- 1Center for Neuroscience, Biosciences Division, SRI International, Menlo Park, CA, United States
- 2Neuroscience and Rare Diseases Discovery & Translational Area, Roche Pharma Research & Early Development, Roche Innovation Center Basel, F. Hoffmann-La Roche Ltd.", Basel, Switzerland
Introduction: This study aimed to investigate the role of TAAR2-9 in sleep/wake regulation, given TAAR1's known involvement in modulating neurotransmitter release and sleep patterns.
Methods: Male TAAR2-9 knockout (KO) and wild-type (WT) mice were compared using baseline sleep/wake patterns, responses to sleep deprivation, effects of TAAR1 agonists, and dopaminergic markers. EEG recordings and tyrosine hydroxylase immunohistochemistry were used for analysis.
Results: KO mice exhibited lower delta and theta power and higher gamma power, with fragmented sleep characterized by 16% more NREM sleep during the dark phase and 23% more REM sleep during the light phase compared to WT mice. High doses of the TAAR1 agonist RO5256390 increased wakefulness and reduced NREM sleep, while both RO5256390 and the partial agonist RO5263397 suppressed REM sleep in KO mice. Elevated tyrosine hydroxylase levels in the ventral tegmental area suggested dopaminergic involvement in these altered sleep patterns.
Discussion: TAAR2-9 modulates sleep/wake states and interacts with TAAR1. These findings highlight the therapeutic potential of targeting TAARs 2-9 in sleep-related neuropsychiatric disorders. Further research is needed to elucidate their roles.
1 Introduction
Trace amine associated receptors (TAARs) are a family of G protein-coupled receptors (GPCRs) that are expressed in vertebrates (1, 2). Among these receptors, TAAR1 has been the most extensively studied and shown to modulate neural systems. Expressed in various regions of the central and peripheral nervous systems (3–6), TAAR1 has been implicated in the modulation of dopaminergic, serotonergic, and glutamatergic neurotransmission (6–8) and has emerged as a promising therapeutic target for neuropsychiatric disorders, including schizophrenia and drug addiction (9–12). TAAR1 has also been associated with sleep/wake regulation, as demonstrated in studies utilizing TAAR1 agonists in rats (13, 14), wildtype, knockout (KO) and over-expressing (OE) mice (15, 16), and in non-human primates (17).
Recent structural studies have provided detailed molecular insights into the recognition of endogenous trace amines and drugs of abuse by TAAR1 as well as the selectivity and pharmacology of potential antipsychotic agents targeting TAAR1 such as ulotaront (SEP-363856) and ralmitaront (RO6889450) (18–20). A clinical study showed that, consistent with TAAR1 agonist suppression of REM sleep in animal studies, the TAAR1 agonist ulotaront reduced daytime sleep onset REM periods and nighttime REM duration in patients with narcolepsy-cataplexy (21). However, the magnitude of the effects of ulotaront was not deemed to be clinically sufficient to treat this disorder.
In contrast to TAAR1, the roles of TAAR subtypes TAAR2-9 remain relatively poorly understood. Recent studies have demonstrated the presence of TAAR family members in various brain regions, suggesting their potential involvement in modulating neural activities and behavior (22–24). The original description of the TAAR family reported TAAR4 expression in the amygdala and hippocampus (1), regions critical for emotional processing and memory formation. Subsequent studies have expanded our understanding of TAAR distribution in the brain, with TAAR2 having been found in the hippocampus, cerebellum, cortex, dorsal raphe, hypothalamus and habenula (25) and TAAR5 expression reported in the amygdala, hippocampus, piriform cortex, and thalamic and hypothalamic nuclei (26, 27). A recent public transcriptomic data analysis demonstrated low but detectable TAAR6 expression in several human brain regions, particularly in limbic structures such as the prefrontal cortex and nucleus accumbens, and validated TAAR6 mRNA expression in the mouse prefrontal cortex (24).
The expression patterns of TAAR2-9 in these diverse brain regions suggest their potential involvement in various neurological and psychiatric processes as shown by improved cognitive performance in TAAR5 KO mice (28). For instance, the presence of TAAR2 in the hippocampus and cortex implies a possible role in learning, memory, and higher order cognitive functions. Its expression in the dorsal raphe, a primary source of serotonergic projections, suggests a potential influence on mood regulation and anxiety-related behaviors. The presence of TAAR5 in the amygdala and hippocampus further supports the involvement of these receptors in emotional processing and memory consolidation.
Moreover, the expression of TAARs in the hypothalamus and thalamic nuclei is particularly intriguing given the critical roles that these regions play in sleep/wake regulation, circadian rhythms, and arousal (29). The hypothalamus, containing key sleep-promoting nuclei such as the ventrolateral preoptic area (VLPO) and wake-promoting regions like the lateral hypothalamus, is central to sleep/wake control (30–33). The thalamus, serving as a relay center for sensory information and playing a crucial role in the generation of sleep spindles during NREM sleep (34), further underscores the potential importance of TAARs in sleep regulation.
Recent functional studies have begun to elucidate the specific roles of TAAR subtypes. TAAR5, for example, has been implicated in adult neurogenesis, dopamine transmission and alterations in anxiety and depressive behaviors (23, 25–27, 35). While the specific functions of these and other TAAR subtypes remain to be fully identified, the expression of these receptors in brain regions associated with monoaminergic neurotransmission, emotion, and cognition suggests their potential involvement in modulating neural activities.
Given the intricate interplay among monoaminergic neurotransmitter systems, sleep regulation and psychiatric disorders (36–39), investigating the roles of TAARs beyond TAAR1 is of considerable interest (40). Thus, we studied TAAR2-9 knockout (KO) mice (41) to elucidate the potential contributions of these receptors to sleep regulation and their interactions with monoaminergic neurotransmitters. Although previously implicated in mating behaviors (41), TAAR2-9 KO mice present a unique opportunity to understand additional functions of these receptors, particularly their involvement in modulating neural activities akin to TAAR1. Given the involvement of TAAR1 in sleep/wake control described above, we evaluated whether TAAR2-9 KO mice exhibit sleep/wake patterns distinct from WT or TAAR1 KO mice.
Our findings reveal that TAAR2-9 KO mice have alterations in non-rapid eye movement (NREM) and rapid eye movement (REM) sleep, characterized by increased fragmentation and disrupted sleep architecture. Immunohistochemical analyses revealed a significantly greater density of tyrosine hydroxylase-immunoreactive neurons in the ventral tegmental area (VTA) of KO mice, potentially contributing to the increase in REM sleep duration. This finding suggests a potential link between elevated dopaminergic neurotransmission and the sleep patterns of TAAR2-9 KO mice.
2 Materials and methods
2.1 Animals
Cryopreserved embryos of TAAR2-9 KO mice (41) maintained in a pure C57BL/6 background were provided by F. Hoffmann-La Roche Ltd. and resuscitated at the Stanford University Transgenic, Knockout and Tumor Model Center (Palo Alto, CA). After weaning from surrogate mothers, mice were transferred to SRI International where they were maintained on a 12h light/12h dark cycle, with ad libitum access to food and water. Room temperature and humidity were maintained at 21-23°C and 30-50%, respectively, and were continuously monitored. A cohort of male C57BL6/J mice (WT; n = 4) bred at SRI International were used as controls; this WT cohort was supplemented by a second cohort of C57BL6/J male mice (n = 9) purchased from JAX mice. All procedures were approved by the Animal Care and Use Committee at SRI International.
2.2 Surgical procedures
Male TAAR2-9 KO (n = 8) and C57BL6/J (n = 13) mice were subcutaneously implanted with sterilized HD-X02 wireless transmitters (DSI, Inc., St. Paul, MN) for the measurement of electroencephalogram (EEG), electromyogram (EMG), locomotor activity (LMA) and subcutaneous body temperature (Tsc). As in our previous studies (15, 16, 42, 43), mice were anesthetized with isoflurane, and EMG and EEG leads were routed subcutaneously. EEG leads were inserted through the intracranial burr holes, with one lead over the hippocampal area (+1 mm A/P from lambda, +1 mm M/L) and the ground lead over the cerebellum (-1 mm A/P from lambda, +1 mm M/L). After placement of the EEG leads, dental cement was applied to the skull to affix the wires. EMG leads were sutured to the right nuchal muscle. An analgesia cocktail of meloxicam (5 mg/kg, s.c.) and buprenorphine (0.05 mg/kg, s.c.) was administered for two days post-surgery; meloxicam was administered for an additional day.
2.3 EEG and EMG recording
Male TAAR2-9 KO (n = 8) and C57BL6/J (n = 13) mice were acclimated to handling at least seven days before data collection was initiated. Recordings occurred in the animal’s home cage no sooner than three weeks post-surgery, with the wireless transmitters turned on at ZT23 (where ZT0 = lights on and ZT 12 = lights off) on Day 0 and turned off 25 hours later at ZT24 on the following day (Day 1). All mice were approximately 5 months of age at the time of recording. Physiological signals collected from transmitters were continuously recorded using Ponemah software (DSI, Inc., St. Paul, MN) and subsequently analyzed.
2.4 Experimental protocols
2.4.1 Baseline EEG and EMG recording, mMSLT, and 1-h, 3-h and 6-h sleep deprivation
After obtaining a 24-h baseline recording for each group, we conducted a murine multiple sleep latency test (mMSLT), consisting of a 20 min sleep deprivation period followed by a 20 min undisturbed sleep opportunity for five consecutive 40 min sessions over a 200 min period (44, 45) beginning at ZT3 (n = 8 KO mice; initially, n = 4 WT mice, supplemented by 9 mice from the second WT cohort at a later date). One week later, the mice were subjected to sleep deprivation (SD) of 1-, 3-, and 6-h duration ending at ZT6, which was then followed by an 18-h recording. At least 48-h elapsed between each SD experiment.
2.4.2 Pharmacological studies
One week after the SD experiments, mice were administered per os (p.o.) one of the following treatments during the light phase at ZT6, with the experimenter blinded to the dosing condition: Vehicle (10% DMSO in DI water), the TAAR1 partial agonist RO5263397 (1mg/kg), the TAAR1 full agonist RO5256390 (3 and 10mg/kg), or caffeine (10mg/kg) as a positive control for the wake-promoting effects of TAAR1 agonists expected from our previous studies (13–17). Drug treatments were administered in a balanced order such that all mice received all treatments. To ensure adequate wash-out between each treatment, at least 72-h elapsed between dosing. Mice were recorded for EEG and EMG from ZT23 on the day prior to dosing to ZT12 on the dosing day. The EEG and EMG recordings from ZT6 to ZT12 were manually scored blind to the drug condition and analyzed to determine the effects of the drug treatments on sleep-wake states.
2.5 Immunohistochemical studies
A separate cohort of TAAR2-9 KO (n = 6) and C57BL6/J (n = 9) mice were anesthetized with Isoflurane and administered 0.1ml of Somnasol (pentobarbital sodium, 390 mg/ml). Mice were then perfused with 0.9% NaCl in 0.1 M phosphate-buffered saline (PBS) at pH 7.4 followed by 4% paraformaldehyde in 0.1 M PBS (pH 7.4). After perfusion, the brains were removed and cryoprotected in 30% sucrose solution. The brains were sliced into 40 μm coronal sections at -20°C using a cryotome (Leica CM3050S, Germany). Sections were collected from the ventral tegmental area (VTA) through the dorsal raphe (DR). To identify dopaminergic neurons expressed in these areas, free-floating sections were incubated overnight at 4°C with a polyclonal rabbit anti-tyrosine hydroxylase (TH) antibody (Sigma-Aldrich, #657012 RRID: AB_566341) diluted 1:250. Sections were then incubated with a donkey anti-rabbit secondary antibody (Jackson Immunoresearch, 711-005-152 RRID: AB_2340585) diluted 1:500 for 2 h at room temperature. Slices were then processed with a mixture of diaminobenzidine (DAB), NaCl, and 0.03% H2O2 (Vectastain DAB kit, Vector Labs, CA). The stained sections were mounted on microscope slides, cover-slipped, and tissue images were obtained using a slide scanner (Olympus VS200, Japan).
2.6 Cell counts
High-resolution images from the slide scanner were analyzed using Fiji ImageJ for cell quantification (46). Images were pre-processed in Fiji ImageJ, adjusting brightness, contrast, and sharpness. Cell identification was performed using a two-step process: first, an initial automated selection based on a set threshold and binary mask creation, followed by manual inspection and confirmation of each identified cell. Cell intensities were evaluated and quantification was performed in the following regions: the ventral tegmental area (VTA), the substantia nigra pars compacta (SNc), the substantia nigra pars reticulata (SNr), and the locus coeruleus (LC). SN sub-areas were delineated according to the Allen Institute’s Mouse Brain Atlas (http://mouse.brain-map.org).
2.7 EEG scoring and spectral analysis
Recordings were scored manually in 10-sec epochs as wakefulness, NREM sleep or REM sleep using Neuroscore (DSI Inc., MN) by expert scorers as described previously (15, 16, 45). Wakefulness was characterized by relatively low voltage EEG signals and high EMG (Figure 1). NREM sleep had a higher EEG voltage and dominant power in the delta frequency band but lower EMG. REM sleep had a low EEG voltage as in wakefulness, but with low EMG and dominant power in the theta frequency range. For the baseline and the sleep deprivation study, manually scored data were used as training sets in Somnivore (47) and the full 24-h recordings were autoscored as described previously (42). In the pharmacology study, only manually scored 6-h recordings were used for analysis.
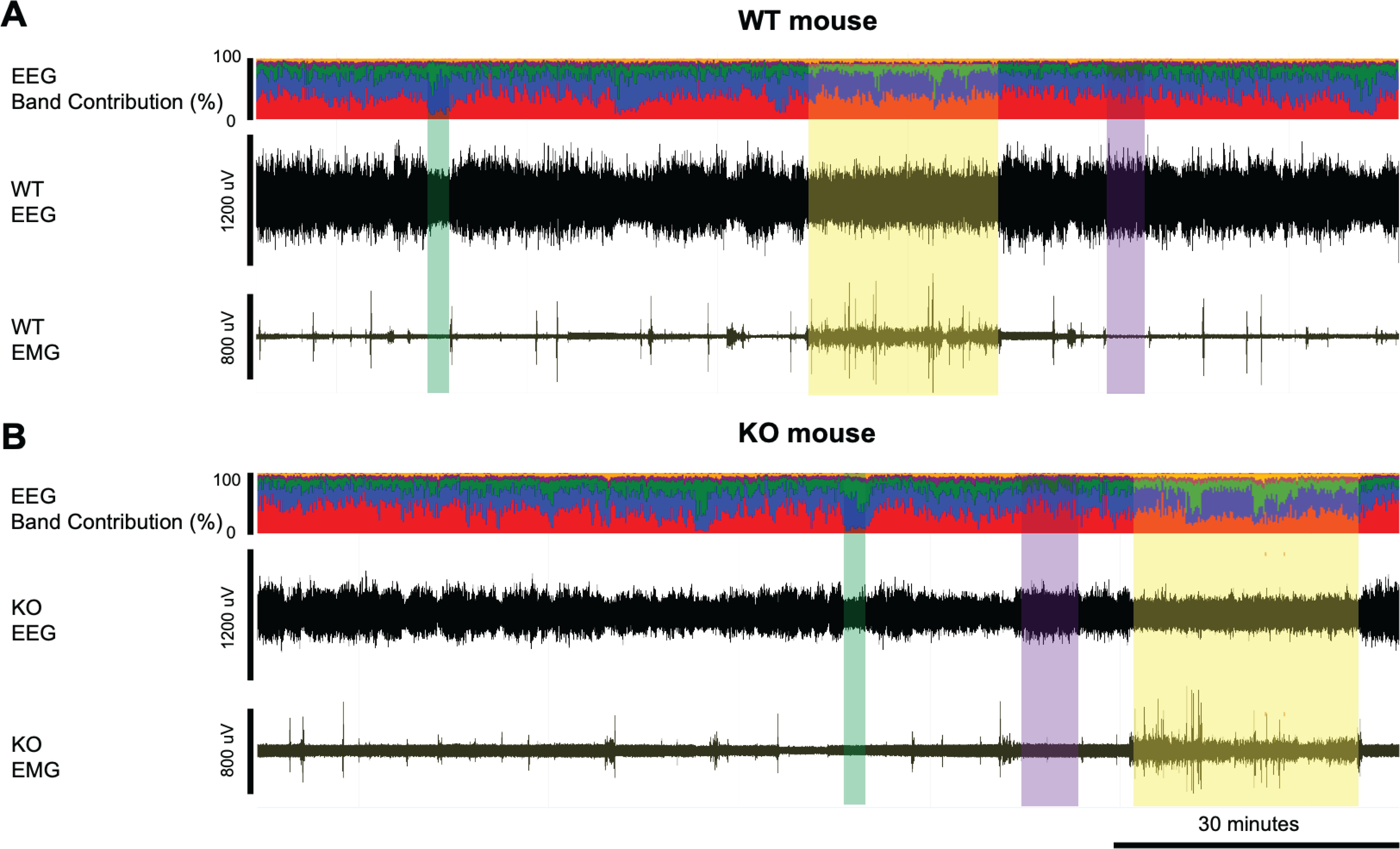
Figure 1. Example EEG and EMG recordings from a (A) WT and (B) KO mouse. Yellow-shaded boxes indicate periods of wakefulness, purple-shaded boxes indicate NREM sleep, and green-shaded boxes indicate REM sleep. The top trace in panels A and B presents the % contribution of each power band in the EEG signal with colors representing the different frequency bands: delta (0.5-4Hz) in red, theta (4-8Hz) in blue, alpha (8-12Hz) in green, sigma (12-16Hz) in purple, and beta (16-24Hz) in yellow. Wakefulness is characterized by relatively low voltage EEG signals and high EMG while NREM sleep has higher EEG voltage and dominant power in the delta frequency band but lower EMG. REM sleep has similar low EEG voltage as in wakefulness, but with low EMG and dominant power in the theta frequency range. KO mice have relatively low EEG signals compared to the WT mice, as also shown in Figure 1.
EEG spectra for each state were analyzed in 0.122 Hz bins and in standard frequency bands, rounded to the nearest half-Hz value (delta: 0.5–4 Hz, theta: 6–9 Hz, alpha: 9–12 Hz, beta: 12–30 Hz, low gamma: 30–60 Hz and high gamma: 60–100 Hz). Both raw power (Figure 2) and power normalized to the wake state (Supplementary Figure S1) are presented.
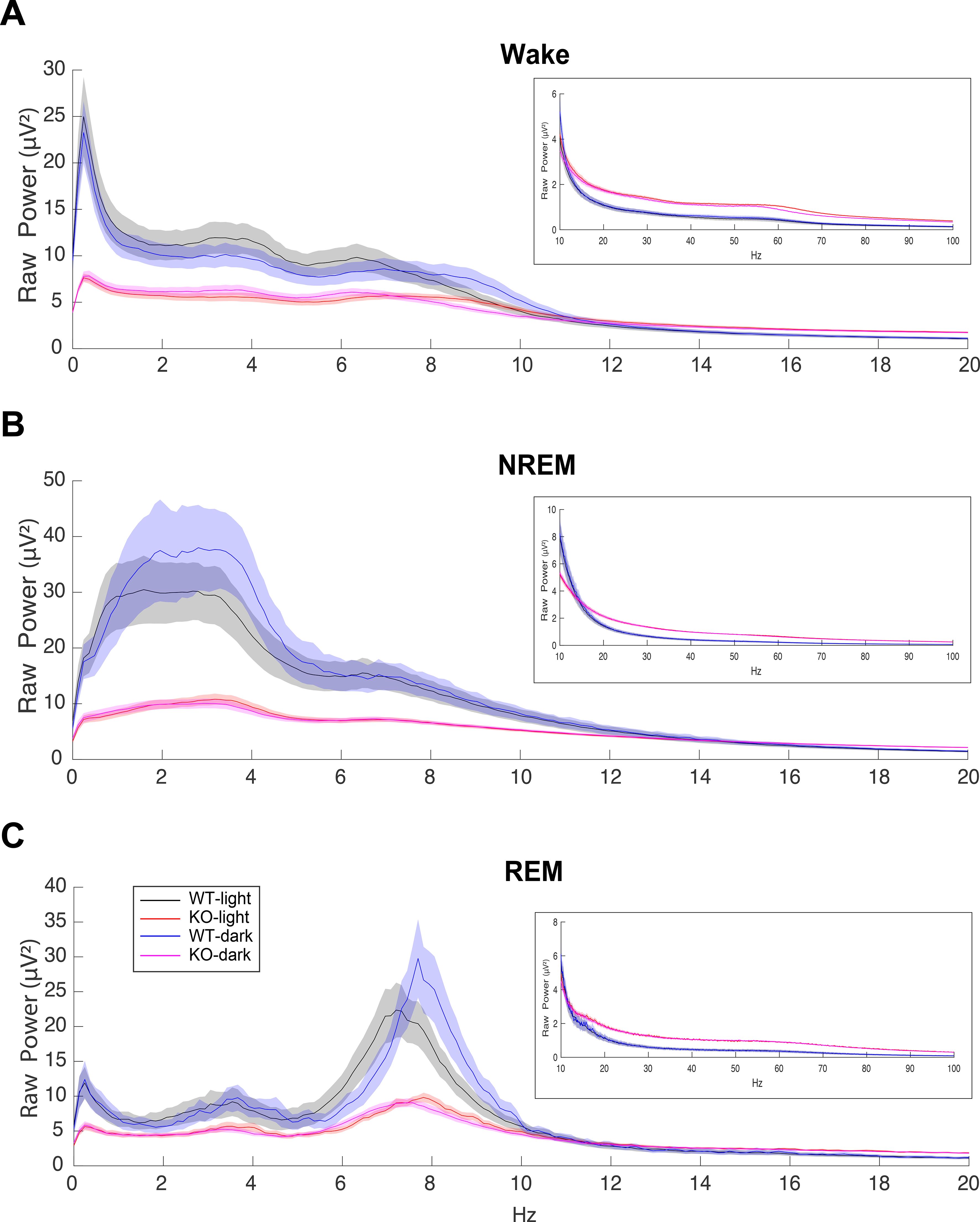
Figure 2. EEG power spectra during (A) Wake, (B) NREM and (C) REM sleep in male C57BL6/J (WT) and TAAR2-9 KO mice during both the light (ZT0-12) and dark (ZT13-24) phases from 0-20 Hz, with insets displaying the 10-100 Hz range. TAAR2-9 KO mice showed reduced EEG power in the low frequency bands in all states during both light and dark phases. However, KOs exhibited higher power in the gamma range (30-100 Hz) compared to WT mice.
2.8 Statistical analysis
Data from the baseline, sleep deprivation and pharmacology studies were analyzed using custom MATLAB R2020b (Mathworks, MA) scripts and statistics functions using Prism 10 (Graphpad, CA). Due to the wireless transfer of signal being lost after several recording sessions, one WT mouse was excluded from the analysis of the sleep/wake studies so the data analysis was based on n = 8 TAAR2-9 KO and n = 12 C57BL6/J male mice. Two-way repeated measures ANOVA (RM-ANOVA) was conducted with mouse strain and time (Figures 3, 4) or drug treatment and time (Figure 5) as factors followed by either Šídák’s or Tukey’s post hoc multiple comparison test. For comparisons within the light or dark phases (Figures 3, 4) and the baseline vs. sleep deprivation study (Figure 6), the KO and WT groups were compared using an unpaired t-test with Welch’s correction
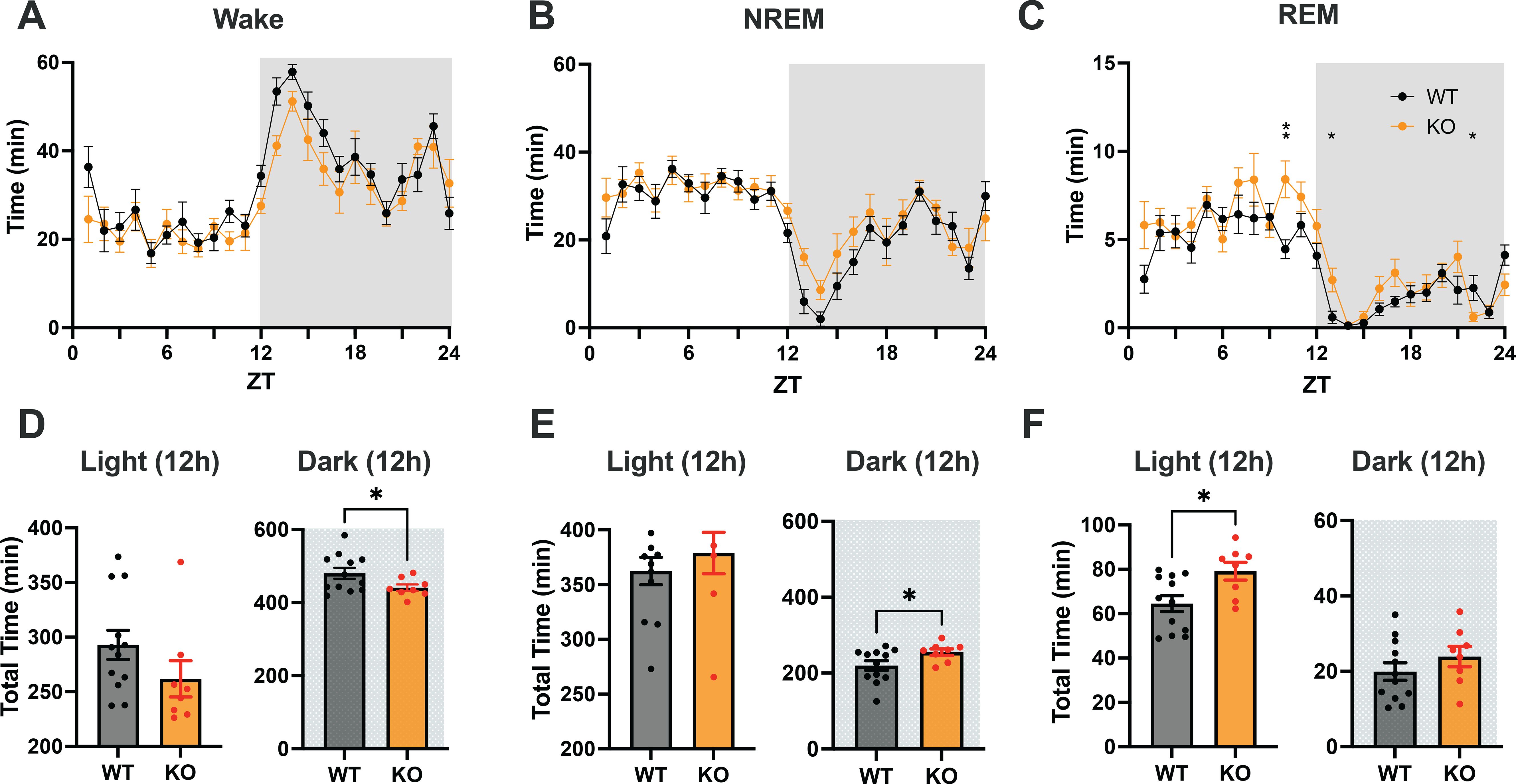
Figure 3. (A–C) Distribution of Wake, NREM and REM sleep in male C57BL6/J (WT) and TAAR2-9 KO mice across a 24-h recording. (D–F) Cumulative time in each state recorded in each strain during 12-h light (ZT0-12) and 12-h dark (ZT12-24) phases; shaded areas denote the dark phases. WT, Wild type mice; KO, TAAR2-9 KO mice; *, p < 0.05; ns, not significant.
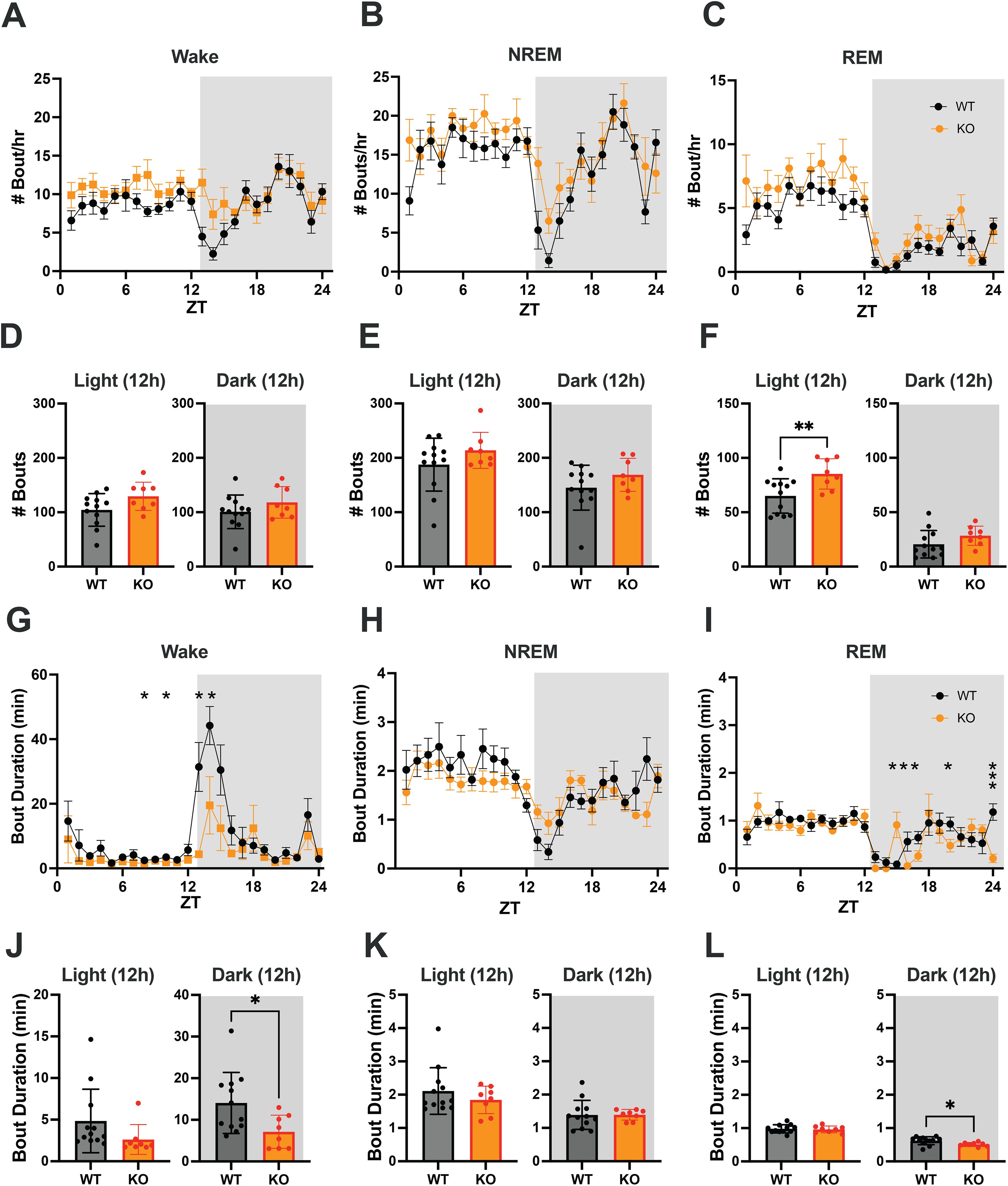
Figure 4. (A–C) Hourly number of Wake, NREM and REM sleep bouts in TAAR2-9 KO and WT mice across a 24-h baseline recording. (D–F) Mean number of Wake (D), NREM sleep (E) and REM sleep (F) bouts for each strain during the light and dark phases. (G–I) Hourly duration of Wake, NREM and REM sleep bouts in TAAR2-9 KO and WT mice across the 24-h recording. (J–L) Mean duration of Wake, NREM and REM sleep bouts for each strain during the light (ZT0-12) and dark (ZT12-24) phases. Shaded areas denote the dark phases. Values are mean ± SEM. *, p < 0.05; **, p < 0.01; ns, not significant.
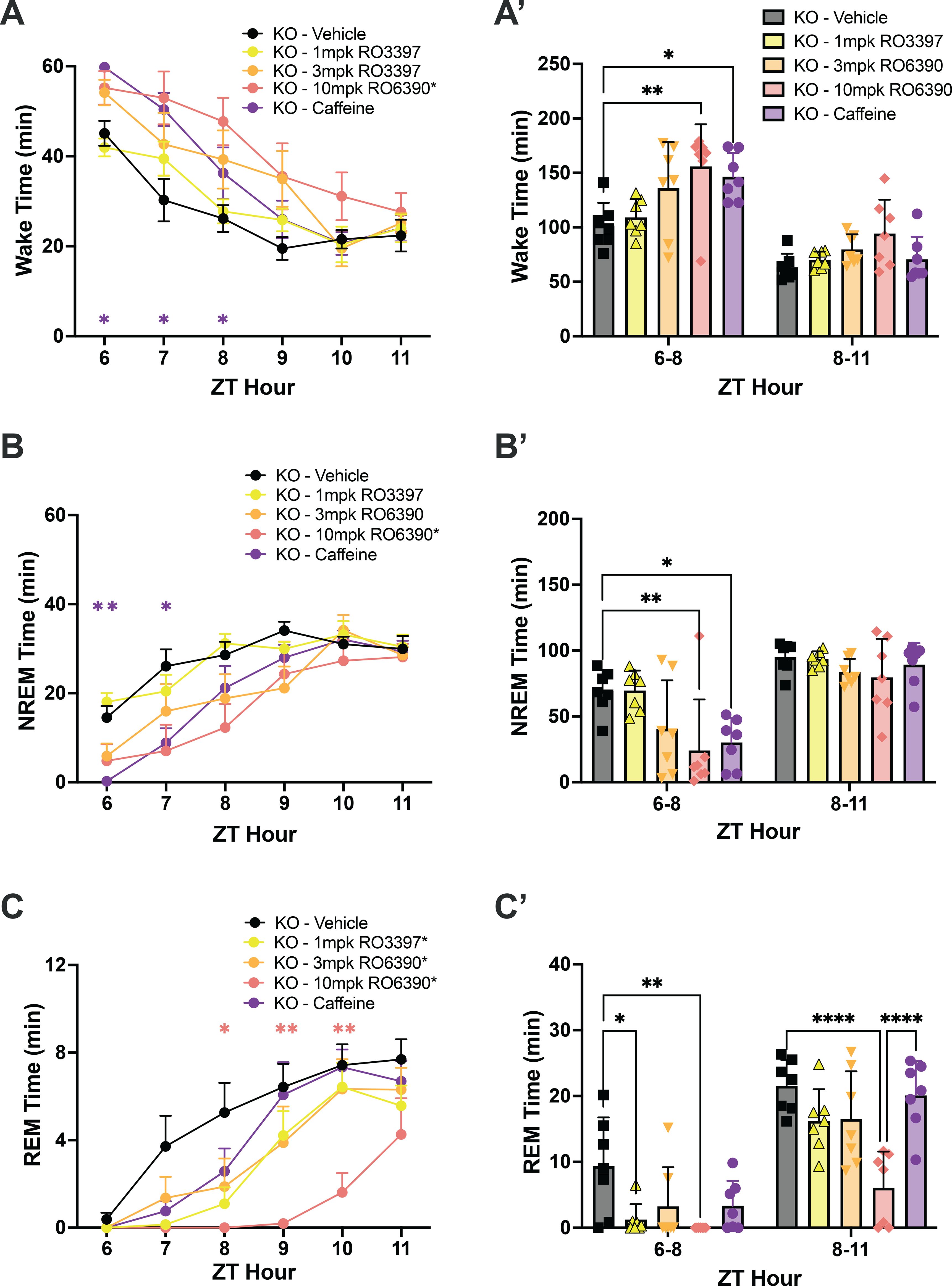
Figure 5. (A) Wake, (B) NREM, and (C) REM time in hourly intervals (left) and summed in 3-hour bins (A’, B’, C’) after dosing with the vehicle, the TAAR1 partial agonist RO3397, the TAAR1 full agonist RO6390 and caffeine. Values are mean ± SEM. Asterisks in the legends indicate a significant condition effect determined by 2-way RM-ANOVA; asterisks within the graphs indicate specific hours when RM-ANOVA indicated a significant time x treatment effect. *, p < 0.05; **, p < 0.01; ***, p < 0.005; ****, p < 0.001. The color of the asterisk in A-C indicates the treatment that differs from vehicle during that hour, as determined by post hoc tests.
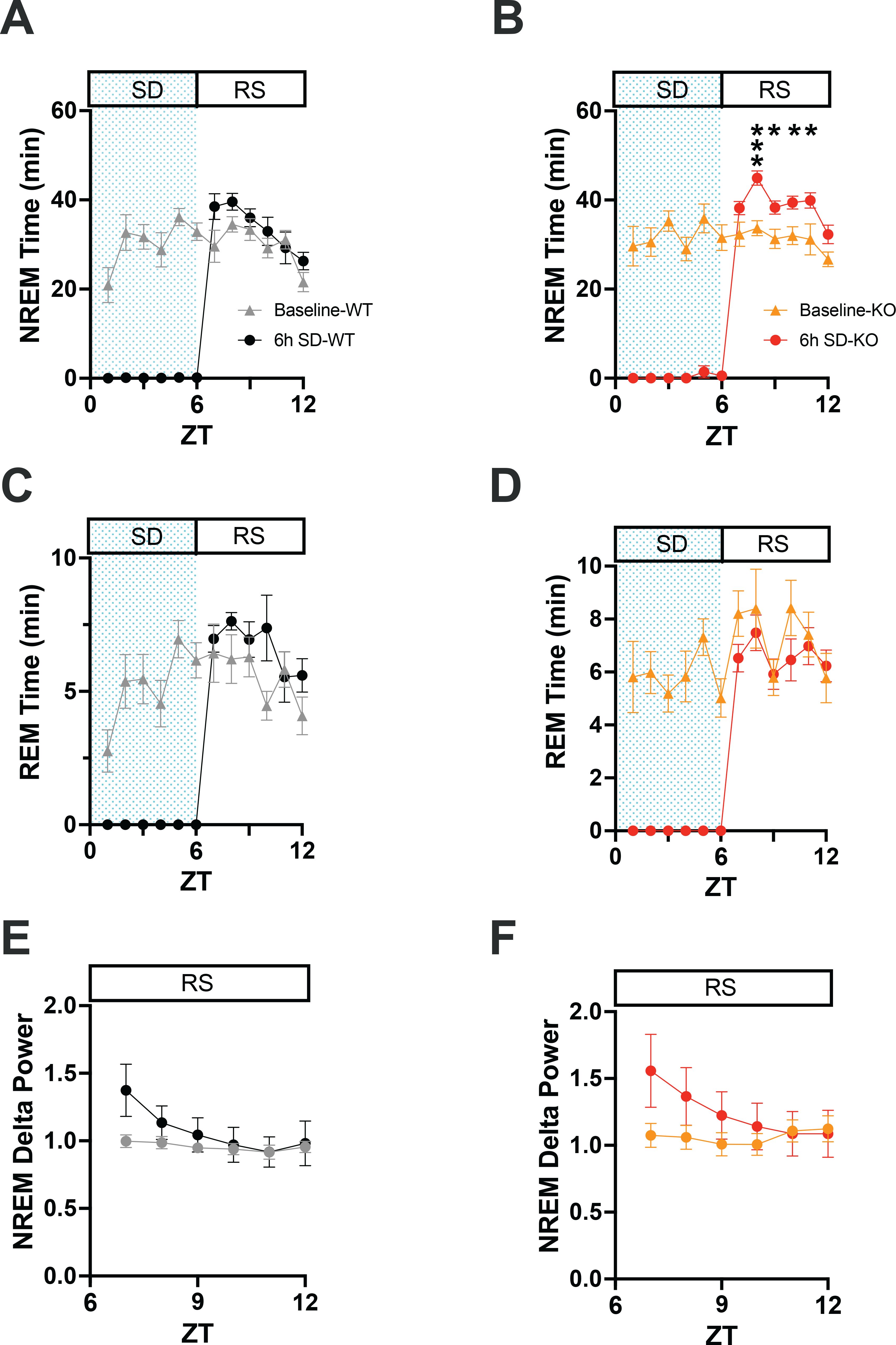
Figure 6. (A) Comparison of NREM sleep time from ZT0 to ZT12 in WT mice on a baseline day vs. a day on which 6-h sleep deprivation (SD) occurred from ZT0-6 (shaded area) followed by a 6-h recovery sleep (RS) opportunity from ZT6-12. (B) Same as A but for TAAR2-9 KO mice. (C, D) Same as A, B but for REM sleep. (E, F) EEG delta power in NREM sleep during the RS period after 6-h SD compared to the baseline day in WT (E) and TAAR2-9 KO (F) mice. Values are mean ± SEM. *, p < 0.05.
Images from the IHC studies (Figure 7) were analyzed using Fiji (ImageJ for MacOS, opensource package), including the cell auto-counting function. Due to the small sample size, the Mann Whitney U test was used to compare cell counts within each brain region.
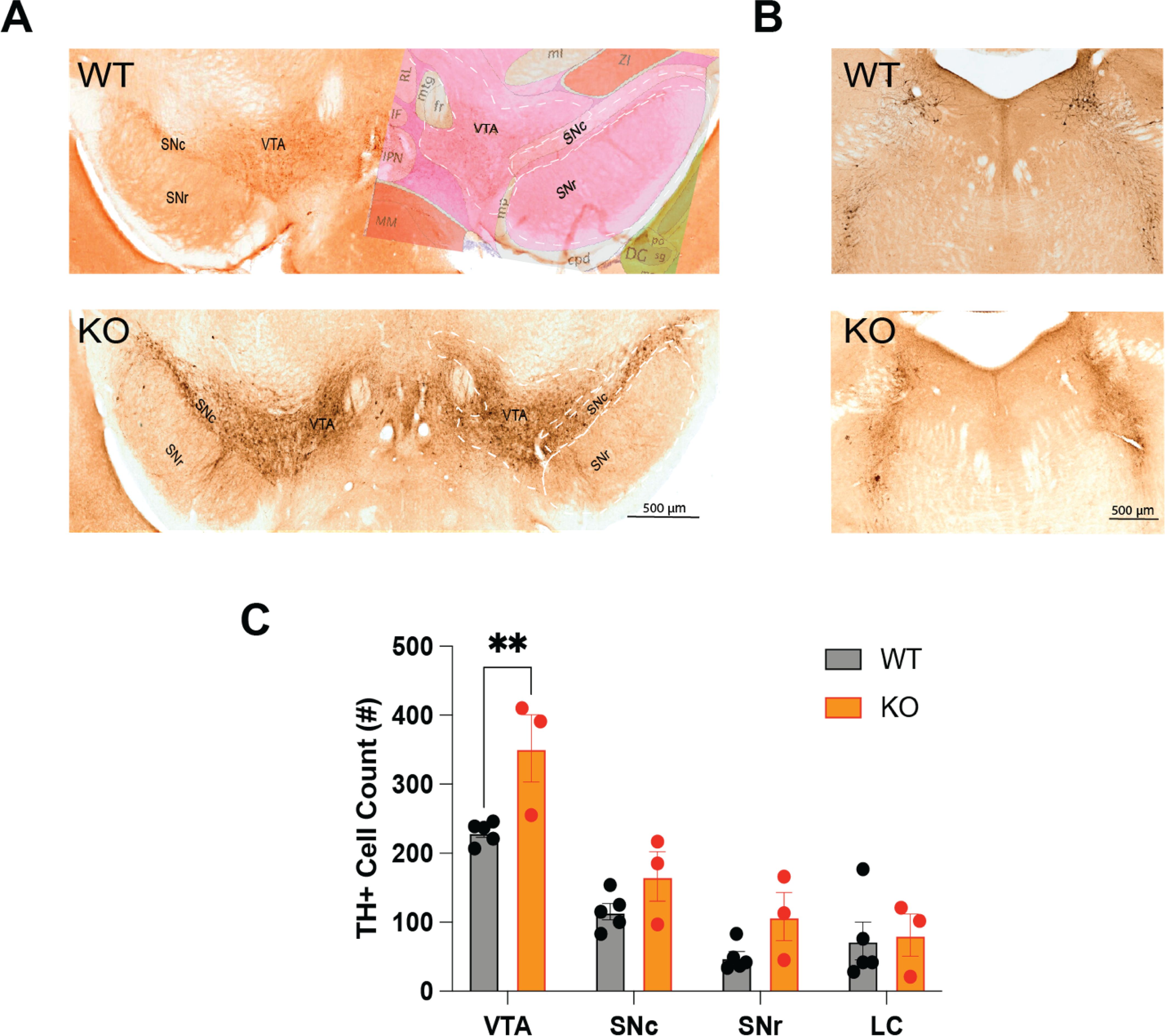
Figure 7. (A) Distribution of tyrosine hydroxylase-immunopositive (TH+) neurons in the ventral tegmental area (VTA), the substantia nigra compacta (SNc) and the substantia nigra reticulata (SNr) of TAAR2-9 KO and WT mice. The right side of the WT image in A is overlaid with an image from the corresponding section from the Allen Mouse Brain Atlas. (B) Distribution of TH+ neurons in the locus coeruleus (LC) of TAAR2-9 KO and WT mice. (C) Number of TH+ neurons in the VTA, SNc, SNr and LC of TAAR2-9KO and WT mice. Values are mean ± SEM. **, p < 0.01.
3 Results
3.1 Baseline physiological recordings
3.1.1 EEG spectral analysis
The EEG spectra differed considerably between WT and KO mice. KO mice exhibited much lower raw power overall up to 10 Hz but higher power above 20 Hz (Figure 2). These features did not change the overall EEG waveform or the spectral band contribution in KO mice except for the amplitude of the EEG signals. Consequently, Wake, NREM sleep, and REM sleep were readily scored (Figure 1). When analyzed by 2-way ANOVA, normalized power (Supplementary Figure S1) showed genotype differences (p < 0.0001) during both NREM and REM sleep for all 3-h bins during the light phase (Supplementary Table S1). Šídák’s multiple comparisons determined that KO mice had significantly greater normalized power in the β, low and high γ bands during NREM sleep from ZT4 to ZT12 (Supplementary Figures S1E, S1H, S1K). In contrast, the increase in normalized power during REM sleep progressed with time as only the low γ band was significantly greater from ZT4-6 (Supplementary Figure S1F), then both the low and high γ bands were significantly greater from ZT7-9 (Supplementary Figure S1I), and the β, low and high γ bands were significantly greater from ZT10-12 (Supplementary Figure S1L). Unlike TAAR1 KO mice which had higher normalized power over all bands compared to WT mice (15), no theta band difference was observed in TAAR2-9 KO mice.
3.1.2 Total sleep/wake time and distribution
To assess the sleep/wake patterns of TAAR2-9 KO mice and WT mice, baseline recordings were conducted from ZT0 to ZT24. Figures 3A–C suggested that KO mice exhibited less wakefulness and higher amounts of sleep across the 24-h period. Two-way ANOVA revealed a strong trend for genotype differences in Wake that did not quite reach significance (p = 0.0512) and significant genotype (F(1, 18) = 7.719, p = 0.0124) and time x genotype (F(23, 414) = 1.857, p = 0.0099) differences for REM sleep. Comparison of the total amounts of sleep/wake across the 24-h period by an unpaired t-test with Welch’s correction indicated significantly less wakefulness (p = 0.0441) and more REM sleep (p = 0.0148; Supplementary Figure S2). Analyses of the light and dark phases indicated that KO mice exhibit reduced wakefulness (p = 0.0369; Figure 3D) and increased NREM sleep (p = 0.0354; Figure 3E) during the dark phase when mice are usually more active. Figures 3A, B indicate that these differences were most prominent during the first few hours of the dark phase. During the 12-hour light phase, KO mice also exhibited a significantly higher amount of REM sleep compared to WT mice (p = 0.0153; Figure 3F), while no significant differences in the total amounts of Wake or NREM sleep were observed between the two strains at this time of day.
3.1.3 Sleep architecture of KO vs. WT mice
To identify changes in sleep/wake architecture that underlie the sleep/wake differences between TAAR2-9 KO and WT mice described above, we compared the number of bouts and bout durations between the strains during light and dark phases. The number of bouts per hour and the cumulative number of bouts of Wake and NREM sleep did not differ between WT and KO mice (Figures 4A, B, D, E). However, 2-way ANOVA revealed a genotype difference in the number of REM sleep bouts between the two strains (F(1, 18) = 9.215, p = 0.0071; Figure 4C). The cumulative number of REM sleep bouts during the light phase was significantly greater in KO mice (Figure 4F; p = 0.0080, Welch’s t-test), although no specific hourly differences were observed.
In contrast, 2-way ANOVA identified both genotype (F(1, 18) = 5.260, p = 0.0341) and time x genotype (F(23, 414) = 2.524, p = 0.0002) differences for the mean Wake bout duration. Tukey’s multiple comparison post hoc test revealed that Wake bouts were significantly shorter in KO mice at ZT8, ZT10, ZT13 and ZT14 (Figure 4G) and Welch’s t-test confirmed that Wake bouts were shorter in the dark phase overall (Figure 4J; p = 0.0142), the time of day when mice are normally active. Two-way ANOVA also identified time x genotype (F(23, 414) = 2.428, p = 0.0003) differences for the mean REM bout duration; Tukey’s post hoc tests revealed shorter REM bouts in KO mice at ZT16, ZT17, ZT20 and ZT24 but longer REM bouts at ZT15 (Figure 4I). Welch’s t-test confirmed that REM bouts were shorter in KO mice during the dark phase overall (Figure 4L; p = 0.0124), suggesting more fragmented sleep in this strain.
These analyses demonstrate that KO mice have disturbed sleep relative to WT mice, particularly fragmented REM sleep with more REM bouts in the light phase and shorter REM bouts in the dark phase. The Multiple Sleep Latency Test (MSLT) further supported these findings, as KO mice spent a significantly higher percentage of time in REM sleep during the recovery sleep (RS) opportunities compared to WT mice (Supplementary Figure S3E; p = 0.0071, Welch’s t-test). KO mice also had difficulty sustaining the long wake bouts that are typical of the early portion of the light phase.
3.2 Response to sleep deprivation
To investigate the integrity of the sleep homeostatic system in TAAR2-9 KO mice, we evaluated their response to sleep deprivation (SD) durations of 1-h, 3-h, and 6-h compared to WT mice. SDs of these varying durations were initiated to end at the same time of day: the middle of the light phase at ZT6. Each strain’s recording during the SD and subsequent recovery phase was compared to its baseline (BL) day recording (Figure 6). Sleep homeostasis was evaluated by two measures: (1) the amount of sleep during the 18-h period following cessation of SD and (2) NREM delta power during the first 6-h after SD.
When the hourly amounts of NREM sleep during the recovery after the 6-h SD were compared to the same ZT6-12 period on the baseline day, 2-way ANOVA found a main effect for condition in both WT (Figure 4A; F(1, 22) = 4.675, p = 0.0417) and KO (Figure 6B; F(1, 13) = 20.36, p = 0.0006) mice. However, the NREM increase was significant on an hourly basis only for the KO mice (ZT8-11). Two-way ANOVA also found a condition effect for hourly REM time in WT mice (Figure 6C; F(1, 22) = 4.498, p = 0.0455). NREM Delta power during recovery from SD (ZT 7-12) showed time x condition effects in both WT (Figure 6E; F(5, 110) = 8.731, p < 0.0001) and KO mice (Figure 6F; F(5, 70) = 12.02, p < 0.0001), although no specific hour during recovery was significantly different from BL for either mouse strain.
These results from the 6-h SD indicate an intact sleep homeostatic system in KO mice as measured by NREM delta power during the first 3 hours of the RS period when delta power was increased relative to the baseline day (Figures 6E, F). The results from the 1-h and 3-h SD were similar although not shown here. Both strains showed reduced wakefulness during recovery in the light phase (ZT6-12; Supplementary Figure S4A), which persisted into the subsequent dark phase (Supplementary Figure S4B). However, KO mice exhibited more NREM sleep during recovery in both the light (Supplementary Figure S4C) and dark phases (Supplementary Figure S4D), suggesting an enhanced homeostatic response to SD as determined by this measure of sleep homeostasis. Recovery of REM sleep was delayed until the dark phase in both strains (Supplementary Figure S4F).
3.3 Response to TAAR1 agonists
We tested the TAAR1 partial agonist RO5263397 (1mpk), the full agonist RO5256390 (3mpk and 10mpk) and caffeine (10mpk) to determine whether TAAR2-9 KO mice responded to these compounds. Two-way ANOVA revealed significant effects of drug treatment on Wake (F(30,30) = 2.032, p = 0.0264; Figure 5A), NREM (F(30,30) = 1.965, p = 0.0346; Figure 5B) and REM sleep (F(30,30) = 2.061, p = 0.0260; Figure 5C). Tukey’s post hoc tests determined that caffeine increased Wake (p = 0.0115; Figure 5A’) and reduced NREM sleep (p = 0.0167; Figure 5B’) during the first 3 hours post-dosing (ZT6-8) while REM sleep was not significantly affected (Figure 5C’). Compared to Vehicle, the high dose of the full agonist RO5256390 (10mpk) increased Wake for the first three post-dosing hours (Figure 5A, A’; p = 0.0013) similar to caffeine. During this period, RO5256390 (10mpk) also reduced both NREM (Figure 5B, B’; p = 0.0038) and REM sleep (Figure 5C, C’; p = 0.0091) The reduction in REM sleep due to RO5256390 (10mpk) persisted into the subsequent 3 hours (ZT8-11; p < 0.0001). Interestingly, although RO5263397 (1mpk) had no significant effect on Wake or NREM sleep, it suppressed REM sleep in KO mice during the first three post-dosing hours (Figure 5C, C’, p = 0.0324).
3.4 Altered monoaminergic functions in TAAR2-9 KO mice
To investigate whether the disrupted sleep patterns observed in TAAR2-9 knockout (KO) mice are associated with alterations in monoaminergic neurotransmitter systems, we conducted immunohistochemical analyses to examine the expression of a key enzyme in dopaminergic neuronal populations. Tyrosine hydroxylase (TH), the rate-limiting enzyme in dopamine synthesis, was used as a marker to label dopaminergic neurons in the ventral tegmental area (VTA), substantia nigra pars compacta (SNc), substantia nigra pars reticulata (SNr), and to identify norepinephrine-producing neurons in the locus coeruleus (LC). A two-way ANOVA revealed significant effects of both genotype (F(1,24) = 11.76, p = 0.0022) and brain region (F(3,24) =32.97, p < 0.0001). Due to the small sample size, a Mann-Whitney U test was performed for pairwise comparisons within each brain region. This analysis determined that TAAR2-9 KO mice exhibited a significantly greater density of TH-positive dopaminergic neurons in the VTA than in wildtype controls (Figure 7; p = 0.036, effect size r =0.791). While similar trends were observed in the SNc and SNr, these differences did not reach statistical significance. No differences were found in the LC between the two strains. These findings suggest that the absence of TAAR2-9 receptors may lead to a selective upregulation of the dopaminergic system, particularly in the VTA. This selective dopaminergic dysregulation could contribute to the altered sleep-wake and sleep architecture measures observed in the TAAR2-9 KO mice.
3.5 Summary of results
As detailed above, this study found that TAAR2-9 KO mice exhibit significant disturbances in sleep/wake patterns compared to C57BL6/J mice. During the dark (active) phase, TAAR2-9 KO mice showed 8.9% less wakefulness and 16% more NREM sleep (Figures 3D, E), while during the light (rest)phase, they exhibited 23% more REM sleep (Figures 3C, F). The reduced wake during the dark phase was a result of the inability of TAAR2-9 KO mice to sustain long wake bouts (Figure 4J), particularly early in the dark phase (Figure 3G) while the increased REM sleep during the light phase was due to more REM bouts (Figure 4F). KO mice also demonstrated increased REM sleep during the rest periods of the MSLT (Supplementary Figure S3E). The elevated spectral power in the low and high gamma ranges of the EEG during NREM and REM sleep (Supplementary Figure S2) provides further evidence for disturbed sleep since these frequencies are usually associated with alertness and cognitive processes
Despite these disturbances, the sleep homeostatic system appeared to be intact, as indicated by the expected increase in NREM delta power in response to SD (Figure 6F). However, TAAR2-9 KO mice showed a delayed increase in NREM sleep time during the subsequent dark phase (Supplementary Figure S4E), suggesting a subtle alteration in homeostatic sleep regulation. TAAR2-9 KO mice responded to a TAAR1 full agonist by increasing Wake (Figure 5A, A’) and reducing both NREM (Figure 5B, B’) and REM (Figure 5C, C’) sleep. In contrast, a TAAR1 partial agonist only suppressed REM sleep in KO mice (Figure 5C, C’).
Lastly, the IHC analysis revealed that TAAR2-9 KO had an increased density of TH-positive neurons specifically in the VTA (Figure 7), suggesting a dysregulation of the dopaminergic system that may contribute to the observed sleep-wake disturbances.
4 Discussion
4.1 TAAR2-9 and sleep/wake
The present study provides novel and unexpected insights into the potential roles of TAARs 2-9 in the EEG, sleep/wake regulation and interactions with monoaminergic neurotransmitter systems. Despite the reduced spectral power in EEG frequencies <10 Hz across all states and increased power in higher EEG frequencies, Wake, NREM and REM sleep were readily detected in conjunction with the EMG in TAAR2-9 KO mice. Normalization across the entire spectrum revealed that increased power in the higher EEG bandwidths during NREM and REM sleep. TAAR1 KO mice also exhibit increased spectral power in the gamma range during both NREM and REM sleep (15). Since TAAR1 is known to modulate cortical glutamate release (8) and NMDA receptor antagonists increase gamma power in the EEG (48–51), these results suggest that TAAR2-9 may also modulate glutamate release.
TAAR2-9 KO mice had less Wake and more REM sleep across the 24-h period and exhibited distinct alterations in sleep architecture, characterized by reduced Wake and increased NREM sleep during the dark phase, elevated REM sleep during the light phase, and more fragmented sleep patterns overall. TAAR2-9 KO mice were also unable to sustain long bouts of wakefulness early in the dark phase, which is typically the major activity period for mice, and had shorter REM bouts during the dark phase. These observations demonstrate that the absence of TAAR2-9 receptors disrupts the normal sleep-wake cycle and REM/NREM sleep balance and further implicates the trace amine/TAAR system in sleep/wake control as suggested by previous studies (13–17).
Despite the sleep disruptions observed in KO mice, neither sleepiness as measured in the mMSLT nor the homeostatic response to SD as measured in the conventional manner by quantification of EEG Slow Wave Activity (aka NREM Delta Power) differed between TAAR2-9 KO mice and WT controls. However, TAAR2-9 KO mice spent more time in NREM sleep during recovery after SD both during the remaining 6-h of the light phase after SD was terminated and during the subsequent 12-h dark phase. The fact that most of the rebound in both NREM and REM sleep occurs during the dark phase is not uncommon in mice (52). However, since the magnitude of the NREM time increase during the dark phase (and corresponding reduction in Wake time) was significantly greater in TAAR2-9 KO mice than in WT mice, the compensatory mechanisms involved in sleep homeostasis are likely altered in the absence of TAAR2-9 receptors. Further investigations are warranted to elucidate these potential compensatory pathways and their interactions with other sleep-regulatory systems (53).
4.2 Effects of TAAR1 agonists in TAAR2-9 KO mice
We previously reported that the TAAR1 partial agonist RO5263397 increased wakefulness and suppressed REM sleep in rats (13, 14), mice (15) and non-human primates (17). In WT mice, 0.3mpk and 1mpk RO5263397 increased wakefulness and suppressed NREM and REM sleep; those effects were eliminated in TAAR1 KO mice (Table 1), indicating that they are mediated through TAAR1 (15). In contrast, 1.0mpk RO5263397 reduced REM sleep without affecting either Wake or NREM sleep in TAAR2-9 KO mice (Table 1). The suppression of REM sleep by RO5263397 in TAAR2-9 KO mice is consistent with this effect being mediated by TAAR1. However, the absence of an increase in Wake and decrease in NREM sleep in TAAR2-9 KO mice that is observed in WT mice at this dose suggests that TAAR1 may only be partially functional in TAAR2-9 KO mice. One possible source of this discrepancy could be a difference in genetic background between the TAAR2-9 KO vs. C57BL6/J control mice. Although the TAAR2-9 KO mice were maintained on a C57BL6/J background, the KO and WT control mice used in this study were not littermates.
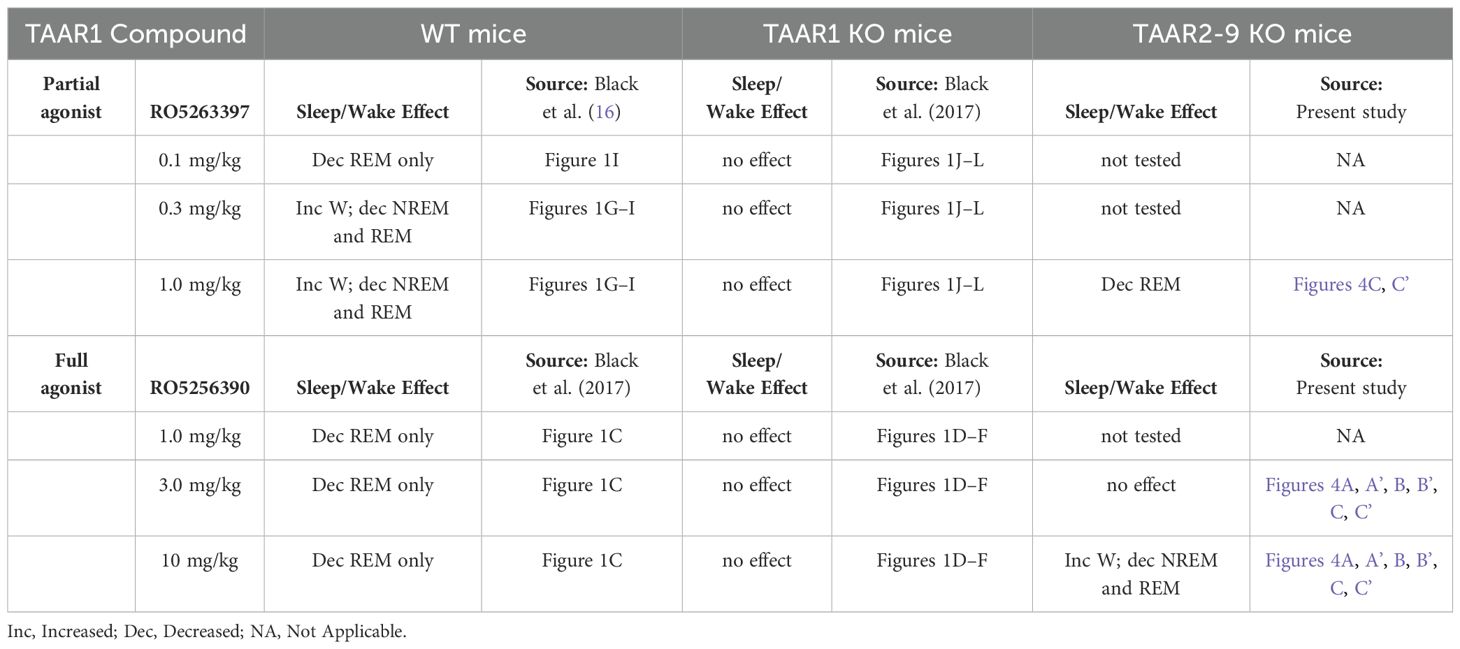
Table 1. Summary of effects of TAAR1 partial and full agonists on sleep/wake parameters in male wildtype, TAAR1 null mutant and TAAR2-9 null mutant mice.
In WT mice, the TAAR1 full agonist RO5256390 decreased REM sleep without affecting Wake or NREM sleep at the doses tested (1mpk, 3mpk, and 10mpk); Table 1 summarizes that this effect was eliminated in TAAR1 KO mice (16). By contrast in the present study, the TAAR1 full agonist RO5256390 (10 mpk) enhanced Wake and suppressed both NREM and REM sleep in TAAR2-9 KO mice and the effects on REM sleep were prolonged (Table 1). These results indicate that, in the absence of TAAR2-9, the full agonist RO5256390 produces effects similar to the partial agonist RO5263397 in WT mice. These findings suggest that TAAR2-9 may modulate the effects of these TAAR1 agonists on sleep/wake regulation and suggest interactions between TAAR1 and TAAR2-9, possibly through monoaminergic neurotransmitter systems.
4.3 TAAR2-9 and monoaminergic systems
The immunohistochemical analysis indicated an increased density of tyrosine hydroxylase-positive (TH+) dopaminergic neurons in the ventral tegmental area (VTA) of TAAR2-9 KO mice, suggesting a dysregulation of the dopaminergic system in this strain. Given the crucial role of the VTA in dopamine neurotransmission and its involvement in regulating motivated behaviors, reward processing, and motor functions, alteration of the dopaminergic system could contribute to the observed sleep phenotypes, particularly the increase in REM sleep (54). Although we do not provide direct evidence of altered dopaminergic activity, increased dopaminergic activity in TAAR2-9 KO mice would align with previous studies indicating that dopamine plays a critical role in regulating sleep architecture (33, 55). Dopamine’s involvement in the sleep/wake cycle is complex, with various studies suggesting that it promotes wakefulness and inhibits REM sleep (54, 56, 57). Thus, the observed increase in REM sleep duration in TAAR2-9 KO mice may reflect a compensatory response to changes in dopamine signaling pathways caused by the absence of TAAR2-9 receptors. Overall, these findings contribute to our understanding of the complex interplay between trace amine receptors and monoaminergic neurotransmission in the regulation of sleep-wake cycles and sleep architecture. The observed sleep disturbances and alterations in dopaminergic signaling in TAAR2-9 KO mice highlight the potential involvement of these lesser-known TAARs in modulating neural processes underlying sleep regulation.
4.4 Limitations of the current study
As stated above, although the TAAR2-9 KO mice in the present study were maintained on a C57BL6/J background, the WT mice to which they were compared were not littermates and strain differences are well known to affect sleep/wake (52, 58). Furthermore, the sleep/wake data from the C57BL6/J mice were collected in two cohorts: one bred at SRI International (N=4) and a second cohort that was purchased from JAX labs (N=9). Thus, the smaller cohort did not experience stress associated with transportation that either the larger cohort or the TAAR2-9 KO mice did.
Our study was also limited to male mice; it is currently unclear whether there are sex differences between these strains or whether female TAAR2-9 KO mice exhibit sleep/wake patterns that vary across the estrus cycle (59). Our study of the monoaminergic system was limited to a comparison of the cell density rather than a full stereological analysis of each brain region. Furthermore, any conclusions with respect to the function of the dopaminergic system in TAAR2-9 KO mice would be premature in the absence of functional studies such as measurement of DA release or chemogenetic manipulation of the DA system.
5 Conclusion
The present study provides novel evidence for the involvement of TAAR2-9 receptors in sleep regulation and their interactions with monoaminergic neurotransmitter systems. The altered sleep architecture, disrupted REM/NREM sleep balance, and indication of dopaminergic dysregulation observed in TAAR2-9 KO mice suggests previously unsuspected roles for these receptors in modulating sleep-wake cycles and associated neural pathways. While further investigations are needed to elucidate the precise mechanisms and pathways involved, the findings from this study suggests a new avenue for exploring the therapeutic potential of targeting TAAR2-9 receptors in sleep disorders and neuropsychiatric conditions involving monoaminergic dysregulation. The interactions between TAAR1 agonists and TAAR2-9 receptors, as evidenced by the pharmacological effects on sleep/wake, suggest potential synergistic or compensatory mechanisms that also warrant further exploration. Understanding these interactions may lead to the development of more effective and targeted therapeutic strategies for sleep-related disorders and associated comorbidities.
Data availability statement
The original contributions presented in the study are included in the article/Supplementary Material. Further inquiries can be directed to the corresponding author/s.
Ethics statement
The animal study was approved by SRI Animal Care and Use Committee. The study was conducted in accordance with the local legislation and institutional requirements.
Author contributions
SP: Conceptualization, Data curation, Formal analysis, Investigation, Methodology, Project administration, Resources, Software, Validation, Visualization, Writing – original draft, Writing – review & editing. JH: Formal analysis, Methodology, Resources, Writing – review & editing. GS: Methodology, Resources, Writing – review & editing. RT: Methodology, Resources, Writing – review & editing. YS: Methodology, Resources, Writing – review & editing. MeH: Resources, Writing – review & editing. S-CM: Resources, Writing – review & editing. MaH: Resources, Writing – review & editing. TK: Funding acquisition, Resources, Supervision, Validation, Writing – review & editing, Writing – original draft.
Funding
The author(s) declare financial support was received for the research, authorship, and/or publication of this article. This work was supported by NIH 1R01NS103529 and 1R01NS136808 to TK.
Acknowledgments
The authors acknowledge the assistance of Dr. Hong Zheng, the Stanford University Transgenic, Knockout and Tumor Model Center staff, and the SRI International veterinary care team.
Conflict of interest
Authors MaH was/were employed by F. Hoffmann-La Roche Ltd.
The remaining authors declare that the research was conducted in the absence of any commercial or financial relationships that could be construed as a potential conflict of interest.
Publisher’s note
All claims expressed in this article are solely those of the authors and do not necessarily represent those of their affiliated organizations, or those of the publisher, the editors and the reviewers. Any product that may be evaluated in this article, or claim that may be made by its manufacturer, is not guaranteed or endorsed by the publisher.
Supplementary material
The Supplementary Material for this article can be found online at: https://www.frontiersin.org/articles/10.3389/fpsyt.2024.1467964/full#supplementary-material
References
1. Borowsky B, Adham N, Jones KA, Raddatz R, Artymyshyn R, Ogozalek KL, et al. Trace amines: identification of a family of mammalian G protein-coupled receptors. Proc Natl Acad Sci USA. (2001) 98:8966–71. doi: 10.1073/pnas.151105198
2. Bunzow JR, Sonders MS, Arttamangkul S, Harrison LM, Zhang G, Quigley DI, et al. Amphetamine, 3,4-methylenedioxymethamphetamine, lysergic acid diethylamide, and metabolites of the catecholamine neurotransmitters are agonists of a rat trace amine receptor. Mol Pharmacol. (2001) 60:1181–8. doi: 10.1124/mol.60.6.1181
3. Berry MD, Gainetdinov RR, Hoener MC, Shahid M. Pharmacology of human trace amine-associated receptors: Therapeutic opportunities and challenges. Pharmacol Ther. (2017) 180:161–80. doi: 10.1016/j.pharmthera.2017.07.002
4. Gainetdinov RR, Hoener MC, Berry MD. Trace amines and their receptors. Pharmacol Rev. (2018) 70:549–620. doi: 10.1124/pr.117.015305
5. Lindemann L, Hoener MC. A renaissance in trace amines inspired by a novel GPCR family. Trends Pharmacol Sci. (2005) 26:274–81. doi: 10.1016/j.tips.2005.03.007
6. Revel FG, Moreau JL, Gainetdinov RR, Bradaia A, Sotnikova TD, Mory R, et al. TAAR1 activation modulates monoaminergic neurotransmission, preventing hyperdopaminergic and hypoglutamatergic activity. Proc Natl Acad Sci USA. (2011) 108:8485–90. doi: 10.1073/pnas.1103029108
7. Lindemann L, Meyer CA, Jeanneau K, Bradaia A, Ozmen L, Bluethmann H, et al. Trace amine-associated receptor 1 modulates dopaminergic activity. J Pharmacol Exp Ther. (2008) 324:948–56. doi: 10.1124/jpet.107.132647
8. Espinoza S, Lignani G, Caffino L, Maggi S, Sukhanov I, Leo D, et al. TAAR1 modulates cortical glutamate NMDA receptor function. Neuropsychopharmacology. (2015) 40:2217–27. doi: 10.1038/npp.2015.65
9. Xu Z, Guo L, Yu J, Shen S, Wu C, Zhang W, et al. Ligand recognition and G-protein coupling of trace amine receptor TAAR1. Nature. (2023) 624:672–81. doi: 10.1038/s41586-023-06804-z
10. Agren R, Betari N, Saarinen M, Zeberg H, Svenningsson P, Sahlholm K. In vitro comparison of ulotaront (SEP-363856) and ralmitaront (RO6889450): two TAAR1 agonist candidate antipsychotics. Int J Neuropsychopharmacol. (2023) 26:599–606. doi: 10.1093/ijnp/pyad049
11. Halff EF, Rutigliano G, Garcia-Hidalgo A, Howes OD. Trace amine-associated receptor 1 (TAAR1) agonism as a new treatment strategy for schizophrenia and related disorders. Trends Neurosci. (2023) 46:60–74. doi: 10.1016/j.tins.2022.10.010
12. Liu J, Wu R, Li JX. TAAR1 as an emerging target for the treatment of psychiatric disorders. Pharmacol Ther. (2024) 253:108580. doi: 10.1016/j.pharmthera.2023.108580
13. Revel FG, Moreau JL, Gainetdinov RR, Ferragud A, Velázquez-Sánchez C, Sotnikova TD, et al. Trace amine-associated receptor 1 partial agonism reveals novel paradigm for neuropsychiatric therapeutics. Biol Psychiatry. (2012) 72:934–42. doi: 10.1016/j.biopsych.2012.05.014
14. Revel FG, Moreau J-L, Pouzet B, Mory R, Bradaia A, Buchy D, et al. A new perspective for schizophrenia: TAAR1 agonists reveal antipsychotic- and antidepressant-like activity, improve cognition, and control body weight. Mol Psychiatry. (2013) 18:543–56. doi: 10.1038/mp.2012.57
15. Schwartz MD, Black SW, Fisher SP, Palmerston JB, Morairty SR, Hoener MC, et al. Trace amine-associated receptor 1 regulates wakefulness and EEG spectral composition. Neuropsychopharmacology. (2017) 42:1305–14. doi: 10.1038/npp.2016.216
16. Black SW, Schwartz MD, Chen TM, Hoener MC, Kilduff TS. Trace amine-associated receptor 1 agonists as narcolepsy therapeutics. Biol Psychiatry. (2017) 82:623–33. doi: 10.1016/j.biopsych.2016.10.012
17. Goonawardena AV, Morairty SR, Dell R, Orellana GA, Hoener MC, Wallace TL, et al. Trace amine-associated receptor 1 agonism promotes wakefulness without impairment of cognition in Cynomolgus macaques. Neuropsychopharmacology. (2019) 44:1485–93. doi: 10.1038/s41386-019-0386-8
18. Yang SM, Ghoshal A, Hubbard JM, Gackiere F, Teyssie R, Neale SA, et al. TAAR1 agonist ulotaront modulates striatal and hippocampal glutamate function in a state-dependent manner. Neuropsychopharmacology. (2024) 49:1091–103. doi: 10.1038/s41386-023-01779-x
19. Saarinen M, Mantas I, Flais I, Agren R, Sahlholm K, Millan MJ, et al. TAAR1 dependent and independent actions of the potential antipsychotic and dual TAAR1/5-HT(1A) receptor agonist SEP-383856. Neuropsychopharmacology. (2022) 47:2319–29. doi: 10.1038/s41386-022-01421-2
20. Liu H, Zheng Y, Wang Y, Wang Y, He X, Xu P, et al. Recognition of methamphetamine and other amines by trace amine receptor TAAR1. Nature. (2023) 624:663–71. doi: 10.1038/s41586-023-06775-1
21. Szabo ST, Hopkins SC, Lew R, Loebel A, Roth T, Koblan KS. A multicenter, double-blind, placebo-controlled, randomized, Phase 1b crossover trial comparing two doses of ulotaront with placebo in the treatment of narcolepsy-cataplexy. Sleep Med. (2023) 107:202–11. doi: 10.1016/j.sleep.2023.04.019
22. Nicoli A, Weber V, Bon C, Steuer A, Gustincich S, Gainetdinov RR, et al. Structure-based discovery of mouse trace amine-associated receptor 5 antagonists. J Chem Inf Model. (2023) 63:6667–80. doi: 10.1021/acs.jcim.3c00755
23. Efimova EV, Katolikova NV, Kanov EV, Gainetdinov RR. Trace amine-associated receptors at the cross-road between innate olfaction of amines, emotions, and adult neurogenesis. Neural Regener Res. (2022) 17:1257–8. doi: 10.4103/1673-5374.327338
24. Vaganova AN, Katolikova NV, Murtazina RZ, Kuvarzin SR, Gainetdinov RR. Public transcriptomic data meta-analysis demonstrates TAAR6 expression in the mental disorder-related brain areas in human and mouse brain. Biomolecules. (2022) 12(9):1259. doi: 10.3390/biom12091259
25. Efimova EV, Kuvarzin SR, Mor MS, Katolikova NV, Shemiakova TS, Razenkova V, et al. Trace amine-associated receptor 2 is expressed in the limbic brain areas and is involved in dopamine regulation and adult neurogenesis. Front Behav Neurosci. (2022) 16:847410. doi: 10.3389/fnbeh.2022.847410
26. Dinter J, Muhlhaus J, Wienchol CL, Yi CX, Nurnberg D, Morin S, et al. Inverse agonistic action of 3-iodothyronamine at the human trace amine-associated receptor 5. PLoS One. (2015) 10:e0117774. doi: 10.1371/journal.pone.0117774
27. Espinoza S, Sukhanov I, Efimova EV, Kozlova A, Antonova KA, Illiano P, et al. Trace amine-associated receptor 5 provides olfactory input into limbic brain areas and modulates emotional behaviors and serotonin transmission. Front Mol Neurosci. (2020) 13:18. doi: 10.3389/fnmol.2020.00018
28. Maggi S, Bon C, Gustincich S, Tucci V, Gainetdinov RR, Espinoza S. Improved cognitive performance in trace amine-associated receptor 5 (TAAR5) knock-out mice. Sci Rep. (2022) 12:14708. doi: 10.1038/s41598-022-18924-z
29. Saper CB, Scammell TE, Lu J. Hypothalamic regulation of sleep and circadian rhythms. Nature. (2005) 437:1257–63. doi: 10.1038/nature04284
30. Sherin JE, Shiromani PJ, McCarley RW, Saper CB. Activation of ventrolateral preoptic neurons during sleep. Science. (1996) 271:216–9. doi: 10.1126/science.271.5246.216
31. Adamantidis AR, de Lecea L. Sleep and the hypothalamus. Science. (2023) 382:405–12. doi: 10.1126/science.adh8285
32. Adamantidis AR, Zhang F, Aravanis AM, Deisseroth K, de Lecea L. Neural substrates of awakening probed with optogenetic control of hypocretin neurons. Nature. (2007) 450:420–4. doi: 10.1038/nature06310
33. Sulaman BA, Wang S, Tyan J, Eban-Rothschild A. Neuro-orchestration of sleep and wakefulness. Nat Neurosci. (2023) 26:196–212. doi: 10.1038/s41593-022-01236-w
34. Steriade M, McCormick DA, Sejnowski TJ. Thalamocortical oscillations in the sleeping and aroused brain. Science. (1993) 262:679–85. doi: 10.1126/science.8235588
35. Gozal EA, O'Neill BE, Sawchuk MA, Zhu H, Halder M, Chou CC, et al. Anatomical and functional evidence for trace amines as unique modulators of locomotor function in the mammalian spinal cord. Front Neural Circuits. (2014) 8:134. doi: 10.3389/fncir.2014.00134
36. Eban-Rothschild A, Rothschild G, Giardino WJ, Jones JR, de Lecea L. VTA dopaminergic neurons regulate ethologically relevant sleep-wake behaviors. Nat Neurosci. (2016) 19:1356–66. doi: 10.1038/nn.4377
37. Palagini L, Hertenstein E, Riemann D, Nissen C. Sleep, insomnia and mental health. J Sleep Res. (2022) 31:e13628. doi: 10.1111/jsr.13628
38. Benca RM. REM sleep and mental disorders on the 70th anniversary of the discovery of REM sleep. J Sleep Res. (2024) 29:e14364. doi: 10.1111/jsr.14364
39. Moderie C, Boivin DB. Diagnosing and treating hypersomnolence in depression. Sleep Med. (2024) 124:462–70. doi: 10.1016/j.sleep.2024.10.008
40. Shemiakova TS, Efimova EV, Gainetdinov RR. TAARs as novel therapeutic targets for the treatment of depression: A narrative review of the interconnection with monoamines and adult neurogenesis. Biomedicines. (2024) 12(6):1263. doi: 10.3390/biomedicines12061263
41. Harmeier A, Meyer CA, Staempfli A, Casagrande F, Petrinovic MM, Zhang YP, et al. How female mice attract males: A urinary volatile amine activates a trace amine-associated receptor that induces male sexual interest. Front Pharmacol. (2018) 9:924. doi: 10.3389/fphar.2018.00924
42. Sun Y, Tisdale R, Park S, Ma SC, Heu J, Haire M, et al. The development of sleep/wake disruption and cataplexy as hypocretin/orexin neurons degenerate in male vs. female Orexin/tTA; TetO-DTA Mice. Sleep. (2022) 45(12):zsac039. doi: 10.1093/sleep/zsac039
43. Park S, Heu J, Hoener MC, Kilduff TS. Wakefulness induced by TAAR1 partial agonism in mice is mediated through dopaminergic neurotransmission. Int J Mol Sci. (2024) 25(21):11351. doi: 10.3390/ijms252111351
44. Veasey SC, Yeou-Jey H, Thayer P, Fenik P. Murine Multiple Sleep Latency Test: phenotyping sleep propensity in mice. Sleep. (2004) 27:388–93. doi: 10.1093/sleep/27.3.388
45. Morairty SR, Dittrich L, Pasumarthi RK, Valladao D, Heiss JE, Gerashchenko D, et al. A role for cortical nNOS/NK1 neurons in coupling homeostatic sleep drive to EEG slow wave activity. Proc Natl Acad Sci USA. (2013) 110:20272–7. doi: 10.1073/pnas.1314762110
46. Schneider CA, Rasband WS, Eliceiri KW. NIH Image to ImageJ: 25 years of image analysis. Nat Methods. (2012) 9:671–5. doi: 10.1038/nmeth.2089
47. Allocca G, Ma S, Martelli D, Cerri M, Del Vecchio F, Bastianini S, et al. Validation of 'Somnivore', a machine learning algorithm for automated scoring and analysis of polysomnography data. Front Neurosci. (2019) 13:207. doi: 10.3389/fnins.2019.00207
48. Hakami T, Jones NC, Tolmacheva EA, Gaudias J, Chaumont J, Salzberg M, et al. NMDA receptor hypofunction leads to generalized and persistent aberrant gamma oscillations independent of hyperlocomotion and the state of consciousness. PLoS One. (2009) 4:e6755. doi: 10.1371/journal.pone.0006755
49. Pinault D. N-methyl d-aspartate receptor antagonists ketamine and MK-801 induce wake-related aberrant gamma oscillations in the rat neocortex. Biol Psychiatry. (2008) 63:730–5. doi: 10.1016/j.biopsych.2007.10.006
50. Ehrlichman RS, Gandal MJ, Maxwell CR, Lazarewicz MT, Finkel LH, Contreras D, et al. N-methyl-d-aspartic acid receptor antagonist-induced frequency oscillations in mice recreate pattern of electrophysiological deficits in schizophrenia. Neuroscience. (2009) 158:705–12. doi: 10.1016/j.neuroscience.2008.10.031
51. Lazarewicz MT, Ehrlichman RS, Maxwell CR, Gandal MJ, Finkel LH, Siegel SJ. Ketamine modulates theta and gamma oscillations. J Cogn Neurosci. (2010) 22:1452–64. doi: 10.1162/jocn.2009.21305
52. Franken P, Malafosse A, Tafti M. Genetic determinants of sleep regulation in inbred mice. Sleep. (1999) 22:155–69.
53. Franken P, Dijk DJ. Sleep and circadian rhythmicity as entangled processes serving homeostasis. Nat Rev Neurosci. (2024) 25:43–59. doi: 10.1038/s41583-023-00764-z
54. Dahan L, Astier B, Vautrelle N, Urbain N, Kocsis B, Chouvet G. Prominent burst firing of dopaminergic neurons in the ventral tegmental area during paradoxical sleep. Neuropsychopharmacology. (2007) 32:1232–41. doi: 10.1038/sj.npp.1301251
55. Sulaman BA, Zhang Y, Matosevich N, Kjaerby C, Foustoukos G, Andersen M, et al. Emerging functions of neuromodulation during sleep. J Neurosci. (2024) 44(40):e1277242024. doi: 10.1523/JNEUROSCI.1277-24.2024
56. Monti JM, Monti D. The involvement of dopamine in the modulation of sleep and waking. Sleep Med Rev. (2007) 11:113–33. doi: 10.1016/j.smrv.2006.08.003
57. Dzirasa K, Ribeiro S, Costa R, Santos LM, Lin SC, Grosmark A, et al. Dopaminergic control of sleep-wake states. J Neurosci. (2006) 26:10577–89. doi: 10.1523/JNEUROSCI.1767-06.2006
58. Franken P, Chollet D, Tafti M. The homeostatic regulation of sleep need is under genetic control. J Neurosci. (2001) 21:2610–21. doi: 10.1523/JNEUROSCI.21-08-02610.2001
Keywords: Trace amine-associated receptors, TAAR2-9, sleep regulation, wakefulness, NREM sleep, REM sleep, sleep deprivation, EEG spectral analysis
Citation: Park S, Heu J, Scheldrup G, Tisdale RK, Sun Y, Haire M, Ma S-C, Hoener MC and Kilduff TS (2025) Trace amine-associated receptors (TAARs)2-9 knockout mice exhibit reduced wakefulness and disrupted REM sleep. Front. Psychiatry 15:1467964. doi: 10.3389/fpsyt.2024.1467964
Received: 21 July 2024; Accepted: 24 December 2024;
Published: 29 January 2025.
Edited by:
Pramod C. Nair, Flinders University, AustraliaReviewed by:
Fabrizio Sanna, University of Cagliari, ItalyMasaru Tanaka, University of Szeged (ELKH-SZTE), Hungary
Copyright © 2025 Park, Heu, Scheldrup, Tisdale, Sun, Haire, Ma, Hoener and Kilduff. This is an open-access article distributed under the terms of the Creative Commons Attribution License (CC BY). The use, distribution or reproduction in other forums is permitted, provided the original author(s) and the copyright owner(s) are credited and that the original publication in this journal is cited, in accordance with accepted academic practice. No use, distribution or reproduction is permitted which does not comply with these terms.
*Correspondence: Thomas S. Kilduff, dGhvbWFzLmtpbGR1ZmZAc3JpLmNvbQ==