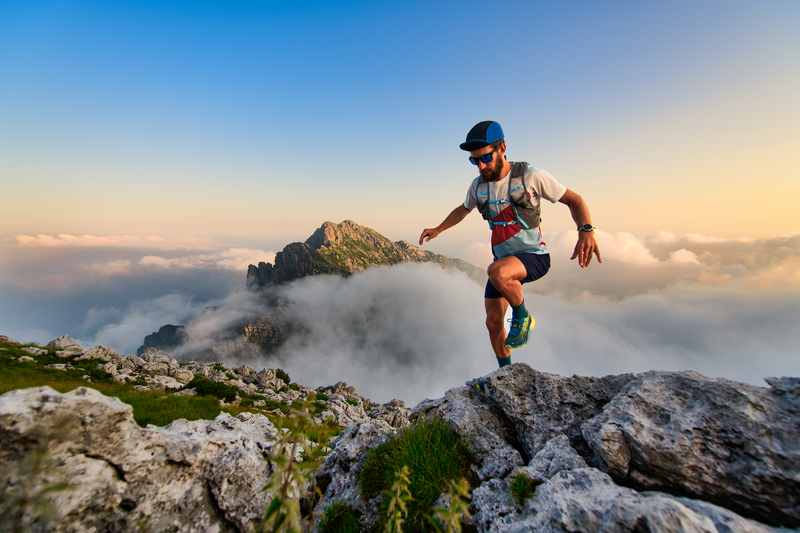
94% of researchers rate our articles as excellent or good
Learn more about the work of our research integrity team to safeguard the quality of each article we publish.
Find out more
SYSTEMATIC REVIEW article
Front. Psychiatry , 13 February 2025
Sec. ADHD
Volume 16 - 2025 | https://doi.org/10.3389/fpsyt.2025.1544816
Background/Objectives: Attention-deficit/hyperactivity disorder (ADHD) currently lacks a universally accepted biomarker or diagnostic test, underscoring the need for objective and effective assessment methods. Transcranial magnetic stimulation (TMS) has emerged as a promising tool for both assessing cortical excitability and providing therapeutic interventions. This study conducted two independent meta-analyses to evaluate: 1) the potential of TMS in assessing cortical excitability, and 2) its therapeutic efficacy in managing ADHD symptoms.
Methods: A systematic search was conducted in EMBASE, MEDLINE, PsycINFO, ClinicalTrials, and PubMed following PRISMA guidelines. The “cortical excitability” meta-analysis included studies comparing TMS-EMG or TMS-EEG neurophysiological measures between ADHD patients and healthy controls. The “therapeutic“ meta-analysis focused on randomized controlled trials (RCTs) evaluating repetitive TMS (rTMS) effects on ADHD symptoms. Standardized mean differences (SMDs) were calculated for pooled effect sizes.
Results: In the “cortical excitability” meta-analysis, 17 studies were included, demonstrating significantly reduced short-interval intracortical inhibition (SICI) in ADHD compared to healthy controls (pooled SMD = 0.65, 95% CI: 0.41–0.88, P < 0.00001). No significant differences were observed for motor evoked potentials (MEP), motor thresholds (aMT/rMT), cortical silent period (cSP), ipsilateral silent period (iSP), or intracortical facilitation (ICF). The “therapeutic“ meta-analysis, encompassing 8 samples from 7 studies, demonstrated that rTMS significantly improved ADHD symptoms compared to control conditions (pooled SMD = 0.45, 95% CI: 0.19–0.70, P = 0.0006).
Conclusions: This study highlights the potential of TMS as both a diagnostic and therapeutic tool in ADHD. Reduced SICI appears to be a key neurophysiological marker of ADHD, reflecting cortical GABAergic dysfunction. Additionally, rTMS shows promise in alleviating ADHD symptoms, though further studies are needed to confirm long-term therapeutic benefits and optimize stimulation protocols.
Systematic review registration: https://www.crd.york.ac.uk/prospero/, identifier CRD42024507867.
Attention-deficit/hyperactivity disorder (ADHD) is a common, highly heritable, and impairing condition characterized by persistent inattention, hyperactivity and impulsivity (1). ADHD’s diagnostic criteria differ between children and adults, reflecting developmental changes in symptom presentation (2). Recent studies suggested an increase in the global prevalence of ADHD, estimated to range from 6% to 7% among children (3) and around 2.5% in adults (3–5).
ADHD symptoms are mainly assessed via rating scales including Conners parent rating scale (CPRS), Conners teacher rating scale (CTRS), Conners adult ADHD rating scale-self report (CAARS), and ADHD rating scale (ADHD-RS) (6–8). However, using rating scales to assess ADHD symptoms is subjective, the reliability and validity remain limited (7, 9). Magnetic resonance imaging (MRI) has identified some brain abnormalities in ADHD (10), but findings are inconsistent across cohorts, even for different cohorts from the same research center (11). Objective hyperactivity assessments using motion-tracking systems provide quantifiable insights, yet environmental factors can affect measurement consistency (12).
Given these challenges, transcranial magnetic stimulation (TMS) emerges as a promising non-invasive technique for assessing cortical excitability, holding diagnostic and biomarker potential in ADHD. Combined with electromyography (EMG) or electroencephalography (EEG), TMS has demonstrated potential in assessing a range of neuropsychiatric disorders (13–15). In addition to its diagnostic potential, repetitive TMS (rTMS) has shown promise as a non-pharmacological treatment for ADHD (16–18).
When single-pulse TMS (spTMS) is applied to the primary motor cortex (M1), it elicits contralateral muscle activation, quantifiable through motor evoked potential (MEP) obtained by EMG (19). This procedure facilitates the determination of the resting motor threshold (rMT) and the active motor threshold (aMT), linking changes in corticospinal excitability to the dysfunction of neurotransmitters (20). Interestingly, a number of studies reported that the motor threshold (MT) and MEP in ADHD did not differ as compared to healthy controls (HCs) (21, 22). Additionally, spTMS applied to M1 can induce periods of electrical silence in muscles that are tonically contracted, both contralaterally and ipsilaterally. These are known respectively as the cortical silent period (cSP) and the ipsilateral silent period (iSP) (19). Recent investigation has elucidated that extended cSP increased cortical inhibition (23), a mechanism primarily mediated by the activation of gamma-aminobutyric type B receptors (GABABRs) and gamma-aminobutyric type A receptors (GABAARs) at different stimulus intensities (24). Similarly, the iSP sheds light on interhemispheric interactions and transcallosal-mediated inhibition. Few studies compared iSP of individuals with ADHD to HC with inconsistent results (25–28). Paired-pulse TMS (ppTMS) paradigms, which assess intracortical excitability, evaluate circuits in the human cortex associated with neurotransmitters. Short-interval intracortical inhibition (SICI) provide insights into intracortical inhibition, whereas intracortical facilitation (ICF) indicates facilitation. Notably, diminished SICI have been observed in individuals with ADHD when compared to HC (28–37), indicating potential dysfunction in GABA-mediated cortical inhibition (19, 38). Furthermore, several studies have revealed that ICF, which is associated with glutamate-mediated excitation (39), shows no significant differences between individuals with ADHD and HC (28–32, 36, 40).
The integration of TMS with EEG introduces innovative approaches for directly assessing cortical excitability. TMS-EEG evoked potentials offer a direct evaluation of cortical excitability, surpassing the specific regional limitations of TMS where it is insufficient in generating accurate proxies for cortical or cortico-spinal excitability such as MEP (41). TMS-evoked potentials (TEPs) are characterized by distinct positive (labeled with ‘P’) and negative (labeled with ‘N’) deflections in EEG recordings, elicited by the application of single TMS pulses (42). The initial response evoked by TMS is believed to originate from the stimulation of neurons localized within the targeted area, subsequently leading to the activation of regions interconnected through axonal pathways (43). TEP responses are characterized by distinct peaks at specific milliseconds after a TMS pulse, serving as markers of the inhibition-excitation balance within cortical circuits (44). To date, only a few studies have utilized the TMS-EEG approach to explore the differences in components such as P30 and N100 between individuals with ADHD and HC (21, 41, 45, 46). While P30 likely represents excitatory activity (47), N100 is suggested to reflect GABAB inhibitory activity (48).
ADHD pharmacotherapy presents variability in effectiveness, safety risks, and adherence issues, with uncertain long-term outcomes (1) and dosage effects (49), emphasizing the need for novel treatment strategies. These limitations emphasize the need for novel treatment strategies. While several studies indicate that rTMS, particularly when targeted at the dorsolateral prefrontal cortex (DLPFC), may help alleviate core symptoms like inattention and hyperactivity in ADHD patients (50, 51), the results and study parameters have been inconsistent, highlighting the need for further systematic analysis.
To comprehensively evaluate the dual role of TMS in assessing cortical excitability and its therapeutic efficacy for ADHD, we conducted a systematic review accompanied by two independent meta-analyses. For cortical excitability, our study expands upon prior research by incorporating studies published after the 2016 meta-analysis (52) and by integrating findings from TMS-EEG evaluations alongside motor cortex measures obtained with TMS-EMG. Regarding therapeutic efficacy, broadened the scope by including more studies compared to previous reviews, focusing on the overall improvement of ADHD symptoms rather than isolated cognitive functions (16, 53, 54). These comprehensive analyses offer an integrated perspective on TMS’s potential as both a diagnostic and therapeutic tool for ADHD.
Our meta-analysis was conducted in accordance with the Preferred Reporting Items for Systematic Reviews and Meta-Analyses (PRISMA) statement (55, 56). The PRISMA flow diagram is presented in Figure 1. A literature search was conducted using EMBASE, MEDLINE, PsycINFO, and PubMed with the following keywords: ((ADHD) OR (“Attention deficit and hyperactivity disorder”) OR (“Attention deficit/hyperactivity disorder”)) AND ((TMS) OR (“transcranial magnetic stimulation”)). Three authors (Yu Han, Zi-Yu Wei, and Zi-Jian Feng) conducted an initial search (last search: February 2024) and assessed eligibility independently. Subsequently, two authors (Yu Han and Zi-Yu Wei) independently double-checked the extracted data, and each dataset was independently confirmed by both authors. The protocol was registered in the International Prospective Register of Systematic Reviews (https://www.crd.york.ac.uk/prospero/) with registration number CRD42024507867.
The inclusion criteria were as follows: For “cortical excitability” cohort, studies were eligible if they met the following requirements: 1) involved TMS studies of ADHD patients; 2) utilized TMS for investigating cortical excitability; 3) included comparisons of TMS-EMG–derived neurophysiological measures, such as MEP, MT, SICI, ICF, cSP, iSP, and TMS-EEG–derived TEP at rest between ADHD patients and HCs; and 4) included HCs. For “therapeutic” cohort, studies were eligible if they met the following requirements: 1) involved TMS studies of ADHD patients; 2) applied TMS as a treatment for ADHD; 3) compared outcomes derived from standardized tests pre- and post-TMS intervention; and 4) included all study types in the narrative synthesis, with only RCTs were included in the meta-analysis.
The exclusion criteria were as follows: 1) animal studies; 2) studies not targeting ADHD as the primary condition; 3) review articles; 4) articles not written in English; 5) studies without full-text availability; and 6) meta-analyses with unavailable or unextractable raw data from figures and tables. Additionally, the reference lists of previously published articles, reviews, and meta-analyses were searched to identify further relevant articles.
For the “cortical excitability” cohort, the following variables were collected: name of the first author, publication year, number of participants, sex distribution, and data of MEP, rMT, aMT, SICI, ICF, cSP, iSP and TEP (mean ± standard deviation) for meta-analysis. If the values of these variables were not explicitly provided in the articles, they were extracted using Web Plot Digitizer (https://automeris.io/WebPlotDigitizer/) from the relevant graphs. Since different studies used varying inter-stimulus intervals (ISIs) for the same TMS paradigms (e.g. 1-6 ms for SICI, 7-20 ms for ICF), these differences were disregarded, provided the ISI was consistent between ADHD and HC groups within the same study. For the “therapeutic” cohort, data collected included the name of the first author, publication year, number of participants, sex distribution, TMS parameters, outcome measures and data of the primary outcome (mean ± standard deviation) for meta-analysis. Given the use of various scales to measure primary outcomes in these studies, standardized mean differences (SMDs) were calculated to facilitate comparison across studies. When there were sham-controlled and active-controlled datasets in a study at the same time, we chose the sham-controlled datasets for meta-analysis. All of the variables were acquired independently by Yu Han and Zi-Yu Wei, discrepancies between these two authors were resolved through consultation with Zi-Jian Feng.
We used the Risk of Bias Assessment Tool for Nonrandomized Studies (57) for “cortical excitability” studies. The following domains were evaluated: selection of participants, confounding variables, measurement of exposure, blinding of outcome assessments, incomplete outcome data and selective outcome reporting. Cochrane risk-of-bias tool for RCTs of “therapeutic” studies was used. The following domains were evaluated: random sequence generation (selection bias), Allocation concealment (selection bias), blinding of patients and personnel (performance bias), blinding of outcome assessment (detection bias), incomplete outcome data (attrition bias), selective reporting (reporting bias), other bias. Yu Han and Zi-Yu Wei performed risk of bias assessment, independently. Discrepancies between these two authors were resolved through consultation with Zi-Jian Feng.
We performed meta-analyses using the Review Manager software (RevMan 5.4; Cochrane Collaboration). SMDs with two-sided 95% confidence interval (CI) employing the inverse variance statistical method were used to demonstrate the differences in neurophysiological measurements measured by TMS paradigms between ADHD and HC groups for ‘cortical excitability’ studies as well as the effect of rTMS on ADHD for ‘therapeutic’ studies. Effect sizes were classified as small (SMD = 0.2), moderate (SMD = 0.5), and large (SMD = 0.8) (58). To assess heterogeneity, we applied the Chi-squared test and the I2 statistic. A fixed-effects model was used when I2 was less than 50%, whereas a random-effects model was adopted when I2 ≥ 50%, we performed sensitivity analyses using the leave-one-out method to evaluate the robustness of the results (59). The funnel plot (60) and Egger’s test (61) were used to evaluate possible publication bias if more than 10 articles were available for this meta-analysis.
We conducted a subgroup analysis from one perspective for the “cortical excitability” cohort, that is population (adults vs. children and adolescents) for each neurophysiological measurements except aMT for only adult studies included and from five perspectives for the “therapeutic” cohort: population (adults vs. children and adolescents), stimulation targets (right prefrontal cortex (rPFC) vs. left prefrontal cortex (lPFC)), coil types (figure of 8 vs. H5/H6), outcome measures (Conners’ Adult ADHD Rating Scales (CAARS) vs. others), and number of sessions (< 20 vs. ≥ 20).
The PRISMA diagram is shown in Figure 1. We identified 1189 records from databases and registers (PubMed = 263, Web of Science = 232, Embase = 528, Medline = 146, ClinicalTrails = 20). No additional records were identified through reference lists of other studies or reviews. After duplicates removed, 699 records were left. After screen titles and abstracts, 643 records were excluded with reasons, the remaining reports which were seek for retrieval were 56. The full-text of 3 records couldn’t be retrieved. After full-text screening, 21 records were excluded with reasons and there were 21 studies and 11 studies left in qualitative synthesis for “cortical excitability” cohort and “therapeutic” cohort, respectively. Finally, there were 17 records and 8 records left in quantitative synthesis for “cortical excitability” cohort and “therapeutic” cohort, respectively. All studies for “cortical excitability” are summarized in Table 1 (21, 22, 25–37, 40, 41, 45, 46, 62, 63), Datasets of 7, 15, 5, 8, 3, 3, 9, and 5 articles were available for further analyses for MEP, rMT, aMT, cSP, iSP latency, iSP duration, SICI and ICF, respectively. All studies for “therapeutic” are summarized in Table 2 (50, 51, 64–70). For one study, there were only active-controlled datasets (69), we chose atomoxetine (ATX) datasets as control group and rTMS-ATX datasets as experimental group.
The risk of bias of the included studies is shown in Figure 2, Figure 3 and Table 3. For “cortical excitability” studies: for ‘selection of participants’, the risk of bias was high for 1 study which excluded too many participants; for ‘confounding variables’, the risk of bias was unclear for 3 studies which had inadequate characteristics of participants; for ‘measurement of exposure’, ‘blinding of outcome assessments’, ‘incomplete outcome data’ and ‘selective outcome reporting’, the risk of bias was low for all of the studies included. For ‘therapeutic’ studies, there are 8 RCTs evaluated, the results are: for ‘random sequence generation (selection bias)’, the risk of bias was unclear for 1 study which didn’t mention the random method; for ‘Allocation concealment (selection bias)’, the risk of bias was unclear for 4 studies which didn’t mention the concealment method; for ‘blinding of patients and personnel (performance bias)’, the risk of bias was high for 5 studies which were no- or single-blinded and the risk of bias was unclear for 1 study for inadequate information; for ‘blinding of outcome assessment (detection bias)’, the risk of bias was unclear for 1 study which had inadequate information; for ‘incomplete outcome data (attrition bias)’ and ‘selective reporting (reporting bias)’, all studies had low risk of bias; for ‘other bias’, the risk of bias was high for 1 study which received funding from a private company.
Figure 2. Risk of bias graph for RCTs of “therapeutic” studies: review authors’ judgements about each risk of bias item presented as percentages across all included studies.
Figure 3. Risk of bias summary for RCTs of “therapeutic” studies: review authors’ judgements about each risk of bias item for each included study.
Table 3. Risk of bias summary for nonrandomized studies of “cortical excitability” studies: review authors’ judgements about each risk of bias item for each included study.
We employed single-pulse and paired-pulse TMS protocols in ADHD populations to investigate TMS-derived measures that reflect potential differences between ADHD patients and HCs. The primary objective was to identify specific measures that differentiate ADHD from HC groups. Due to the limited number of studies included in the meta-analysis, a subgroup analysis was conducted solely based on population characteristics (adults vs. children and adolescents). The results of this analysis are provided in the Supplementary Materials for reference (See Supplementary Material).
There are 9 studies comparing MEP between ADHD and HC groups (21, 25, 27, 29–31, 33, 35, 40), all of which consistently indicated no significant differences in MEPs between the two groups. Furthermore, a meta-analysis incorporating 7 (21, 25, 29–31, 35, 40) of these studies calculated the pooled standardized mean difference (SMD) for MEPs as 0.05 (95% CI: −0.15 to 0.24; P = 0.63) (Figure 4), suggesting that MEP does not significantly differ in individuals with ADHD compared to HC groups.
Figure 4. Forest plot of standard mean difference (SMD) comparing motor evoked potential (MEP) of attention-deficit/hyperactivity disorder (ADHD) and HC (healthy control). The size of the green box reflects how much weight each study received in the meta-analysis. Black bars represent the 95% CI for the SMD in each study. CI, confidence interval; IV, inverse variance; ADHD, attention-deficit/hyperactivity disorder; SMD, standard mean difference.
There are 17 studies comparing rMT between ADHD and HC groups, 15 of which indicated no significant differences (21, 22, 25–29, 31, 32, 35–37, 40, 46, 63). However, two studies (30, 62) reported a decreased rMT in individuals with ADHD compared to HC group. Among these studies, 15 (21, 22, 25, 28–33, 35–37, 40, 46, 63) of them are included in the meta-analysis yielded a pooled SMD of 0.06 (95% CI: −0.07 to 0.20; P = 0.36) (Figure 5), suggesting no overall change in rMT for ADHD compared to HCs.
Figure 5. Forest plot of standard mean difference (SMD) comparing resting motor threshold (rMT) of attention-deficit/hyperactivity disorder (ADHD) and HC (healthy control). The size of the green box reflects how much weight each study received in the meta-analysis. Black bars represent the 95% CI for the SMD in each study. CI, confidence interval; IV, inverse variance; ADHD, attention-deficit/hyperactivity disorder; SMD, standard mean difference.
There are 6 studies comparing aMT between ADHD and HC groups, all of which indicated that there were no differences between the groups (26, 28, 30–32, 36). 5 (28, 30–32, 36) of these studies were included in a meta-analysis for aMT, yielding a pooled SMD of -0.01 (95% CI: −0.20 to 0.18; P = 0.93) (Supplementary Figure 1), which indicated that aMT did not differ in individuals with ADHD compared to HC groups.
There are 8 studies comparing cSP between ADHD and HC groups. 7 of these studies found no differences between the groups (25, 27, 28, 30–32, 36), while the remaining study observed an increase in cSP in ADHD relative to HC (63). All of these studies were included in a meta-analysis for cSP, which yielded a pooled SMD of -0.09 (95% CI: −0.26 to 0.08; P = 0.29) (Figure 6), indicating that cSP cannot distinguish ADHD from HC.
Figure 6. Forest plot of standard mean difference (SMD) comparing cortical silent period (cSP) of attention-deficit/hyperactivity disorder (ADHD) and HC (healthy control). The size of the green box reflects how much weight each study received in the meta-analysis. Black bars represent the 95% CI for the SMD in each study. CI, confidence interval; IV, inverse variance; ADHD, attention-deficit/hyperactivity disorder; SMD, standard mean difference.
There are 4 studies comparing iSP between ADHD and HC groups (25–28). 2 studies reported an increase in iSP latency in ADHD compared with HC (25, 28), and 1 study observed a decrease in iSP latency with age in the control group but not in the ADHD group (26). However, 1 study found no difference in iSP latency (27). Additionally, 2 studies noted a decrease in iSP duration in ADHD relative to HC (25, 27), while 2 studies observed no differences (26, 28). A meta-analysis including 3 (25, 27, 28) of these studies for iSP duration yielded a pooled SMD of -1.24 (95% CI: −2.44 to -0.04; P = 0.04) (Supplementary Figure 2), indicating that ADHD patients have a shorter iSP duration compared to HCs. Another meta-analysis for iSP latency, also including 3 studies, showed an SMD of 0.45 (95% CI: -0.17 to 1.08; P = 0.15) (Supplementary Figure 3), suggesting that iSP latency cannot reliably distinguish between ADHD and HC groups.
There are 12 studies comparing SICI between ADHD and HC groups (28–37, 40, 63). 10 of which indicated that SICI was decreased in the ADHD groups compared to the HCs (28–37). 2 studies found no difference (40, 63). 9 (28, 30–36, 63) of these studies were included in a meta-analysis for SICI, yielding a pooled SMD of 0.65 (95% CI: 0.41 to 0.88; P < 0.00001) (Figure 7), indicating a significant reduction of SICI in ADHD compared to HCs.
Figure 7. Forest plot of standard mean difference (SMD) comparing short-interval intracortical inhibition (SICI) of attention-deficit/hyperactivity disorder (ADHD) and HC (healthy control). The size of the green box reflects how much weight each study received in the meta-analysis. Black bars represent the 95% CI for the SMD in each study. CI, confidence interval; IV, inverse variance; ADHD, attention-deficit/hyperactivity disorder; SMD, standard mean difference.
There are 9 studies comparing ICF between ADHD and HC groups (28–32, 36, 37, 40, 63). 7 of these studies found no differences in ICF between the two groups (28, 30–32, 36, 37, 40). 1 study reported a decrease in ICF in the ADHD group (29), while another observed an increase (63). Among these, 5 studies were included in a meta-analysis for ICF, which reported a pooled SMD of 0.20 (95% CI: -0.00 to 0.41; p = 0.05) (Supplementary Figure 4), suggesting that ICF does not effectively distinguish between ADHD and HC.
This section provides a systematic review of the therapeutic efficacy of rTMS in both children and adults with ADHD. Additionally, a meta-analysis was conducted on RCTs to quantitatively evaluate the effects of rTMS on ADHD symptoms. Given the limited number of studies included in the meta-analysis, detailed subgroup analyses including population, stimulation targets, coil types, outcome measures, and session numbers were not presented in the main text. Instead, the results of these analyses are provided in the Supplementary Materials for reference (See Supplementary Material).
5 rTMS clinical trials have been conducted on children and adolescents with ADHD (51, 65, 66, 69, 71). 4 of these trials were blinded RCTs (51, 65, 69, 71), while 1 was an open-label trial without controls (66). Weaver et al. (2012) found no differences between the active and sham groups on the Clinical Global Impression-Improvement Scale (CGI-I) and ADHD-IV scale, although scores for the active group decreased significantly from baseline (65). Cao et al. (2018) found significant improvements across all groups on several metrics, including the Swanson, Nolan, and Pelham Version IV (SNAP-IV) questionnaire, Continuous Performance Test (CPT), Wechsler Intelligence Scale for Children (WISC), and Iowa Gambling Tasks (IGT), compared to baseline. Notably, this study found no superior effectiveness of rTMS-ATX over ATX (69). Building on these findings, Cao et al. (2019) expanded their research to explore the specific effects of rTMS in a similar cohort. After six weeks of treatment, only the real rTMS group showed a significant reduction in SNAP-IV scores from baseline, a result not mirrored in the sham rTMS group. Unlike the 2018 study, the 2019 investigation did not evaluate the comparative effectiveness of rTMS and ATX directly (51). The clinical trial NCT03663179 (71) was a parallel, double-blinded RCT with no conclusion, but it has data results published online (https://clinicaltrials.gov/study/NCT03663179?tab=results) and advanced into further meta-analysis. Gómez et al. (2014) found a significant improvement in the Symptoms Checklist (SCL) for ADHD from DSM-IV scores relative to baseline (66).
4 rTMS clinical trials (50, 64, 67, 68) and 2 case reports (70, 72) have been conducted on adults with ADHD. The studies explored various treatment protocols, with mixed findings on therapeutic efficacy. In the study of Bloch et al. (2010), a significant enhanced scores on the Positive and Negative Affect Scale (PANAS) and Visual Analogue Scales (VASs) compared to sham treatments were founded (64). Similarly, Paz et al. (2018) reported improvements in Conners’ Adult ADHD Rating Scale (CAARS) scores after treatment, yet no significant differences were found between the treatment and sham groups (67). Further trials by Alyagon et al. (2020) showed that only the participants receiving real rTMS demonstrated significant improvement in CAARS scores (68). Bleich-Cohen et al. (2021) found notable improvements in the CAARS inattention/memory subscale for the right PFC group (50). The case studies added depth to these findings: Niederhofer (2012) reported successful reduction in hyperactivity and medication dosage with minimal rTMS at 1-Hz over 21 sessions (70), while Ustohal et al. (2012) encountered severe side effects from 10-Hz stimulation on the right DLPFC, necessitating a switch to the left DLPFC which ultimately resulted in improved clinical scores (72). These collective results underscore the potential of rTMS as a treatment for ADHD in adults, although they also highlight the variability in patient response. Overall, 8 datasets from 7 studies were included in the meta-analysis of therapeutic effect of rTMS on ADHD (50, 51, 64, 67–69).
The analysis demonstrated that rTMS led to a significant improvement in overall ADHD symptoms, with a standardized mean difference (SMD) of 0.45 (95% CI: 0.19 to 0.70; P = 0.0006) (Figure 8).
Figure 8. Forest plot of standard mean difference (SMD) comparing therapeutic effects of rTMS on attention-deficit/hyperactivity disorder (ADHD) and control condition on ADHD. The size of the green box reflects how much weight each study received in the meta-analysis. Black bars represent the 95% CI for the SMD in each study. CI, confidence interval; IV, inverse variance; ADHD, attention-deficit/hyperactivity disorder; SMD, standard mean difference.
There are 15 studies included in meta-analysis of rMT, Visual inspection of the funnel plot of rMT didn’t indicate an asymmetry in the shape (Supplementary Figure 5). Egger’s test also didn’t show a significant publication bias for rMT (P = 0.302). For meta-analyses of iSP latency, iSP duration, and SICI, I2 was ≥ 50% (64%, 88%, and 51%, respectively), No single study contributed to the heterogeneity of SICI when removed included studies one by one, indicating that the result of SICI obtained was robust and credible. For iSP duration, two studies were found to contribute to the heterogeneity (25, 27). For iSP latency, one study was found to contribute to the heterogeneity (27). Note that, there were only 3 studies included in the meta-analysis of iSP duration and latency, respectively.
This systematic review evaluated the utility of TMS in assessing cortical excitability and its therapeutic efficacy in patients with ADHD. A total of 21 original studies investigating TMS-derived neurophysiological measures of cortical excitability and 11 studies evaluating the therapeutic effects of rTMS on ADHD symptoms were identified.
In the meta-analysis of “cortical excitability”, 17 studies were included. The findings revealed a significant reduction in SICI in ADHD patients compared to HCs, suggesting potential GABAergic dysfunction. However, no significant differences were observed for MEP, motor thresholds (aMT/rMT), cSP, iSP, or ICF. The meta-analysis of “therapeutic”, which included 8 samples from 7 studies, demonstrated that rTMS significantly improved overall ADHD symptoms compared to control conditions.
Subgroup analyses highlighted specific findings, including reduced rMT (n = 3) and shortened iSP duration (n = 1) in adults, as well as prolonged iSP latency (n = 2) and enhanced ICF (n = 4) in children. However, due to the limited sample sizes, these analyses were conducted for reference purposes only (Supplementary Figures 7, 9, 10, 12). Additionally, stimulation target of the left prefrontal cortex (n = 5) and the use of figure-of-8 coils (n = 4) were associated with potential therapeutic advantages (Supplementary Figures 14, 15). However, significant heterogeneity across studies limits the generalizability of these findings.
MEP are electrical signals captured from descending motor pathways or directly from muscles following stimulation of the brain’s motor pathways. These potentials primarily serve to gauge the excitability of neurons within theM1 that correlate with the targeted muscle, as well as the excitability of motor neurons in the brainstem or spinal cord. This is quantified using the rMT and aMT, which measure cortical excitability at different stimulus thresholds (23). Pharmacological research underscores that MT reflects the excitability of the membrane in corticospinal neurons and is influenced by short-term glutamatergic AMPA transmission, indicating a direct link between neurotransmitter activity and motor threshold variability (73). According to our meta-analysis, which reviewed studies employing MEP, rMT, and aMT, there are no substantial differences between ADHD and HC groups across these indicators. This is corroborated by similar findings in a range of neurological assessments where MEPs did not differentiate between ADHD and normative profiles (21, 31, 33), as well as rMT (28, 35, 63) and aMT (26, 30, 32). In other neurological disorders, such as stroke, MEPs are considered to have potential prognostic utility (74). Studies indicate that the absence of upper limb MEPs is a strong predictor of poor motor recovery and overall negative outcomes (75). Yet, the current meta-analytical results imply that the utility of MEP, rMT, and aMT as diagnostic tools in ADHD is limited, reflecting the complex neurobiology of ADHD that may not significantly alter motor cortex excitability as measured by these thresholds.
In addition, given that a sufficient number (N = 15) of studies were included in rMT meta-analysis, we conducted a subgroup analysis based on age (adults VS. children and adolescents). It revealed no significant differences in rMT between ADHD and HC groups among children and adolescents (SMD: 0.00; 95% CI: -0.15 to 0.15; P = 0.97), whereas a significant difference was observed in adults (SMD: 0.41; 95% CI: 0.06 to 0.76; P = 0.02) (Supplementary Figure 6), indicating variability in rMT changes with age. This suggests a possible age-related modulation of neurophysiological responses in ADHD, which might not be captured in younger populations. However, it is worth noting that there were only three studies in the adult group, which significantly affects the robustness of this conclusion.
The duration of the cSP is influenced by various factors, including the direction of the TMS current, muscle contraction level, and the intensity of the stimulus (76, 77). These variables can complicate comparisons across studies. For instance, Orth and Rothwell (2004) demonstrated that the cSP duration is longer with anterior-to-posterior directed currents compared to posterior-to-anterior currents, indicating that methodological differences could account for variability in cSP findings. Given the complexity of cSP and its modulation by various neuromodulators beyond GABA, including dopaminergic transmission (73, 78), the absence of cSP duration differences between ADHD and HCs may indicate that ADHD-related neurophysiological changes do not substantially impact GABAergic inhibition as measured by cSP. Alternatively, it may reflect compensatory mechanisms in the motor cortex that maintain normal cSP durations despite underlying neurochemical changes in ADHD.
Unlike cSP, which reflects both cortical and spinal inhibitory circuits, the iSP primarily reflects transcallosal inhibition processes with minimal spinal influence (79), making it a potentially more reliable marker for cortical inhibition. Our meta-analysis identified a significant reduction in iSP duration in ADHD patients compared to HCs. This finding suggests that iSP could be valuable for understanding inhibitory deficits in ADHD, although the limited number of studies (n = 3) included in this meta-analysis warrants cautious interpretation.
Measuring the SICI/ICF provides an estimate of the relative strengths of local intracortical inhibitory and excitatory activities, thereby offering insights into cortical excitability and inhibitory control mechanisms in ADHD. Cortical hyperexcitability in ADHD may be directly related to dysfunctions in inhibitory GABAergic interneurons (80). These interneurons are crucial for generating precise oscillatory rhythms that coordinate the timing of pyramidal cell firing, which is essential for maintaining appropriate cortical excitability and overall cortical function (81).
Imaging studies comparing SICI with magnetic resonance spectroscopy data have identified an inverse correlation between SICI and GABA concentrations in the motor cortex of children (33). Interestingly, in ADHD, this relationship between GABA and SICI appears anomalous at rest but normalizes during tasks, while MRS has also shown reduced glutamate and glutamine levels in ADHD patients (62). Conversely, our meta-analysis indicated that ICF was elevated in ADHD compared to HCs, an outcome differing from MRS findings and suggesting complexity in the excitatory/inhibitory balance in ADHD.
SICI has shown acceptable test-retest reliability across both healthy and clinical populations, reinforcing its utility as a stable measure (82–86). For example, in Tourette syndrome (TS), SICI has correlated reliably with ADHD-related hyperactivity scores (87). However, no studies have specifically assessed SICI test-retest reliability in ADHD patients. It’s also important to note that while reduced SICI is common across various psychiatric and neurological disorders, including Alzheimer’s (88), schizophrenia (89), major depression disorder (MDD) (90), increased SICI has only been observed in functional neurological (paretic) disorders (74). Thus, using SICI as a standalone diagnostic biomarker for ADHD is not feasible and would require complementary diagnostic tools.
Meta-analysis was not conducted for TMS-EEG studies because of heterogeneity among the methodologies and study characteristics. Four studies compared TEPs between ADHD and HC groups. Bruckmann et al. (2012) reported significantly reduced N100 amplitude at rest in ADHD, with a tendency toward shorter N100 latency (46), while D’Agati et al. (2014) found no N100 differences between groups (21). Hadas et al. (2021) found significantly reduced P30 amplitude correlated with ADHD severity (41), while Avnit et al. (2023) reported a reduced area under the rectified curve (AURC) for TEPs in ADHD but did not specify individual components (45). Further research with consistent protocols is needed to clarify TEP alterations in ADHD.
Our meta-analysis revealed a significant improvement in ADHD symptoms with active TMS compared to sham. However, due to the limited number of samples (n = 8), these results should be interpreted cautiously.
Most studies targeted the prefrontal cortex without individualized localization, typically using the 5-cm method or F3 position (50, 51, 68). There is evidence that individualized MRI-guided TMS, such as functional connectivity-guided targeting of the DLPFC, can improve treatment precision in conditions like depression (91–93), suggesting that individualized precision targeting could potentially improve the therapeutic outcomes of rTMS. The dorsal anterior cingulate cortex (dACC) has been consistently identified as an abnormal brain region in individuals with ADHD (94, 95). In our previous study, we stimulated the DLPFC guided by dACC functional connectivity to modulate the local activity of the dACC in healthy participants. Our findings revealed that rTMS significantly decreased local activity in the dACC (96). Additionally, stronger dACC-DLPFC functional connectivity was associated with a more pronounced effect on dACC activity. The cingulo-frontal-parietal cognitive-attention network is a core impaired network in ADHD. Given that the cingulo-frontal-parietal (CFP) network is critical to cognitive attention and consistently implicated in ADHD (97), further studies applying dACC-guided DLPFC stimulation via fMRI connectivity in ADHD are warranted to assess this approach’s efficacy.
This study has several limitations that should be acknowledged. First, the sample sizes of the included studies were relatively small, particularly for subgroup analyses, which may limit the statistical power and generalizability of the findings. For example, while age-based subgroup analyses provided some insights into differences between children, adolescents, and adults, the small number of studies in each subgroup warrants cautious interpretation.
Second, significant heterogeneity was observed across studies, particularly in the therapeutic efficacy meta-analysis. This heterogeneity likely reflects variability in TMS protocols, such as stimulation targets, coil types, stimulation parameters, and the number of sessions, which complicates direct comparisons and limits the reliability of pooled effect sizes.
Third, the included studies used a wide range of outcome measures to assess ADHD symptoms and neurophysiological markers, which may contribute to inconsistencies in the findings. For instance, the reliance on standardized mean differences to account for variations in measurement scales introduces potential bias due to differences in effect size reporting.
Fourth, publication bias cannot be ruled out, as studies with significant findings are more likely to be published than those with null results. While funnel plots and Egger’s tests were conducted to assess this, the limited number of studies may reduce the robustness of these assessments.
Finally, most of the included studies were cross-sectional or had short follow-up periods, limiting the ability to evaluate the long-term effects and sustainability of TMS interventions in ADHD.
Future longitudinal studies with larger sample sizes and standardized TMS protocols are needed to confirm these findings and explore the potential of TMS as a diagnostic and therapeutic tool for ADHD.
This systematic review and meta-analysis provide evidence supporting the potential utility of TMS in both assessing cortical excitability and improving symptoms in patients with ADHD. The findings reveal that SICI is significantly reduced in ADHD patients, suggesting GABAergic dysfunction as a neurophysiological marker of the disorder. Furthermore, rTMS demonstrates significant therapeutic efficacy in alleviating overall ADHD symptoms, with potential advantages observed for specific stimulation parameters and target regions.
Despite these promising results, the limited sample sizes, heterogeneity across studies, and variability in methodologies highlight the need for further research. Future studies should focus on larger, well-designed trials with standardized protocols to confirm these findings and explore the long-term effects of TMS interventions. Overall, this study underscores the emerging role of TMS as a valuable tool in both the diagnosis and treatment of ADHD.
The original contributions presented in the study are included in the article/Supplementary Material. Further inquiries can be directed to the corresponding authors.
YH: Writing – original draft. Z-YW: Writing – original draft. NZ: Writing – review & editing. QZ: Writing – review & editing. HZ: Writing – review & editing. H-LF: Writing – review & editing. Y-FZ: Writing – review & editing. Z-JF: Writing – review & editing.
The author(s) declare financial support was received for the research, authorship, and/or publication of this article. This research was funded by Pioneer and Leading Goose R&D Program of Zhejiang (No. 2023C03002), NSFC (82071537, 32200904).
The authors declare that the research was conducted in the absence of any commercial or financial relationships that could be construed as a potential conflict of interest.
The handling editor LY declared a past co-authorship with the author Y-FZ.
The author(s) declare that no Generative AI was used in the creation of this manuscript.
All claims expressed in this article are solely those of the authors and do not necessarily represent those of their affiliated organizations, or those of the publisher, the editors and the reviewers. Any product that may be evaluated in this article, or claim that may be made by its manufacturer, is not guaranteed or endorsed by the publisher.
The Supplementary Material for this article can be found online at: https://www.frontiersin.org/articles/10.3389/fpsyt.2025.1544816/full#supplementary-material
1. Posner J, Polanczyk GV, Sonuga-Barke E. Attention-deficit hyperactivity disorder. Lancet. (2020) 395:450–62. doi: 10.1016/S0140-6736(19)33004-1
2. Association AP. Diagnostic and statistical manual of mental disorders. fifth edition. Arlington, VA: American Psychiatric Association Publishing (2013). p. 191.
3. Giannoulis SV, Muller D, Kennedy JL, Goncalves V. Systematic review of mitochondrial genetic variation in attention-deficit/hyperactivity disorder. Eur Child Adolesc Psychiatry. (2024) 33:16750–85. doi: 10.1007/s00787-022-02030-6
4. Faraone SV, Asherson P, Banaschewski T, Biederman J, Buitelaar JK, Ramos-Quiroga JA, et al. Attention-deficit/hyperactivity disorder. Nat Rev Dis Primers. (2015) 1:15020. doi: 10.1038/nrdp.2015.20
5. Sayal K, Prasad V, Daley D, Ford T, Coghill D. ADHD in children and young people: prevalence, care pathways, and service provision. Lancet Psychiatry. (2018) 5:175–86. doi: 10.1016/S2215-0366(17)30167-0
6. Hirsch O, Christiansen H. Factorial structure and validity of the quantified behavior test plus (Qb+(c)). Assessment. (2017) 24:1037–49. doi: 10.1177/1073191116638426
7. Mayfield AR, Parke EM, Barchard KA, Zenisek RP, Thaler NS, Etcoff LM, et al. Equivalence of mother and father ratings of ADHD in children. Child Neuropsychol. (2018) 24:166–83. doi: 10.1080/09297049.2016.1236186
8. Reh V, Schmidt M, Lam L, Schimmelmann BG, Hebebrand J, Rief W, et al. Behavioral assessment of core ADHD symptoms using the qbTest. J Atten Disord. (2015) 19:1034–45. doi: 10.1177/1087054712472981
9. Sollie H, Larsson B, Morch WT. Comparison of mother, father, and teacher reports of ADHD core symptoms in a sample of child psychiatric outpatients. J Atten Disord. (2013) 17:699–710. doi: 10.1177/1087054711436010
10. Bhullar A, Kumar K, Anand A. ADHD and neuropsychology: developmental perspective, assessment, and interventions. Ann Neurosci. (2023) 30:5–7. doi: 10.1177/09727531231171765
11. Wang JB, Zheng LJ, Cao QJ, Wang YF, Sun L, Zang YF, et al. Inconsistency in abnormal brain activity across cohorts of ADHD-200 in children with attention deficit hyperactivity disorder. Front Neurosci. (2017) 11:320. doi: 10.3389/fnins.2017.00320
12. Wang XQ, Albitos PJ, Hao YF, Zhang H, Yuan LX, Zang YF. A review of objective assessments for hyperactivity in attention deficit hyperactivity disorder. J Neurosci Methods. (2022) 370:109479. doi: 10.1016/j.jneumeth.2022.109479
13. Brighina F, Raieli V, Messina LM, Santangelo G, Puma D, Drago F, et al. Non-invasive brain stimulation in pediatric migraine: A perspective from evidence in adult migraine. Front Neurol. (2019) 10:364. doi: 10.3389/fneur.2019.00364
14. Jannati A, Ryan MA, Kaye HL, Tsuboyama M, Rotenberg A. Biomarkers obtained by transcranial magnetic stimulation in neurodevelopmental disorders. J Clin Neurophysiol. (2022) 39:135–48. doi: 10.1097/WNP.0000000000000784
15. Tsuboyama M, Lee Kaye H, Rotenberg A. Biomarkers obtained by transcranial magnetic stimulation of the motor cortex in epilepsy. Front Integr Neurosci. (2019) 13:57. doi: 10.3389/fnint.2019.00057
16. Chen YH, Liang SC, Sun CK, Cheng YS, Tzang RF, Chiu HJ, et al. A meta-analysis on the therapeutic efficacy of repetitive transcranial magnetic stimulation for cognitive functions in attention-deficit/hyperactivity disorders. BMC Psychiatry. (2023) 23:756. doi: 10.1186/s12888-023-05261-2
17. Fitzgerald PB. Targeting repetitive transcranial magnetic stimulation in depression: do we really know what we are stimulating and how best to do it? Brain Stimul. (2021) 14:730–6. doi: 10.1016/j.brs.2021.04.018
18. Khaleghi A, Zarafshan H, Vand SR, Mohammadi MR. Effects of non-invasive neurostimulation on autism spectrum disorder: A systematic review. Clin Psychopharmacol Neurosci. (2020) 18:527–52. doi: 10.9758/cpn.2020.18.4.527
19. Lefaucheur JP. Transcranial magnetic stimulation. Handb Clin Neurol. (2019) 160:559–80. doi: 10.1016/B978-0-444-64032-1.00037-0
20. Mimura Y, Nishida H, Nakajima S, Tsugawa S, Morita S, Yoshida K, et al. Neurophysiological biomarkers using transcranial magnetic stimulation in Alzheimer’s disease and mild cognitive impairment: A systematic review and meta-analysis. Neurosci Biobehav Rev. (2021) 121:47–59. doi: 10.1016/j.neubiorev.2020.12.003
21. D’Agati E, Hoegl T, Dippel G, Curatolo P, Bender S, Kratz O, et al. Motor cortical inhibition in ADHD: modulation of the transcranial magnetic stimulation-evoked N100 in a response control task. J Neural Transm (Vienna). (2014) 121:315–25. doi: 10.1007/s00702-013-1097-7
22. Ewen JB, Puts NA, Mostofsky SH, Horn PS, Gilbert DL. Associations between task-related modulation of motor-evoked potentials and EEG event-related desynchronization in children with ADHD. Cereb Cortex. (2021) 31:5526–35. doi: 10.1093/cercor/bhab176
23. Rossini PM, Burke D, Chen R, Cohen LG, Daskalakis Z, Di Iorio R, et al. Non-invasive electrical and magnetic stimulation of the brain, spinal cord, roots and peripheral nerves: Basic principles and procedures for routine clinical and research application. An updated report from an I.F.C.N. Committee. Clin Neurophysiol. (2015) 126:1071–107. doi: 10.1016/j.clinph.2015.02.001
24. Kimiskidis VK, Papagiannopoulos S, Kazis DA, Sotirakoglou K, Vasiliadis G, Zara F, et al. Lorazepam-induced effects on silent period and corticomotor excitability. Exp Brain Res. (2006) 173:603–11. doi: 10.1007/s00221-006-0402-1
25. Buchmann J, Wolters A, Haessler F, Bohne S, Nordbeck R, Kunesch E. Disturbed transcallosally mediated motor inhibition in children with attention deficit hyperactivity disorder (ADHD). Clin Neurophysiol. (2003) 114:2036–42. doi: 10.1016/S1388-2457(03)00208-6
26. Garvey MA, Barker CA, Bartko JJ, Denckla MB, Wassermann EM, Castellanos FX, et al. The ipsilateral silent period in boys with attention-deficit/hyperactivity disorder. Clin Neurophysiol. (2005) 116:1889–96. doi: 10.1016/j.clinph.2005.03.018
27. Hoeppner J, Wandschneider R, Neumeyer M, Gierow W, Haessler F, Herpertz SC, et al. Impaired transcallosally mediated motor inhibition in adults with attention-deficit/hyperactivity disorder is modulated by methylphenidate. J Neural Transm (Vienna). (2008) 115:777–85. doi: 10.1007/s00702-007-0008-1
28. Wu SW, Gilbert DL, Shahana N, Huddleston DA, Mostofsky SH. Transcranial magnetic stimulation measures in attention-deficit/hyperactivity disorder. Pediatr Neurol. (2012) 47:177–85. doi: 10.1016/j.pediatrneurol.2012.06.003
29. Buchmann J, Gierow W, Weber S, Hoeppner J, Klauer T, Benecke R, et al. Restoration of disturbed intracortical motor inhibition and facilitation in attention deficit hyperactivity disorder children by methylphenidate. Biol Psychiatry. (2007) 62:963–9. doi: 10.1016/j.biopsych.2007.05.010
30. Detrick JA, Zink C, Rosch KS, Horn PS, Huddleston DA, Crocetti D, et al. Motor cortex modulation and reward in children with attention-deficit/hyperactivity disorder. Brain Commun. (2021) 3:fcab093. doi: 10.1093/braincomms/fcab093
31. Gilbert DL, Huddleston DA, Wu SW, Pedapati EV, Horn PS, Hirabayashi K, et al. Motor cortex inhibition and modulation in children with ADHD. Neurology. (2019) 93:e599–610. doi: 10.1212/WNL.0000000000007899
32. Gilbert DL, Isaacs KM, Augusta M, Macneil LK, Mostofsky SH. Motor cortex inhibition: a marker of ADHD behavior and motor development in children. Neurology. (2011) 76:615–21. doi: 10.1212/WNL.0b013e31820c2ebd
33. Harris AD, Gilbert DL, Horn PS, Crocetti D, Cecil KM, Edden RAE, et al. Relationship between GABA levels and task-dependent cortical excitability in children with attention-deficit/hyperactivity disorder. Clin Neurophysiol. (2021) 132:1163–72. doi: 10.1016/j.clinph.2021.01.023
34. Heinrich H, Hoegl T, Moll GH, Kratz O. A bimodal neurophysiological study of motor control in attention-deficit hyperactivity disorder: a step towards core mechanisms? Brain. (2014) 137:1156–66. doi: 10.1093/brain/awu029
35. Hoegl T, Heinrich H, Barth W, Losel F, Moll GH, Kratz O. Time course analysis of motor excitability in a response inhibition task according to the level of hyperactivity and impulsivity in children with ADHD. PloS One. (2012) 7:e46066. doi: 10.1371/journal.pone.0046066
36. Moll GH, Heinrich H, Trott G, Wirth S, Rothenberger A. Deficient intracortical inhibition in drug-naive children with attention-deficit hyperactivity disorder is enhanced by methylphenidate. Neurosci Lett. (2000) 284:121–5. doi: 10.1016/S0304-3940(00)00980-0
37. Richter MM, Ehlis AC, Jacob CP, Fallgatter AJ. Cortical excitability in adult patients with attention-deficit/hyperactivity disorder (ADHD). Neurosci Lett. (2007) 419:137–41. doi: 10.1016/j.neulet.2007.04.024
38. Samusyte G, Bostock H, Rothwell J, Koltzenburg M. Short-interval intracortical inhibition: Comparison between conventional and threshold-tracking techniques. Brain Stimul. (2018) 11:806–17. doi: 10.1016/j.brs.2018.03.002
39. Badawy RA, Loetscher T, Macdonell RA, Brodtmann A. Cortical excitability and neurology: insights into the pathophysiology. Funct Neurol. (2012) 27:131–45.
40. Hoeppner J, Neumeyer M, Wandschneider R, Herpertz SC, Gierow W, Haessler F, et al. Intracortical motor inhibition and facilitation in adults with attention deficit/hyperactivity disorder. J Neural Transm (Vienna). (2008) 115:1701–7. doi: 10.1007/s00702-008-0091-y
41. Hadas I, Hadar A, Lazarovits A, Daskalakis ZJ, Zangen A. Right prefrontal activation predicts ADHD and its severity: A TMS-EEG study in young adults. Prog Neuropsychopharmacol Biol Psychiatry. (2021) 111:110340. doi: 10.1016/j.pnpbp.2021.110340
42. Lioumis P, Kicic D, Savolainen P, Makela JP, Kahkonen S. Reproducibility of TMS-evoked EEG responses. Hum Brain Mapp. (2009) 30:1387–96. doi: 10.1002/hbm.20608
43. Bortoletto M, Bonzano L, Zazio A, Ferrari C, Pedulla L, Gasparotti R, et al. Asymmetric transcallosal conduction delay leads to finer bimanual coordination. Brain Stimul. (2021) 14:379–88. doi: 10.1016/j.brs.2021.02.002
44. Ferrarelli F, Phillips ML. Examining and modulating neural circuits in psychiatric disorders with transcranial magnetic stimulation and electroencephalography: present practices and future developments. Am J Psychiatry. (2021) 178:400–13. doi: 10.1176/appi.ajp.2020.20071050
45. Avnit A, Zibman S, Alyagon U, Zangen A. Abnormal functional asymmetry and its behavioural correlates in adults with ADHD: A TMS-EEG study. PloS One. (2023) 18:e0285086. doi: 10.1371/journal.pone.0285086
46. Bruckmann S, Hauk D, Roessner V, Resch F, Freitag CM, Kammer T, et al. Cortical inhibition in attention deficit hyperactivity disorder: new insights from the electroencephalographic response to transcranial magnetic stimulation. Brain. (2012) 135:2215–30. doi: 10.1093/brain/aws071
47. Farzan F. Transcranial magnetic stimulation-electroencephalography for biomarker discovery in psychiatry. Biol Psychiatry. (2024) 95:564–80. doi: 10.1016/j.biopsych.2023.12.018
48. Premoli I, Rivolta D, Espenhahn S, Castellanos N, Belardinelli P, Ziemann U, et al. Characterization of GABAB-receptor mediated neurotransmission in the human cortex by paired-pulse TMS-EEG. Neuroimage. (2014) 103:152–62. doi: 10.1016/j.neuroimage.2014.09.028
49. Farhat LC, Flores JM, Avila-Quintero VJ, Polanczyk GV, Cipriani A, Furukawa TA, et al. Treatment outcomes with licensed and unlicensed stimulant doses for adults with attention-deficit/hyperactivity disorder: A systematic review and meta-analysis. JAMA Psychiatry. (2024) 81:157–66. doi: 10.1001/jamapsychiatry.2023.3985
50. Bleich-Cohen M, Gurevitch G, Carmi N, Medvedovsky M, Bregman N, Nevler N, et al. A functional magnetic resonance imaging investigation of prefrontal cortex deep transcranial magnetic stimulation efficacy in adults with attention deficit/hyperactive disorder: A double blind, randomized clinical trial. NeuroImage Clin. (2021) 30:102670. doi: 10.1016/j.nicl.2021.102670
51. Cao P, Wang L, Cheng Q, Sun X, Kang Q, Dai L, et al. Changes in serum miRNA-let-7 level in children with attention deficit hyperactivity disorder treated by repetitive transcranial magnetic stimulation or atomoxetine: An exploratory trial. Psychiatry Res. (2019) 274:189–94. doi: 10.1016/j.psychres.2019.02.037
52. Dutra TG, Baltar A, Monte-Silva KK. Motor cortex excitability in attention-deficit hyperactivity disorder (ADHD): A systematic review and meta-analysis. Res Dev Disabil. (2016) 56:1–9. doi: 10.1016/j.ridd.2016.01.022
53. Peng L, Tian L, Wang T, Wang Q, Li N, Zhou H. Effects of non-invasive brain stimulation (NIBS) for executive function on subjects with ADHD: a protocol for a systematic review and meta-analysis. BMJ Open. (2023) 13:e069004. doi: 10.1136/bmjopen-2022-069004
54. Westwood SJ, Radua J, Rubia K. Noninvasive brain stimulation in children and adults with attention-deficit/hyperactivity disorder: a systematic review and meta-analysis. J Psychiatry Neurosci. (2021) 46:E14–33. doi: 10.1503/jpn.190179
55. Page MJ, Moher D, Bossuyt PM, Boutron I, Hoffmann TC, Mulrow CD, et al. PRISMA 2020 explanation and elaboration: updated guidance and exemplars for reporting systematic reviews. BMJ. (2021) 372:n160. doi: 10.1136/bmj.n160
56. Page MJ, McKenzie JE, Bossuyt PM, Boutron I, Hoffmann TC, Mulrow CD, et al. The PRISMA 2020 statement: an updated guideline for reporting systematic reviews. BMJ. (2021) 372:n71. doi: 10.1136/bmj.n71
57. Kim SY, Park JE, Lee YJ, Seo HJ, Sheen SS, Hahn S, et al. Testing a tool for assessing the risk of bias for nonrandomized studies showed moderate reliability and promising validity. J Clin Epidemiol. (2013) 66:408–14. doi: 10.1016/j.jclinepi.2012.09.016
58. DerSimonian R, Laird N. Meta-analysis in clinical trials revisited. Contemp Clin Trials. (2015) 45:139–45. doi: 10.1016/j.cct.2015.09.002
59. Willis BH, Riley RD. Measuring the statistical validity of summary meta-analysis and meta-regression results for use in clinical practice. Stat Med. (2017) 36:3283–301. doi: 10.1002/sim.v36.21
60. Sterne JA, Sutton AJ, Ioannidis JP, Terrin N, Jones DR, Lau J, et al. Recommendations for examining and interpreting funnel plot asymmetry in meta-analyses of randomised controlled trials. BMJ. (2011) 343:d4002. doi: 10.1136/bmj.d4002
61. Egger M, Davey Smith G, Schneider M, Minder C. Bias in meta-analysis detected by a simple, graphical test. BMJ. (1997) 315:629–34. doi: 10.1136/bmj.315.7109.629
62. Kahl CK, Swansburg R, Hai T, Wrightson JG, Bell T, Lemay JF, et al. Differences in neurometabolites and transcranial magnetic stimulation motor maps in children with attention-deficit/hyperactivity disorder. J Psychiatry Neurosci. (2022) 47:E239–E49. doi: 10.1503/jpn.210186
63. Hasan A, Schneider M, Schneider-Axmann T, Ruge D, Retz W, Rosler M, et al. A similar but distinctive pattern of impaired cortical excitability in first-episode schizophrenia and ADHD. Neuropsychobiology. (2013) 67:74–83. doi: 10.1159/000343912
64. Bloch Y, Harel EV, Aviram S, Govezensky J, Ratzoni G, Levkovitz Y. Positive effects of repetitive transcranial magnetic stimulation on attention in ADHD Subjects: a randomized controlled pilot study. World J Biol Psychiatry. (2010) 11:755–8. doi: 10.3109/15622975.2010.484466
65. Weaver L, Rostain AL, Mace W, Akhtar U, Moss E, O’Reardon JP. Transcranial magnetic stimulation (TMS) in the treatment of attention-deficit/hyperactivity disorder in adolescents and young adults: a pilot study. J ECT. (2012) 28:98–103. doi: 10.1097/YCT.0b013e31824532c8
66. Gómez L, Vidal B, Morales L, Baez M, Maragoto C, Galvizu R, et al. Low frequency repetitive transcranial magnetic stimulation in children with attention deficit/hyperactivity disorder. Preliminary results. Brain Stimul. (2014) 7:760–2. doi: 10.1016/j.brs.2014.06.001
67. Paz Y, Friedwald K, Levkovitz Y, Zangen A, Alyagon U, Nitzan U, et al. Randomised sham-controlled study of high-frequency bilateral deep transcranial magnetic stimulation (dTMS) to treat adult attention hyperactive disorder (ADHD): Negative results. World J Biol Psychiatry. (2018) 19:561–6. doi: 10.1080/15622975.2017.1282170
68. Alyagon U, Shahar H, Hadar A, Barnea-Ygael N, Lazarovits A, Shalev H, et al. Alleviation of ADHD symptoms by non-invasive right prefrontal stimulation is correlated with EEG activity. NeuroImage Clin. (2020) 26:102206. doi: 10.1016/j.nicl.2020.102206
69. Cao P, Xing J, Cao Y, Cheng Q, Sun X, Kang Q, et al. Clinical effects of repetitive transcranial magnetic stimulation combined with atomoxetine in the treatment of attention-deficit hyperactivity disorder. Neuropsychiatr Dis Treat. (2018) 14:3231–40. doi: 10.2147/NDT.S182527
70. Niederhofer H. Additional biological therapies for attention-deficit hyperactivity disorder: repetitive transcranical magnetic stimulation of 1 Hz helps to reduce methylphenidate. Clin Pract. (2012) 2:e8. doi: 10.4081/cp.2012.e8
71. University of Pennsylvania. Transcranial magnetic stimulation for attention deficit/hyperactivity disorder (ADHD) (2018). Available online at: https://clinicaltrials.gov/study/NCT03663179?tab=results. (Accessed February 10, 2024).
72. Ustohal L, Prikryl R, Kucerova HP, Sisrova M, Stehnova I, Venclikova S, et al. Emotional side effects after high-frequency rTMS of the right dorsolateral prefrontal cortex in an adult patient with ADHD and comorbid depression. Psychiatr Danub. (2012) 24:102–3.
73. Ziemann U, Bruns D, Paulus W. Enhancement of human motor cortex inhibition by the dopamine receptor agonist pergolide: evidence from transcranial magnetic stimulation. Neurosci Lett. (1996) 208:187–90. doi: 10.1016/0304-3940(96)12575-1
74. Vucic S, Stanley Chen KH, Kiernan MC, Hallett M, Benninger DH, Di Lazzaro V, et al. Clinical diagnostic utility of transcranial magnetic stimulation in neurological disorders. Updated report of an IFCN committee. Clin Neurophysiol. (2023) 150:131–75. doi: 10.1016/j.clinph.2023.03.010
75. Stinear CM, Byblow WD, Ackerley SJ, Barber PA, Smith MC. Predicting recovery potential for individual stroke patients increases rehabilitation efficiency. Stroke. (2017) 48:1011–9. doi: 10.1161/STROKEAHA.116.015790
76. Orth M, Rothwell JC. The cortical silent period: intrinsic variability and relation to the waveform of the transcranial magnetic stimulation pulse. Clin Neurophysiol. (2004) 115:1076–82. doi: 10.1016/j.clinph.2003.12.025
77. Triggs WJ, Macdonell RA, Cros D, Chiappa KH, Shahani BT, Day BJ. Motor inhibition and excitation are independent effects of magnetic cortical stimulation. Ann Neurol. (1992) 32:345–51. doi: 10.1002/ana.410320307
78. Priori A, Berardelli A, Inghilleri M, Accornero N, Manfredi M. Motor cortical inhibition and the dopaminergic system. Pharmacological changes in the silent period after transcranial brain stimulation in normal subjects, patients with Parkinson’s disease and drug-induced parkinsonism. Brain. (1994) 117:317–23. doi: 10.1093/brain/117.2.317
79. Hupfeld KE, Swanson CW, Fling BW, Seidler RD. TMS-induced silent periods: A review of methods and call for consistency. J Neurosci Methods. (2020) 346:108950. doi: 10.1016/j.jneumeth.2020.108950
80. Ferranti AS, Luessen DJ, Niswender CM. Novel pharmacological targets for GABAergic dysfunction in ADHD. Neuropharmacology. (2024) 249:109897. doi: 10.1016/j.neuropharm.2024.109897
81. Hestrin S, Galarreta M. Electrical synapses define networks of neocortical GABAergic neurons. Trends Neurosci. (2005) 28:304–9. doi: 10.1016/j.tins.2005.04.001
82. Presland JD, Tofari PJ, Timmins RG, Kidgell DJ, Opar DA. Reliability of corticospinal excitability and intracortical inhibition in biceps femoris during different contraction modes. Eur J Neurosci. (2023) 57:91–105. doi: 10.1111/ejn.15868
83. Du X, Hong LE. Test-retest reliability of short-interval intracortical inhibition and intracortical facilitation in patients with schizophrenia. Psychiatry Res. (2018) 267:575–81. doi: 10.1016/j.psychres.2018.06.014
84. Fried PJ, Jannati A, Davila-Perez P, Pascual-Leone A. Reproducibility of single-pulse, paired-pulse, and intermittent theta-burst TMS measures in healthy aging, type-2 diabetes, and alzheimer’s disease. Front Aging Neurosci. (2017) 9:263. doi: 10.3389/fnagi.2017.00263
85. Hermsen AM, Haag A, Duddek C, Balkenhol K, Bugiel H, Bauer S, et al. Test-retest reliability of single and paired pulse transcranial magnetic stimulation parameters in healthy subjects. J Neurol Sci. (2016) 362:209–16. doi: 10.1016/j.jns.2016.01.039
86. Ngomo S, Leonard G, Moffet H, Mercier C. Comparison of transcranial magnetic stimulation measures obtained at rest and under active conditions and their reliability. J Neurosci Methods. (2012) 205:65–71. doi: 10.1016/j.jneumeth.2011.12.012
87. Gilbert DL, Sallee FR, Zhang J, Lipps TD, Wassermann EM. Transcranial magnetic stimulation-evoked cortical inhibition: a consistent marker of attention-deficit/hyperactivity disorder scores in tourette syndrome. Biol Psychiatry. (2005) 57:1597–600. doi: 10.1016/j.biopsych.2005.02.022
88. Chou YH, Sundman M, Ton That V, Green J, Trapani C. Cortical excitability and plasticity in Alzheimer’s disease and mild cognitive impairment: A systematic review and meta-analysis of transcranial magnetic stimulation studies. Ageing Res Rev. (2022) 79:101660. doi: 10.1016/j.arr.2022.101660
89. Bunse T, Wobrock T, Strube W, Padberg F, Palm U, Falkai P, et al. Motor cortical excitability assessed by transcranial magnetic stimulation in psychiatric disorders: a systematic review. Brain Stimul. (2014) 7:158–69. doi: 10.1016/j.brs.2013.08.009
90. Kinjo M, Wada M, Nakajima S, Tsugawa S, Nakahara T, Blumberger DM, et al. Transcranial magnetic stimulation neurophysiology of patients with major depressive disorder: a systematic review and meta-analysis. Psychol Med. (2021) 51:1–10. doi: 10.1017/S0033291720004729
91. Li B, Zhao N, Tang N, Friston KJ, Zhai W, Wu D, et al. Targeting suicidal ideation in major depressive disorder with MRI-navigated Stanford accelerated intelligent neuromodulation therapy. Transl Psychiatry. (2024) 14:21. doi: 10.1038/s41398-023-02707-9
92. Cole EJ, Phillips AL, Bentzley BS, Stimpson KH, Nejad R, Barmak F, et al. Stanford neuromodulation therapy (SNT): A double-blind randomized controlled trial. Am J Psychiatry. (2022) 179:132–41. doi: 10.1176/appi.ajp.2021.20101429
93. Cole EJ, Stimpson KH, Bentzley BS, Gulser M, Cherian K, Tischler C, et al. Stanford accelerated intelligent neuromodulation therapy for treatment-resistant depression. Am J Psychiatry. (2020) 177:716–26. doi: 10.1176/appi.ajp.2019.19070720
94. Lei D, Du M, Wu M, Chen T, Huang X, Du X, et al. Functional MRI reveals different response inhibition between adults and children with ADHD. Neuropsychology. (2015) 29:874–81. doi: 10.1037/neu0000200
95. Norman LJ, Carlisi C, Lukito S, Hart H, Mataix-Cols D, Radua J, et al. Structural and functional brain abnormalities in attention-deficit/hyperactivity disorder and obsessive-compulsive disorder: A comparative meta-analysis. JAMA Psychiatry. (2016) 73:815–25. doi: 10.1001/jamapsychiatry.2016.0700
96. Feng ZJ, Deng XP, Zhao N, Jin J, Yue J, Hu YS, et al. Resting-State fMRI Functional Connectivity Strength Predicts Local Activity Change in the Dorsal Cingulate Cortex: A Multi-Target Focused rTMS Study. Cereb Cortex. (2022) 32:2773–84. doi: 10.1093/cercor/bhab380
Keywords: ADHD, TMS, cortical excitability, therapeutic efficacy, meta-analysis
Citation: Han Y, Wei Z-Y, Zhao N, Zhuang Q, Zhang H, Fang H-L, Zang Y-F and Feng Z-J (2025) Transcranial magnetic stimulation in attention-deficit/hyperactivity disorder: a systematic review and meta-analysis of cortical excitability and therapeutic efficacy. Front. Psychiatry 16:1544816. doi: 10.3389/fpsyt.2025.1544816
Received: 13 December 2024; Accepted: 27 January 2025;
Published: 13 February 2025.
Edited by:
Li Yang, Peking University Sixth Hospital, ChinaReviewed by:
Lawrence Maayan, New York State Psychiatric Institute (NYSPI), United StatesCopyright © 2025 Han, Wei, Zhao, Zhuang, Zhang, Fang, Zang and Feng. This is an open-access article distributed under the terms of the Creative Commons Attribution License (CC BY). The use, distribution or reproduction in other forums is permitted, provided the original author(s) and the copyright owner(s) are credited and that the original publication in this journal is cited, in accordance with accepted academic practice. No use, distribution or reproduction is permitted which does not comply with these terms.
*Correspondence: Yu-Feng Zang, emFuZ3lmQGh6bnUuZWR1LmNu; Zi-Jian Feng, emlqaWFuQGNicy5tcGcuZGU=
Disclaimer: All claims expressed in this article are solely those of the authors and do not necessarily represent those of their affiliated organizations, or those of the publisher, the editors and the reviewers. Any product that may be evaluated in this article or claim that may be made by its manufacturer is not guaranteed or endorsed by the publisher.
Research integrity at Frontiers
Learn more about the work of our research integrity team to safeguard the quality of each article we publish.