- 1Department of Environmental Health, Harvard T.H. Chan School of Public Health, Boston, MA, United States
- 2John B. Little Center for Radiation Sciences, Harvard T.H. Chan School of Public Health, Boston, MA, United States
- 3Environmental and Occupational Medicine and Epidemiology Program, Harvard T.H. Chan School of Public Health, Boston, MA, United States
During air travel, flight crew (flight attendants, pilots) can be exposed to numerous flight-related environmental DNA damaging agents that may be at the root of an excess risk of cancer and other diseases. This already complex mix of exposures is now joined by SARS-CoV-2, the virus that causes COVID-19. The complex exposures experienced during air travel present a challenge to public health research, but also provide an opportunity to consider new strategies for understanding and countering their health effects. In this article, we focus on threats to genomic integrity that occur during air travel and discuss how these threats and our ability to respond to them may influence the risk of SARS-CoV-2 infection and the development of range of severity of the symptoms. We also discuss how the virus itself may lead to compromised genome integrity. We argue that dauntingly complex public health problems, such as the challenge of protecting flight crews from COVID-19, must be met with interdisciplinary research teams that include epidemiologists, engineers, and mechanistic biologists.
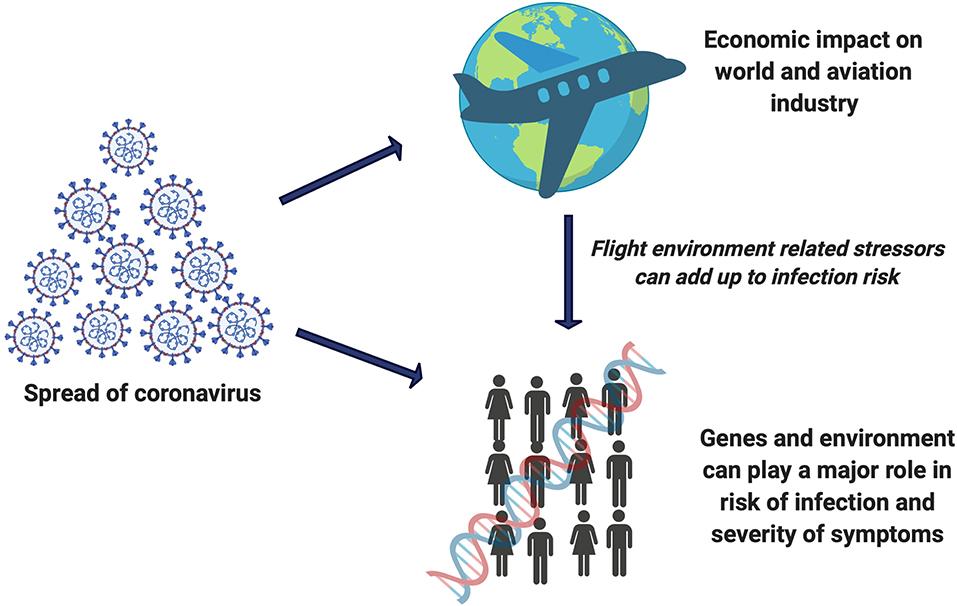
Graphical Abstract. SARS-CoV-2 risk is an add-on entity to the list of flight related damaging effects which can impact the genome integrity of flight crew.
Introduction
Understanding and solving public health crises require consideration of diverse inputs spanning from the molecular to the global level. The current COVID-19 pandemic illustrates this dynamic particularly well in the context of air travel, which entails exposure to a complex variety of potentially hazardous agents, some of which are likely responsible for the excess risk for cancer and other diseases in flight crews (1–8).
Ensuring safety during air travel represents a large and complex challenge. Approximately 4.1 billion passengers travel by air each year (9). There are estimated to be over 140,000 airline pilots employed internationally, and approximately 120,000 flight attendants are employed just in the U.S. (10). On 11th March 2020, the World Health Organization declared COVID-19, the disease caused by severe acute respiratory syndrome coronavirus 2 (SARS-CoV-2) to be a pandemic. The disease has now touched nearly every aspect of our lives and has severely impacted the aviation industry (11). Unprecedented flight cancellations, restriction of non-essential travel, border closures, and policies taken in response to the pandemic have hit the travel industry very hard (12). With the uncertainty about the development and eventual efficacy of a vaccine for COVID-19, social distancing and engineering solutions such as the usage of personal protective equipment (PPE) are pivotal measures to lower the risk of COVID-19 transmission. New guidelines have been implemented by the Center for Disease Control and Prevention (CDC) and Federal Aviation Administration (FAA) for air travel during the COVID-19 pandemic, but airlines still struggle to balance scheduling of fewer flights with maximizing social distancing (13, 14). Further complicating matters, air travel demand is in flux, and social distancing in airplanes may be impossible in some aircraft due to seat configuration. Other necessary aspects of air travel that occur outside the airplane, such as passing through Transport Security Administration (TSA) precheck, can also potentially increase the risk of COVID-19 exposure for flight crew. Thus, every single aspect during air travel needs to be considered in order to avoid increased risk of exposure and spread of SARS-CoV-2.
The high frequency of asymptomatic SARS-CoV-2 carriers and the period between exposure and infection of up to 2 weeks pose an acute challenge for estimating the risk of transmission in general, and during the air travel in particular. Furthermore, we do not yet understand why the virus has such disparate impacts on different people. Although some clues are beginning to emerge and are discussed in more detail below, relatively little is known about the role of environmental exposures and inter-individual biological variation with regard to susceptibility to viral infection or development of severe symptoms. We note, however, that several of the exposures that are associated with greater risk of severe disease include agents that compromise genome integrity (15, 16). Genome integrity refers to the protection of DNA from damage and mutations that can impact cellular function or lead to cell death (17). Compromised genome integrity is linked to various health risks (17, 18). This article briefly highlights the possible role of genome integrity in the etiology of COVID-19 and other diseases that are of concern for flight crew. We recommend a multidisciplinary paradigm for advancing our understanding of complex public health problems by focusing on key biological pathways, in this case genome integrity.
Air Travel and Genome Integrity
Flight crew are subjected to diverse sources of flight related exposures that may pose health risks. These exposures include physical stressors (air pressure changes, vibration, changes in oxygen levels, prolonged immobility, aircraft noise); in-flight chemical exposures (pesticides, flame retardants); radiation (cosmic ionizing radiation, ultraviolet light) and biological changes (circadian rhythm disruption, mental and physical stress) (7, 19, 20). In addition, flight attendants are at higher risk of viral exposure due to their interactions with passengers and are expected to experience an increased work-related stress during the pandemic (21–23).
A growing body of literature suggests that flight-related exposures can directly or indirectly affect the human body. In particular, some of these exposures damage DNA, the genetic blueprint of the cell (24–26). DNA damage can lead to cell death or mutations, which are key biological mechanisms underlying a variety of diseases. To avoid cell death and mutagenesis, DNA repair mechanisms in human cells remove DNA damage and thereby maintain genome integrity (27–30). Inefficient DNA repair in humans has also been associated with susceptibility to several diseases including cancer and immune disorders that predispose to infection (8, 31–34). Epidemiological studies have documented excess cancer risk in flight attendants and pilots (1–8), indicating that compromised genome integrity may be a particular problem for this population. Although not all of the agents to which flight crew are exposed are known DNA damaging agents, some flight crew-based research studies have demonstrated that exposure to cosmic ionizing radiation, which damages DNA, is associated with elevated cancer risk (35–40). Additional evidence comes from studies that have found links between cosmic ionizing radiation exposure and reproductive health risk (2, 41, 42), but the underlying biological mechanism has not yet been elucidated.
One testable hypothesis is that DNA damage and alterations in repair responses due to flight related exposures are responsible for the diminished genomic integrity that leads to increased risk of cancer and perhaps other diseases among flight crew. Notably, viral infections also pose a threat to genome integrity (43) and there is evidence suggesting that the severity of disease caused by respiratory RNA viruses may be related to the extent of DNA damage induced during and after infection (44). Since the ability to repair DNA damage would influence the extent of DNA damage that accumulates during infection, and since DNA repair pathways play a central role in immune function, it is possible that DNA repair capacity impacts susceptibility to severe COVID-19. Thus, some of the same principles that are increasingly well-understood in the context of genome integrity and cancer risk may also apply to understanding the origins of inter-individual differences in susceptibility to viral infections and the development of severe symptoms, but this idea has yet to be explored.
COVID-19 Risk and Genome Integrity
SARS-CoV-2 is an RNA virus that hijacks host cellular mechanisms during its lifecycle (45, 46). RNA viruses can trigger a multitude of cellular changes including activation of the DNA damage response, DNA replication stress, dysregulation of the cell cycle, induction of apoptosis, and several other stress responses (47–49). In the case of SARS-CoV-2, alterations in cellular processes that promote viral replication also lead to activation of oncogenic pathways (50), which raises questions regarding the potential for SARS-CoV-2 to promote cancer (51). As is the case for cancer risk (29, 34, 51) genetic background contributes toward inter-individual variation in susceptibility and treatment outcomes for respiratory disease (52). In the case of SARS-CoV-2, polymorphisms in the gene encoding angiotensin converting enzyme 2 (ACE2) have been associated with variation in susceptibility to SARS-CoV-2 infection (53, 54). The etiology of SARS-CoV-2 infection and severe COVID-19 is multifactorial and can be attributed to polymorphisms immune system genes (55, 56), viral mutations (57), variation in the respiratory microbiome (58). Although DNA repair has not been studied in the context of SARS-CoV-2 susceptibility, limited studies that have been conducted in other infectious disease contexts suggest that polymorphisms in DNA repair genes may affect host responses to pathogens (59, 60).
The potential interaction between cancer and COVID-19 outcomes remains largely unexplored and may provide additional insights into a possible shared role for genome integrity in the etiology of both diseases. A study among 1,590 COVID-19 patients in China revealed cancer to be an important risk factor for health endpoints associated with severe COVID-19 (61), raising important ethical questions regarding the prioritization of treatment (62). In further support of a link between cancer and COVID-19, an analysis by WHO found the fatality rate among COVID-19 infected patients with cancer was double the rate for COVID-19 patients without cancer (51). This excess mortality may be attributed at least in part to compromised immune function in cancer patients due to treatments and the effects of the disease itself. However, the biological mechanisms underlying these associations remain unclear. Given the well-established relationship between cancer risk and inter-individual variation in DNA repair capacity (34) and given the potential role for persistent DNA damage in the pathogenesis of COVID-19, an intriguing possibility is that DNA repair capacity might play a role in the severity of COVID-19 infection and treatment outcomes. A more complete understanding of how SARS-CoV-2 impacts genome integrity would provide valuable information for developing potential DNA damage and repair biomarkers that could help to assess an individual's risk infection and severe illness due to SARS-CoV-2 and other viral infections that may be influenced by genome integrity pathways.
Current and Future Approaches to Risk Mitigation
Despite aggressive efforts to minimize SARS-CoV-2 risk during air travel, this exposure joins a long list of others that cannot be entirely eliminated for those who must travel. In-flight transmission risk is still not completely understood, and several studies have shown compelling evidence of validated in-flight COVID-19 mass transmission from past in-flight events (63, 64). The advanced particle filtration and air exchange systems used in commercial aircraft should decrease transmission risk, which was supported by a recent in-flight aerosol dispersion study that found minimal aerosol exposure risk even during long duration flights (65). In-flight COVID-19 risk is likely impacted by differences between aircraft type, ventilation systems and real-world flight environments as compared to experimental flight conditions, however one factor that appears to have a noteworthy impact on in-flight transmission is use of face-masking during air travel (66). For the time being, global, one-size-fits-all preventive measures are the mainstay of efforts to protect the public. Hand washing, masks, and other PPE (13), together with temperature checks, touchless check-in, one-way boarding (back to front), plexiglass shields and empty middle seats in aircraft, are among the available strategies for mitigating air travel-related spread of SARS-CoV-2 (67). Additional measures can likely be developed to augment these strategies. Improved screening tools can be developed to upgrade the existing non-laboratory-based methods for detecting COVID-19 infected individuals and ensuring asymptomatic infectious individuals do not travel. Coordination within airports is also essential for reducing the spread of SARS-CoV-2 infection among airport employees such as ticketing staff, TSA, security personnel, airport workers and others. In the US, TSA security procedures have already been updated and implemented to minimize the risk of COVID-19 transmission (68).
Looking forward, the myriad exposures encountered by flight crews will remain for the foreseeable future, necessitating new approaches for understanding and mitigating their potential health effects. Even after the COVID-19 pandemic passes, lessons learned during this time should inform preparedness for similar events in the future. Some strategic measures available to individuals that have been proposed include avoiding itineraries that may disrupt circadian rhythm (69) or using antioxidants to counteract the production of free radicals produced as a consequence of viral infection or exposures encountered during travel (70). Many historical areas of concern for adverse flight environment exposures such as ultraviolet (UV) radiation have been mostly mitigated through upgrades in aircraft materials or systems (71). However, a comprehensive understanding of the biological mechanisms underlying the excess risk of disease in flight crews will be needed in order to develop truly personalized prevention strategies that take into account individual exposures and inter-individual differences in responses to exposures.
There is a need for multidisciplinary approaches to address this issue and gather information about the impact of air travel on genomic integrity. Although many factors contribute to disease risk, one promising approach toward improved and more personalized prevention is to focus on genome integrity, a fundamental biological pathway that links many of the exposures encountered by flight crew. Well-designed studies should make use of dosimetry or sensors to quantify as many occupational exposures as possible. Comprehensive exposure analysis will enable efforts to determine the effect of complex mixtures. By joining these analyses with laboratory based mechanistic studies using genomics approaches and functional assays in biospecimens and model systems, we can improve our understanding of how DNA damage and repair influence health outcomes in flight crew. Such studies will provide a foundation for developing personalized prevention or risk assessment profiles for the flight crew that may also benefit passengers.
Conclusions
The COVID-19 pandemic has severely impacted the global economy, affecting several industries and having a ripple effect on every aspect of human life. The complexity and magnitude of the challenge of responding to COVID-19 is exemplified by the multiple, in some cases ill-defined risks associated with air travel. Because these risks are unavoidable for some, it appears the only way out is through. A multidisciplinary approach toward COVID-19 mitigation to support the safe and secure return of normal air travel operations is already underway (72). There still exist many unraveling puzzles regarding the biological basis for infection risk in humans that need to be explored and could strongly influence the preventive measures implemented by airlines in the future. Activation of host DNA damage responses are associated with RNA virus replication (46, 47), raising the possibility that inter-individual differences in DNA repair capacity may play a role in susceptibility to severe COVID-19 symptoms. DNA damage and repair biomarkers may provide insights into an individual's risk toward viral infection or poor outcomes. Better understanding of the etiology of COVID-19 will shed light on optimal approaches for limiting COVID-19 spread and may inform preparedness for future pandemics.
Research aimed at understanding individual disease susceptibility following flight-related exposures can assist policy makers in making data-driven decisions regarding travel regulations. Efforts to understand the biological mechanisms underlying disease as they operate in a real world context must be a central element of this research. This form of mechanistic epidemiology is sometimes perceived to be at odds with the conventional reductionist approach to biology, which favors the isolation of variables and experimentation with the simplest possible model. As a result, there will be a need for funding agencies to promote work that follows this paradigm. To achieve success, there is an utmost need to establish collaborative working platforms among researchers, engineers, clinicians and the pharmaceutical industry to identify new strategies for reducing the risk of COVID-19 during air travel. In turn this will help the aviation industry to develop measures for safeguarding passengers and aircrew during air travel. Although we have chosen in this article to focus on the complex interplay of COVID-19 and threats to genome integrity during air travel, the interdisciplinary mechanistic epidemiology approach we favor is applicable to studying COVID-19 in other settings, and for unraveling complex public health problems in general.
Data Availability Statement
The original contributions generated for this study are included in the article/supplementary materials, further inquiries can be directed to the corresponding author/s.
Author Contributions
ST: expert on biological DNA damage and repair mechanisms. CS: expert on flight-related exposures and overall medical/clinical effects and outcomes. ZN: expert on DNA damage and repair and drafting and editing. All authors contributed to the article and approved the submitted version.
Conflict of Interest
The authors declare that the research was conducted in the absence of any commercial or financial relationships that could be construed as a potential conflict of interest.
References
1. Blettner M, Zeeb H, Auvinen A, Ballard TJ, Caldora M, Eliasch H, et al. Mortality from cancer and other causes among male airline cockpit crew in Europe. Int J Cancer. (2003) 106:946–52. doi: 10.1002/ijc.11328
2. Dreger S, Wollschlager D, Schafft T, Hammer GP, Blettner M, Zeeb H. Cohort study of occupational cosmic radiation dose and cancer mortality in German aircrew, 1960-2014. Occup Environ Med. (2020) 77:285–91. doi: 10.1136/oemed-2019-106165
3. Hammer GP, Auvinen A, De Stavola BL, Grajewski B, Gundestrup M, Haldorsen, et al. Mortality from cancer and other causes in commercial airline crews: a joint analysis of cohorts from 10 countries. Occup Environ Med. (2014) 71:313–22. doi: 10.1136/oemed-2013-101395
4. Paridou A, Velonakis E, Langner I, Zeeb H, Blettner M, Tzonou A. Mortality among pilots and cabin crew in Greece, 1960-1997. Int J Epidemiol. (2003) 32:244–7. doi: 10.1093/ije/dyg056
5. Zeeb H, Blettner M, Langner I, Hammer GP, Ballard TJ, Santaquilani M. Mortality from cancer and other causes among airline cabin attendants in Europe: a collaborative cohort study in eight countries. Am J Epidemiol. (2003) 158:35–46. doi: 10.1093/aje/kwg107
6. dos Santos Silva I, De Stavola B, Pizzi C, Evans AD, Evans SA. Cancer incidence in professional flight crew and air traffic control officers: disentangling the effect of occupational versus lifestyle exposures. Int J Cancer. (2013) 132:374–84. doi: 10.1002/ijc.27612
7. McNeely E, Mordukhovich I, Staffa S, Tideman S, Gale S, Coull B. Cancer prevalence among flight attendants compared to the general population. Environ Health. (2018) 17:49. doi: 10.1186/s12940-018-0396-8
8. McNeely E, Mordukhovich I, Tideman S, Gale S, Coull B. Estimating the health consequences of flight attendant work: comparing flight attendant health to the general population in a cross-sectional study. BMC Public Health. (2018) 18:346. doi: 10.1186/s12889-018-5221-3
9. International Air Transport Association (2018) Traveler Numbers Reach New Heights. Press release 51, Annual Review 2018. Available online at: https://www.iata.org/en/pressroom/pr/2018-09-06-01/ (accessed September 6, 2018).
10. Bureau of Labor Statistics (2020) U.S. Department of Labor, Occupational Outlook Handbook, Flight Attendants. Available online at: https://www.bls.gov/ooh/transportation-and-material-moving/flight-attendants.htm (Accessed July 18, 2020).
11. WHO (2020) WHO Timeline-COVID-19. Available online at: https://www.who.int/news/item/27-04-2020-who-timeline---covid-19 (accessed April 27, 2018).
12. Nicola M, Alsafi Z, Sohrabi C, Kerwan A, Al-Jabir A, Iosifidis, et al. The socio-economic implications of the coronavirus pandemic (COVID-19): a review. Int J Surg (London, England). (2020) 78:185–93. doi: 10.1016/j.ijsu.2020.04.018
13. CDC (2020) Updated interim guidance for airlines and airline crew: Coronavirus disease 2019 (COVID-19). (4 March 2020). Available online at: https://www.cdc.gov/quarantine/air/managing-sick-travelers/ncov-airlines.html (accessed July 15, 2020).
14. Federal Aviation Administration (FAA) U.S. Department of Transportation. Safety Alert for Operators: SAFO 20009. Washington DC: Flight Standards Service (29 Sep 2020).
15. Wu X, Nethery RC, Sabath BM, Braun D, Dominici F. Exposure to air pollution and COVID-19 mortality in the United States: a nationwide cross-sectional study. medRxiv [Preprint]. (2020). doi: 10.1101/2020.04.05.20054502
16. WHO (2020) Smoking and COVID-19. COVID-19: Scientific Briefs. Available online at: https://www.who.int/publications/i/item/smoking-and-covid-19 WHO/2019-nCoV/Sci_Brief/Smoking/2020.2 (accessed October 10, 2020).
17. Shen Z. Genomic instability and cancer: an introduction. J Mol Cell Biol. (2011) 3:1–3. doi: 10.1093/jmcb/mjq057
18. Hakem R. DNA-damage repair; the good, the bad, the ugly. EMBO J. (2008) 27:589–605. doi: 10.1038/emboj.2008.15
19. Ballard TJ, Corradi L, Lauria L, Mazzanti C, Scaravelli G, Sgorbissa F, et al. Integrating qualitative methods into occupational health research: a study of women flight attendants. Occup Environ Med. (2004) 61:163–6. doi: 10.1136/oem.2002.006221
20. Griffiths RF, Powell DM. The occupational health and safety of flight attendants. Aviat Space Environ Med. (2012) 83:514–21. doi: 10.3357/ASEM.3186.2012
21. Andenaes S, Lie A, Degre M. Prevalence of hepatitis A, B, C, and E antibody in flying airline personnel. Aviat Space Environ Med. (2000) 71:1178–80.
22. Gutersohn T, Steffen R, Van Damme P, Holdener F, Beutels P. Hepatitis A infection in aircrews: risk of infection and cost-benefit analysis of hepatitis A vaccination. Aviat Space Environ Med. (1996) 67:153–6.
23. Holdener F, Grob PJ, Joller-Jemelka HL. Hepatitis virus infection in flying airline personnel. Aviat Space Environ Med. (1982) 53:587–90.
24. National Research Council (US) Committee on Air Quality in Passenger Cabins of Commercial Aircraft. The Airliner Cabin Environment and the Health of Passengers and Crew. Washington (DC): National Academies Press (US) (2002). Available online at: https://www.ncbi.nlm.nih.gov/books/NBK207471/ (accessed May 21, 2020).
25. Sancar A, Lindsey-Boltz LA, Kang TH, Reardon JT, Lee JH, Ozturk N. Circadian clock control of the cellular response to DNA damage. FEBS Let. (2010) 584:2618–25. doi: 10.1016/j.febslet.2010.03.017
26. Forlenza MJ, Latimer JJ, Baum A. The effects of stress on DNA repair capacity. Psychol Health. (2000) 15:881–91. doi: 10.1080/08870440008405589
27. Toprani SM, Das B. Role of base excision repair genes and proteins in gamma-irradiated resting human peripheral blood mononuclear cells. Mutagenesis. (2015) 30:247–61. doi: 10.1093/mutage/geu065
28. Nagel ZD, Engelward BP, Brenner DJ, Begley TJ, Sobol RW, Bielas, et al. Towards precision prevention: technologies for identifying healthy individuals with high risk of disease. Mutat Res - Fundam Mol Mech Mutagen. (2017) 800–802:14–28. doi: 10.1016/j.mrfmmm.2017.03.007
29. Toprani SM, Das B. Radio-adaptive response, individual radio-sensitivity and correlation of base excision repair gene polymorphism (hOGG1, APE1, XRCC1, and LIGASE1) in human peripheral blood mononuclear cells exposed to gamma radiation. Environ Mol Mutagen. (2020) 61:551–9. doi: 10.1002/em.22383
30. Soren DC, Toprani SM, Jain V, Saini D, Das B. Quantitation of genome damage and transcriptional profile of DNA damage response genes in human peripheral blood mononuclear cells exposed in vitro to low doses of neutron radiation. Int J Radiat Res. (2019) 17:1–14. Available online at: http://ijrr.com/article-1-2453-en.html
31. Altmann T, Gennery AR. DNA ligase IV syndrome; a review. Orphanet J Rare Dis. (2016) 11:137. doi: 10.1186/s13023-016-0520-1
32. Toprani SM. DNA damage and repair scenario in ameloblastoma. Oral Oncol. (2020) 108:104804. doi: 10.1016/j.oraloncology.2020.104804
33. Toprani SM. Mane VK. Role of DNA damage and repair mechanisms in uterine fibroid/leiomyomas: a review. Biol Reprod. (2020) ioaa157. doi: 10.1093/biolre/ioaa157
34. Nagel ZD, Chaim IA, Samson LD. Inter-individual variation in DNA repair capacity: a need for multi-pathway functional assays to promote translational. DNA Repair Res DNA Repair (Amst). (2014) 19:199–213. doi: 10.1016/j.dnarep.2014.03.009
35. Heimers A. Cytogenetics analysis in human lymphocytes after exposure to stimulated cosmic radiation which reflects the inflight radiation environment. Int J Radiat Biol. (1999) 75:691–8. doi: 10.1080/095530099140023
36. Grajewski B, Yong LC, Bertke SJ, Bhatti P, Ltille MP, Ramsey, et al. Chromosome transolcations and cosmic radiation. Aerosp Med Hum Perform. (2018) 89:616–25. doi: 10.3357/AMHP.4502.2018
37. Romano E, Ferrucci L, Nicolai F, Derme V, De Stefano GF. Increase of chromosomal aberrations induced by ionizing radiation in peripheral blood lymphocytes of civil aviation pilots and crew members. Mutat Res. (1997) 377:89–93. doi: 10.1016/S0027-5107(97)00064-X
38. Bolzan AD, Bianchi MS, Gimenez EM, Flaque MC, Cinacio VR. Analysis of spontenaous and bleomycin induced chromosome damage in peripheral lymphocytes of long-haul aircrew members from Argentina. Mutat Res. (2008) 639:64–79. doi: 10.1016/j.mrfmmm.2007.11.003
39. Wolf G, Pieper R, Obe G. Chromosomal alterations in peripheral lymphocytes of female cabin attendants. Int J Radiat Biol. (1999) 75:829–36. doi: 10.1080/095530099139881
40. Cavallo D, Marinaccio A, Perniconi B, Tomao P, Pecoriello V, Moccaldi R, et al. Chromosomal aberrations in long haul air crew members. Mutat Res. (2002) 513:11–5. doi: 10.1016/S1383-5718(01)00276-5
41. Kojo K, Helminen M, Pukkala E, Auvinen A. Risk factors for skin cancer among Finnish airline cabin crew. Ann Occup Hyg. (2013) 57:695–704. doi: 10.1093/annhyg/mes106
42. Kojo K, Pukkala E, Auvinen A. Breast cancer risk among Finnish cabin attendants: a nested case-control study. Occup Environ Med. (2005) 62:488–93. doi: 10.1136/oem.2004.014738
43. Sahan AZ, Hazra TK, Das S. The pivotal role of dna repair in infection mediated-inflammation and cancer. Front Microbiol. (2018) 9:663. doi: 10.3389/fmicb.2018.00663
44. Li N, Parrish M, Chan TK, Yin L, Rai P, Yoshiyuki Y, et al. Influenza infection induces host DNA damage and dynamic DNA damage responses during tissue regeneration. Cell Mol Life Sci. (2015) 72:2973–88. doi: 10.1007/s00018-015-1879-1
45. Xu LH, Huang M, Fang SG, Liu DX. Coronavirus infection induces DNA replication stress partly through interaction of its nonstructural protein 13 with the p125 subunit of DNA polymerase δ. J Biol Chem. (2011) 286:39546–59. doi: 10.1074/jbc.M111.242206
46. Lim YX, Ng YL, Tam JP, Liu DX. Human coronaviruses: a review of virus-host interactions. Diseases. (2016) 4:26. doi: 10.3390/diseases4030026
47. Ryan EL, Hollingworth R, Grand RJ. Activation of the DNA Damage Response by RNA Viruses. Biomolecules. (2016) 6:2. doi: 10.3390/biom6010002
48. Sun T, Yang W, Toprani SM, Guo W, He L, DeLeo, et al. Induction of immunogenic cell death in radiation-resistant breast cancer stem cells by repurposing anti-alcoholism drug disulfiram. Cell Commun Signal. (2020) 18:53. doi: 10.1186/s12964-020-00567-0
49. Tutuncuoglu B, Cakir M, Batra J, Bouhaddou M, Eckhardt M, Gordon, et al. The Landscape of Human Cancer proteins targeted by SARS-CoV-2. Cancer Discov. (2020) 10:916–21. doi: 10.1158/2159-8290.CD-20-0559
50. Gomes MGM, Corder RM, King KJ, Langwig KE, Maior CS, Carneiro J, et al. Individual variation in susceptibility or exposure to SARS-CoV-2 lowers the herd immunity threshold. medRxiv [Preprint]. (2020). doi: 10.1101/2020.04.27.20081893
51. Jyotsana N, King MR. The impact of COVID-19 on cancer risk and treatment. Cell Mol Bioeng. (2020) 13:1–7. doi: 10.1007/s12195-020-00630-3
52. Toprani SM, Das B. Radio-adaptive response of base excision repair genes and proteins in human peripheral blood mononuclear cells exposed to gamma radiation. Mutagenesis. (2015) 30:663–76. doi: 10.1093/mutage/gev032
53. Lee PL, West C, Crain K, Wang L. Genetic polymorphisms and susceptibility to lung disease. J Negat Res Biomed. (2006) 5:5. doi: 10.1186/1477-5751-5-5
54. Devaux CA, Rolain JM, Raoult D. ACE2 receptor polymorphism: Susceptibility to SARS-CoV-2, hypertension, multi-organ failure, and COVID-19 disease outcome. J Microbiol Immunol Infect. (2020) 53:425–35. doi: 10.1016/j.jmii.2020.04.015
55. Ghafouri-Fard S, Noroozi R, Vafaee R, Branicki W, Poṡpiech E, Pyrc K, et al. Effects of host genetic variations on response to, susceptibility and severity of respiratory infections. Biomed Pharmacother. (2020) 128:110296. doi: 10.1016/j.biopha.2020.110296
56. Ovsyannikova IG, Haralambieva IH, Crooke SN, Poland GA, Kennedy RB. The role of host genetics in the immune response to SARS-CoV-2 and COVID-19 susceptibility and severity. Immunol Rev. (2020) 296:205–19. doi: 10.1111/imr.12897
57. Abdullahi IN, Emeribe AU, Ajayi OA, Oderinde BS, Amadu DO, Osuji AI. Implications of SARS-CoV-2 genetic diversity and mutations on pathogenicity of the COVID-19 and biomedical interventions. J Taibah Univer Med Sci. (2020) 15:258–64. doi: 10.1016/j.jtumed.2020.06.005
58. Fanos V, Pintus MC, Pintus R, Marcialis MA. Lung microbiota in the acute respiratory disease: from coronavirus to metabolomics. J Pediatr Neonatal Individualized Med. (2020) 9:e090139. doi: 10.7363/090139
59. da Silva TA, Fontes FL, Coutinho LG, de Souza FR, de Melo JT, de Souto JT, et al. SNPs in DNA repair genes associated to meningitis and host immune response. Mutat Res. (2011) 713:39–47. doi: 10.1016/j.mrfmmm.2011.05.012
60. Ragu S, Matos-Rodrigues G, Lopez BS. Replication stress, DNA damage, inflammatory cytokines and innate immune response. Genes (Basel). (2020) 11:409. doi: 10.3390/genes11040409
61. Guan WJ, Liang WH, Zhao Y, Liang HR, Chen ZS, Li, et al. Comorbidity and its impact on 1590 patients with COVID-19 in China: a nationwide analysis. Eur Respir J. (2020) 55:2000547. doi: 10.1183/13993003.00547-2020
62. Hanna TP, Evans GA, Booth CM. Cancer, COVID-19 and the precautionary principle: prioritizing treatment during a global pandemic. Nat Rev Clin Oncol. (2020) 17:268–70. doi: 10.1038/s41571-020-0362-6
63. Khanh NC, Thai PQ, Quach H-L, Thi N-AH, Dinh PC, Duong TN, et al. Transmission of SARS-CoV-2 during long-haul flight. Emerg Infect Dis. (2020) 26:2617–24.doi: 10.3201/eid2611.203299
64. Choi E, Chu D, Cheng P, Tsang D, Peiris M, Bausch, et al. In-flight transmission of Severe acute respiratory SARS-CoV-2. Emerg Infect Dis. (2020) 26:2713–6. doi: 10.3201/eid2611.203254
65. Silcott D, Kinahan S, Santarpia J, Silcott B, Silcott R, Silcott, et al. TRANSCOM/AMC Commercial Aircraft Cabin Aerosol Dispersion Tests. United States Transportation Command & Air Mobility Command. 1-53. Available online at: https://www.ustranscom.mil/cmd/docs/TRANSCOM%20Report%20Final.pdf (accessed October 21, 2020).
66. Freedman D, Wilder-Smith A. In-flight transmission of SARS-CoV-2: a review of the attack rates and available data on the efficacy of face masks. J Trav Med. (2020) 1–7:taaa178. doi: 10.1093/jtm/taaa178
67. Sider A. A new guide to air travel during coronavirus. The wall street journal. (18 June 2020). Available online at: https://www.wsj.com/amp/articles/the-new-rules-of-air-travel-from-masks-to-temperature-checks-11592488120 (accessed October 21, 2020).
68. DHS National Press Release (2020): TSA Prepared for Summer Travelers With Updated Security Procedures Department of Homeland Security (21 May 2020). Available online at: https://www.tsa.gov/news/press/releases/2020/05/21/tsa-prepared-summer-travelers-updated-security-procedures (accessed May 24, 2020).
69. Karatsoreos IN, Bhagat S, Bloss EB, Morrison JH, McEwen BS. Disruption of circadian clocks has ramifications for metabolism, brain behavior. PNAS. (2011) 108:1657–62. doi: 10.1073/pnas.1018375108
70. Mitrea D, Moshkenani H, Hoteiuc O, Bidian C, Toader AM, Clichici S. Antioxidant protection against cosmic radiation-induced oxidative stress at commercial flight altitude. J Physiol Pharmacol. (2018) 69. doi: 10.26402/jpp.2018.4.03
71. Cadilhac P, Bouton MC, Cantegril M, Cardines C, Gisquet A, Kaufman N, et al. In-flight ultraviolet radiation on commercial airplanes. Aerosp Med Hum Perform. (2017) 88:947–51. doi: 10.3357/AMHP.4852.2017
72. U.S. Department of Transportation, U.S. Department of Homeland Security, U.S. Department of Health and Human Services. Runway to Recovery: The United States Framework for Airlines and Airports to Mitigate the Public Health Risks of Coronavirus. Washington DC: US Department of Homeland Security (DHS.gov) (July 2020). Available online at: https://www.dhs.gov/news/2020/07/02/dhs-dot-and-hhs-issue-new-guidance-airline-industry-partners-facilitate-safe-air
Keywords: flight attendant, pilot, COVID-19, coronavirus, DNA damage repair, air travel, aviation industry, genomic integrity
Citation: Toprani SM, Scheibler C and Nagel ZD (2020) Interplay Between Air Travel, Genome Integrity, and COVID-19 Risk vis-a-vis Flight Crew. Front. Public Health 8:590412. doi: 10.3389/fpubh.2020.590412
Received: 01 August 2020; Accepted: 16 November 2020;
Published: 18 December 2020.
Edited by:
Nur A. Hasan, University of Maryland, United StatesReviewed by:
Xianding Deng, University of California, San Francisco, United StatesMarie-Cecile Genevieve Chalbot, New York City College of Technology, United States
Copyright © 2020 Toprani, Scheibler and Nagel. This is an open-access article distributed under the terms of the Creative Commons Attribution License (CC BY). The use, distribution or reproduction in other forums is permitted, provided the original author(s) and the copyright owner(s) are credited and that the original publication in this journal is cited, in accordance with accepted academic practice. No use, distribution or reproduction is permitted which does not comply with these terms.
*Correspondence: Zachary D. Nagel, znagel@hsph.harvard.edu