated not only that the NP is of NC origin but also identified the expression of many important transcription and signaling factors that are involved in these early patterning events such as Shh, Sox5, Sox6 and Sox9; the Paired box genes Pax1 and Pax9 alongside Nkx3.2 (Bapx1), Noto and Brachyury (Tbx1) to name a few (26–37). NC cells make up the NP of early vertebrate IVDs (38, 39) but depending on the species, these cells might be reduced to a minimum population with different ratios compared to other IVD cells, rendering the NP a heterogeneous cell population (40–44). This loss of NC cells is often correlated with the onset of disc degeneration (45). Morphology and cell composition of the adult NP can differ between mammalian species (46, 47). In some rodents popular in research NC-like cells are retained into adulthood, whereas in other animals, chondrocyte-like (CL) cells become more prominent over time. The origin of these CL cells is of debate. Transdifferentiation of NC cells into CL cells, or progenitor cell recruitment into the NP by NC cells prior to undergoing regulated cell death are considered. Previously receptor tyrosine kinase (Tek or Tie2) and disialoganglioside 2 (Gd2) expression was used to identify multipotent stem cell populations in the IVD, while the siaologlycoprotein encoding gene CD24 plays a role in the differentiation of different cell types. Tie2+/Gd2–/CD24– progenitor cells were identified in the mouse, human and bovine NP and described as dormant stem cells (47–49). A gradual change from a Tie2+/Gd2+/CD24– phenotype with self-renewal potential and stem cell properties to a Tie2–/Gd2+/CD24– phenotype of potential and a Tie2–/Gd2+/CD24+ phenotype of committed NP progenitors before committing to a mature NP phenotype (Tie2–/Gd2–/CD24+) was suggested (47, 48). Recent single cell RNA sequencing (scRNASeq) analysis of sorted murine NP cells from 1 month old C57BL/6 mice identified four subpopulations. One with an enrichment of stemness genes was considered as NP progenitor cells involved in the regulation of cell growth and differentiation based on their transcription factor profile (50). A mouse NP progenitor cell population expressing the G-protein-coupled receptor Uts2R was located in the peripheral NP, with the majority of these cells expressing Tie2 and ~1/3 Tie2 and Gd2. The authors demonstrated that this progenitor population declines in IVDD (50) supporting work pioneered by Sakai et al. (47). It was further suggested through Krt19 fate mapping that CL cells in the lumbar NP of mice older than 18 months represent a NP cell derived terminal differentiation stage and that < 15% thereof remain Shh positive, suggesting age-related transdifferentiation over cell invasion (51). In the human NP, NC cells disappear in early childhood (4–10 years of age) (46, 52, 53). ScRNASeq recently deciphered several cell clusters in the healthy human IVD, amongst them three chondrocyte subclusters with many cells expressing Noggin (NOG), a small group of cells expressing NC markers TBXT and KRT8 and a group of multipotent NP progenitor cells expressing PROCR, a gene associated with signaling receptor activity and stemness, and PDGFRA associated with mesenchymal stem cells (MSC), molecular evidence suggesting that different cell morphologies in the NP reflect phases of NC lineage cells during aging and degeneration (54). Based on these findings a correlation between declining numbers of UTS2R+ or TIE2+/GD2+ stem-like cells and the onset of IVDD would also be expected, yet was not specifically described for human NP CL cell populations of various IVDD degrees (55, 56), however the relevant stages might have been missed.
One of the earliest changes in IVDD is a loss of PG content and composition, resulting in reduced hydration, height and flexibility of the disc (11) as seen in Figure 1B. IVDD is a chronic disorder characterized by a progressive loss of mechanical stability and shock absorber function, which can lead to the formation of osteophytes and restricted motion in spinal segments (57). IVDD is frequently associated with spondylolisthesis, disc herniation, sciatica, spinal canal stenosis, and degenerative scoliosis (58). About 20% of teenagers show signs of beginning IVDD (5, 59) including athletes, especially those involved in high impact sports such as football, gymnastics and diving. While frequencies of cervical spine injuries were studied amongst school-age and college athletes (60, 61), IVD damage amongst this cohort is less well documented (62). IVDD also affects the ability of the spine to resist physiologically acceptable loads during daily activities, and impacts on the function of adjacent tissues, such as the muscles and ligaments (63). Chronic LBP continues to limit abilities and the quality of life for a large percentage of the population, despite access to invasive and expensive surgical interventions for discogenic pain such as arthroplasty and arthrodesis (5, 64). Restoring the ECM components of the IVD to their initial state would be preferable (65). Therefore, initiatives have been undertaken to create non-operative therapy modalities that are both efficient and secure. A major area of study is the direct injection of active compounds to prevent, slow down, or even reverse IVDD (66, 67). In the past 30 years, numerous clinical trials investigating biologic, cell- and scaffold-based injectable therapies for symptomatic IVDD have been undertaken (6, 17, 68). Several preclinical animal studies and fundamental scientific investigations support each of the clinical trials (69) (Table 1). However, scientifically established methods to prevent or reverse IVDD and the accompanying discogenic pain are not yet available. The current lack of success in treatments demonstrates the complexity of this illness (137). In this review, we present the status of IVDD causes alongside the challenges of available therapies.
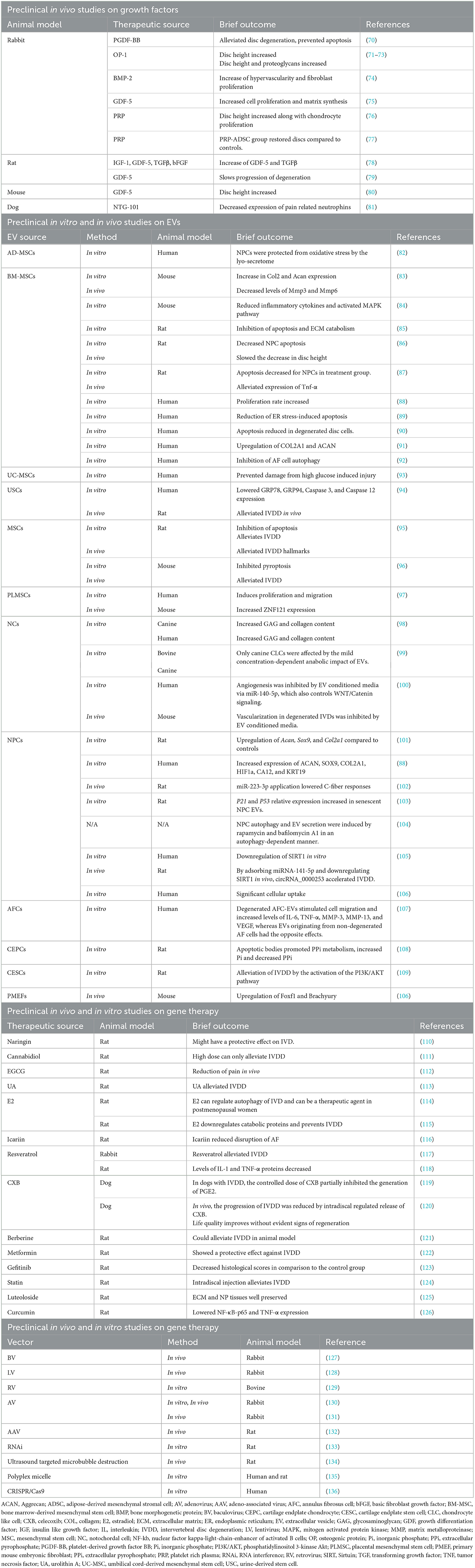
Table 1. Preclinical studies for different interventions using various model systems to assess therapeutic potentials in IVDD.
Causes of IVDD
IVDD is usually caused by a conflux of genetic, environmental and lifestyle factors as well as trauma as seen in Figure 2. Therefore, the genetic and environmental risk factors outlined here might each or in combination contribute to and aggravate IVDD as seen for comorbidities in other chronic illnesses (138). Magnetic resonance imaging (MRI) has improved classifications of disc degeneration (139–141). IVDD frequently occurs when ECM catabolism outweighs its anabolism (142). The pathophysiology of IVDD is influenced by many other factors such as genetics and the environment including an unhealthy lifestyle, inactivity, smoking, occupational exposure to vibration, mechanical loading, severe trauma, psychosocial problems, benefit payments and more (143, 144).
Genetic factors
IVD cells are impacted by changes in ECM composition, structure and function resulting from genetic polymorphisms and DNA mutations (Table 2) (162, 163). Recent research on heredity and linkage has undoubtedly increased awareness of genetic predisposition to IVDD. However, the degree and kind of genetic influences are still not fully understood. The association between disc degeneration and genetic polymorphisms such as variable number of tandem repeats (VNTR) or single nucleotide polymorphisms (SNP) of certain ECM macromolecules is considered a main genetic factor (164, 165). For example, polymorphisms in PG encoding genes such as ACAN were associated with IVDD. Polymorphisms affecting fibrillar collagen ECM constituents like COL11A1 were reported in the context of disc herniation and IVDD (166–169). Transgenic mice with a mutation in Col9a1 demonstrated progressive IVDD, likely affecting synthesis or assembly of non-fibrillar Col9a1 chains (170); while COL9A2 and COL9A3 variants were significantly correlated with sciatica and lumbar disc degeneration in a Finnish population (171, 172). Other genetic associations involve ECM remodeling enzymes such as matrix metalloproteinases (MMP), more specifically MMP2 and MMP9, both a gelatinase and type IV collagenase and MMP3, a PG degrading enzyme (173–178). Other disintegrin, and metalloproteinases (ADAM), and those with thrombospondin motifs (ADAMTS) show changes in expression patterns during IVDD (173, 179–182). In this context, polymorphisms in the vitamin D receptor (VDR) across diverse ethnic backgrounds were linked to IVDD (168, 183–188). While the foundation for some genetic studies was based on a limited cohort size (189) the rapid development of next and third generation sequencing technologies allowed for genome wide association studies (GWAS) as seen beneficial in other fields, alongside more targeted, specific studies of susceptibility regions in large patient and control cohorts of different ethnic backgrounds. This genetic association suggests a pleiotropic nature of IVDD (190). For instance, transcriptional regulators NFAT1 and SOX9 control the expression of many genes that are both anabolic and catabolic and mediate ECM production (191, 192). CHST3, encodes for an enzyme that catalyzes the sulfation of chondroitin, an ECM PG (148). Amongst other susceptibility loci identified through GWAS are known players in the context or ECM production, chondrogenesis and cell survival like BARX1, COL11A1, COLGALT2, TGFA, FGFR3, FOXA3, GDF5, SMAD3, and TGFA (190). While most studies so far focused on caucasian populations, a recent GWAS focusing on a Chinese cohort identified polymorphisms near Gasdermin-C (GSDMC). Interestingly, gasdermins are involved in mediating pyroptosis as a form of regulated cell death (193), however the studies phenotype/genetic variant association differed from previous findings (150), indicating the importance of such studies across different ethnic backgrounds as well as the need for a precise definition of IVDD phenotypes in such studies. Also, non-lethal polymorphisms in early IVD patterning genes will likely surface over time as underlying cause. Furthermore, both single cell and bulk transcript analysis of IVD derived cells through RNASeq and other methods will likely point to biomarkers for NP and AF cells of healthy or degenerated discs worth investigating in IVDD linkage analysis (41, 42, 194–198). Going forward it will be crucial to investigate not only polymorphisms in coding and regulatory regions such as promoters or enhancer/silencer binding sites of genes but also epigenetic modifications from methylation, acetylation and lactylation involved in metabolic reprogramming among other effects (197, 199). This could facilitate the identification of environmental variables as a factor in disc degeneration as IVDD is a multifactorial disease (162, 200, 201).
Environmental factors
Metabolic stress factors
The disc's microenvironment is complex. The healthy adult NP is the largest avascular, organ in the vertebrate body and the distance to the closest blood vessel can be up to 8 mm (202). Residing cells rely on diffusion from capillaries penetrating the outer AF and adjacent CEPs to transport nutrients or oxygen and to remove metabolic waste products. This generates challenging circumstances for NP cell survival in this unique in vivo niche (203–205). In a healthy NP, the oxygen tension is 2%; in a degenerated NP, it is 1% (206). These anaerobic conditions result in lactic acid fermentation for energy production (11, 203, 207, 208), which alongside proton retention via ECM-PGs renders even the healthy NP slightly acidic (~pH 7.1). The acidity increases further in the degenerate stage with pH readings of 6.5–5.7 (209–212). It was reported that the activity of disc cells is extremely sensitive to extracellular oxygen and pH in vitro, that ECM production rates fall sharply at acidic pH and at low oxygen concentrations, and that cells are not able to withstand extended exposure to low pH (16, 213). Decreased nutrition supply was further considered a cause of progressive IVDD with aging potentially as an implication of increased calcification and erosion of the CEP (22, 205, 214–217). Experimentally and in human patients, it has been demonstrated that disruptions in nutrition delivery have an impact on how oxygen and lactic acid are transported in and out of the disc (206). However, it was also shown that lactate can serve as carbon source for various cell types (218, 219) and NP cells in their unique niche likely developed metabolic adaptions catering to the use of lactic acid, given that primary cells isolated from a healthy coccygeal bovine IVD preferred the absence of glucose in serum containing monolayer culture (197). Disc degeneration and back discomfort are linked to conditions that influence the blood flow to the vertebral body, such as abdominal aortic atherosclerosis, increased CEP erosion and calcification (216, 217, 220). Impairment of the CEPs also alters the NP's mechanical loading, which causes alterations in the disc's metabolism (221, 222). Endplate calcification as seen in scoliotic discs can affect nutrients and metabolite transport through the endplate and further aggravate hypoxia and an acidity (223, 224). Calcified CEP with 50% reduction in permeability resulted in disc deformity and a drop in IVD glucose levels to half of levels in the healthy NP (225, 226). This could prevent IVD cells from sustaining the ECM (222, 227). Additionally, it has been shown that deteriorated IVDs exhibit chronic inflammation (228), with increased expression of a number of pro-inflammatory cytokines (207). This includes interleukin (IL) 1, MMP10 (229), MMP12 (230), cyclooxygenase 2 (COX2) (231), IL8, tumor necrosis factor- (TNF) 22 (232), IL10 (233), IL2, IL4, and IL17 (234), among others, which may be strongly associated with discogenic pain (235).
Mechanical stress factors
The IVD is an important part of the vertebral column facilitating protection of the vertebrae and spinal cord during regular daily activities, exercise, and accidental trauma. Abnormal mechanical load and stress can lead to disc injury and degeneration. For many years, it was believed that injuries, which result in structural damage, are a major contributor to spinal disorders (236). These injuries eventually result in IVDD and associated symptoms like back pain. This finding has been supported by animal models (237). While exercise is generally considered a healthy activity, some forms including impact or strenuous loading (diving, gymnastics, weight lifting, and high impact contact sports) can trigger IVDD, while other forms are beneficial resulting in increased anabolic responses with increased glycosaminoglycan and hydration levels in the IVD (62). For instance, more lumbar IVD degeneration was seen in gymnasts compared to controls who weren't athletes (238), as well as in soccer players and weightlifters in comparison to shooters (186). Evidence supporting the positive effects of exercise on the IVD in humans, however, is less clear (239). Basketball, baseball, swimming, and soccer were linked to better IVD parameters over controls, while poorer NP hydration was marginally linked with a longer career and heavier training load (62). The transport of nutrients into the disc and, consequently, their concentration in the tissue, appears to be influenced by exercise (223, 224). Although the exact mechanism is unknown, it has been proposed that exercise alters the capillary bed's morphology at the disc-bone interface (223).
Other environmental factors
Risk factors such as age, low income, prior cervical spine surgery, type of health insurance, and medical comorbidities like cancer, diabetes, hypertension, depression, hypothyroidism, peripheral vascular disease chronic obstructive pulmonary disease (COPD), and lifestyle choices such as smoking were linked to IVDD (240–244). Numerous studies have linked tobacco use to lower back pain. Smoking is known to prevent the fusion and healing of bones and initially reduces the proliferation and activity of fibroblasts and osteoblasts and the usual inflammatory response (245–248). It subsequently interferes with neovascularization and the normal vascular supply, encouraging net bone resorption rather than net bone growth (245, 247). Notably, after lumbar or cervical fusion surgery, pseudarthrosis occurs at a rate that is two times higher among smokers (245, 249–252). Tobacco inhalation and nicotine caused vasoconstriction and decreased the exchange of nutrients and anabolic substances, resulting in inadequate IVD nourishment, ECM and NP cell development all contributing to the IVD's instability and degeneration (253–258). Toxins from cigarette smoke impaired spinal blood flow and nutrition supply, accelerated spondylosis or resulted in rapid infection, and other surgical problems (259–261). While the pathophysiological mechanism and pathological characteristics of IVDD brought on by cigarette smoke remain unknown and a clear link between smoking and IVDD remains speculative, smoking appeared to increase and accelerate the chance of disc herniation through capillary constriction as an independent risk factor in patients with lumbar disc herniation (249, 257, 262–265).
Degeneration is quickened by the interaction of hereditary and environmental factors. There is currently no study that acknowledges the independent influence of environmental factors without genetic predisposition (144). However, subtypes of herniation may develop as a result of sedentary lifestyle (266, 267). The composition of the disc retains water to keep the hydrostatic pressure constant, keeping the NP elastic, flexible and able to withstand compression (268). There is convincing evidence that as people age, the likelihood of disc degeneration increases, partially as a result of the accumulation of senescent cells (269, 270). Although in mitotic arrest, these cells remain metabolically active and anaerobic metabolism contributes to increased acidity (271) and their senescence associated secretory phenotype (SASP) is likely luring more neighboring cells into the same fate (272). Lastly, obesity is linked to biomechanical alterations that lead to a variety of spinal disorders like IVDD, osteoarthritis, disc herniation, and spinal stenosis (273, 274).
Current and future therapy options for IVDD
IVDD is closely tied to the loss of ECM producing cells in the maturing NP. Cell survival especially in the degenerating NP environment is challenging and cell death can have complex consequences on tissue homeostasis and immunity, triggering amongst many outcomes the release of proinflammatory cytokines (275, 276). Therapeutic interventions for IVDD (Figure 3) changed substantially over the years, however no treatment leading to a cure has been established so far. Owing to the nature of the IVD, many strategies are based on endogenous approaches that aim to stimulate resident progenitor cell populations, whereas exogenous approaches try to replenish the IVD with new cells. Efforts are made to minimize cell death and SASP associated signaling cascades.
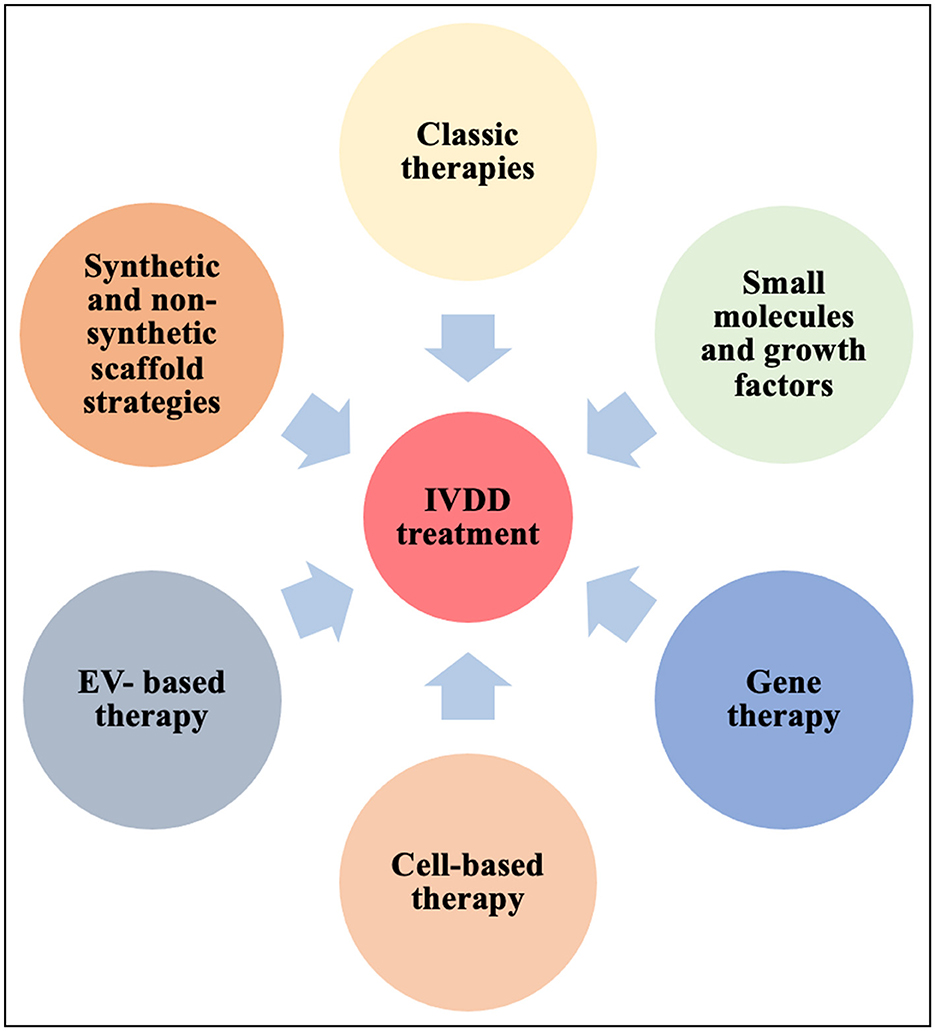
Figure 3. Current strategies for IVDD therapies. EV, extracellular vesicles; IVDD, intervertebral disc degeneration; MSC, mesenchymal stem cells.
Classic therapies
Surgery, steroids and non-steroidal anti-inflammatory drugs (NSAIDs), analgesics, opioids, muscle relaxants, and physical therapy are some of the classic therapies to alleviate IVDD symptoms like pain (277, 278) enabling short term relief, but not eradicating the problem. Radiographic imaging techniques like MRI can often demonstrate that the ongoing discomfort is caused by nerve compression (279). In recent years, regenerative medicine-based approaches along with other therapeutic interventions are gaining increased attention for advanced IVDD therapies.
Cell therapies
Cell therapy to refurbish the IVD is an important area of research (280). As the number of healthy resident cells gradually declines during IVDD, catabolic activities take place over tissue anabolism (281). To encourage endogenous repair of the degenerated IVD, stem cells can be extracted from various sources and transplanted into ailing host tissues. Harnessing “stemness” is intriguing and includes the transplantation of transdifferentiated somatic cells, induced pluripotent stem cells and embryonic stem cells. Undifferentiated stem cells have the capacity to self-renew and multiply, giving rise to committed, differentiated cells that replenish the cell pool in a particular tissue (282). There, they may secrete growth factors and cytokines to support resident cell activity, and attract or stimulate local progenitor cells (281–283). Stem cells have been isolated from a number of tissues, including the IVD (46, 284–290). Pluripotent cells however pose a risk of tumorigenesis. Additionally, some cell types are deemed uneconomical on an individualized basis, are not fully understood in their differentiation potential, or their generation and use is of ethical concern (291–294). Among candidates that have emerged for cell-based therapies for IVDD are NC cells, chondrocytes, MSC and NP cells, some have undergone preclinical and/or clinical examinations (197). Selecting a cell type requires understanding of disc development as well as knowledge of the cellular changes induced by maturation and degeneration (6, 44, 295). Some IVD cell populations exhibit progenitor cell potential as discussed above (46, 47, 49, 50, 197, 296–299), yet would require surgery for harvesting. Autologous or allogeneic MSC gained popularity as their less-tumorigenic multipotent phenotype might be directed into the appropriate cell type via endogenous cues from the recipient tissue or ECM. MSCs, especially subcutaneous adipose MSCs, offer a promising option owing to their ease of harvest, capacity for self-renewal, multilineage potential, and immunosuppressive properties (197, 300–302). However, transplanted stem cells face delivery and survival challenges in the harsh environment of the IVD which are exacerbated in the degenerated disc (6, 205, 290, 303, 304). MPC-06-ID, a Phase 3 product candidate was developed to address IVDD related chronic pain with 6 million mesenchymal precursor cells per dose for patients who have exhausted other therapy options (www.mesoblast.com). A recent subjective review indicated that result considering impairment, pain, and quality of life were influenced by the placebo effect. Therefore, more quantifiable and objective measures such as MRI and other radiographic exams are needed (305). A study to examine the clinical applicability, safety, and efficacy of NOVOCART® Disc in the repair of herniated discs requiring an elective sequestrectomy employs an autologous cell compound (306). The Sponsor has permanently halted the NOVOCART® Disc development program since there was no evident advantage of the investigational intervention above standard therapies (https://www.tetec-ag.de/en.html). An updated list of clinical trials for MSC in IVDD can be seen in Table 3 (clinicaltrials.gov). Further large-scale, randomized (placebo), controlled studies for cell based IVDD therapeutics are needed.
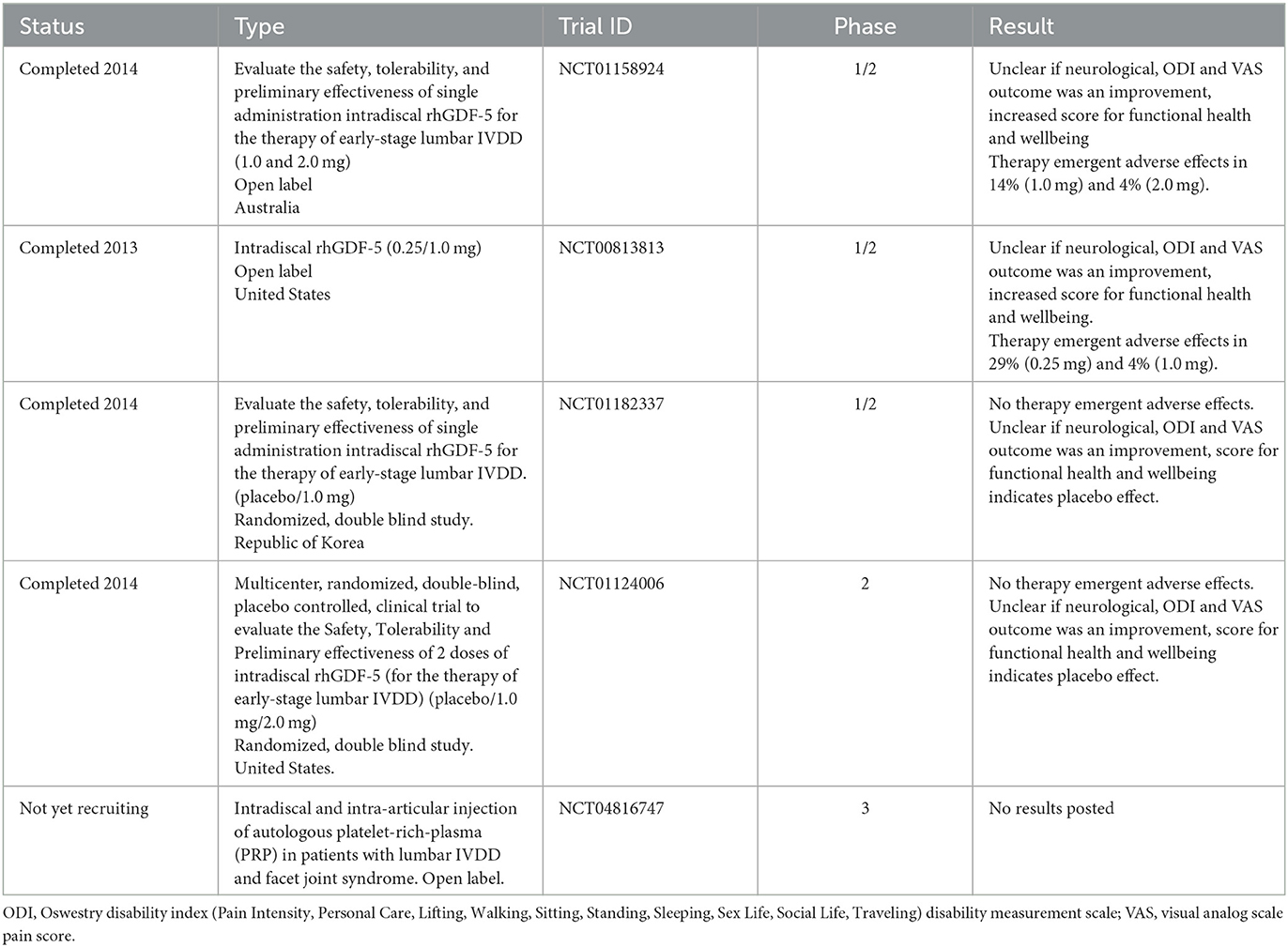
Table 3. Clinical trials reported with growth factors in the context of IVDD based on data from May 2023 (www.clinicaltrials.com).
Extracellular vesicle therapies
Cell-to-cell communication is fundamental for the maintenance of microenvironment homeostasis (307). Our knowledge of cell-cell communication has improved with the development of large-scale “-omics” technologies for analyzing the secretome of cells. These technologies have also allowed us to investigate extracellular vesicles (EV) with cell-type specific cargos of proteins and nucleic acids (285, 307). Although the classification of EVs is constantly changing, they usually fall into one of three categories: Exosomes (50–150 nm) are created by the endosomal formation of multivesicular bodies (MVB). Apoptotic bodies (up to 5,000 nm) and ectosomes (up to 1,000 nm) are generated by outward budding of the plasma membrane (308, 309) as seen in Figure 4. Most cell types produce exosomes, and their release into body fluids and culture media has sparked interest in finding cancer biomarkers (310). In fact, researchers from a variety of sectors are increasingly interested in analyzing EVs produced by resident cells in the hopes of identifying specific cell or disease-related biomarkers (311). Exosomes with cell-specific proteins, lipids, and nucleic acids are now recognized as a form of intercellular communication method (312). This theory is supported by the observation that exosomes generated by parental cells may interact with target cells, causing target cell behavior and phenotypic traits to be influenced (313). Limited research has been done on IVD-derived EVs (105, 107, 311, 314). In the area of biomarkers of LBP and disc disorders, there have been a lot of encouraging research findings such as the ongoing study and validation of pertinent, correct, and sensitive biomarkers of disc disorders (315). NC-derived EVs enhanced DNA and glycosaminoglycan content in human NP cell micro-aggregates compared to untreated control conditions although the underlying mechanism and associated EV content were not examined (316). EVs derived from human NP cells of patients with lumbar degenerative disease were found to promote MSC migration and differentiation into an NP-like phenotype via the Notch1 pathway, though the precise EV content responsible for this action is unknown (88, 101). Several examples of MSC derived exosomes impact on cell survival, often through their micro RNA (miRNA) cargo affecting key signaling pathways controlling events in apoptosis and pyroptosis (276). Human UC-MSCs exosomes prevented NP cell pyroptosis by targeting METTL14 with a methyltransferase that catalyzes the m6A change (317). NP cell apoptosis decreased by miR-142-3p reducing IL1-induced inflammatory cytokine release and MAPK pathway activation (84). TNFα induced apoptosis, ECM breakdown, and fibrosis in NP cells was prevented via miR-532-5p targeting Ras association domain-containing protein 5 (RASSF5) (85). Pyroptosis in IVDD was reduced by miR-410 by binding to the pyrin domain containing 3 (NLRP3) mRNA (96, 318) and miR-26a-5p prevented pyroptosis by reducing NLRP3, IL1, and IL18 expression (317). IVDD and gait abnormalities improved through miR-4450 targeting the zinc finger protein 121 (97) and miR-141-3p via the Kelch-like ECH-associated protein 1 (Keap1)-Nuclear factor (erythroid-derived-2) like 2 (Nrf2) pathway reduced oxidative stress-induced pyroptosis in NP cells (319). In a recent systematic review it was further reported that stem cell-derived EVs can slow the progression of IVDD at the cellular, molecular and organ levels (320). Lastly, an ongoing clinical trial (NCT04849429) uses platelet-derived exosomes for IVDD and may soon provide useful in vivo evidence on the therapeutic effect of exosomes.
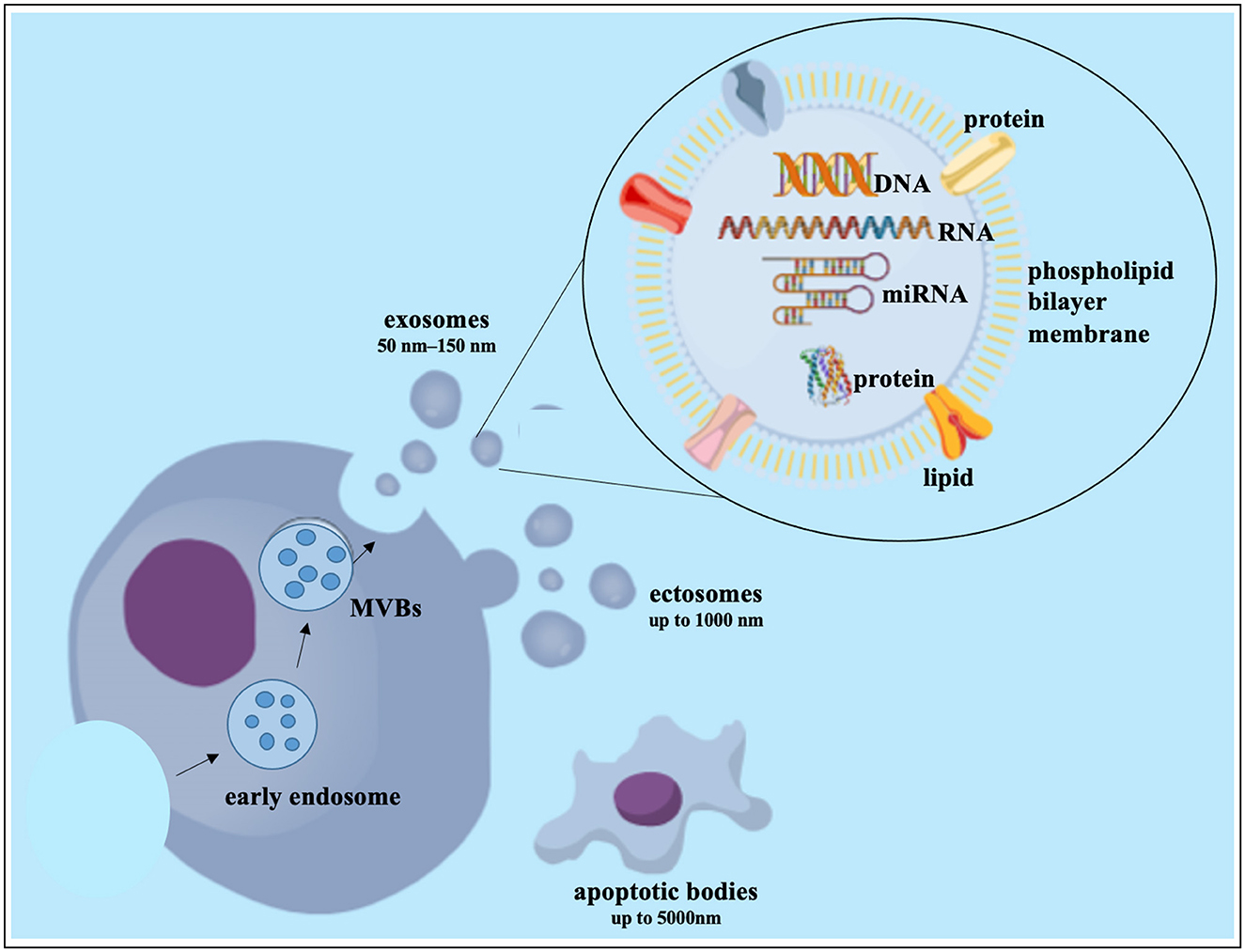
Figure 4. Exosome biogenesis and composition. DNA, deoxyribonucleic acid; miRNA, microRNA; MVB, multivesicular bodies; RNA, ribonucleic acid. This figure created in the Mind the Graph platform (www.mindthegraph.com).
Synthetic and non-synthetic scaffold-based therapies
Structural integrity and support can be provided via three-dimensional (3D) tissue scaffolds that enable cellular interactions between native tissues and the implant and provide structural support for the cells by mimicking cell-ECM interactions. The ECM, a composite of fibers, bioactive molecules and solutes is dynamic in vivo providing structure and signals to the cells that generate it (321). Regenerative medicine makes use of “inductive” properties of the ECM. In the early 1960s scaffolds were created as cell substrates to resemble the niche in which cells thrive, enabling cells to attach, differentiate and proliferate (17, 322). Ideally such a scaffold would be non-cytotoxic, biocompatible and eventually biodegradable (323). Recent research demonstrates promising biomaterials and processing techniques for IVD repair or regenerative strategies. Composite scaffolds that allow for simultaneous regeneration of cells and ECM would be most beneficial because IVDD affects both (324). In the past, 3D biomimetic scaffolds were created using a variety of methods: solvent casting, freeze drying, phase separation, leaching and electrospinning (325–327). Bioprinting is also investigated for the IVD. Although in its early stages, using this cutting-edge method could enhance the creation of IVD-based scaffolds (328). Based on the polymer used, these scaffolds can be considered synthetic or non-synthetic (natural).
Natural materials
The most prevalent protein in mammals, collagen, is employed extensively in biomedical procedures and its function is likely crucial for IVD regeneration (289, 329). Owing to its minimal antigenicity, atelocollagen is recognized as one of the best basic matrices for implantable materials (330). A mechanically stable, manageable, honeycomb-shaped atelocollagen scaffold promotes the development of high-density cell cultures (330) and may be beneficial as a 3D scaffold in tissue engineering given these properties (331). Silk scaffolds provide strength and stability through compressive and tensile properties. Silk fibroin proteins are synthesized by silkworms and other insects and are biodegradable (332, 333). Once implanted, the silk scaffold would decay slowly enough to permit healthy tissue growth (332, 334). Silk scaffolds are of interest for AF tissue engineering owing to their mechanical properties (335). The naturally occurring polymer chitosan is a very adaptable biomaterial (336). It comes from a natural and regenerative source: crab shells (337–340). Chitosan possesses a hydrophilic surface that encourages cell attachment and growth, and its degradation products are non-toxic (341). Alginate is utilized in a number of biomedical applications, including tissue engineering and drug delivery, because of its qualities in terms of biocompatibility, biodegradability, non-antigenicity, and chelating ability (342–346). A hybrid alginate/chitosan scaffold promotes ECM deposition, enhances AF cell proliferation, and degrades more slowly than a pure alginate scaffold (346). Another biomaterial which develops a stable hydrogel structure as a result of gelation is gellan gum (347). A 3D gel network that can be employed as a matrix for cell seeding is created when untangled sections of polysaccharide chains connect to orientated bundles of double helix structures (348–350). Natural polymers support cell adhesion and function (351).
Decellularized ECM-based scaffolds have drawn a lot of interest and have begun to be utilized extensively in a variety of tissues (heart valves, vascular grafts, cornea, etc.) (352–356). However, decellularization protocols cannot rely on perfusion in the largely avascular IVD and a balance between complete cell removal to avoid inflammatory triggers and ECM preservation to allow for bioactivity is important (357, 358). Decellularized scaffolds are currently commercialized for numerous therapeutic uses because of their pro-regenerative capabilities, and they may offer a promising alternative for IVD regeneration (357, 359).
Synthetic materials
Necessary forms and implants can also be created from synthetic polymers. Synthetic biodegradable polymers can generate stable porous materials that are predesigned 3D scaffolds and do not melt or disintegrate in in vitro tissue culture settings (360). The synthetic biodegradable polymers most frequently employed in tissue regeneration are aliphatic polyesters (351). The ester groups in these polymers' backbones are often hydrolyzed to produce deterioration, which can be regulated depending on the polymer's composition, structure, and molecular weight (361). A ring-opening polymerization of the monomers (lactide and/or glycolide) is a typical method for producing polylactide (PLA), polyglycolide (PGA), and their copolymer poly (lactide-co-glycolide) (PLGA) (362). These polymers are among the few synthetic polymers that the U.S. Food and Drug Administration (FDA) has approved for human clinical applications, such as surgical sutures and some implanted devices. Synthetic polyesters with a wide range of applications in AF tissue regeneration include poly ε-caprolactone (PCL), PGA, PLA and copolymers produced from these monomers (363, 364). PCL has been widely employed as a biocompatible polymer with reasonable cost and high mechanical qualities for electrospun fibrous scaffolds (365). Many synthetic polymers are hydrophobic with restricted water absorption requiring modifications for cell attachment. Some limitations in their biomedical applications, may be solved by including other polymers, such as natural or synthetic proteins and polysaccharides (366). A development in the field of synthetic scaffolds is the use of “conductive” or “smart” biomaterials. Conductive materials are typically polymer or nanomaterial-based additives to the scaffold allowing for the transfer of electromechanical signals to target cells (367). A conductive effect could also be achieved with natural polymers like collagen based on a described piezoelectric effect under load for ordered collagen fibers, especially collagen I (368). A piezoelectric potential of the AF and to a lesser degree NP tissue was described. This approach could facilitate more effective mechanically induced tissue remodeling and cell homing in the IVD (369).
NuCore® injectable nucleus hydrogel (Spine Wave, Inc., Shelton, CT, USA) as a substitute for NP tissue lost to herniation and microdiscectomy was investigated and seemed to prevent the disc from collapsing too soon after microdiscectomy (370). The FDA approved Discseel® which relieves chronic neck and low back discogenic pain offers a procedure to effectively repair discs to their normal states, both mechanically and biochemically (371). Owing to the relative short timeframe of follow up with some of these procedures not much clinical evidence to support these therapies is available. Also, secondary effects of IVDD, such as spinal stenosis and muscle fatigue caused by lumbar lordosis and loss of sagittal stability of the spine, may be more excruciating than structural transformation in the disc themselves (372).
Small molecules and growth factors-based therapies
Small molecules are substances that attach to certain biological molecules and aid in the regulation of a specific biological process (222). Small molecules can be taken orally (373). The maximum molecular weight for a molecule that needs to quickly diffuse through the cell membrane and be absorbed by the digestive system is 900 Daltons (374). Small molecules can significantly alter signaling transduction and gene transcription by intervening on specific signaling pathways regulating cell physiology and function (375). There are various benefits of using small molecules as a therapeutic agent. They cause fewer immune response in the host owing to their small size, and are considered to have anti-inflammatory, anti-apoptotic, and anti-oxidative effects accompanied by anabolism and anti-catabolic effects (222). The anti-inflammatory effect of small molecules such as berberine, morin, notoginsenoside R1, cannabidiol, curcumin, icariin, resveratrol, epigallocatechin gallate, naringenin, and tofacitinib was shown by the downregulation of IL1 and TNFα levels in IVD cells in a number of in vitro studies (222, 375, 376). Src homology region 2-containing protein tyrosine phosphatase 2 (SHP2) is an important contributor to the development of IVDD, and its small molecule inhibitor SHP099 prevented SHP2 expression and NP cell degeneration (377). Following toll-like receptor (TLR) 2/6 agonist induction, o-vanillin reduced TLR2 expression and SASP (378). Other small molecules acted in a multipotent manner. Curcumin showed cell-type and experiment dependent pro-apoptotic or anti-apoptotic effects. In IVDD it reduced the activity of proinflammatory cytokines by inhibition of the nuclear factor kappa B (NF-kB) and mitogen-activated protein kinase (MAPK) pathways, protected mitochondria and induced autophagy via its reactive oxygen species (ROS) scavenging capacity (379–384). Icariin, a bioactive and peroxylated flavonol glycoside compound isolated from herba epimedii or horny goat weed was investigated as a therapy of articular cartilage degenerative diseases (385). Its anti-oxidative and mitochondrial protective effects were attributed to the activation of the PI3K/Akt and Nrf2 signaling pathways, culminating in decreased ROS production and programmed cell death in NP cell (385, 386). Melatonin induced parkin-dependent mitophagy, also protected mitochondria (380, 387) and exhibited anti-inflammatory effects by inhibiting IL1 release and NLRP3 primed pyroptosis (388). When high hyperglycemia caused mitochondrial damage in end plate cells, alpha lipoic acid prevented apoptosis by increasing mitochondrial membrane potential (389).
Growth factor (GF) therapy involves the injection of bioactive molecules into the IVD to promote ECM production, prevent degeneration, and decrease inflammation (390, 391). GFs are peptides that bind to receptors and trigger physiological processes such as protein synthesis, differentiation, apoptosis, and cellular proliferation (392). Bone morphogenic proteins (BMPs) and other transforming growth factor (TGFβ) members, which promote osteogenesis and chondrogenesis, are the most well-known GFs in spine and orthopedic therapies (215). In an IVDD mouse model, TGFβ inhibitors decreased Nerve growth factor (Ngf) expression, indicating that TGFβ may control Ngf expression in vivo (393). Other GFs like BMPs, platelet derived growth factors (PDGF) and epidermal growth factor (EGF) inhibit proinflammatory cytokines including IL1, IL6, TNFα, MMPs, nitric oxide, and prostaglandin E2 (PGE2) and decrease catabolic activity (391, 394). The biological half-life of GFs is only a few hours to days, making it unsuitable for restoring degenerative discs when GF stability or long lasting effects are required (391, 394). Platelet-rich plasma (PRP) contains a variety of GFs (395, 396). PDGF decreased the percentage of apoptotic AF cells in vitro after of serum deprivation (397). An updated list of clinical trials for GFs in IVDD can be seen in Table 4 (clinicaltrials.gov).
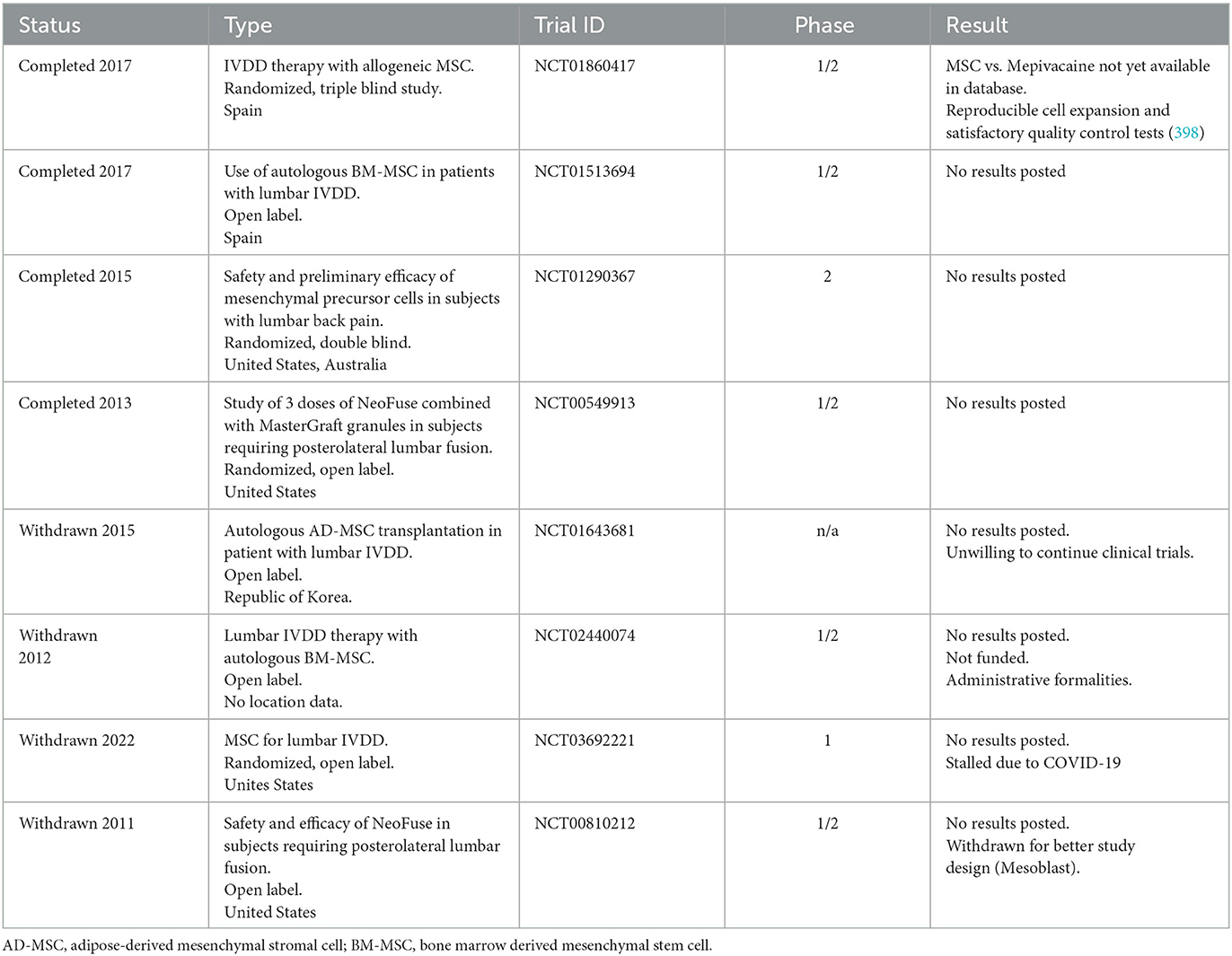
Table 4. Clinical trials reported with mesenchymal stem cells (MSC) in the context of intervertebral disc degeneration (IVDD) based on data from May 2023 (www.clinicaltrials.com).
Gene therapy
The use of nucleic acids such as DNA or RNA to cure a disease is known as gene therapy (399), often targeting monogenic congenital diseases or cancer. A plasmid (400) or oligonucleotide can be used (401). Gene therapy's potential long-term efficacy is a key benefit (402). Transfected cells that have received a therapeutic gene produce the desired gene products (RNAs or proteins). Stable transfection facilitates long-term expression of a transgene even in dividing cells if the foreign gene integrates into the host genome, however this can come at the expense of insertional mutagenesis. Cells that have been transiently transfected with an episomal vector also express a foreign gene but the foreign gene will be lost in dividing cells (403). IVDD is a chronic problem (129, 402, 404–406). Retrovirus (RV), lentivirus (LV), adenovirus (AV), and adeno-associated viruses (AAV) are common vectors (407). Replication incompetent RV were used in vitro to deliver DNA to cells purified from bovine coccygeal vertebral endplates suggesting that local gene therapy may be used to treat disc degeneration (129, 408, 409). Non-human LVs are considered apathogenic in humans but can transduce human cells. Replication-incompetent LV vectors are available (410). To demonstrate that LV-mediated MMP3 knockdown may lessen IVDD, LV-MMP3-shRNA and/or LVSox9 were administered to rabbit lumbar discs. This significantly delayed the progression of IVDD and increased collagen type II and proteoglycan expression (128). Insertional mutagenesis remains a concern associated with highly efficient RV and LV vectors (411). The AV genome persists in an extrachromosomal state. Standard recombinant AV vectors can carry up to 7.5 kb of foreign DNA (412). To further increase the packaging capacity to more than 30 kb AV genes are provided in-trans by a helper virus (413). A recombinant AV vector was used to deliver the lacZ gene to female New Zealand white rabbit NP cells in vitro and in vivo (130). The AV-lacZ construct was directly injected into the NP of the rabbit's lumbar IVD for the in vivo model. The successful transduction of disc NP cells was demonstrated by X-Gal (5-bromo-4-chloro-3-indolyl-D-galactopyranoside) staining and reporter gene expression persisted in vivo for at least 12 weeks. This study demonstrated the promise of direct gene therapy for a treatment of IVDD by successfully delivering a foreign gene to the IVD (414, 415). AV have several different serotypes, including 51 in humans (412); Ad5 is the most common and 45–80% of the population has neutralizing antibodies against this serotype (412). Unfortunately AV vectors in general can cause severe and even lethal inflammatory reactions (416, 417). AAV's are used more recently as non-pathogenic, generally non-integrating gene therapy vectors suitable for dividing and non-dividing cells. However, it is challenging to generate the high titers needed for human clinical studies and the packaging capacity is limited (415, 418). The activatorprotein-2 (Ap2α) impacts IVDD via controlling the expression of Tgfβ and Smad3 (132). Rat IVDs injected AAV-Ap2α and AAV-Tgfβ, increased the expression of Acan, Collagen II and decreased the expression of Mmp2, Mmp9, and Smad3 in NP tissue (132). However, in general, viral vector based gene therapy carries a risk of viral component-related complications (419, 420).
The post-transcriptional RNA interference (RNAi) mechanism evolved as a crucial biological strategy for targeted gene silencing (402). The reporter genes firefly and renilla luciferase were downregulated in NP cells in vitro in a co-transfection experiment and achieved considerable inhibition of reporter gene expression in both cell types for 3 weeks, suggesting siRNA-mediated gene silencing as effective in NP cells (421). Moreover, in rat coccygeal IVDs, siRNA-mediated RNAi remained active for at least 24 weeks to down regulate in vivo expression of the endogenous Fas ligand, as well as a reporter gene (422). MRI and histological studies showed that a single injection of ADAMTS5 siRNA prevented NP tissue breakdown after annular puncture in vivo (423). Apoptosis in the discs was also significantly reduced by siRNA therapy intervention against Caspase3 and ADAMTS5 (424). Inhibiting TLR4 and overexpressing Klotho via RNAi in a rat IVDD model decreased ROS induced inflammation (133). Klotho promotes antiaging through the modulation of numerous signaling pathways, including TLR4/NF-kB signaling (133, 425). However, in vivo applications could be hampered by RNAi associated immune stimulation, off-target effects and the low number of target cells in the IVD (426). There were no clinical trials reported for gene therapy-based therapeutics in IVDD until now (clinicaltrials.gov).
Most recently CRISPR/Cas9 (427) was also added to the growing toolkit for IVDD therapy development. Potential applications for CRISPR/Cas9 gene editing, targeting or labeling to enhance IVD research by generating new disease models, new means of studying IVD cell phenotypes and possible clinical translations thereof were suggested and reviewed (428). As a promising recent example, AAV delivered CRISPR/Cas9 to target β-catenin reduced IVDD in the mouse model (429) and CRISPR epigenome editing systems could be introduced into pathological human IVDs in vitro using LV vectors to control expression of inflammatory receptors. This could suppress negative impacts of inflammatory cytokines in the IVD. TNFR1 epigenome-edited cells showed decreased NF-kB activation, reduced apoptosis, and suppression of catabolic gene expression changes (430).
Discussion
The IVD at first glance appears as a simple organ comprised of just two major tissue types with few residing cells under extreme mechanical or physiological stress yet it is possibly one of the most challenging enigmas in the vertebrate body to solve. Therefore, despite IVDD being a primary health concern, it still must find a permanent cure. IVDD decreases the quality of life by causing chronic discomfort and discogenic pain due to multifactorial changes in the degenerating IVD as previously described in detail (431). The IVD is susceptible to a variety of risk factors and can deteriorate because of a pathologic cascade resulting in metabolic and cellular changes in IVD cells. Classic IVDD therapies were reviewed before (431) and are available in health centers, but often encounter a “roadblock” in that they only relieve symptoms but do not restore structure and functionality to the disc. Surgical options for IVDD are often ambiguous and carry underlying hazards and complications, hence, they should only be used after conservative measures have failed, as their outcome depends on a surgeons' experience and technical expertise, as well as a patients' comorbidities (391).
Advanced therapies of low back pain as summarized in Figure 5 show some promising results in mostly animal studies (Table 1) but still have their own safety concerns and limitations. To start, these novel interventions once intended for clinical applications first require the approval by appropriate government bodies such as the FDA in the United States, European Medicines Agency (EMA) in Europe or the Central Drugs Standard Control Organization (CDSCO) in India. Based on successful outcomes of pre-clinical studies an investigational new drug application (IND) can be filed with the FDA triggering several phases and years of clinic trials with uncertain outcome for the investigator and high financial risk for sponsors as previously described (320). Despite all, progress is evident and current, and future research will hopefully translate many of these cutting-edge technologies from benchside to bedside as alternative IVDD therapies despite plenty of challenges that remain to be addressed.
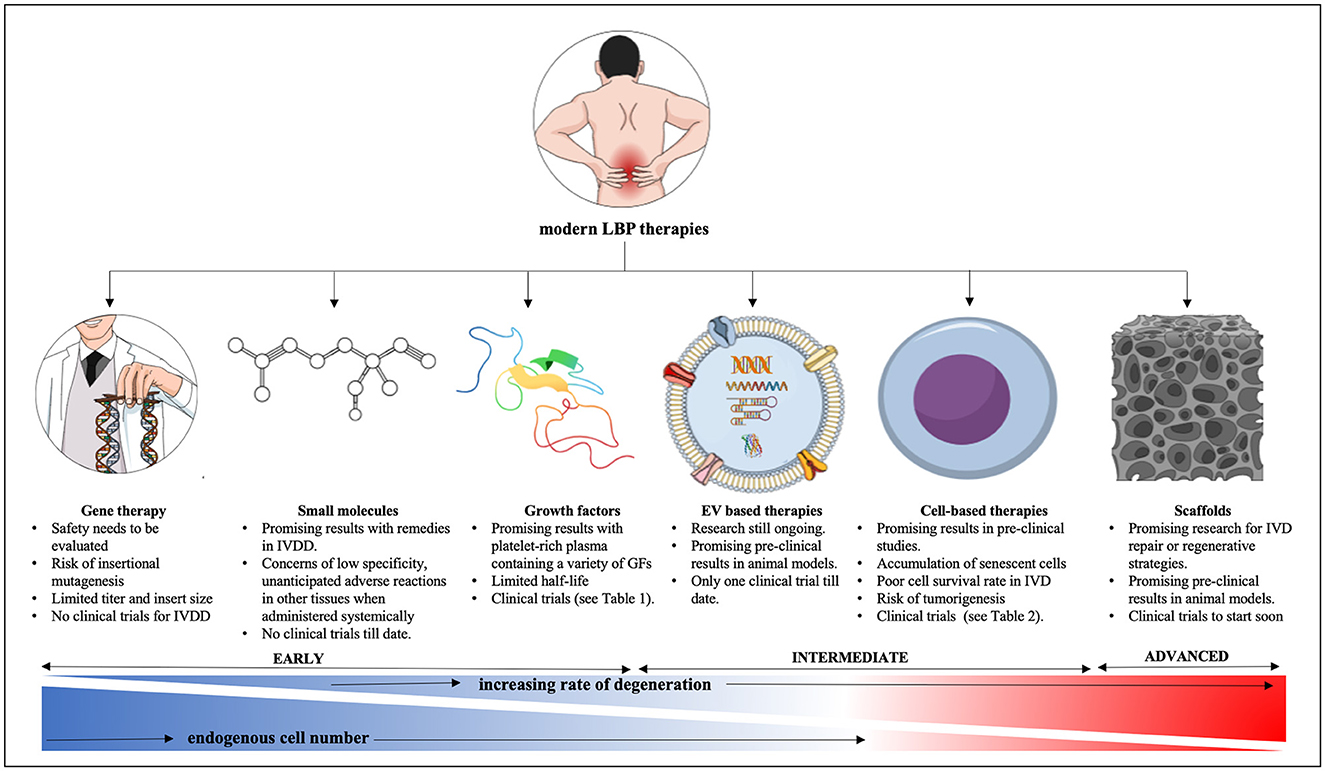
Figure 5. Summary of modern therapy approaches for lower back pain from IVDD. IVD, intervertebral disc; IVDD, intervertebral disc degeneration; GF, growth factor; AAV, adeno-associated virus. This figure was created in the Mind the Graph platform (www.mindthegraph.com).
Owing to the avascular nature of the IVD, systemically applied therapies are less suitable. Therefore, exogeneous and endogenous interventions would require intradiscal injections of cells, hydrogels, GF, small molecules, viral vectors or combinations thereof. Such injections produce a bolus of compressed fluid at the injection site that may take a long time to diffuse into the surrounding tissue due to a high degree of stiffness and limited permeability in NP tissue. This pressure may cause fluid to leak through the AF defect after the needle retraction (432–434). The severity of AF disruption can vary depending on the needle gauge used, stiffness and fluid viscosity and would require oversight to assess the danger of leakage, especially for more advanced therapies such as gene therapy involving viral vectors (435–438). While the avascular nature of the NP limits the use of systemic interventions it could keep side effects limited after intradiscal delivery, provided no leakage at the injection site. Despite promising potential, currently, small molecule drugs have little clinical relevance in IVDD as they do not appear to offer a significant advantage over NSAIDs (375, 439, 440). The reasons might be low specificity, the avascular nature of the IVD and unanticipated adverse reactions in other tissues when administered systemically. To date, most in vivo studies have focused on rodent models, and more appropriate translational models are needed for an honest assessment of safety and efficacy of small molecules as alternative strategy to NSAIDs.
Recent advancements in sequencing technologies identified genetic defects associated with IVDD and LBP and will enable more personalized therapy approaches. At the same time, increased knowledge of cellular events at the molecular level facilitates more targeted therapies with recombinant or xenofree bioactive molecules or inhibitors thereof down to modulations of intracellular signaling pathways, for example those involving cytokine triggered inflammation, regulated cell death or SASP in the IVD (222, 441, 442). However, despite success in animal models, IVDD human gene therapy in the classic sense of gene delivery is unlikely to be a mainstream intervention any time in the near future as IVDD is not a monogenic disease and current technologies do not allow to effectively and safely alter multiple genes in vivo. Safer viral and non-viral vectors with improved cargo capacity and better transfection efficiency at a lower dosage alongside reduced immune response activation are needed for increased safety and efficacy of gene therapy in general. Promising work using engineered AAVs and serotypes with different tropism were underway for several diseases until recent setbacks sent once again alarming signals through the gene therapy community (443). Gene therapy for IVDD ideally employs vectors that can target NP cells specifically through unique cell surface/viral capsid protein interactions, however, this could arise as one of the bigger challenges given that the adult NP cell population is heterogeneous and suitable NP cell unique cell surface markers have yet to be discovered (41, 42). RNAi as tool to downregulate proinflammatory responses seems more promising. Recent research showed that miR-370-3p-regulated circular RNA (circRNA) PKNOX1 controlled the expression of KIAA0355, which impacted on IVDD progression, hence circPKNOX1-based therapy may become useful (444). However, in vivo off-target effects remain a concern for RNAi and CRISPR gene editing for now. A better understanding of the pleiotropic impact of bioactive molecules like miRNAs on various, often connected signaling pathways including those critical in inflammatory response, senescence, cell cycle arrest and regulated cell death is crucial for safety and efficacy (222).
Endogenous cell-based therapies stimulating native IVD progenitor cells depend largely on effective and safe delivery of the stimulant, while exogenous approaches transplanting autologous/allogenic cells depend on the ability of those cells to settle, survive and be productive in a challenging or degenerated environment. Cell therapies struggle with the accumulation of senescent cells, a poor survival rate of transplanted cells and the necessity of correct differentiation (445). Increased cell death post transplantation could trigger inflammasome related pyroptosis and further aggravate IVDD. Many in vitro studies have investigated the efficacy of MSCs in preserving and reactivating NP cells isolated from healthy or degenerate discs by maintaining or enhancing ECM synthesis as well as by encouraging upregulation of NP markers, which are diminished within the diseased disc (197, 446–448). In a variety of studies, MSCs such as those produced from bone marrow (BM-MSCs), adipose tissue (AD-SCs), and umbilical cord (UC-MSCs) were employed alone or in combination with biomaterial scaffolds and carriers to repair and regenerate the ailing IVD (137, 449, 450). However, if non-autologous cells are used the problem of host rejection presents itself and even if the cells are tolerated, it remains unclear if these added cells can survive long enough under conditions they encounter in the degenerate IVD (285). Few studies have examined how transplanted cells interact with the native disc microniche. However, some evidence backs the delivered cells' ability to reduce inflammation in degenerating discs (6, 451). In vitro data from 2D culture where required culture supplements such as serum or glucose and frequent medium changes might not reflect a natural IVD environment need to be evaluated with skepticism. Further large animal and advanced organ culture models, as well as clinical trials, are needed to confirm findings from these in vitro experiments. Numerous animal models were used in preclinical research examining cell therapies for IVD regeneration (452). Mechanical, enzymatic, or surgical methods can be used to study disc degeneration in a variety of species, including mice, rats, rabbits, pigs, sheep, goats, cows, and dogs (Table 1). Yet comparative interpretations are challenging and frequently do not yield knowledge that is easily applicable to human studies owing to a lack of agreement between different animal models (453). In particular distinctions in NP cell composition, the variable persistence of NC cells, as well as biomechanical differences hamper the translatability of small animal models (37, 41, 46, 165, 223, 454–459).
The recently developing field of EV based IVDD therapies faces challenges and bottlenecks with production cost, quality assurance of batch-to-batch homogeneity, and long-term stability of EVs. High purity production of EVs is often based on costly differential ultracentrifugation or affinity chromatography (460). The International Society for Extracellular Vesicles (ISEV) so far proposes only minimal guidelines for EV isolation and functional analysis and a range of investigator determined EV isolation and characterization methods exists (320, 461, 462). Cold chain storage for EVs was suggested but different opinions on how storage affects EV quality exist as well (463–465). A range of responses in EV recipient cells or EV parent culture conditions as well as different interaction modes between cells and EV types might complicate the interpretation of regenerative outcomes (320). Despite success in the purification of exosomes, the exact molecular mechanisms of exosome function are still under investigation. Establishing large-scale upstream and downstream manufacturing processes, accurate dosing regiments and efficacy evaluations will likely present major obstacles for quality EV-based therapeutics, yet it will be important to safely implement their application for IVDD therapy (445).
Progress made in tissue engineering over the years using a combination of natural and synthetic biomedical scaffolds, cells and bioactive molecules represents an exciting new era. In clinical trials these approaches often fail to address discogenic pain (372). For example, to date no research on ectopic sensory nerve distribution after MSC delivery to the painful disc is available. Detailed reviews and discussions of different scaffold types exist, and a “holistic” approach for IVD regeneration was emphasized by simultaneous NP, AF and CEP repair (368). Successful strategies to replace IVD tissue with non-biological scaffolds must address the unique biological shock absorbing function of the NP and/or the ECM-provided structural architecture such as the angle-ply arrangement in the AF or the spacing of different size fibers in the NP alongside the importance of continued CEP porosity, as a whole facilitating inductive and permissive signals for cells and tissue homeostasis. In light of the abundance of studies aimed at IVD regeneration presented in the literature, regeneration of CEPs is rarely addressed, despite being a significant source of nutrients and water supply for the IVD (368). A recent study found that the human CEPs have a distinct structure and, ECM composition when compared to the NP, AF, and articular cartilage (466), while others investigate CEP composition for diagnostic purposes (467) or how impaired CEP healing after surgery relates to IVDD (468). Generally, research on CEPs and the AF still does not have the momentum seen in NP research, therefore a need to include AF and CEPs more in overarching regenerative research and the development of therapeutic strategies remains.
In summary, promising IVDD therapies are developing in different areas, and possibly the combined effort will lead to biocompatible scaffolds loaded with protected bioactive molecules, EVs and/or MSC that can mobilize and recruit local progenitor cells. Examples of such efforts are underway. In a preclinical IVDD rabbit model, platelet-derived growth factor BB (PDFG-BB) delivery in a thiol-modified hyaluronic acid hydrogel significantly reduced disc degeneration by preventing apoptosis and raising collagen-3 production, preserved disc structure, and enabled biomechanical functions (70, 200). Combining a thermosensitive acellular ECM hydrogel with AD-MSC-exosomes to create an injectable functionalized ECM hydrogel could prevent pyroptosis in rat discs by lowering the expression of NLRP3 inflammasomes and minimizing the inflammatory response (469). However, additional data from pre-clinical research, clinical trials and long-term follow up assessments will be needed to ensure safety and efficacy of any approach. Several recent scRNASeq and GWAS projects provide very valuable data to better understand IVDD and it would be constructive to the field to expand these studies to more age groups, all genders, ethnicities, and stages of IVDD to identify master regulators in NP development and IVDD progression. IVDD is multifactorial and likely results from a combination of environmental risk factors and genetic predisposition. An overarching concept of modern therapies for IVD tissue homeostasis relies on the introduction, maintenance or stimulation and directed differentiation of stem/progenitor cells supported by suitable scaffolds preventing triggers of senescence or regulated cell death.
Author contributions
AS, TL, and PK contributed to drafting this manuscript. All authors contributed to the article and approved the submitted version.
Funding
The authors would like to thank Bayard and Virginia Clarkson Endowment and NIH Grant HD099588-01 granted to TL.
Conflict of interest
The authors declare that the research was conducted in the absence of any commercial or financial relationships that could be construed as a potential conflict of interest.
Publisher's note
All claims expressed in this article are solely those of the authors and do not necessarily represent those of their affiliated organizations, or those of the publisher, the editors and the reviewers. Any product that may be evaluated in this article, or claim that may be made by its manufacturer, is not guaranteed or endorsed by the publisher.
References
1. Gatchel R. The continuing and growing epidemic of chronic low back pain. Healthcare. (2015) 3:838–45. doi: 10.3390/healthcare3030838
2. Ren BO, Khambete P, Rasendran C, O'Donnell JA, Ahn NU. Quantifying the economic impact of depression for spine patients in the United States. Clin Spine Surg Spine Publ. (2022) 35:E374–9. doi: 10.1097/BSD.0000000000001220
3. Yong RJ, Mullins PM, Bhattacharyya N. Prevalence of chronic pain among adults in the United States. Pain. (2022) 163:e328–32. doi: 10.1097/j.pain,.0000000000002291
4. Diwan AD, Melrose J. Intervertebral Disc Degeneration and How it Leads to Low Back Pain JOR Spine. (2022) 1231. doi: 10.1002/jsp2.1231
5. Sampara P, Banala RR, Vemuri SK, Av GR, Gpv S. Understanding the molecular biology of intervertebral disc degeneration and potential gene therapy strategies for regeneration: a review. Gene Ther. (2018) 25:67–82. doi: 10.1038/s41434-018-0004-0
6. Binch ALA, Fitzgerald JC, Growney EA, Barry F. Cell-based strategies for IVD repair: clinical progress and translational obstacles. Nat Rev Rheumatol. (2021) 17:158–75. doi: 10.1038/s41584-020-00568-w
7. Guo HR, Tanaka S, Halperin WE, Cameron LL. Back pain prevalence in US industry and estimates of lost workdays. Am J Public Health. (1999) 89:1029–35. doi: 10.2105/AJPH.89.7.1029
8. Taylor TK, Ghosh P, Bushell GR. The contribution of the intervertebral disk to the scoliotic deformity. Clin Orthop. (1981) 79–90. doi: 10.1097/00003086-198105000-00010
9. Fenn J Olby NJ The The Canine Spinal Cord Injury Consortium (CANSORT-SCI) Moore SA Olby NJ Gjessing KM . Classification of intervertebral disc disease. Front Vet Sci. (2020) 7:e579025. doi: 10.3389/fvets.2020.579025
10. Bayliss MT, Johnstone B, O'brien JP. Volvo award in basic science: proteoglycan synthesis in the human intervertebral disc: variation with age, region and pathology. Spine. (1988) 13:972–81. doi: 10.1097/00007632-198809000-00003
11. Bibby SRS, Jones DA, Lee RB, Yu J, Urban JPG. The pathophysiology of the intervertebral disc. Joint Bone Spine. (2001) 68:537–42. doi: 10.1016/S1297-319X(01)00332-3
12. Eyre DR. Biochemistry of the intervertebral disc. In: International Review of Connective Tissue Research. Elsevier (1979). p. 227–91. doi: 10.1016/b978-0-12-363708-6.50012-6
13. Humzah MD, Soames RW. Human intervertebral disc: structure and function. Anat Rec. (1988) 220:337–56. doi: 10.1002/ar.1092200402
14. Oegema TR. Biochemistry of the intervertebral disc. Clin Sports Med. (1993) 12:419–38. doi: 10.1016/S0278-5919(20)30404-X
15. Sivakamasundari V, Lufkin T. Stemming the degeneration: IVD stem cells and stem cell regenerative therapy for degenerative disc disease. Adv Stem Cells. (2013) 2013:724547. doi: 10.5171/2013.724547
16. Nachemson A, Lewin T, Maroudas A, Freeman MA. In vitro diffusion of dye through the end-plates and the annulus fibrosus of human lumbar inter-vertebral discs. Acta Orthop Scand. (1970) 41:589–607. doi: 10.3109/17453677008991550
17. Dou Y, Sun X, Ma X, Zhao X, Yang Q. Intervertebral disk degeneration: the microenvironment and tissue engineering strategies. Front Bioeng Biotechnol. (2021) 9:e592118. doi: 10.3389/fbioe.2021.592118
18. Raj PP. Intervertebral disc: anatomy-physiology-pathophysiology-treatment. Pain Pract. (2008) 8:18–44. doi: 10.1111/j.1533-2500.2007.00171.x
19. Wang Sz, Rui Yf, Lu J, Wang C. Cell and molecular biology of intervertebral disc degeneration: current understanding and implications for potential therapeutic strategies. Cell Prolif. (2014) 47:381–90. doi: 10.1111/cpr.12121
20. Frost B, Camarero-Espinosa S, Foster E. Materials for the spine: anatomy, problems, and solutions. Materials. (2019) 12:253. doi: 10.3390/ma12020253
21. Urban J, Maroudas A. 4 - The chemistry of the intervertebral disc in relation to its physiological function and requirements. Clin Rheum Dis. (1980) 6:51–76. doi: 10.1016/S0307-742X(21)00280-0
22. Buckwalter JA. Aging and degeneration of the human intervertebral disc. Spine. (1995) 20:1307–14. doi: 10.1097/00007632-199506000-00022
23. Christ B, Wilting J. From somites to vertebral column. Ann Anat Anat Anz. (1992) 174:23–32. doi: 10.1016/S0940-9602(11)80337-7
24. Walmsley R. The development and growth of the intervertebral disc. Edinb Med J. (1953) 60:341–64.
25. De Bree K, De Bakker BS, Oostra R-J. The development of the human notochord. PLoS ONE. (2018) 13:e0205752. doi: 10.1371/journal.pone.0205752
26. Barrionuevo F, Taketo MM, Scherer G, Kispert A. Sox9 is required for notochord maintenance in mice. Dev Biol. (2006) 295:128–40. doi: 10.1016/j.ydbio.2006.03.014
27. Chatterjee S, Sivakamasundari V, Yap SP, Kraus P, Kumar V, Xing X, et al. In vivo genome-wide analysis of multiple tissues identifies gene regulatory networks, novel functions and downstream regulatory genes for Bapx1 and its co-regulation with Sox9 in the mammalian vertebral column. BMC Genomics. (2014) 15:1072. doi: 10.1186/1471-2164-15-1072
28. Choi K-S, Lee C, Harfe BD. Sonic hedgehog in the notochord is sufficient for patterning of the intervertebral discs. Mech Dev. (2012) 129:255–62. doi: 10.1016/j.mod.2012.07.003
29. Choi K-S, Cohn MJ, Harfe BD. Identification of nucleus pulposus precursor cells and notochordal remnants in the mouse: Implications for disk degeneration and chordoma formation. Dev Dyn. (2008) 237:3953–8. doi: 10.1002/dvdy.21805
30. Choi K-S, Harfe BD. Hedgehog signaling is required for formation of the notochord sheath and patterning of nuclei pulposi within the intervertebral discs. Proc Natl Acad Sci. (2011) 108:9484–9. doi: 10.1073/pnas.1007566108
31. Lee WJ, Chatterjee S, Yap SP, Lim SL, Xing X, Kraus P, et al. An integrative developmental genomics and systems biology approach to identify an in vivo sox trio-mediated gene regulatory network in murine embryos. BioMed Res Int. (2017) 2017:1–16. doi: 10.1155/2017/8932583
32. Peters H, Wilm B, Sakai N, Imai K, Maas R, Balling R. Pax1 and Pax9 synergistically regulate vertebral column development. Development. (1999) 126:5399–408. doi: 10.1242/dev.126.23.5399
33. Sharma S. Dehydro-emetine induced depression. Indian J Med Sci. (1976) 30:239–40. doi: 10.1163/157007276X00302
34. Sivakamasundari V, Kraus P, Sun W, Hu X, Lim SL, Prabhakar S, et al. A developmental transcriptomic analysis of Pax1 and Pax9 in embryonic intervertebral disc development. Biol. Open. (2016) 6:023218. doi: 10.1242/bio.023218
35. Smits P, Lefebvre V. Sox5 and Sox6 are required for notochord extracellular matrix sheath formation, notochord cell survival and development of the nucleus pulposus of intervertebral discs. Development. (2003) 130:1135–48. doi: 10.1242/dev.00331
36. Tribioli C, Lufkin T. The murine Bapx1 homeobox gene plays a critical role in embryonic development of the axial skeleton and spleen. Development. (1999) 126:5699–711. doi: 10.1242/dev.126.24.5699
37. Vujovic S, Henderson S, Presneau N, Odell E, Jacques T, Tirabosco R, et al. Brachyury, a crucial regulator of notochordal development, is a novel biomarker for chordomas. J Pathol. (2006) 209:157–65. doi: 10.1002/path.1969
38. Doskocil M, Valouch P, Pazderka V. On vertebral body growth. Funct Dev Morphol. (1993) 3:149–55.
39. Hunter CJ, Matyas JR, Duncan NA. Cytomorphology of notochordal and chondrocytic cells from the nucleus pulposus: a species comparison. J Anat. (2004) 205:357–62. doi: 10.1111/j.0021-8782.2004.00352.x
40. Chen J, Yan W, Setton LA. Molecular phenotypes of notochordal cells purified from immature nucleus pulposus. Eur Spine. (2006) 15(Suppl. 3):S303–311. doi: 10.1007/s00586-006-0088-x
41. Li K, Kapper D, Youngs B, Kocsis V, Mondal S, Kraus P, et al. Potential biomarkers of the mature intervertebral disc identified at the single cell level. J Anat. (2019) 234:16–32. doi: 10.1111/joa.12904
42. Li K, Kapper D, Mondal S, Lufkin T, Kraus P. Quantitative single-cell transcript assessment of biomarkers supports cellular heterogeneity in the bovine IVD. Vet Sci. (2019) 6:42. doi: 10.3390/vetsci6020042
43. Miyazaki T, Kobayashi S, Takeno K, Meir A, Urban J, Baba H. A phenotypic comparison of proteoglycan production of intervertebral disc cells isolated from rats, rabbits, and bovine tails; which animal model is most suitable to study tissue engineering and biological repair of human disc disorders? Tissue Eng. Part A. (2009) 15:3835–46. doi: 10.1089/ten.tea.2009.0250
44. Pattappa G, Li Z, Peroglio M, Wismer N, Alini M, Grad S. Diversity of intervertebral disc cells: phenotype and function: diversity of intervertebral disc cells. J Anat. (2012) 221:480–96. doi: 10.1111/j.1469-7580.2012.01521.x
45. Harfe BD. Intervertebral disc repair and regeneration: insights from the notochord. Semin Cell Dev Biol. (2022) 127:3–9. doi: 10.1016/j.semcdb.2021.11.012
46. Kraus P, Yerden R, Kocsis V, Lufkin T. RNA in situ hybridization characterization of non-enzymatic derived bovine intervertebral disc cell lineages suggests progenitor cell potential. Acta Histochem. (2017) 119:150–60. doi: 10.1016/j.acthis.2016.12.004
47. Sakai D, Nakamura Y, Nakai T, Mishima T, Kato S, Grad S, et al. Exhaustion of nucleus pulposus progenitor cells with ageing and degeneration of the intervertebral disc. Nat Commun. (2012) 3:1264. doi: 10.1038/ncomms2226
48. Séguin CA, Chan D, Dahia CL, Gazit Z. Latest advances in intervertebral disc development and progenitor cells. JOR Spine. (2018) 1:e1030. doi: 10.1002/jsp2.1030
49. Tekari A, Chan SCW, Sakai D, Grad S, Gantenbein B. Angiopoietin-1 receptor Tie2 distinguishes multipotent differentiation capability in bovine coccygeal nucleus pulposus cells. Stem Cell Res Ther. (2016) 7:75. doi: 10.1186/s13287-016-0337-9
50. Gao B, Jiang B, Xing W, Xie Z, Luo Z, Zou W. Discovery and application of postnatal nucleus pulposus progenitors essential for intervertebral disc homeostasis and degeneration. Adv Sci. (2022) 9:2104888. doi: 10.1002/advs.202104888
51. Mohanty S, Pinelli R, Pricop P, Albert TJ, Dahia CL. Chondrocyte-like nested cells in the aged intervertebral disc are late-stage nucleus pulposus cells. Aging Cell. (2019) 18:e13006. doi: 10.1111/acel.13006
52. Hunter CJ, Matyas JR, Duncan NA. The notochordal cell in the nucleus pulposus: a review in the context of tissue engineering. Tissue Eng. (2003) 9:667–77. doi: 10.1089/107632703768247368
53. Iwata M, Aikawa T, Hakozaki T, Arai K, Ochi H, Haro H, et al. Enhancement of Runx2 expression is potentially linked to β-catenin accumulation in canine intervertebral disc degeneration. J Cell Physiol. (2015) 230:180–90. doi: 10.1002/jcp.24697
54. Gan Y, He J, Zhu J, Xu Z, Wang Z, Yan J, et al. Spatially defined single-cell transcriptional profiling characterizes diverse chondrocyte subtypes and nucleus pulposus progenitors in human intervertebral discs. Bone Res. (2021) 9:1–15. doi: 10.1038/s41413-021-00163-z
55. Han S, Zhang Y, Zhang X, Zhang H, Meng S, Kong M, et al. Single-cell RNA sequencing of the nucleus pulposus reveals chondrocyte differentiation and regulation in intervertebral disc degeneration. Front Cell Dev Biol. (2022) 10:e824771. doi: 10.3389/fcell.2022.824771
56. Li Z, Ye D, Dai L, Xu Y, Wu H, Luo W, et al. Single-cell RNA sequencing reveals the difference in human normal and degenerative nucleus pulposus tissue profiles and cellular interactions. Front Cell Dev Biol. (2022) 10:e910626. doi: 10.3389/fcell.2022.910626
57. Boni M, Denaro V. Anatomo-clinical correlations in cervical spondylosis. In:Kehr P, Weidner A, , editors. Cervical Spine I. Vienna: Springer (1987). p. 3–20.
58. Marcia S, Zini C, Bellini M, Clerk-Lamalice O. Minimally invasive percutaneous treatment of lumbar disk degeneration and stenosis. In:Khan M, Kushchayev SV, Faro SH, , editors. Image Guided Interventions of the Spine. Cham: Springer International Publishing (2021). p. 329–50.
59. Miller JA, Schmatz C, Schultz AB. Lumbar disc degeneration: correlation with age, sex, and spine level in 600 autopsy specimens. Spine. (1988) 13:173–8. doi: 10.1097/00007632-198802000-00008
60. DePasse JM, Durand W, Palumbo MA, Daniels AH. Sex- and sport-specific epidemiology of cervical spine injuries sustained during sporting activities. World Neurosurg. (2019) 122:e540–5. doi: 10.1016/j.wneu.2018.10.097
61. Meron A, McMullen C, Laker SR, Currie D, Comstock RD. Epidemiology of cervical spine injuries in high school athletes over a ten-year period. PMandR. (2018) 10:365–72. doi: 10.1016/j.pmrj.2017.09.003
62. Owen PJ, Hangai M, Kaneoka K, Rantalainen T, Belavy DL. Mechanical loading influences the lumbar intervertebral disc. A cross-sectional study in 308 athletes and 71 controls. J Orthop Res. (2021) 39:989–97. doi: 10.1002/jor.24809
63. Lotz JC, Ulrich JA. Innervation, inflammation, and hypermobility may characterize pathologic disc degeneration: review of animal model data. J Bone Joint Surg Am. (2006) 88(Suppl. 2):76–82. doi: 10.2106/00004623-200604002-00016
64. Elsaka O, Noureldean MA, Gamil MA, Ghazali MT, Al-Razik AHA, Hisham D. Pathophysiology, investigations and operative management in cases of lumbar degenerative disc disease. Asian Basic Appl Res J. (2022) 4:44–65.
65. Tow BPB, Hsu WK, Wang JC. Disc regeneration: a glimpse of the future. Clin Neurosurg. (2007) 54:122–8.
66. An HS, Masuda K, Cs-Szabo G, Zhang Y, Chee A, Andersson GBJ, et al. Biologic repair and regeneration of the intervertebral disk. J Am Acad Orthop Surg. (2011) 19:450–2. doi: 10.5435/00124635-201107000-00008
67. Ju DG, Kanim LE, Bae HW. Intervertebral disc repair: current concepts. Glob Spine J. (2020) 10:130S–6S. doi: 10.1177/2192568219872460
68. Lyu F-J, Cheung KM, Zheng Z, Wang H, Sakai D, Leung VY. IVD progenitor cells: a new horizon for understanding disc homeostasis and repair. Nat Rev Rheumatol. (2019) 15:102–12. doi: 10.1038/s41584-018-0154-x
69. Moriguchi Y, Alimi M, Khair T, Manolarakis G, Berlin C, Bonassar LJ, et al. Biological treatment approaches for degenerative disk disease: a literature review of in vivo animal and clinical data. Glob Spine J. (2016) 6:497–518. doi: 10.1055/s-0036-1571955
70. Paglia DN, Singh H, Karukonda T, Drissi H, Moss I. PDGF-BB delays degeneration of the intervertebral discs in a rabbit preclinical model. Spine. (2016) 41:E449–58. doi: 10.1097/BRS.0000000000001336
71. An HS, Takegami K, Kamada H, Nguyen CM, Thonar EJ-MA, Singh K, et al. Intradiscal administration of osteogenic protein-1 increases intervertebral disc height and proteoglycan content in the nucleus pulposus in normal adolescent rabbits. Spine. (2005) 30:25–31; discussion 31–32. doi: 10.1097/01.brs.0000148002.68656.4d
72. Masuda K. Biological repair of the degenerated intervertebral disc by the injection of growth factors. Eur Spine J. (2008) 17:441. doi: 10.1007/s00586-008-0749-z
73. Imai Y, Miyamoto K, An HS, Thonar EJ-MA, Andersson GBJ, Masuda K. Recombinant human osteogenic protein-1 upregulates proteoglycan metabolism of human anulus fibrosus and nucleus pulposus cells. Spine. (2007) 32:1303–9; discussion 1310. doi: 10.1097/BRS.0b013e3180593238
74. Huang K-Y, Yan J-J, Hsieh C-C, Chang M-S, Lin R-M. The in vivo biological effects of intradiscal recombinant human bone morphogenetic protein-2 on the injured intervertebral disc: an animal experiment. Spine. (2007) 32:1174–80. doi: 10.1097/01.brs.0000263369.95182.19
75. Chujo T, An HS, Akeda K, Miyamoto K, Muehleman C, Attawia M, et al. Effects of growth differentiation factor-5 on the intervertebral disc–in vitro bovine study and in vivo rabbit disc degeneration model study. Spine. (2006) 31:2909–17. doi: 10.1097/01.brs.0000248428.22823.86
76. Obata S, Akeda K, Imanishi T, Masuda K, Bae W, Morimoto R, et al. Effect of autologous platelet-rich plasma-releasate on intervertebral disc degeneration in the rabbit anular puncture model: a preclinical study. Arthritis Res Ther. (2012) 14:R241. doi: 10.1186/ar4084
77. Ma C, Wang R, Zhao D, Wang N, Han Y, Wang S, et al. Efficacy of platelet-rich plasma containing xenogenic adipose tissue-derived stromal cells on restoring intervertebral disc degeneration: a preclinical study in a rabbit model. Pain Res Manag. (2019) 2019:e6372356. doi: 10.1155/2019/6372356
78. Walsh AJL, Bradford DS, Lotz JC. In vivo growth factor treatment of degenerated intervertebral discs. Spine. (2004) 29:156–63. doi: 10.1097/01.BRS.0000107231.67854.9F
79. Zhu J, Xia K, Yu W, Wang Y, Hua J, Liu B, et al. Sustained release of GDF5 from a designed coacervate attenuates disc degeneration in a rat model. Acta Biomater. (2019) 86:300–11. doi: 10.1016/j.actbio.2019.01.028
80. Liang H, Ma S-Y, Feng G, Shen FH, Joshua Li X. Therapeutic effects of adenovirus-mediated growth and differentiation factor-5 in a mice disc degeneration model induced by annulus needle puncture. Spine J. (2010) 10:32–41. doi: 10.1016/j.spinee.2009.10.006
81. Matta A, Karim MZ, Gerami H, Benigno BZ, Cheng I, Mehrkens A, et al. A single injection of NTG-101 reduces the expression of pain-related neurotrophins in a canine model of degenerative disc disease. Int J Mol Sci. (2022) 23:5717. doi: 10.3390/ijms23105717
82. Bari E, Perteghella S, Di Silvestre D, Sorlini M, Catenacci L, Sorrenti M, et al. Pilot production of mesenchymal stem/stromal freeze-dried secretome for cell-free regenerative nanomedicine: a validated GMP-compliant process. Cells. (2018) 7:190. doi: 10.3390/cells7110190
83. Wen T, Wang H, Li Y, Lin Y, Zhao S, Liu J, et al. Bone mesenchymal stem cell-derived extracellular vesicles promote the repair of intervertebral disc degeneration by transferring microRNA-199a. Cell Cycle. (2021) 20:256–70. doi: 10.1080/15384101.2020.1863682
84. Zhu L, Shi Y, Liu L, Wang H, Shen P, Yang H. Mesenchymal stem cells-derived exosomes ameliorate nucleus pulposus cells apoptosis via delivering miR-142-3p: therapeutic potential for intervertebral disc degenerative diseases. Cell Cycle. (2020) 19:1727–39. doi: 10.1080/15384101.2020.1769301
85. Zhu G, Yang X, Peng C, Yu L, Hao Y. Exosomal miR-532–5p from bone marrow mesenchymal stem cells reduce intervertebral disc degeneration by targeting RASSF5. Exp Cell Res. (2020) 393:112109. doi: 10.1016/j.yexcr.2020.112109
86. Xia C, Zeng Z, Fang B, Tao M, Gu C, Zheng L, et al. Mesenchymal stem cell-derived exosomes ameliorate intervertebral disc degeneration via anti-oxidant and anti-inflammatory effects. Free Radic Biol Med. (2019) 143:1–15. doi: 10.1016/j.freeradbiomed.2019.07.026
87. Cheng X, Zhang G, Zhang L, Hu Y, Zhang K, Sun X, et al. Mesenchymal stem cells deliver exogenous miR-21 via exosomes to inhibit nucleus pulposus cell apoptosis and reduce intervertebral disc degeneration. J Cell Mol Med. (2018) 22:261–76. doi: 10.1111/jcmm.13316
88. Lu K, Li H-Y, Yang K, Wu J-L, Cai X-W, Zhou Y, et al. Exosomes as potential alternatives to stem cell therapy for intervertebral disc degeneration: in-vitro study on exosomes in interaction of nucleus pulposus cells and bone marrow mesenchymal stem cells. Stem Cell Res Ther. (2017) 8:108. doi: 10.1186/s13287-017-0563-9
89. Liao Z, Luo R, Li G, Song Y, Zhan S, Zhao K, et al. Exosomes from mesenchymal stem cells modulate endoplasmic reticulum stress to protect against nucleus pulposus cell death and ameliorate intervertebral disc degeneration in vivo. Theranostics. (2019) 9:4084–100. doi: 10.7150/thno.33638
90. Hingert D, Ekström K, Aldridge J, Crescitelli R, Brisby H. Extracellular vesicles from human mesenchymal stem cells expedite chondrogenesis in 3D human degenerative disc cell cultures. Stem Cell Res Ther. (2020) 11:323. doi: 10.1186/s13287-020-01832-2
91. Li M, Li R, Yang S, Yang D, Gao X, Sun J, et al. Exosomes derived from bone marrow mesenchymal stem cells prevent acidic pH-induced damage in human nucleus pulposus cells. Med Sci Monit Int Med J Exp Clin Res. (2020) 26:e922928-1-e922928-8. doi: 10.12659/MSM.922928
92. Li Z, Kong L, Liu C, Xu H-G. Human bone marrow mesenchymal stem cell-derived exosomes attenuate IL-1β-induced annulus fibrosus cell damage. Am J Med Sci. (2020) 360:693–700. doi: 10.1016/j.amjms.2020.07.025
93. Qi L, Wang R, Shi Q, Yuan M, Jin M, Li D. Umbilical cord mesenchymal stem cell conditioned medium restored the expression of collagen II and aggrecan in nucleus pulposus mesenchymal stem cells exposed to high glucose. J Bone Miner Metab. (2019) 37:455–66. doi: 10.1007/s00774-018-0953-9
94. Xiang H, Su W, Wu X, Chen W, Cong W, Yang S, et al. Exosomes derived from human urine-derived stem cells inhibit intervertebral disc degeneration by ameliorating endoplasmic reticulum stress. Oxid Med Cell Longev. (2020) 2020:e6697577. doi: 10.1155/2020/6697577
95. Xie L, Chen Z, Liu M, Huang W, Zou F, Ma X, et al. MSC-derived exosomes protect vertebral endplate chondrocytes against apoptosis and calcification via the miR-31-5p/ATF6 Axis. Mol Ther Nucleic Acids. (2020) 22:601–14. doi: 10.1016/j.omtn.2020.09.026
96. Zhang J, Zhang J, Zhang Y, Liu W, Ni W, Huang X, et al. Mesenchymal stem cells-derived exosomes ameliorate intervertebral disc degeneration through inhibiting pyroptosis. J Cell Mol Med. (2020) 24:11742–54. doi: 10.1111/jcmm.15784
97. Yuan Q, Wang X, Liu L, Cai Y, Zhao X, Ma H, et al. Exosomes derived from human placental mesenchymal stromal cells carrying antagomiR-4450 alleviate intervertebral disc degeneration through upregulation of ZNF121. Stem Cells Dev. (2020) 29:1038–58. doi: 10.1089/scd.2020.0083
98. Bach F, Libregts S, Creemers L, Meij B, Ito K, Wauben M, et al. Notochordal-cell derived extracellular vesicles exert regenerative effects on canine and human nucleus pulposus cells. Oncotarget. (2017) 8:88845–56. doi: 10.18632/oncotarget.21483
99. Bach FC, de Vries SA, Riemers FM, Boere J, van Heel FW, van Doeselaar M, et al. Soluble and pelletable factors in porcine, canine and human notochordal cell-conditioned medium: implications for IVD regeneration. Eur Cell Mater. (2016) 32:163–80. doi: 10.22203/eCM.v032a11
100. Sun Z, Liu B, Liu Z-H, Song W, Wang D, Chen B-Y, et al. Notochordal-cell-derived exosomes induced by compressive load inhibit angiogenesis via the miR-140-5p/Wnt/β-catenin axis. Mol Ther Nucleic Acids. (2020) 22:1092–106. doi: 10.1016/j.omtn.2020.10.021
101. Lan W, Pan S, Li H, Sun C, Chang X, Lu K, et al. Inhibition of the Notch1 pathway promotes the effects of nucleus pulposus cell-derived exosomes on the differentiation of mesenchymal stem cells into nucleus pulposus-like cells in rats. Stem Cells Int. (2019) 2019:8404168. doi: 10.1155/2019/8404168
102. Moen A, Jacobsen D, Phuyal S, Legfeldt A, Haugen F, Røe C, et al. MicroRNA-223 demonstrated experimentally in exosome-like vesicles is associated with decreased risk of persistent pain after lumbar disc herniation. J Transl Med. (2017) 15:89. doi: 10.1186/s12967-017-1194-8
103. Chen C, Chen J, Wang W, Xie L, Shao C, Zhang Y. Inhibition of the P53/P21 pathway attenuates the effects of senescent nucleus pulposus cell-derived exosomes on the senescence of nucleus pulposus cells. Orthop Surg. (2020) 13:583–91. doi: 10.1111/os.12886
104. Hu S-Q, Zhang Q-C, Meng Q-B, Hu A-N, Zou J-P, Li X-L. Autophagy regulates exosome secretion in rat nucleus pulposus cells via the RhoC/ROCK2 pathway. Exp Cell Res. (2020) 395:112239. doi: 10.1016/j.yexcr.2020.112239
105. Song J, Chen Z-H, Zheng C-J, Song K-H, Xu G-Y, Xu S, et al. Exosome-transported circRNA_0000253 competitively adsorbs microRNA-141-5p and increases IDD. Mol Ther Nucleic Acids. (2020) 21:1087–99. doi: 10.1016/j.omtn.2020.07.039
106. Tang S, Salazar-Puerta A, Richards J, Khan S, Hoyland JA, Gallego-Perez D, et al. Non-viral reprogramming of human nucleus pulposus cells with FOXF1 via extracellular vesicle delivery: an in vitro and in vivo study. Eur Cell Mater. (2021) 41:90–107. doi: 10.22203/eCM.v041a07
107. Sun Z, Zhao H, Liu B, Gao Y, Tang W-H, Liu Z-H, et al. AF cell derived exosomes regulate endothelial cell migration and inflammation: implications for vascularization in intervertebral disc degeneration. Life Sci. (2021) 265:118778. doi: 10.1016/j.lfs.2020.118778
108. Yuan F, Xu R, Ye J, Zhao M, Ren L, Li X. Apoptotic bodies from endplate chondrocytes enhance the oxidative stress-induced mineralization by regulating PPi metabolism. J Cell Mol Med. (2019) 23:3665–75. doi: 10.1111/jcmm.14268
109. Luo L, Jian X, Sun H, Qin J, Wang Y, Zhang J, et al. Cartilage endplate stem cells inhibit intervertebral disc degeneration by releasing exosomes to nucleus pulposus cells to activate Akt/autophagy. Stem Cells. (2021) 39:467–81. doi: 10.1002/stem.3322
110. Zhang Z, Wang C, Lin J, Jin H, Wang K, Yan Y, et al. Therapeutic potential of naringin for intervertebral disc degeneration: involvement of autophagy against oxidative stress-induced apoptosis in nucleus pulposus cells. Am J Chin Med. (2018) 46:1561–80. doi: 10.1142/S0192415X18500805
111. Silveira JW, Issy AC, Castania VA, Salmon CEG, Nogueira-Barbosa MH, Guimarães FS, et al. Protective effects of cannabidiol on lesion-induced intervertebral disc degeneration. PLoS ONE. (2014) 9:e113161. doi: 10.1371/journal.pone.0113161
112. Krupkova O, Sekiguchi M, Klasen J, Hausmann O, Konno S, Ferguson SJ, et al. Epigallocatechin 3-gallate suppresses interleukin-1β-induced inflammatory responses in intervertebral disc cells in vitro and reduces radiculopathic pain in rats. Eur Cell Mater. (2014) 28:372–86. doi: 10.22203/eCM.v028a26
113. Liu H, Kang H, Song C, Lei Z, Li L, Guo J, et al. Urolithin A inhibits the catabolic effect of TNFα on nucleus pulposus cell and alleviates intervertebral disc degeneration in vivo. Front Pharmacol. (2018) 9:e01043. doi: 10.3389/fphar.2018.01043
114. Jin L-Y, Lv Z-D, Wang K, Qian L, Song X-X, Li X-F, et al. Estradiol alleviates intervertebral disc degeneration through modulating the antioxidant enzymes and inhibiting autophagy in the model of menopause rats. Oxid Med Cell Longev. (2018) 2018:7890291. doi: 10.1155/2018/7890291
115. Liu S, Yang S-D, Huo X-W, Yang D-L, Ma L, Ding W-Y. 17β-Estradiol inhibits intervertebral disc degeneration by down-regulating MMP-3 and MMP-13 and up-regulating type II collagen in a rat model. Artif Cells Nanomed Biotechnol. (2018) 46:182–91. doi: 10.1080/21691401.2018.1453826
116. Hua W, Li S, Luo R, Wu X, Zhang Y, Liao Z, et al. Icariin protects human nucleus pulposus cells from hydrogen peroxide-induced mitochondria-mediated apoptosis by activating nuclear factor erythroid 2-related factor 2. Biochim. Biophys. Acta Mol Basis Dis. (2020) 1866:165575. doi: 10.1016/j.bbadis.2019.165575
117. Kwon Y-J. Resveratrol has anabolic effects on disc degeneration in a rabbit model. J Korean Med Sci. (2013) 28:939–45. doi: 10.3346/jkms.2013.28.6.939
118. Lin B, Yu H, He Y, Xu Y, Zhang W, Lu C, et al. Protective effects of resveratrol on autologous nucleus pulposus model of radiculopathy. Exp Ther Med. (2016) 12:3917–22. doi: 10.3892/etm.2016.3878
119. Willems N, Yang H, Langelaan MLP, Tellegen AR, Grinwis GCM, Kranenburg H-JC, et al. Biocompatibility and intradiscal application of a thermoreversible celecoxib-loaded poly-N-isopropylacrylamide MgFe-layered double hydroxide hydrogel in a canine model. Arthritis Res Ther. (2015) 17:214. doi: 10.1186/s13075-015-0727-x
120. Tellegen AR, Rudnik-Jansen I, Beukers M, Miranda-Bedate A, Bach FC, de Jong W, et al. Intradiscal delivery of celecoxib-loaded microspheres restores intervertebral disc integrity in a preclinical canine model. J Controlled Release. (2018) 286:439–50. doi: 10.1016/j.jconrel.2018.08.019
121. Luo R, Liao Z, Song Y, Yin H, Zhan S, Li G, et al. Berberine ameliorates oxidative stress-induced apoptosis by modulating ER stress and autophagy in human nucleus pulposus cells. Life Sci. (2019) 228:85–97. doi: 10.1016/j.lfs.2019.04.064
122. Chen D, Xia D, Pan Z, Xu D, Zhou Y, Wu Y, et al. Metformin protects against apoptosis and senescence in nucleus pulposus cells and ameliorates disc degeneration in vivo. Cell Death Dis. (2016) 7:e2441. doi: 10.1038/cddis.2016.334
123. Pan Z, Sun H, Xie B, Xia D, Zhang X, Yu D, et al. Therapeutic effects of gefitinib-encapsulated thermosensitive injectable hydrogel in intervertebral disc degeneration. Biomaterials. (2018) 160:56–68. doi: 10.1016/j.biomaterials.2018.01.016
124. Than KD, Rahman SU, Wang L, Khan A, Kyere KA, Than TT, et al. Intradiscal injection of simvastatin results in radiologic, histologic, and genetic evidence of disc regeneration in a rat model of degenerative disc disease. Spine J. (2014) 14:1017–28. doi: 10.1016/j.spinee.2013.11.034
125. Lin J, Chen J, Zhang Z, Xu T, Shao Z, Wang X, et al. Luteoloside inhibits IL-1β-induced apoptosis and catabolism in nucleus pulposus cells and ameliorates intervertebral disk degeneration. Front Pharmacol. (2019) 10:e00868. doi: 10.3389/fphar.2019.00868
126. Ma T, Guo C-J, Zhao X, Wu L, Sun S-X, Jin Q-H. The effect of curcumin on NF-κB expression in rat with lumbar intervertebral disc degeneration. Eur Rev Med Pharmacol Sci. (2015) 19:1305–14.
127. Liu X, Li K, Song J, Liang C, Wang X, Chen X. Efficient and stable gene expression in rabbit intervertebral disc cells transduced with a recombinant baculovirus vector. Spine. (2006) 31:732–5. doi: 10.1097/01.brs.0000206977.61305.43
128. Zhao Z, Li S, Huang H, Fang J, Wei H, Xi Y. In vivo delivery of MMP3-shRNA and Sox9 lentivirus cocktail enhances matrix synthesis to prevent lumbar disc degeneration. Adv Clin Exp Med. (2020) 29:639–47. doi: 10.17219/acem/121509
129. Wehling P, Schulitz KP, Robbins PD, Evans CH, Reinecke JA. Transfer of genes to chondrocytic cells of the lumbar spine. Proposal for a treatment strategy of spinal disorders by local gene therapy. Spine. (1997) 22:1092–7. doi: 10.1097/00007632-199705150-00008
130. Nishida K, Kang JD, Suh J-K, Robbins PD, Evans CH, Gilbertson LG. Adenovirus-mediated gene transfer to nucleus pulposus cells: implications for the treatment of intervertebral disc degeneration. Spine. (1998) 23:2437–42. doi: 10.1097/00007632-199811150-00016
131. Nishida K, Kang JD, Gilbertson LG, Moon SH, Suh JK, Vogt MT, et al. Modulation of the biologic activity of the rabbit intervertebral disc by gene therapy: an in vivo study of adenovirus-mediated transfer of the human transforming growth factor beta 1 encoding gene. Spine. (1999) 24:2419–25. doi: 10.1097/00007632-199912010-00002
132. Li H, Li W, Liang B, Wei J, Yin D, Fan Q. Role of AP-2α/TGF-β1/Smad3 axis in rats with intervertebral disc degeneration. Life Sci. (2020) 263:118567. doi: 10.1016/j.lfs.2020.118567
133. Bi F, Liu W, Wu Z, Ji C, Chang C. Antiaging factor klotho retards the progress of intervertebral disc degeneration through the toll-like receptor 4-NF-κB pathway. Int J Cell Biol. (2020) 2020304:8319516. doi: 10.1155/2020/8319516
134. Nishida K, Doita M, Takada T, Kakutani K, Miyamoto H, Shimomura T, et al. Sustained transgene expression in intervertebral disc cells in vivo mediated by microbubble-enhanced ultrasound gene therapy. Spine. (2006) 31:1415–9. doi: 10.1097/01.brs.0000219945.70675.dd
135. Huang Y, Huang L, Li L, Ge Z, Feng G, Liu L, et al. MicroRNA-25-3p therapy for intervertebral disc degeneration by targeting the IL-1β/ZIP8/MTF1 signaling pathway with a novel thermo-responsive vector. Ann Transl Med. (2020) 8:1500. doi: 10.21037/atm-20-6595
136. Cambria E, Brunner S, Heusser S, Fisch P, Hitzl W, Ferguson SJ, et al. Cell-laden agarose-collagen composite hydrogels for mechanotransduction studies. Front Bioeng Biotechnol. (2020) 8:e00346. doi: 10.3389/fbioe.2020.00346
137. Vedicherla S, Buckley CT. Cell-based therapies for intervertebral disc and cartilage regeneration- Current concepts, parallels, and perspectives. J Orthop Res. (2017) 35:8–22. doi: 10.1002/jor.23268
138. Lufkin L, Budišić M, Mondal S, Sur S. A Bayesian model to analyze the association of rheumatoid arthritis with risk factors and their interactions. Front Public Health. (2021) 9:e693830. doi: 10.3389/fpubh.2021.693830
139. Hemanta D, Jiang X-X, Feng Z-Z, Chen Z-X, Cao Y-W. Etiology for degenerative disc disease. Chin Med Sci J Chung-Kuo Hsueh Ko Hsueh Tsa Chih. (2016) 31:185–91. doi: 10.1016/S1001-9294(16)30049-9
140. Iatridis JC, Mente PL, Stokes IA, Aronsson DD, Alini M. Compression-induced changes in intervertebral disc properties in a rat tail model. Spine. (1999) 24:996–1002. doi: 10.1097/00007632-199905150-00013
141. Jay Lipson S, Muir H. Experimental intervertebral disc degeneration morphologic and proteoglycan changes over time. Arthritis Rheum. (1981) 24:12–21. doi: 10.1002/art.1780240103
142. Roh E, Darai A, Kyung J, Choi H, Kwon S, Bhujel B, et al. Genetic therapy for intervertebral disc degeneration. Int J Mol Sci. (2021) 22:1579. doi: 10.3390/ijms22041579
143. Virtanen IM, Karppinen J, Taimela S, Ott J, Barral S, Kaikkonen K, et al. Occupational and genetic risk factors associated with intervertebral disc disease. Spine. (2007) 32:1129–34. doi: 10.1097/01.brs.0000261473.03274.5c
144. Zielinska N, Podgórski M, Haładaj R, Polguj M, Olewnik Ł. Risk factors of intervertebral disc pathology—a point of view formerly and today—a review. J Clin Med. (2021) 10:409. doi: 10.3390/jcm10030409
145. Williams FMK, Popham M, Hart DJ, de Schepper E, Bierma-Zeinstra S, Hofman A, et al. GDF5 single-nucleotide polymorphism rs143383 is associated with lumbar disc degeneration in Northern European women. Arthritis Rheum. (2011) 63:708–12. doi: 10.1002/art.30169
146. Solovieva S, Noponen N, Männikkö M, Leino-Arjas P, Luoma K, Raininko R, et al. Association between the aggrecan gene variable number of tandem repeats polymorphism and intervertebral disc degeneration. Spine. (2007) 32:1700–5. doi: 10.1097/BRS.0b013e3180b9ed51
147. Bortsov AV, Parisien M, Khoury S, Zaykin DV, Martinsen AE, Lie MU, et al. Genome-Wide Analysis Identifies Significant Contribution of Brain-Expressed Genes in Chronic, But Not Acute, Back Pain (2020). doi: 10.1101/2020.09.04.20187575
148. Song Y-Q, Karasugi T, Cheung KMC, Chiba K, Ho DWH, Miyake A, et al. Lumbar disc degeneration is linked to a carbohydrate sulfotransferase 3 variant. J Clin Invest. (2013) 123:4909–17. doi: 10.1172/JCI69277
149. Williams FMK, Bansal AT, van Meurs JB, Bell JT, Meulenbelt I, Suri P, et al. Novel genetic variants associated with lumbar disc degeneration in northern Europeans: a meta-analysis of 4600 subjects. Ann Rheum Dis. (2013) 72:1141–8. doi: 10.1136/annrheumdis-2012-201551
150. Jiang H, Moro A, Liu Y, Wang J, Meng D, Zhan X, et al. Two GWAS-identified variants are associated with lumbar spinal stenosis and Gasdermin-C expression in Chinese population. Sci Rep. (2020) 10:21069. doi: 10.1038/s41598-020-78249-7
151. Näther P, Kersten JF, Kaden I, Irga K, Nienhaus A. Distribution patterns of degeneration of the lumbar spine in a cohort of 200 patients with an indication for lumbar MRI. Int J Environ Res Public Health. (2022) 19:3721. doi: 10.3390/ijerph19063721
152. Hanhivaara J, Määttä JH, Karppinen J, Niinimäki J, Nevalainen MT. The association of lumbosacral transitional vertebrae with low back pain and lumbar degenerative findings in MRI : a large cohort study. Spine. (2022) 47:153–62. doi: 10.1097/BRS.0000000000004244
153. Brauer C, Mikkelsen S, Pedersen EB, Møller KL, Simonsen EB, Koblauch H, et al. Occupational lifting predicts hospital admission due to low back pain in a cohort of airport baggage handlers. Int Arch Occup Environ Health. (2020) 93:111–22. doi: 10.1007/s00420-019-01470-z
154. Maurer E, Klinger C, Lorbeer R, Rathmann W, Peters A, Schlett CL, et al. Long-term effect of physical inactivity on thoracic and lumbar disc degeneration—an MRI-based analysis of 385 individuals from the general population. Spine J. (2020) 20:1386–96. doi: 10.1016/j.spinee.2020.04.016
155. Dragsbæk L, Kjaer P, Hancock M, Jensen TS. An exploratory study of different definitions and thresholds for lumbar disc degeneration assessed by MRI and their associations with low back pain using data from a cohort study of a general population. BMC Musculoskelet Disord. (2020) 21:253. doi: 10.1186/s12891-020-03268-4
156. Breen A, Breen A. Uneven intervertebral motion sharing is related to disc degeneration and is greater in patients with chronic, non-specific low back pain: an in vivo, cross-sectional cohort comparison of intervertebral dynamics using quantitative fluoroscopy. Eur Spine J. (2018) 27:145–53. doi: 10.1007/s00586-017-5155-y
157. van den Berg R, Jongbloed LM, Kuchuk NO, Roorda LD, Oostveen JCM, Koes BW, et al. The association between self-reported low back pain and radiographic lumbar disc degeneration of the cohort hip and cohort knee (CHECK) study. Spine. (2017) 42:1464–71. doi: 10.1097/BRS.0000000000002228
158. Jamaludin A, Kadir T, Zisserman A, McCall I, Williams FMK, Lang H, et al. Age and Disc Degeneration in Low Back Pain: Automated Analysis Enables a Magnetic Resonance Imaging Comparison of Large Cross-Sectional Cohorts of Symptomatic and Asymptomatic Subjects (2021). doi: 10.1101/2021.11.08.21265571
159. Saukkonen J, Määttä J, Oura P, Kyllönen E, Tervonen O, Niinimäki J, et al. Association between modic changes and low back pain in middle age: a Northern Finland birth cohort study. Spine. (2020) 45:1360. doi: 10.1097/BRS.0000000000003529
160. Mertimo T, Karppinen J, Niinimäki J, Blanco R, Määttä J, Kankaanpää M, et al. Association of lumbar disc degeneration with low back pain in middle age in the Northern Finland Birth Cohort 1966. BMC Musculoskelet Disord. (2022) 23:359. doi: 10.1186/s12891-022-05302-z
161. Takatalo J, Karppinen J, Taimela S, Niinimäki J, Laitinen J, Blanco Sequeiros R, et al. Body mass index is associated with lumbar disc degeneration in young Finnish males: subsample of Northern Finland birth cohort study 1986. BMC Musculoskelet Disord. (2013) 14:87. doi: 10.1186/1471-2474-14-87
162. Määttä JH, Kraatari M, Wolber L, Niinimäki J, Wadge S, Karppinen J, et al. Vertebral endplate change as a feature of intervertebral disc degeneration: a heritability study. Eur Spine J. (2014) 23:1856–62. doi: 10.1007/s00586-014-3333-8
163. Sebastine IM, Williams DJ. Current developments in tissue engineering of nucleus pulposus for the treatment of intervertebral disc degeneration. Annu. Int. Conf. IEEE Eng. Med. Biol. Soc. IEEE Eng. Med. Biol. Soc. Annu. Int. Conf. (2007) 2007:6401–6. doi: 10.1109/IEMBS.2007.4353821
164. Martirosyan NL, Patel AA, Carotenuto A, Kalani MYS, Belykh E, Walker CT, et al. Genetic alterations in intervertebral disc disease. Front Surg. (2016) 3:e00059. doi: 10.3389/fsurg.2016.00059
165. Urban JP, Roberts S. Degeneration of the intervertebral disc. Arthritis Res Ther. (2003) 5:120. doi: 10.1186/ar629
166. Pluijm SMF. Collagen type I 1 Sp1 polymorphism, osteoporosis, and intervertebral disc degeneration in older men and women. Ann Rheum Dis. (2004) 63:71–7. doi: 10.1136/ard.2002.002287
167. Tilkeridis C. Association of a COL1A1 polymorphism with lumbar disc disease in young military recruits. J Med Genet. (2005) 42:e44–e44. doi: 10.1136/jmg.2005.033225
168. Toktaş ZO, Ekşi MS, Yilmaz B, Demir MK, Özgen S, Kiliç T, et al. (2015). Association of collagen I, IX and vitamin D receptor gene polymorphisms with radiological severity of intervertebral disc degeneration in Southern European Ancestor. Eur Spine J. 24:2432–2441. doi: 10.1007/s00586-015-4206-5
169. Urban JPG, Winlove CP. Pathophysiology of the intervertebral disc and the challenges for MRI. J Magn Reson Imaging. (2007) 25:419–32. doi: 10.1002/jmri.20874
170. Kimura T, Nakata K, Tsumaki N, Miyamoto S, Matsui Y, Ebara S, et al. Progressive degeneration of articular cartilage and intervertebral discs. Int Orthop. (1996) 20:177–81. doi: 10.1007/s002640050058
171. Annunen S, Paassilta P, Lohiniva J, Perälä M, Pihlajamaa T, Karppinen J, et al. An allele of COL9A2 associated with intervertebral disc disease. Science. (1999) 285:409–12. doi: 10.1126/science.285.5426.409
172. Paassilta P, Lohiniva J, Göring HH, Perälä M, Räinä SS, Karppinen J, et al. Identification of a novel common genetic risk factor for lumbar disk disease. JAMA. (2001) 285:1843–9. doi: 10.1001/jama.285.14.1843
173. Dong DM, Yao M, Liu B, Sun CY, Jiang YQ, Wang YS. Association between the−1306C/T polymorphism of matrix metalloproteinase-2 gene and lumbar disc disease in Chinese young adults. Eur Spine J. (2007) 16:1958–61. doi: 10.1007/s00586-007-0454-3
174. Fiani B, Covarrubias C, Jarrah R. Genetic predictors of early-onset spinal intervertebral disc degeneration: part one of two. Cureus. (2021) 13:e15182. doi: 10.7759/cureus.15182
175. Hendry NGC. The hydration of the nucleus pulposus and its relation to intervertebral disc derangement. J Bone Joint Surg Br. (1958) 40-B:132–44. doi: 10.1302/0301-620X.40B1.132
176. Sun Z, Miao L, Zhang Y, Ming L. Association between the−1562 C/T polymorphism of matrix metalloproteinase-9 gene and lumbar disc disease in the young adult population in North China. Connect Tissue Res. (2009) 50:181–5. doi: 10.1080/03008200802585630
177. Takahashi M, Haro H, Wakabayashi Y, Kawa-uchi T, Komori H, Shinomiya K. The association of degeneration of the intervertebral disc with 5a/6a polymorphism in the promoter of the human matrix metalloproteinase-3 gene. J Bone Joint Surg Br. (2001) 83-B:491–5. doi: 10.1302/0301-620X.83B4.0830491
178. Zhang Y, Gu Z, Qiu G. Association of the polymorphism of MMP2 with the risk and severity of lumbar disc degeneration in the Chinese Han population. Eur Rev Med Pharmacol Sci. (2013) 17:1830–4.
179. Aripaka SS, Bech-Azeddine R, Jørgensen LM, Mikkelsen JD. The expression of metalloproteinases in the lumbar disc correlates strongly with Pfirrmann MRI grades in lumbar spinal fusion patients. Brain Spine. (2022) 2:100872. doi: 10.1016/j.bas.2022.100872
180. Basaran R, Senol M, Ozkanli S, Efendioglu M, Kaner T. Correlation of matrix metalloproteinase (MMP)-1,−2,−3, and –9 expressions with demographic and radiological features in primary lumbar intervertebral disc disease. J Clin Neurosci. (2017) 41:46–9. doi: 10.1016/j.jocn.2017.03.001
181. Liu S, Wu N, Liu J, Liu H, Su X, Liu Z, et al. Association between ADAMTS-4 gene polymorphism and lumbar disc degeneration in Chinese Han population: association between ADAMTS-4 gene polymorphism. J Orthop Res. (2016) 34:860–4. doi: 10.1002/jor.23081
182. Vo NV, Hartman RA, Yurube T, Jacobs LJ, Sowa GA, Kang JD. Expression and regulation of metalloproteinases and their inhibitors in intervertebral disc aging and degeneration. Spine J. (2013) 13:331–41. doi: 10.1016/j.spinee.2012.02.027
183. Eser B, Cora T, Eser O, Kalkan E, Haktanir A, Erdogan MO, et al. Association of the polymorphisms of vitamin D receptor and aggrecan genes with degenerative disc disease. Genet Test Mol Biomark. (2010) 14:313–7. doi: 10.1089/gtmb.2009.0202
184. Kawaguchi Y, Kanamori M, Ishihara H, Ohmori K, Matsui H, Kimura T. The association of lumbar disc disease with vitamin-D receptor gene polymorphism. J Bone Jt Surg-Am Vol. (2002) 84:2022–8. doi: 10.2106/00004623-200211000-00018
185. Mayer JE, Iatridis JC, Chan D, Qureshi SA, Gottesman O, Hecht AC. Genetic polymorphisms associated with intervertebral disc degeneration. Spine J. (2013) 13:299–317. doi: 10.1016/j.spinee.2013.01.041
186. Videman T, Leppävuori J, Kaprio J, Battié MC, Gibbons LE, Peltonen L, et al. 1998 Volvo award winner in basic science studies: intragenic polymorphisms of the vitamin D receptor gene associated with intervertebral disc degeneration. Spine. (1998) 23:2477–85. doi: 10.1097/00007632-199812010-00002
187. Vieira LA, De Marchi PL, dos Santos AA, Christofolini DM, Barbosa CP, Fonseca FLA, et al. Analysis of FokI polymorphism of vitamin D receptor gene in intervertebral disc degeneration. Genet Test Mol Biomark. (2014) 18:625–9. doi: 10.1089/gtmb.2014.0030
188. Zawilla NH, Darweesh H, Mansour N, Helal S, Taha FM, Awadallah M, et al. Matrix metalloproteinase-3, vitamin D receptor gene polymorphisms, and occupational risk factors in lumbar disc degeneration. J Occup Rehabil. (2014) 24:370–81. doi: 10.1007/s10926-013-9472-7
189. Feng Y, Egan B, Wang J. Genetic factors in intervertebral disc degeneration. Genes Dis. (2016) 3:178–85. doi: 10.1016/j.gendis.2016.04.005
190. Ou-Yang DC, Kleck CJ, Ackert-Bicknell CL. Genetics of intervertebral disc degeneration. Curr Osteoporos Rep. (2023) 21:56–64. doi: 10.1007/s11914-022-00769-0
191. Rodova M, Lu Q, Li Y, Woodbury BG, Crist JD, Gardner BM, et al. Nfat1 regulates adult articular chondrocyte function through its age-dependent expression mediated by epigenetic histone methylation. J Bone Miner Res Off J Am Soc Bone Miner Res. (2011) 26:1974–86. doi: 10.1002/jbmr.397
192. Shi S, Wang C, Acton AJ, Eckert GJ, Trippel SB. Role of sox9 in growth factor regulation of articular chondrocytes. J Cell Biochem. (2015) 116:1391–400. doi: 10.1002/jcb.25099
193. Yang F, Liu W, Huang Y, Yang S, Shao Z, Cai X, et al. Regulated cell death: Implications for intervertebral disc degeneration and therapy. J Orthop Transl. (2022) 37:163–72. doi: 10.1016/j.jot.2022.10.009
194. Calió M, Gantenbein B, Egli M, Poveda L, Ille F. The cellular composition of bovine coccygeal intervertebral discs: a comprehensive single-cell RNAseq analysis. Int J Mol Sci. (2021) 22:4917. doi: 10.3390/ijms22094917
195. Cherif H, Mannarino M, Pacis AS, Ragoussis J, Rabau O, Ouellet JA, et al. Single-cell RNA-seq analysis of cells from degenerating and non-degenerating intervertebral discs from the same individual reveals new biomarkers for intervertebral disc degeneration. Int J Mol Sci. (2022) 23:3993. doi: 10.3390/ijms23073993
196. Kraus P, Li K, Sipes D, Varden L, Yerden R, Henderson A, et al. Single-cell phenotyping of complex heterogeneous tissue. In:Santra TS, Tseng F-G, , editors. Handbook of Single Cell Technologies. Singapore: Springer (2019). p. 1–17.
197. Lufkin L, Samanta A, Baker D, Lufkin S, Schulze J, Ellis B, et al. Glis1 and oxaloacetate in nucleus pulposus stromal cell somatic reprogramming and survival. Front Mol Biosci. (2022) 9:e1009402. doi: 10.3389/fmolb.2022.1009402
198. Rohanifar M, Clayton SW, Easson GWD, Patil DS, Lee F, Jing L, et al. Single cell RNA-sequence analyses reveal uniquely expressed genes and heterogeneous immune cell involvement in the rat model of intervertebral disc degeneration. Appl Sci. (2022) 12:8244. doi: 10.3390/app12168244
199. Zhang D, Tang Z, Huang H, Zhou G, Cui C, Weng Y, et al. Metabolic regulation of gene expression by histone lactylation. Nature. (2019) 574:575–80. doi: 10.1038/s41586-019-1678-1
200. Kennon JC, Awad ME, Chutkan N, DeVine J, Fulzele S. Current insights on use of growth factors as therapy for Intervertebral Disc Degeneration. Biomol Concepts. (2018) 9:43–52. doi: 10.1515/bmc-2018-0003
201. Sun Z, Liu B, Luo Z-J. The immune privilege of the intervertebral disc: implications for intervertebral disc degeneration treatment. Int J Med Sci. (2020) 17:685–92. doi: 10.7150/ijms.42238
202. Brodin H. Paths of nutrition in articular cartilage and intervertebral discs. Acta Orthop Scand. (1954) 24:177–83. doi: 10.3109/17453675408988561
203. Holm S, Maroudas A, Urban JPG, Selstam G, Nachemson A. Nutrition of the intervertebral disc: solute transport and metabolism. Connect Tissue Res. (1981) 8:101–19. doi: 10.3109/03008208109152130
204. Kauppila LI. Prevalence of stenotic changes in arteries supplying the lumbar spine. A postmortem angiographic study on 140 subjects. Ann Rheum Dis. (1997) 56:591–5. doi: 10.1136/ard.56.10.591
205. Urban JPG, Smith S, Fairbank JCT. Nutrition of the intervertebral disc. Spine. (2004) 29:2700–9. doi: 10.1097/01.brs.0000146499.97948.52
206. Bartels EM, Fairbank JC, Winlove CP, Urban JP. Oxygen and lactate concentrations measured in vivo in the intervertebral discs of patients with scoliosis and back pain. Spine. (1998) 23:1–7; discussion 8. doi: 10.1097/00007632-199801010-00001
207. Lyu F-J. Impact of microenvironmental changes during degeneration on intervertebral disc progenitor cells: a comparison with mesenchymal stem cells. Bioengineering. (2022) 9:148. doi: 10.3390/bioengineering9040148
208. Malandrino A. Chapter 6 - intervertebral disc. In:Galbusera F, Wilke H-J, , editors. Biomechanics of the Spine. Academic Press (2018). p. 89–103.
209. Diamant B, Karlsson J, Nachemson A. Correlation between lactate levels and pH in discs of patients with lumbar rhizopathies. Experientia. (1968) 24:1195–6. doi: 10.1007/BF02146615
210. Gilbert HTJ, Hodson N, Baird P, Richardson SM, Hoyland JA. Acidic pH promotes intervertebral disc degeneration: acid-sensing ion channel-3 as a potential therapeutic target. Sci Rep. (2016) 6:37360. doi: 10.1038/srep37360
211. Kitano T, Zerwekh JE, Usui Y, Edwards ML, Flicker PL, Mooney V. Biochemical changes associated with the symptomatic human intervertebral disk. Clin Orthop. (1993) 372–7. doi: 10.1097/00003086-199308000-00050
212. Wuertz K, Godburn K, Iatridis JC. MSC response to pH levels found in degenerating intervertebral discs. Biochem Biophys Res Commun. (2009) 379:824–9. doi: 10.1016/j.bbrc.2008.12.145
213. Ishihara H, Urban JP. Effects of low oxygen concentrations and metabolic inhibitors on proteoglycan and protein synthesis rates in the intervertebral disc. J Orthop Res. (1999) 17:829–35. doi: 10.1002/jor.1100170607
214. Bibby SRS, Urban JPG. Effect of nutrient deprivation on the viability of intervertebral disc cells. Eur Spine J. (2004) 13:695–701. doi: 10.1007/s00586-003-0616-x
215. Dowdell J, Erwin M, Choma T, Vaccaro A, Iatridis J, Cho SK. Intervertebral disk degeneration and repair. Neurosurgery. (2017) 80:S46–54. doi: 10.1093/neuros/nyw078
216. Kurunlahti M, Tervonen O, Vanharanta H, Ilkko E, Suramo I. Association of atherosclerosis with low back pain and the degree of disc degeneration. Spine. (1999) 24:2080–4. doi: 10.1097/00007632-199910150-00003
217. Zehra U, Tryfonidou M, Iatridis JC, Illien-Jünger S, Mwale F, Samartzis D. Mechanisms and clinical implications of intervertebral disc calcification. Nat Rev Rheumatol. (2022) 18:352–62. doi: 10.1038/s41584-022-00783-7
218. Hui S, Ghergurovich JM, Morscher RJ, Jang C, Teng X, Lu W, et al. Glucose feeds the TCA cycle via circulating lactate. Nature. (2017) 551:115–8. doi: 10.1038/nature24057
219. Wang D, Hartman R, Han C, Zhou C, Couch B, Malkamaki M, et al. Lactate oxidative phosphorylation by annulus fibrosus cells: evidence for lactate-dependent metabolic symbiosis in intervertebral discs. Arthritis Res Ther. (2021) 23:145. doi: 10.1186/s13075-021-02501-2
220. Illien-Jünger S, Torre O, Kindschuh W, Chen X, Laudier D, Iatridis J. AGEs induce ectopic endochondral ossification in intervertebral discs. Eur Cell Mater. (2016) 32:257–70. doi: 10.22203/eCM.v032a17
221. Adams MA, Freeman BJ, Morrison HP, Nelson IW, Dolan P. Mechanical initiation of intervertebral disc degeneration. Spine. (2000) 25:1625–36. doi: 10.1097/00007632-200007010-00005
222. Kamali A, Ziadlou R, Lang G, Pfannkuche J, Cui S, Li Z, et al. Small molecule-based treatment approaches for intervertebral disc degeneration: current options and future directions. Theranostics. (2021) 11:27–47. doi: 10.7150/thno.48987
223. Horner HA, Urban JPG. 2001 Volvo award winner in basic science studies: effect of nutrient supply on the viability of cells from the nucleus pulposus of the intervertebral disc. Spine. (2001) 26:2543–9. doi: 10.1097/00007632-200112010-00006
224. Ohshima H, Urban JP. The effect of lactate and pH on proteoglycan and protein synthesis rates in the intervertebral disc. Spine. (1992) 17:1079–82. doi: 10.1097/00007632-199209000-00012
225. Jackson AR, Huang C-Y, Gu WY. Effect of endplate calcification and mechanical deformation on the distribution of glucose in intervertebral disc: a 3D finite element study. Comput Methods Biomech Biomed Engin. (2011) 14:195–204. doi: 10.1080/10255842.2010.535815
226. Mokhbi Soukane D, Shirazi-Adl A, Urban JPG. Investigation of solute concentrations in a 3D model of intervertebral disc. Eur Spine J. (2009) 18:254–62. doi: 10.1007/s00586-008-0822-7
227. Benneker LM, Heini PF, Alini M, Anderson SE, Ito K. 2004 Young Investigator Award Winner: vertebral endplate marrow contact channel occlusions and intervertebral disc degeneration. Spine. (2005) 30:167–73. doi: 10.1097/01.brs.0000150833.93248.09
228. Peng Y, Lv F-J. Symptomatic versus asymptomatic intervertebral disc degeneration: is inflammation the key? Crit Rev Eukaryot Gene Expr. (2015) 25:13–21. doi: 10.1615/CritRevEukaryotGeneExpr.2015012369
229. Richardson SM, Doyle P, Minogue BM, Gnanalingham K, Hoyland JA. Increased expression of matrix metalloproteinase-10, nerve growth factor and substance P in the painful degenerate intervertebral disc. Arthritis Res Ther. (2009) 11:R126. doi: 10.1186/ar2793
230. Lv F-J, Peng Y, Lim FL, Sun Y, Lv M, Zhou L, et al. Matrix metalloproteinase 12 is an indicator of intervertebral disc degeneration co-expressed with fibrotic markers. Osteoarthritis Cartilage. (2016) 24:1826–36. doi: 10.1016/j.joca.2016.05.012
231. Liu C, Liang G, Deng Z, Tan J, Zheng Q, Lyu F-J. The upregulation of COX2 in human degenerated nucleus pulposus: the association of inflammation with intervertebral disc degeneration. Mediators Inflamm. (2021) 2021:e2933199. doi: 10.1155/2021/2933199
232. Igarashi T, Kikuchi S, Shubayev V, Myers RR. 2000 Volvo Award winner in basic science studies: exogenous tumor necrosis factor-alpha mimics nucleus pulposus-induced neuropathology. Molecular, histologic, and behavioral comparisons in rats. Spine. (2000) 25:2975–80. doi: 10.1097/00007632-200012010-00003
233. Ahn S-H, Cho Y-W, Ahn M-W, Jang S-H, Sohn Y-K, Kim H-S. mRNA expression of cytokines and chemokines in herniated lumbar intervertebral discs. Spine. (2002) 27:911–7. doi: 10.1097/00007632-200205010-00005
234. Shamji MF, Setton LA, Jarvis W, So S, Chen J, Jing L, et al. Proinflammatory cytokine expression profile in degenerated and herniated human intervertebral disc tissues. Arthritis Rheum. (2010) 62:1974–82. doi: 10.1002/art.27444
235. Lyu F-J, Cui H, Pan H, MC, Cheung K, Cao X, et al. Painful intervertebral disc degeneration and inflammation: from laboratory evidence to clinical interventions. Bone Res. (2021) 9:7. doi: 10.1038/s41413-020-00125-x
236. Allan DB, Waddell G. An historical perspective on low back pain and disability. Acta Orthop Scand. (1989) 60:1–23. doi: 10.3109/17453678909153916
237. Puustjärvi K, Lammi M, Helminen H, Inkinen R, Tammi M. Proteoglycans in the intervertebral disc of young dogs following strenuous running exercise. Connect Tissue Res. (1994) 30:225–40. doi: 10.3109/03008209409061974
238. Swärd L, Hellström M, Jacobsson B, Nyman R, Peterson L. Disc degeneration and associated abnormalities of the spine in elite gymnasts. A magnetic resonance imaging study. Spine. (1991) 16:437–43. doi: 10.1097/00007632-199104000-00009
239. Kaneoka K, Shimizu K, Hangai M, Okuwaki T, Mamizuka N, Sakane M, et al. Lumbar intervertebral disk degeneration in elite competitive swimmers: a case control study. Am J Sports Med. (2007) 35:1341–5. doi: 10.1177/0363546507300259
240. Dieleman JL, Baral R, Birger M, Bui AL, Bulchis A, Chapin A, et al. US spending on personal health care and public health, 1996-2013. JAMA. (2016) 316:2627. doi: 10.1001/jama.2016.16885
241. Divo MJ, Martinez CH, Mannino DM. Ageing and the epidemiology of multimorbidity. Eur Respir J. (2014) 44:1055–68. doi: 10.1183/09031936.00059814
242. Hangai M, Kaneoka K, Kuno S, Hinotsu S, Sakane M, Mamizuka N, et al. Factors associated with lumbar intervertebral disc degeneration in the elderly. Spine J. (2008) 8:732–40. doi: 10.1016/j.spinee.2007.07.392
243. Samartzis D, Bow C, Karppinen J, Luk KDK, Cheung BMY, Cheung KMC. Hypertension is independently associated with lumbar disc degeneration: a large-scale population-based study. Glob Spine J. (2014) 4:s-0034–1376579. doi: 10.1055/s-0034-1376579
244. Teraguchi M, Yoshimura N, Hashizume H, Yamada H, Oka H, Minamide A, et al. Progression, incidence, and risk factors for intervertebral disc degeneration in a longitudinal population-based cohort: the Wakayama Spine Study. Osteoarthritis Cartilage. (2017) 25:1122–31. doi: 10.1016/j.joca.2017.01.001
245. Berman D, Oren JH, Bendo J, Spivak J. The effect of smoking on spinal fusion. Int J Spine Surg. (2017) 11:29. doi: 10.14444/4029
246. Hadley MN, Reddy SV. Smoking and the human vertebral column: a review of the impact of cigarette use on vertebral bone metabolism and spinal fusion. Neurosurgery. (1997) 41:116–24. doi: 10.1097/00006123-199707000-00025
247. Jackson KL, Devine JG. The effects of smoking and smoking cessation on spine surgery: a systematic review of the literature. Glob Spine J. (2016) 6:695–701. doi: 10.1055/s-0036-1571285
248. Khurana VG. Adverse impact of smoking on the spine and spinal surgery. Surg Neurol Int. (2021) 12:118. doi: 10.25259/SNI_6_2021
249. Andersen SB, Smith EC, Støttrup C, Carreon LY, Andersen MO. Smoking is an independent risk factor of reoperation due to recurrent lumbar disc herniation. Glob Spine J. (2018) 8:378–81. doi: 10.1177/2192568217730352
250. Glassman SD, Anagnost SC, Parker A, Burke D, Johnson JR, Dimar JR. The effect of cigarette smoking and smoking cessation on spinal fusion. Spine. (2000) 25:2608–15. doi: 10.1097/00007632-200010150-00011
251. Hilibrand AS, Fye MA, Emery SE, Palumbo MA, Bohlman HH. Impact of smoking on the outcome of anterior cervical arthrodesis with interbody or strut-grafting. J Bone Joint Surg Am. (2001) 83:668–73. doi: 10.2106/00004623-200105000-00004
252. Lau D, Chou D, Ziewacz JE, Mummaneni PV. The effects of smoking on perioperative outcomes and pseudarthrosis following anterior cervical corpectomy: clinical article. J Neurosurg Spine. (2014) 21:547–58. doi: 10.3171/2014.6.SPINE13762
253. Akmal M, Kesani A, Anand B, Singh A, Wiseman M, Goodship A. Effect of nicotine on spinal disc cells: a cellular mechanism for disc degeneration. Spine. (2004) 29:568–75. doi: 10.1097/01.BRS.0000101422.36419.D8
254. Baucher G, Taskovic J, Troude L, Molliqaj G, Nouri A, Tessitore E. Risk factors for the development of degenerative cervical myelopathy: a review of the literature. Neurosurg Rev. (2022) 45:1675–89. doi: 10.1007/s10143-021-01698-9
255. Elmasry S, Asfour S, de Rivero Vaccari JP, Travascio F. Effects of tobacco smoking on the degeneration of the intervertebral disc: a finite element study. PLoS ONE. (2015) 10:e0136137. doi: 10.1371/journal.pone.0136137
256. Nasto LA, Ngo K, Leme A, Robinson AR, Dong Q, Roughley P, et al. Investigating the role of DNA damage in tobacco smoking-induced spine degeneration. Spine J. (2014) 14:416–23. doi: 10.1016/j.spinee.2013.08.034
257. Rajesh N, Moudgil-Joshi J, Kaliaperumal C. Smoking and degenerative spinal disease: a systematic review. Brain Spine. (2022) 2:100916. doi: 10.1016/j.bas.2022.100916
258. Yin L, Morita A, Tsuji T. Alterations of extracellular matrix induced by tobacco smoke extract. Arch Dermatol Res. (2000) 292:188–94. doi: 10.1007/s004030050476
259. Ambrose JA, Barua RS. The pathophysiology of cigarette smoking and cardiovascular disease: an update. J Am Coll Cardiol. (2004) 43:1731–7. doi: 10.1016/j.jacc.2003.12.047
260. Ernst E. Smoking, a cause of back trouble? Rheumatology. (1993) 32:239–42. doi: 10.1093/rheumatology/32.3.239
261. Sørensen LT. Wound healing and infection in surgery: the pathophysiological impact of smoking, smoking cessation, and nicotine replacement therapy: a systematic review. Ann Surg. (2012) 255:1069–79. doi: 10.1097/SLA.0b013e31824f632d
262. Battié MC, Videman T, Gill K, Moneta GB, Nyman R, Kaprio J, et al. 1991 Volvo Award in clinical sciences. Smoking and lumbar intervertebral disc degeneration: an MRI study of identical twins. Spine. (1991) 16:1015–21. doi: 10.1097/00007632-199109000-00001
263. Huang W, Qian Y, Zheng K, Yu L, Yu X. Is smoking a risk factor for lumbar disc herniation? Eur Spine J. (2016) 25:168–76. doi: 10.1007/s00586-015-4103-y
264. Leboeuf-Yde C. Smoking and low back pain. A systematic literature review of 41 journal articles reporting 47 epidemiologic studies. Spine. (1999) 24:1463–70. doi: 10.1097/00007632-199907150-00012
265. Schumann B, Bolm-Audorff U, Bergmann A, Ellegast R, Elsner G, Grifka J, et al. Lifestyle factors and lumbar disc disease: results of a German multi-center case-control study (EPILIFT). Arthritis Res Ther. (2010) 12:R193. doi: 10.1186/ar3164
266. Amin RM, Andrade NS, Neuman BJ. Lumbar Disc Herniation. Curr Rev Musculoskelet Med. (2017) 10:507–16. doi: 10.1007/s12178-017-9441-4
267. Paul CPL, de Graaf M, Bisschop A, Holewijn RM, van de Ven PM, van Royen BJ, et al. Static axial overloading primes lumbar caprine intervertebral discs for posterior herniation. PLoS ONE. (2017) 12:e0174278. doi: 10.1371/journal.pone.0174278
268. Fiol AG. Disc herniation. In:Mankowitz SKW, , editor. Consults in Obstetric Anesthesiology. Cham: Springer International Publishing (2018). p. 165–7.
269. Gruber HE, Ingram JA, Norton HJ, Hanley EN. Senescence in cells of the aging and degenerating intervertebral disc: immunolocalization of senescence-associated beta-galactosidase in human and sand rat discs. Spine. (2007) 32:321–7. doi: 10.1097/01.brs.0000253960.57051.de
270. Roberts S, Evans EH, Kletsas D, Jaffray DC, Eisenstein SM. Senescence in human intervertebral discs. Eur Spine J. (2006) 15(Suppl. 3):S312–6. doi: 10.1007/s00586-006-0126-8
271. Wu Q, Huang JH. Intervertebral disc aging, degeneration, and associated potential molecular mechanisms. J Head Neck Spine Surg. (2017) 1:555569. doi: 10.19080/jhnss.2017.01.555569
272. Kumari R, Jat P. Mechanisms of cellular senescence: cell cycle arrest and senescence associated secretory phenotype. Front Cell Dev Biol. (2021) 9:e645593. doi: 10.3389/fcell.2021.645593
273. Knutsson B, Sandén B, Sjödén G, Järvholm B, Michaëlsson K. Body mass index and risk for clinical lumbar spinal stenosis: a cohort study. Spine. (2015) 40:1451–6. doi: 10.1097/BRS.0000000000001038
274. Onyemaechi NO, Anyanwu GE, Obikili EN, Onwuasoigwe O, Nwankwo OE. Impact of overweight and obesity on the musculoskeletal system using lumbosacral angles. Patient Prefer Adherence. (2016) 10:291–6. doi: 10.2147/PPA.S90967
275. Bertheloot D, Latz E, Franklin BS. Necroptosis, pyroptosis and apoptosis: an intricate game of cell death. Cell Mol Immunol. (2021) 18:1106–21. doi: 10.1038/s41423-020-00630-3
276. Tang D, Kang R, Berghe TV, Vandenabeele P, Kroemer G. The molecular machinery of regulated cell death. Cell Res. (2019) 29:347–64. doi: 10.1038/s41422-019-0164-5
277. Kadow T, Sowa G, Vo N, Kang JD. Molecular basis of intervertebral disc degeneration and herniations: what are the important translational questions? Clin Orthop. (2015) 473:1903–12. doi: 10.1007/s11999-014-3774-8
278. Risbud MV, Shapiro IM. Role of cytokines in intervertebral disc degeneration: pain and disc content. Nat Rev Rheumatol. (2014) 10:44–56. doi: 10.1038/nrrheum.2013.160
279. Blanquer SBG, Grijpma DW, Poot AA. Delivery systems for the treatment of degenerated intervertebral discs. Adv Drug Deliv Rev. (2015) 84:172–87. doi: 10.1016/j.addr.2014.10.024
280. Sakai D, Schol J, Watanabe M. Clinical development of regenerative medicine targeted for intervertebral disc disease. Medicina. (2022) 58:267. doi: 10.3390/medicina58020267
281. Vadalà G, Ambrosio L, Russo F, Papalia R, Denaro V. Interaction between mesenchymal stem cells and intervertebral disc microenvironment: from cell therapy to tissue engineering. Stem Cells Int. (2019) 2019:e2376172. doi: 10.1155/2019/2376172
282. Clouet J, Fusellier M, Camus A, Le Visage C, Guicheux J. Intervertebral disc regeneration: from cell therapy to the development of novel bioinspired endogenous repair strategies. Adv Drug Deliv Rev. (2019) 146:306–24. doi: 10.1016/j.addr.2018.04.017
283. Kraus P, Lufkin T. Implications for a stem cell regenerative medicine based approach to human intervertebral disk degeneration. Front Cell Dev Biol. (2017) 5:e00017. doi: 10.3389/fcell.2017.00017
284. Hu B, He R, Ma K, Wang Z, Cui M, Hu H, et al. Intervertebral disc-derived stem/progenitor cells as a promising cell source for intervertebral disc regeneration. Stem Cells Int. (2018) 2018:e7412304. doi: 10.1155/2018/7412304
285. Kraus P, Samanta A, Lufkin S, Lufkin T. Stem cells in intervertebral disc regeneration-more talk than action? Biocell. (2021) 46:893–8. doi: 10.32604/biocell.2022.018432
286. Loebel C, Burdick JA. Engineering stem and stromal cell therapies for musculoskeletal tissue repair. Cell Stem Cell. (2018) 22:325–39. doi: 10.1016/j.stem.2018.01.014
287. Petaroudi M, Rodrigo-Navarro A, Dobre O, Dalby MJ, Salmeron-Sanchez M. Living biomaterials to engineer hematopoietic stem cell niches. Adv Healthc Mater. (2022) 11:2200964. doi: 10.1002/adhm.202200964
288. Richardson SM, Hoyland JA, Mobasheri R, Csaki C, Shakibaei M, Mobasheri A. Mesenchymal stem cells in regenerative medicine: opportunities and challenges for articular cartilage and intervertebral disc tissue engineering. J Cell Physiol. (2010) 222:23–32. doi: 10.1002/jcp.21915
289. Sakai D, Grad S. Advancing the cellular and molecular therapy for intervertebral disc disease. Adv Drug Deliv Rev. (2015) 84:159–71. doi: 10.1016/j.addr.2014.06.009
290. Wuertz K, Godburn K, Neidlinger-Wilke C, Urban J, Iatridis JC. Behavior of mesenchymal stem cells in the chemical microenvironment of the intervertebral disc. Spine. (2008) 33:1843. doi: 10.1097/BRS.0b013e31817b8f53
291. Jopling C, Boue S, Izpisua Belmonte JC. Dedifferentiation, transdifferentiation and reprogramming: three routes to regeneration. Nat Rev Mol Cell Biol. (2011) 12:79–89. doi: 10.1038/nrm3043
292. Kamao H, Mandai M, Okamoto S, Sakai N, Suga A, Sugita S, et al. Characterization of human induced pluripotent stem cell-derived retinal pigment epithelium cell sheets aiming for clinical application. Stem Cell Rep. (2014) 2:205–18. doi: 10.1016/j.stemcr.2013.12.007
293. Wang H, Yang Y, Liu J, Qian L. Direct cell reprogramming: approaches, mechanisms and progress. Nat Rev Mol Cell Biol. (2021) 22:410–24. doi: 10.1038/s41580-021-00335-z
294. Xia K, Gong Z, Zhu J, Yu W, Wang Y, Wang J, et al. Differentiation of pluripotent stem cells into nucleus pulposus progenitor cells for intervertebral disc regeneration. Curr Stem Cell Res Ther. (2019) 14:57–64. doi: 10.2174/1574888X13666180918095121
295. Akker GGH, van den Surtel DAM, Cremers A, Richardson SM, Hoyland JA, Rhijn LW, et al. Novel immortal cell lines support cellular heterogeneity in the human annulus fibrosus. PLoS ONE. (2016) 11:e0144497. doi: 10.1371/journal.pone.0144497
296. Henriksson HB, Thornemo M, Karlsson C, Hägg O, Junevik K, Lindahl A, et al. Identification of cell proliferation zones, progenitor cells and a potential stem cell niche in the intervertebral disc region: a study in four species. Spine. (2009) 34:2278. doi: 10.1097/BRS.0b013e3181a95ad2
297. Risbud MV, Schoepflin ZR, Mwale F, Kandel RA, Grad S, Iatridis JC, et al. Defining the phenotype of young healthy nucleus pulposus cells: recommendations of the Spine Research Interest Group at the 2014 annual ORS meeting. J Orthop Res. (2015) 33:283–93. doi: 10.1002/jor.22789
298. Risbud MV, Guttapalli A, Tsai T-T, Lee JY, Danielson KG, Vaccaro AR, et al. Evidence for skeletal progenitor cells in the degenerate human intervertebral disc. Spine. (2007) 32:2537–44. doi: 10.1097/BRS.0b013e318158dea6
299. Thorpe AA, Bach FC, Tryfonidou MA, Le Maitre CL, Mwale F, Diwan AD, et al. Leaping the hurdles in developing regenerative treatments for the intervertebral disc from preclinical to clinical. JOR Spine. (2018) 1:e1027. doi: 10.1002/jsp2.1027
300. Law S, Chaudhuri S. Mesenchymal stem cell and regenerative medicine: regeneration versus immunomodulatory challenges. Am J Stem Cells. (2013) 2:22–38.
301. Rodriguez A-M, Elabd C, Amri E-Z, Ailhaud G, Dani C. The human adipose tissue is a source of multipotent stem cells. Biochimie. (2005) 87:125–8. doi: 10.1016/j.biochi.2004.11.007
302. Rodriguez A-M, Elabd C, Delteil F, Astier J, Vernochet C, Saint-Marc P, et al. Adipocyte differentiation of multipotent cells established from human adipose tissue. Biochem Biophys Res Commun. (2004) 315:255–63. doi: 10.1016/j.bbrc.2004.01.053
303. Liang C-Z, Li H, Tao Y-Q, Zhou X-P, Yang Z-R, Li F-C, et al. The relationship between low pH in intervertebral discs and low back pain: a systematic review. Arch Med Sci AMS. (2012) 8:952–6. doi: 10.5114/aoms.2012.32401
304. Urban JP, Holm S, Maroudas A, Nachemson A. Nutrition of the intervertebral disk. An in vivo study of solute transport. Clin Orthop. (1977) 101–14. doi: 10.1097/00003086-197711000-00012
305. Schol J, Sakai D. Comprehensive narrative review on the analysis of outcomes from cell transplantation clinical trials for discogenic low back pain. North Am Spine Soc J NASSJ. (2023) 13:100195. doi: 10.1016/j.xnsj.2022.100195
306. Tschugg A, Diepers M, Simone S, Michnacs F, Quirbach S, Strowitzki M, et al. A prospective randomized multicenter phase I/II clinical trial to evaluate safety and efficacy of NOVOCART disk plus autologous disk chondrocyte transplantation in the treatment of nucleotomized and degenerative lumbar disks to avoid secondary disease: safety results of Phase I-a short report. Neurosurg Rev. (2017) 40:155–62. doi: 10.1007/s10143-016-0781-0
307. Camussi G, Deregibus MC, Bruno S, Cantaluppi V, Biancone L. Exosomes/microvesicles as a mechanism of cell-to-cell communication. Kidney Int. (2010) 78:838–48. doi: 10.1038/ki.2010.278
308. Kalluri R, LeBleu VS. The biology, function, and biomedical applications of exosomes. Science. (2020) 367:eaau6977. doi: 10.1126/science.aau6977
309. Kowal J, Arras G, Colombo M, Jouve M, Morath JP, Primdal-Bengtson B, et al. Proteomic comparison defines novel markers to characterize heterogeneous populations of extracellular vesicle subtypes. Proc Natl Acad Sci U S A. (2016) 113:E968–977. doi: 10.1073/pnas.1521230113
310. Ruivo CF, Adem B, Silva M, Melo SA. The biology of cancer exosomes: insights and new perspectives. Cancer Res. (2017) 77:6480–8. doi: 10.1158/0008-5472.CAN-17-0994
311. Chen Y, Tang Y, Fan G-C, Duan DD. Extracellular vesicles as novel biomarkers and pharmaceutic targets of diseases. Acta Pharmacol Sin. (2018) 39:499–500. doi: 10.1038/aps.2018.15
312. Di Bella MA. Overview and update on extracellular vesicles: considerations on exosomes and their application in modern medicine. Biology. (2022) 11:804. doi: 10.3390/biology11060804
313. Nazimek K, Bryniarski K, Santocki M, Ptak W. Exosomes as mediators of intercellular communication: clinical implications. Pol Arch Med Wewn. (2015) 125:370–80. doi: 10.20452/pamw.2840
314. Gebraad A. Tissue Engineering Approaches for the Treatment of Degenerated Intervertebral Discs. Helsinki: University of Helsinki. (2018).
315. Khan AN, Jacobsen HE, Khan J, Filippi CG, Levine M, Lehman RA, et al. Inflammatory biomarkers of low back pain and disc degeneration: a review. Ann N Y Acad Sci. (2017) 1410:68–84. doi: 10.1111/nyas.13551
316. Li C, Zhao Z, Zhou J, Liu Y, Wang H, Zhao X. Relationship between the TERT, TNIP1 and OBFC1 genetic polymorphisms and susceptibility to colorectal cancer in Chinese Han population. Oncotarget. (2017) 8:56932–41. doi: 10.18632/oncotarget.18378
317. Yuan X, Li T, Shi L, Miao J, Guo Y, Chen Y. Human umbilical cord mesenchymal stem cells deliver exogenous miR-26a-5p via exosomes to inhibit nucleus pulposus cell pyroptosis through METTL14/NLRP3. Mol Med. (2021) 27:91. doi: 10.1186/s10020-021-00355-7
318. Chao-yang G, Peng C, Hai-hong Z. Roles of NLRP3 inflammasome in intervertebral disc degeneration. Osteoarthritis Cartilage. (2021) 29:793–801. doi: 10.1016/j.joca.2021.02.204
319. Xu J, Xie G, Yang W, Wang W, Zuo Z, Wang W. Platelet-rich plasma attenuates intervertebral disc degeneration via delivering miR-141-3p-containing exosomes. Cell Cycle. (2021) 20:1487–99. doi: 10.1080/15384101.2021.1949839
320. DiStefano TJ, Vaso K, Danias G, Chionuma HN, Weiser JR, Iatridis JC. Extracellular vesicles as an emerging treatment option for intervertebral disc degeneration: therapeutic potential, translational pathways, and regulatory considerations. Adv Healthc Mater. (2022) 11:2100596. doi: 10.1002/adhm.202100596
321. Brown BN, Badylak SF. Extracellular matrix as an inductive scaffold for functional tissue reconstruction. Transl Res J Lab Clin Med. (2014) 163:268–85. doi: 10.1016/j.trsl.2013.11.003
322. Qu H, Fu H, Han Z, Sun Y. Biomaterials for bone tissue engineering scaffolds: a review. RSC Adv. (2019) 9:26252–62. doi: 10.1039/C9ra05214C
323. Turnbull G, Clarke J, Picard F, Riches P, Jia L, Han F, et al. 3D bioactive composite scaffolds for bone tissue engineering. Bioact Mater. (2018) 3:278–314. doi: 10.1016/j.bioactmat.2017.10.001
324. Schutgens E, Tryfonidou M, Smit T, Öner F, Krouwels A, et al. Biomaterials for intervertebral disc regeneration: past performance and possible future strategies. Eur Cell Mater. (2015) 30:210–31. doi: 10.22203/eCM.v030a15
325. Du L, Zhu M, Yang Q, Zhang J, Ma X, Kong D, et al. A novel integrated biphasic silk fibroin scaffold for intervertebral disc tissue engineering. Mater Lett. (2014) 117:237–40. doi: 10.1016/j.matlet.2013.12.029
326. Kim SH, Yoon SJ, Choi B, Ha HJ, Rhee JM, Kim MS, et al. Evaluation of various types of scaffold for tissue engineered intervertebral disc. Adv Exp Med Biol. (2006) 585:167–81. doi: 10.1007/978-0-387-34133-0_12
327. Nazarov R, Jin H-J, Kaplan DL. Porous 3-D scaffolds from regenerated silk fibroin. Biomacromolecules. (2004) 5:718–26. doi: 10.1021/bm034327e
328. Vernengo AJ, Grad S, Eglin D, Alini M, Li Z. Bioprinting tissue analogues with decellularized extracellular matrix bioink for regeneration and tissue models of cartilage and intervertebral discs. Adv Funct Mater. (2020) 30:1909044. doi: 10.1002/adfm.201909044
329. Sarker B, Hum J, Nazhat SN, Boccaccini AR. Combining collagen and bioactive glasses for bone tissue engineering: a review. Adv Healthc Mater. (2015) 4:176–94. doi: 10.1002/adhm.201400302
330. Lee K-I, Moon S-H, Kim H, Kwon U-H, Kim H-J, Park S-N, et al. Tissue engineering of the intervertebral disc with cultured nucleus pulposus cells using atelocollagen scaffold and growth factors. Spine. (2012) 37:452–8. doi: 10.1097/BRS.0b013e31823c8603
331. Itoh H, Aso Y, Furuse M, Noishiki Y, Miyata T. A honeycomb collagen carrier for cell culture as a tissue engineering scaffold. Artif Organs. (2001) 25:213–7. doi: 10.1046/j.1525-1594.2001.025003213.x
332. Liu B, Song Y, Jin L, Wang Z, Pu D, Lin S, et al. Silk structure and degradation. Colloids Surf B Biointerfaces. (2015) 131:122–8. doi: 10.1016/j.colsurfb.2015.04.040
333. Park S-H, Gil ES, Mandal BB, Cho H, Kluge JA, Min B-H, et al. Annulus fibrosus tissue engineering using lamellar silk scaffolds: annulus fibrosis regeneration. J Tissue Eng Regen Med. (2012) 6:s24–33. doi: 10.1002/term.541
334. Horan RL, Antle K, Collette AL, Wang Y, Huang J, Moreau JE, et al. In vitro degradation of silk fibroin. Biomaterials. (2005) 26:3385–93. doi: 10.1016/j.biomaterials.2004.09.020
335. Chang G, Kim H-J, Kaplan D, Vunjak-Novakovic G, Kandel RA. Porous silk scaffolds can be used for tissue engineering annulus fibrosus. Eur Spine J. (2007) 16:1848–57. doi: 10.1007/s00586-007-0364-4
336. Stergar J, Gradisnik L, Velnar T, Maver U. Intervertebral disc tissue engineering: a brief review. Bosn J Basic Med Sci. (2019) 19:130–7. doi: 10.17305/bjbms.2019.3778
337. Bhattarai N, Edmondson D, Veiseh O, Matsen FA, Zhang M. Electrospun chitosan-based nanofibers and their cellular compatibility. Biomaterials. (2005) 26:6176–84. doi: 10.1016/j.biomaterials.2005.03.027
338. Jayakumar R, Prabaharan M, Nair SV, Tamura H. Novel chitin and chitosan nanofibers in biomedical applications. Biotechnol Adv. (2010) 28:142–50. doi: 10.1016/j.biotechadv.2009.11.001
339. Kim I-Y, Seo S-J, Moon H-S, Yoo M-K, Park I-Y, Kim B-C, et al. Chitosan and its derivatives for tissue engineering applications. Biotechnol Adv. (2008) 26:1–21. doi: 10.1016/j.biotechadv.2007.07.009
340. Lee KY, Jeong L, Kang YO, Lee SJ, Park WH. Electrospinning of polysaccharides for regenerative medicine. Adv Drug Deliv Rev. (2009) 61:1020–32. doi: 10.1016/j.addr.2009.07.006
341. Levengood SL, Zhang M. Chitosan-based scaffolds for bone tissue engineering. J Mater Chem B Mater Biol Med. (2014) 2:3161–84. doi: 10.1039/c4tb00027g
342. Chou AI, Nicoll SB. Characterization of photocrosslinked alginate hydrogels for nucleus pulposus cell encapsulation. J Biomed Mater Res A. (2009) 91A:187–94. doi: 10.1002/jbm.a.32191
344. Li Z, Ramay HR, Hauch KD, Xiao D, Zhang M. Chitosan–alginate hybrid scaffolds for bone tissue engineering. Biomaterials. (2005) 26:3919–28. doi: 10.1016/j.biomaterials.2004.09.062
345. Maver T, Hribernik S, Mohan T, Smrke DM, Maver U, Stana-Kleinschek K. Functional wound dressing materials with highly tunable drug release properties. RSC Adv. (2015) 5:77873–84. doi: 10.1039/C5RA11972C
346. Shao X, Hunter CJ. Developing an alginate/chitosan hybrid fiber scaffold for annulus fibrosus cells. J Biomed Mater Res A. (2007) 82A:701–10. doi: 10.1002/jbm.a.31030
347. Stevens LR, Gilmore KJ, Wallace GG. Tissue engineering with gellan gum. Biomater. Sci. (2016) 4:1276–90. doi: 10.1039/C6BM00322B
348. Assunção-Silva RC, Gomes ED, Sousa N, Silva NA, Salgado AJ. Hydrogels and cell based therapies in spinal cord injury regeneration. Stem Cells Int. (2015) 2015:1–24. doi: 10.1155/2015/948040
349. Silva-Correia J, Oliveira JM, Caridade SG, Oliveira JT, Sousa RA, Mano JF, et al. Gellan gum-based hydrogels for intervertebral disc tissue-engineering applications. J Tissue Eng Regen Med. (2011) 5:e97–e107. doi: 10.1002/term.363
350. Smith AM, Shelton RM, Perrie Y, Harris JJ. An initial evaluation of gellan gum as a material for tissue engineering applications. J Biomater Appl. (2007) 22:241–54. doi: 10.1177/0885328207076522
351. Guo B, Ma PX. Synthetic biodegradable functional polymers for tissue engineering: a brief review. Sci China Chem. (2014) 57:490–500. doi: 10.1007/s11426-014-5086-y
352. Mercuri JJ, Gill SS, Simionescu DT. Novel tissue-derived biomimetic scaffold for regenerating the human nucleus pulposus. J Biomed Mater Res A. (2011) 96:422–35. doi: 10.1002/jbm.a.33001
353. Norbertczak HT, Ingham E, Fermor HL, Wilcox RK. Decellularized intervertebral discs: a potential replacement for degenerate human discs. Tissue Eng Part C Methods. (2020) 26:565–76. doi: 10.1089/ten.tec.2020.0104
354. Peng X, Yu L, Shi L, Dong H, Meng X, Zhu B. Polymeric hydrogels and decellularized nucleus pulposus extracellular matrix technology for nucleus pulposus repair and regeneration. Polym Test. (2023) 117:107854. doi: 10.1016/j.polymertesting.2022.107854
355. Penolazzi L, Lambertini E, D'Agostino S, Pozzobon M, Notarangelo MP, Greco P, et al. Decellularized extracellular matrix-based scaffold and hypoxic priming: a promising combination to improve the phenotype of degenerate intervertebral disc cells. Life Sci. (2022) 301:120623. doi: 10.1016/j.lfs.2022.120623
356. Fiordalisi M, Silva A, Barbosa M, Gonçalves R, Caldeira J. Intervertebral disc decellularisation: progress and challenges. Eur Cell Mater. (2021) 42:196–219. doi: 10.22203/eCM.v042a15
357. Gilbert TW, Sellaro TL, Badylak SF. Decellularization of tissues and organs. Biomaterials. (2006) 27:3675–83. doi: 10.1016/j.biomaterials.2006.02.014
358. Reing JE, Brown BN, Daly KA, Freund JM, Gilbert TW, Hsiong SX, et al. The effects of processing methods upon mechanical and biologic properties of porcine dermal extracellular matrix scaffolds. Biomaterials. (2010) 31:8626–33. doi: 10.1016/j.biomaterials.2010.07.083
359. Zhou X, Wang J, Huang X, Fang W, Tao Y, Zhao T, et al. Injectable decellularized nucleus pulposus-based cell delivery system for differentiation of adipose-derived stem cells and nucleus pulposus regeneration. Acta Biomater. (2018) 81:115–28. doi: 10.1016/j.actbio.2018.09.044
360. Cameron DJA, Shaver MP. Aliphatic polyester polymer stars: synthesis, properties and applications in biomedicine and nanotechnology. Chem Soc Rev. (2011) 40:1761–76. doi: 10.1039/C0CS00091D
361. Hakkarainen M, Albertsson A-C. Degradation products of aliphatic and aliphatic–aromatic polyesters. In:Albertsson A-C, Hakkarainen M, , editors. Chromatography for Sustainable Polymeric Materials: Renewable, Degradable and Recyclable, Advances in Polymer Science. Berlin; Heidelberg: Springer (2008). p. 85–116.
362. Jérôme C, Lecomte P. Recent advances in the synthesis of aliphatic polyesters by ring-opening polymerization. Adv Drug Deliv Rev. (2008) 60:1056–76. doi: 10.1016/j.addr.2008.02.008
363. Chan LKY, Leung VYL, Tam V, Lu WW, Sze KY, Cheung KMC. Decellularized bovine intervertebral disc as a natural scaffold for xenogenic cell studies. Acta Biomater. (2013) 9:5262–72. doi: 10.1016/j.actbio.2012.09.005
364. Kandel R, Roberts S, Urban JPG. Tissue engineering and the intervertebral disc: the challenges. Eur Spine J. (2008) 17:480–91. doi: 10.1007/s00586-008-0746-2
365. Aragon J, Navascues N, Mendoza G, Irusta S. Laser-treated electrospun fibers loaded with nano-hydroxyapatite for bone tissue engineering. Int J Pharm. (2017) 525:112–22. doi: 10.1016/j.ijpharm.2017.04.022
366. Aragón J, Salerno S, De Bartolo L, Irusta S, Mendoza G. Polymeric electrospun scaffolds for bone morphogenetic protein 2 delivery in bone tissue engineering. J Colloid Interface Sci. (2018) 531:126–37. doi: 10.1016/j.jcis.2018.07.029
367. Dixon DT, Gomillion CT. Conductive scaffolds for bone tissue engineering: current state and future outlook. J Funct Biomater. (2022) 13:1. doi: 10.3390/jfb13010001
368. van Uden S, Silva-Correia J, Oliveira JM, Reis RL. Current strategies for treatment of intervertebral disc degeneration: substitution and regeneration possibilities. Biomater Res. (2017) 21:22. doi: 10.1186/s40824-017-0106-6
369. Poillot P, Snuggs JW, Le Maitre CL, Huyghe JM. L-type voltage-gated calcium channels partly mediate mechanotransduction in the intervertebral disc. JOR Spine. (2022) 5:e1213. doi: 10.1002/jsp2.1213
370. Berlemann U, Schwarzenbach O. An injectable nucleus replacement as an adjunct to microdiscectomy: 2 year follow-up in a pilot clinical study. Eur Spine J. (2009) 18:1706–12. doi: 10.1007/s00586-009-1136-0
371. Pauza KJ, Moradian M, Lutz G. Intra-annular fibrin discseel®. In:Hunter CW, Davis TT, DePalma MJ, , editors. Regenerative Medicine : A Complete Guide for Musculoskeletal and Spine Disorders. Cham: Springer International Publishing (2023). p. 61–71.
372. Mohd Isa IL, Mokhtar SA, Abbah SA, Fauzi MB, Devitt A, Pandit A. Intervertebral disc degeneration: biomaterials and tissue engineering strategies toward precision medicine. Adv Healthc Mater. (2022) 11:e2102530. doi: 10.1002/adhm.202102530
373. O'Shea JJ, Holland SM, Staudt LM. JAKs and STATs in immunity, immunodeficiency, and cancer. N Engl J Med. (2013) 368:161–70. doi: 10.1056/NEJMra1202117
374. Khan AA, Mudassir J, Mohtar N, Darwis Y. Advanced drug delivery to the lymphatic system: lipid-based nanoformulations. Int J Nanomed. (2013) 8:2733–44. doi: 10.2147/IJN.S41521
375. Chen Y, Huang J, Tang C, Chen X, Yin Z, Heng BC, et al. Small molecule therapeutics for inflammation-associated chronic musculoskeletal degenerative diseases: past, present and future. Exp Cell Res. (2017) 359:1–9. doi: 10.1016/j.yexcr.2017.07.027
376. Ge Y, Chen Y, Guo C, Luo H, Fu F, Ji W, et al. Pyroptosis and intervertebral disc degeneration: mechanistic insights and therapeutic implications. J Inflamm Res. (2022) 15:5857–71. doi: 10.2147/JIR.S382069
377. Wang H, Li Z, Huo Y, Tian T, Yang D, Ma L, et al. 17β-Estradiol alleviates intervertebral disc degeneration by inhibiting NF-κB signal pathway. Life Sci. (2021) 284:119874. doi: 10.1016/j.lfs.2021.119874
378. Mannarino M, Cherif H, Li L, Sheng K, Rabau O, Jarzem P, et al. Toll-like receptor 2 induced senescence in intervertebral disc cells of patients with back pain can be attenuated by o-vanillin. Arthritis Res Ther. (2021) 23:117. doi: 10.1186/s13075-021-02504-z
379. Klawitter M, Quero L, Klasen J, Gloess AN, Klopprogge B, Hausmann O, et al. Curcuma DMSO extracts and curcumin exhibit an anti-inflammatory and anti-catabolic effect on human intervertebral disc cells, possibly by influencing TLR2 expression and JNK activity. J Inflamm. (2012) 9:29. doi: 10.1186/1476-9255-9-29
380. Saberi M, Zhang X, Mobasheri A. Targeting mitochondrial dysfunction with small molecules in intervertebral disc aging and degeneration. GeroScience. (2021) 43:517–37. doi: 10.1007/s11357-021-00341-1
381. Sen S, Sharma H, Singh N. Curcumin enhances vinorelbine mediated apoptosis in NSCLC cells by the mitochondrial pathway. Biochem Biophys Res Commun. (2005) 331:1245–52. doi: 10.1016/j.bbrc.2005.04.044
382. Wei Q-Y, Chen W-F, Zhou B, Yang L, Liu Z-L. Inhibition of lipid peroxidation and protein oxidation in rat liver mitochondria by curcumin and its analogues. Biochim Biophys Acta BBA - Gen Subj. (2006) 1760:70–7. doi: 10.1016/j.bbagen.2005.09.008
383. Yu Z, Xu N, Wang W, Pan S, Li K. Interleukin-1 inhibits Sox9 and collagen type II expression via nuclear factor-κB in the cultured human intervertebral disc cells. Chin Med J. (2009) 122:2483–8.
384. Zhu Y, Chen X, Chen Z, Zeng Y, Shi G, Su Y, et al. Curcumin protects mitochondria from oxidative damage and attenuates apoptosis in cortical neurons. Acta Pharmacol Sin. (2004) 25:1606–12.
385. Wei CC, Ping DQ, You FT, Qiang CY, Tao C. Icariin prevents cartilage and bone degradation in experimental models of arthritis. Mediators Inflamm. (2016) 2016:9529630. doi: 10.1155/2016/9529630
386. Hua W, Zhang Y, Wu X, Kang L, Tu J, Zhao K, et al. Icariin attenuates interleukin-1β-induced inflammatory response in human nucleus pulposus cells. Curr Pharm Des. (2018) 23:6071–8. doi: 10.2174/1381612823666170615112158
387. Chen Y, Wu Y, Shi H, Wang J, Zheng Z, Chen J, et al. Melatonin ameliorates intervertebral disc degeneration via the potential mechanisms of mitophagy induction and apoptosis inhibition. J Cell Mol Med. (2019) 23:2136–48. doi: 10.1111/jcmm.14125
388. Chen F, Jiang G, Liu H, Li Z, Pei Y, Wang H, et al. Melatonin alleviates intervertebral disc degeneration by disrupting the IL-1β/NF-κB-NLRP3 inflammasome positive feedback loop. Bone Res. (2020) 8:1–13. doi: 10.1038/s41413-020-0087-2
389. Jiang Z, Lu W, Zeng Q, Li D, Ding L, Wu J. High glucose-induced excessive reactive oxygen species promote apoptosis through mitochondrial damage in rat cartilage endplate cells. J Orthop Res. (2018) 36:2476–83. doi: 10.1002/jor.24016
390. Masuda K, Oegema TRJ, An HS. Growth factors and treatment of intervertebral disc degeneration. Spine. (2004) 29:2757–69. doi: 10.1097/01.brs.0000146048.14946.af
391. Wu PH, Kim HS, Jang I-T. I. PART 2: a review of the current diagnostic and treatment strategies for intervertebral disc disease. Int J Mol Sci. (2020) 21:2135. doi: 10.3390/ijms21062135
392. Badiu C. Williams textbook of endocrinology. Acta Endocrinol Buchar. (2019) 15:416. doi: 10.4183/aeb.2019.416
393. Yokozeki Y, Uchida K, Kawakubo A, Nakawaki M, Okubo T, Miyagi M, et al. TGF-β regulates nerve growth factor expression in a mouse intervertebral disc injury model. BMC Musculoskelet Disord. (2021) 22:634. doi: 10.1186/s12891-021-04509-w
394. Romaniyanto MF, Sigit Prakoeswa CR, Notobroto HB, Tinduh D, Ausrin R, Rantam FA, et al. An update of current therapeutic approach for intervertebral disc degeneration: a review article. Ann Med Surg. (2022) 77:103619. doi: 10.1016/j.amsu.2022.103619
395. Chou R, Hashimoto R, Friedly J, Fu R, Bougatsos C, Dana T, et al. Epidural corticosteroid injections for radiculopathy and spinal stenosis: a systematic review and meta-analysis. Ann Intern Med. (2015) 163:373–81. doi: 10.7326/M15-0934
396. Fernandez-Moure J, Moore CA, Kim K, Karim A, Smith K, Barbosa Z, et al. Novel therapeutic strategies for degenerative disc disease: Review of cell biology and intervertebral disc cell therapy. SAGE Open Med. (2018) 6:205031211876167. doi: 10.1177/2050312118761674
397. Gruber HE, Norton HJ, Hanley EN. Anti-apoptotic effects of IGF-1 and PDGF on human intervertebral disc cells in vitro. Spine. (2000) 25:2153–7. doi: 10.1097/00007632-200009010-00002
398. Orozco L, Soler R, Morera C, Alberca M, Sánchez A, García-Sancho J. Intervertebral disc repair by autologous mesenchymal bone marrow cells: a pilot study. Transplantation. (2011) 92:822–8. doi: 10.1097/TP.0b013e3182298a15
399. Mulligan RC. The basic science of gene therapy. Science. (1993) 260:926–32. doi: 10.1126/science.8493530
400. Nejepinska J, Malik R, Moravec M, Svoboda P. Deep sequencing reveals complex spurious transcription from transiently transfected plasmids. PLoS ONE. (2012) 7:e43283. doi: 10.1371/journal.pone.0043283
401. Igoucheva O, Alexeev V, Yoon K. Differential cellular responses to exogenous DNA in mammalian cells and its effect on oligonucleotide-directed gene modification. Gene Ther. (2006) 13:266–75. doi: 10.1038/sj.gt.3302643
402. Takeoka Y, Yurube T, Nishida K. Gene therapy approach for intervertebral disc degeneration: an update. Neurospine. (2020) 17:3–14. doi: 10.14245/ns.2040042.021
403. Fus-Kujawa A, Prus P, Bajdak-Rusinek K, Teper P, Gawron K, Kowalczuk A, et al. An overview of methods and tools for transfection of eukaryotic cells in vitro. Front Bioeng Biotechnol. (2021) 9:e701031. doi: 10.3389/fbioe.2021.701031
404. Evans CH, Ghivizzani SC, Robbins PD. Orthopaedic gene therapy: twenty-five years on. JBJS Rev. (2021) 9:e2000220. doi: 10.2106/JBJS.RVW.20.00220
405. Fiani B, Jarrah R, Cathel A, Sarhadi K, Covarrubias C, Soula M. The role of gene therapy as a valuable treatment modality for multiple spinal pathologies. Regen Med. (2021) 16:175–88. doi: 10.2217/rme-2020-0147
406. Watson-Levings RS, Palmer GD, Levings PP, Dacanay EA, Evans CH, Ghivizzani SC. Gene therapy in orthopaedics: progress and challenges in pre-clinical development and translation. Front Bioeng Biotechnol. (2022) 10:901317. doi: 10.3389/fbioe.2022.901317
407. Schaly S, Ghebretatios M, Prakash S. Baculoviruses in gene therapy and personalized medicine. Biol Targets Ther. (2021) 15:115–32. doi: 10.2147/BTT.S292692
408. Gardlík R, Pálffy R, Hodosy J, Lukács J, Turna J, Celec P. Vectors and delivery systems in gene therapy. Med Sci Monit Int Med J Exp Clin Res. (2005) 11:RA110–121.
409. Pannell D, Ellis J. Silencing of gene expression: implications for design of retrovirus vectors. Rev Med Virol. (2001) 11:205–17. doi: 10.1002/rmv.316
410. Vannucci L, Lai M, Chiuppesi F, Ceccherini-Nelli L, Pistello M. Viral vectors: a look back and ahead on gene transfer technology. New Microbiol. (2013) 36:1–22.
411. Pauwels K, Gijsbers R, Toelen J, Schambach A, Willard-Gallo K, Verheust C, et al. State-of-the-art lentiviral vectors for research use: risk assessment and biosafety recommendations. Curr Gene Ther. (2009) 9:459–74. doi: 10.2174/156652309790031120
412. Tatsis N, Ertl HCJ. Adenoviruses as vaccine vectors. Mol Ther J Am Soc Gene Ther. (2004) 10:616–29. doi: 10.1016/j.ymthe.2004.07.013
413. Alba R, Bosch A, Chillon M. Gutless adenovirus: last-generation adenovirus for gene therapy. Gene Ther. (2005) 12(Suppl. 1):S18–27. doi: 10.1038/sj.gt.3302612
414. High KA, Roncarolo MG. Gene therapy. N Engl J Med. (2019) 381:455–64. doi: 10.1056/NEJMra1706910
415. Naso MF, Tomkowicz B, Perry WL, Strohl WR. Adeno-associated virus (AAV) as a vector for gene therapy. BioDrugs Clin Immunother Biopharm Gene Ther. (2017) 31:317–34. doi: 10.1007/s40259-017-0234-5
416. Lehrman S. Virus treatment questioned after gene therapy death. Nature. (1999) 401:517–8. doi: 10.1038/43977
417. Stilwell JL, Samulski RJ. Role of viral vectors and virion shells in cellular gene expression. Mol Ther J Am Soc Gene Ther. (2004) 9:337–46. doi: 10.1016/j.ymthe.2003.11.007
418. Daya S, Berns KI. Gene therapy using adeno-associated virus vectors. Clin Microbiol Rev. (2008) 21:583–93. doi: 10.1128/CMR.00008-08
419. Jiao Y, Xia ZL, Ze LJ, Jing H, Xin B, Fu S. Research Progress of nucleic acid delivery vectors for gene therapy. Biomed Microdevices. (2020) 22:16. doi: 10.1007/s10544-020-0469-7
420. Kotterman MA, Chalberg TW, Schaffer DV. Viral vectors for gene therapy: translational and clinical outlook. Annu Rev Biomed Eng. (2015) 17:63–89. doi: 10.1146/annurev-bioeng-071813-104938
421. Kakutani K, Nishida K, Uno K, Takada T, Shimomura T, Maeno K, et al. Prolonged down regulation of specific gene expression in nucleus pulposus cell mediated by RNA interference in vitro. J Orthop Res. (2006) 24:1271–8. doi: 10.1002/jor.20171
422. Suzuki T, Nishida K, Kakutani K, Maeno K, Yurube T, Takada T, et al. Sustained long-term RNA interference in nucleus pulposus cells in vivo mediated by unmodified small interfering RNA. Eur Spine J. (2009) 18:263–70. doi: 10.1007/s00586-008-0873-9
423. Seki S, Asanuma-Abe Y, Masuda K, Kawaguchi Y, Asanuma K, Muehleman C, et al. Effect of small interference RNA (siRNA) for ADAMTS5on intervertebral disc degeneration in the rabbit anular needle-puncture model. Arthritis Res Ther. (2009) 11:R166. doi: 10.1186/ar2851
424. Banala RR, Vemuri SK, Dar GH, Palanisamy V, Penkulinti M, Surekha M, et al. Efficiency of dual siRNA-mediated gene therapy for intervertebral disc degeneration (IVDD). Spine J. (2019) 19:896–904. doi: 10.1016/j.spinee.2018.10.016
425. Bian A, Neyra JA, Zhan M, Hu MC. Klotho, stem cells, and aging. Clin Interv Aging. (2015) 10:1233–43. doi: 10.2147/CIA.S84978
426. Meng Z, Lu M. RNA interference-induced innate immunity, off-target effect, or immune adjuvant? Front Immunol. (2017) 8:e00331. doi: 10.3389/fimmu.2017.00331
427. Hsu PD, Lander ES, Zhang F. Development and applications of CRISPR-Cas9 for genome engineering. Cell. (2014) 157:1262–78. doi: 10.1016/j.cell.2014.05.010
428. Krupkova O, Cambria E, Besse L, Besse A, Bowles R, Wuertz-Kozak K. The potential of CRISPR/Cas9 genome editing for the study and treatment of intervertebral disc pathologies. JOR Spine. (2018) 1:e1003. doi: 10.1002/jsp2.1003
429. Fan Y, Zhao L, Lai Y, Lu K, Huang J. CRISPR-Cas9-mediated loss of function of β-catenin attenuates intervertebral disc degeneration. Mol Ther Nucleic Acids. (2022) 28:387–96. doi: 10.1016/j.omtn.2022.03.024
430. Farhang N, Ginley-Hidinger M, Berrett KC, Gertz J, Lawrence B, Bowles RD. Lentiviral CRISPR epigenome editing of inflammatory receptors as a gene therapy strategy for disc degeneration. Hum Gene Ther. (2019) 30:1161–75. doi: 10.1089/hum.2019.005
431. Mohd Isa IL, Teoh SL, Mohd Nor NH, Mokhtar SA. Discogenic low back pain: anatomy, pathophysiology and treatments of intervertebral disc degeneration. Int J Mol Sci. (2022) 24:208. doi: 10.3390/ijms24010208
432. Recuerda M, Périé D, Gilbert G, Beaudoin G. Assessment of mechanical properties of isolated bovine intervertebral discs from multi-parametric magnetic resonance imaging. BMC Musculoskelet Disord. (2012) 13:195. doi: 10.1186/1471-2474-13-195
433. Varden LJ, Turner EJ, Coon AT, Michalek AJ. Establishing a through-puncture model for assessing post-injection leakage in the intervertebral disc. Eur Spine J. (2022) 31:865–73. doi: 10.1007/s00586-022-07140-y
434. Varden LJ, Nguyen DT, Michalek AJ. Slow depressurization following intradiscal injection leads to injectate leakage in a large animal model. JOR Spine. (2019) 2:e1061. doi: 10.1002/jsp2.1061
435. Bertram H, Kroeber M, Wang H, Unglaub F, Guehring T, Carstens C, et al. Matrix-assisted cell transfer for intervertebral disc cell therapy. Biochem Biophys Res Commun. (2005) 331:1185–92. doi: 10.1016/j.bbrc.2005.04.034
436. Michalek AJ, Buckley MR, Bonassar LJ, Cohen I, Iatridis JC. The effects of needle puncture injury on microscale shear strain in the intervertebral disc annulus fibrosus. Spine J. (2010) 10:1098–105. doi: 10.1016/j.spinee.2010.09.015
437. Michalek AJ, Funabashi KL, Iatridis JC. Needle puncture injury of the rat intervertebral disc affects torsional and compressive biomechanics differently. Eur Spine J. (2010) 19:2110–6. doi: 10.1007/s00586-010-1473-z
438. Michalek AJ, Iatridis JC. Penetrating annulus fibrosus injuries affect dynamic compressive behaviors of the intervertebral disc via altered fluid flow: an analytical interpretation. J Biomech Eng. (2011) 133:915. doi: 10.1115/1.4004915
439. Daily JW, Yang M, Park S. Efficacy of turmeric extracts and curcumin for alleviating the symptoms of joint arthritis: a systematic review and meta-analysis of randomized clinical trials. J Med Food. (2016) 19:717–29. doi: 10.1089/jmf.2016.3705
440. Grothe K, Flechsenhar K, Paehler T, Ritzeler O, Beninga J, Saas J, et al. IκB kinase inhibition as a potential treatment of osteoarthritis – results of a clinical proof-of-concept study. Osteoarthritis Cartilage. (2017) 25:46–52. doi: 10.1016/j.joca.2016.08.010
441. Angriman F, Ferreyro BL, Burry L, Fan E, Ferguson ND, Husain S, et al. Interleukin-6 receptor blockade in patients with COVID-19: placing clinical trials into context. Lancet Respir Med. (2021) 9:655–64. doi: 10.1016/S2213-2600(21)00139-9
442. Wang Y, Che M, Xin J, Zheng Z, Li J, Zhang S. The role of IL-1β and TNF-α in intervertebral disc degeneration. Biomed Pharmacother. (2020) 131:110660. doi: 10.1016/j.biopha.2020.110660
443. Suoranta T, Laham-Karam N, Ylä-Herttuala S. Strategies to improve safety profile of AAV vectors. Front Mol Med. (2022) 2:1054069. doi: 10.3389/fmmed.2022.1054069
444. Huang Y, Gao J, Wang J, Ye H, Yao T, Xu Y, et al. Inhibition of intervertebral disc disease progression via the circPKNOX1–miR-370-3p–KIAA0355 axis. Cell Death Discov. (2021) 7:1–13. doi: 10.1038/s41420-021-00420-4
445. Zhang X, Hu Y, Hao D, Li T, Jia Y, Hu W, et al. New Strategies for the treatment of intervertebral disc degeneration: Cell, exosome, gene, and tissue engineering. Am J Transl Res. 14:8031–48. (2022).
446. Strassburg S, Richardson SM, Freemont AJ, Hoyland JA. Co-culture induces mesenchymal stem cell differentiation and modulation of the degenerate human nucleus pulposus cell phenotype. Regen Med. (2010) 5:701–11. doi: 10.2217/rme.10.59
447. Svanvik T, Henriksson HB, Karlsson C, Hagman M, Lindahl A, Brisby H. Human disk cells from degenerated disks and mesenchymal stem cells in co-culture result in increased matrix production. Cells Tissues Organs. (2010) 191:2–11. doi: 10.1159/000223236
448. Watanabe T, Sakai D, Yamamoto Y, Iwashina T, Serigano K, Tamura F, et al. Human nucleus pulposus cells significantly enhanced biological properties in a coculture system with direct cell-to-cell contact with autologous mesenchymal stem cells. J Orthop Res. (2010) 28:623–30. doi: 10.1002/jor.21036
449. Buckley CT, Hoyland JA, Fujii K, Pandit A, Iatridis JC, Grad S. Critical aspects and challenges for intervertebral disc repair and regeneration-Harnessing advances in tissue engineering. JOR Spine. (2018) 1:e1029. doi: 10.1002/jsp2.1029
450. Iatridis JC, Kang J, Kandel R, Risbud MV. New horizons in spine research: intervertebral disc repair and regeneration. J Orthop Res Off Publ Orthop Res Soc. (2017) 35:5–7. doi: 10.1002/jor.23499
451. Peng B, Li Y. Concerns about cell therapy for intervertebral disc degeneration. Npj Regen Med. (2022) 7:46. doi: 10.1038/s41536-022-00245-4
452. Poletto DL, Crowley JD, Tanglay O, Walsh WR, Pelletier MH. Preclinical in vivo animal models of intervertebral disc degeneration Part 1: a systematic review. JOR Spine. (2022) 6:1234. doi: 10.1002/jsp2.1234
453. Sakai D, Andersson GBJ. Stem cell therapy for intervertebral disc regeneration: obstacles and solutions. Nat Rev Rheumatol. (2015) 11:243–56. doi: 10.1038/nrrheum.2015.13
454. Alkhatib B, Ban GI, Williams S, Serra R. IVD Development: nucleus pulposus development and sclerotome specification. Curr Mol Biol Rep. (2018) 4:132–41. doi: 10.1007/s40610-018-0100-3
455. Lawson L, Harfe BD. Notochord to nucleus pulposus transition. Curr Osteoporos Rep. (2015) 13:336–41. doi: 10.1007/s11914-015-0284-x
456. McCann M, Séguin C. Notochord cells in intervertebral disc development and degeneration. J Dev Biol. (2016) 4:3. doi: 10.3390/jdb4010003
457. McCann MR, Tamplin OJ, Rossant J, Séguin CA. Tracing notochord-derived cells using a Noto-cre mouse: implications for intervertebral disc development. Dis Model Mech. (2012) 5:73–82. doi: 10.1242/dmm.008128
458. Risbud MV, Schaer TP, Shapiro IM. Toward an understanding of the role of notochordal cells in the adult intervertebral disc: from discord to accord. Dev Dyn. (2010) 239:2141–8. doi: 10.1002/dvdy.22350
459. Trout JJ, Buckwalter JA, Moore KC, Landas SK. Ultrastructureofthe human intervertebral disc. I Changes in notochordal cells with age. Tissue Cell. (1982) 14:359–69. doi: 10.1016/0040-8166(82)90033-7
460. Théry C, Amigorena S, Raposo G, Clayton A. Isolation and characterization of exosomes from cell culture supernatants and biological fluids. Curr Protoc Cell Biol. (2006) 3:22. doi: 10.1002/0471143030.cb0322s30
461. Ludwig N, Whiteside TL, Reichert TE. Challenges in exosome isolation and analysis in health and disease. Int J Mol Sci. (2019) 20:4684. doi: 10.3390/ijms20194684
462. Théry C, Witwer KW, Aikawa E, Alcaraz MJ, Anderson JD, Andriantsitohaina R, et al. Minimal information for studies of extracellular vesicles 2018 (MISEV2018): a position statement of the International Society for Extracellular Vesicles and update of the MISEV2014 guidelines. J Extracell Vesicles. (2018) 7:1535750. doi: 10.1080/20013078.2018.1535750
463. Davidson SM, Boulanger CM, Aikawa E, Badimon L, Barile L, Binder CJ, et al. Methods for the identification and characterization of extracellular vesicles in cardiovascular studies: from exosomes to microvesicles. Cardiovasc Res. (2022) 119:cvac031. doi: 10.1093/cvr/cvac031
464. Gelibter S, Marostica G, Mandelli A, Siciliani S, Podini P, Finardi A, et al. The impact of storage on extracellular vesicles: A systematic study. J Extracell Vesicles. (2022) 11:e12162. doi: 10.1002/jev2.12162
465. van de Wakker SI, van Oudheusden J, Mol EA, Roefs MT, Zheng W, Görgens A, et al. Influence of short term storage conditions, concentration methods and excipients on extracellular vesicle recovery and function. Eur J Pharm Biopharm. (2022) 170:59–69. doi: 10.1016/j.ejpb.2021.11.012
466. Lakstins K, Arnold L, Gunsch G, Flanigan D, Khan S, Gadde N, et al. Characterization of the human intervertebral disc cartilage endplate at the molecular, cell, and tissue levels. J Orthop Res. (2021) 39:1898–907. doi: 10.1002/jor.24854
467. Bonnheim NB, Wang L, Lazar AA, Zhou J, Chachad R, Sollmann N, et al. The contributions of cartilage endplate composition and vertebral bone marrow fat to intervertebral disc degeneration in patients with chronic low back pain. Eur Spine J. (2022) 31:1866–72. doi: 10.1007/s00586-022-07206-x
468. Su Y, Ren D, Liu D, Li J, Wang T, Qi W, et al. Effects of endplate healing morphology on intervertebral disc degeneration after pedicle screw fixation for thoracolumbar fractures. Medicine. (2021) 100:e25636. doi: 10.1097/MD.0000000000025636
Keywords: intervertebral disc degeneration, therapy, exosome, small molecule, stem cell
Citation: Samanta A, Lufkin T and Kraus P (2023) Intervertebral disc degeneration—Current therapeutic options and challenges. Front. Public Health 11:1156749. doi: 10.3389/fpubh.2023.1156749
Received: 01 February 2023; Accepted: 12 June 2023;
Published: 06 July 2023.
Edited by:
Qiling Yuan, Xi'an Jiaotong University, ChinaReviewed by:
Min Lu, Shanghai Jiao Tong University, ChinaDanny Chan, The University of Hong Kong, Hong Kong SAR, China
Copyright © 2023 Samanta, Lufkin and Kraus. This is an open-access article distributed under the terms of the Creative Commons Attribution License (CC BY). The use, distribution or reproduction in other forums is permitted, provided the original author(s) and the copyright owner(s) are credited and that the original publication in this journal is cited, in accordance with accepted academic practice. No use, distribution or reproduction is permitted which does not comply with these terms.
*Correspondence: Petra Kraus, cGtyYXVzQGNsYXJrc29uLmVkdQ==