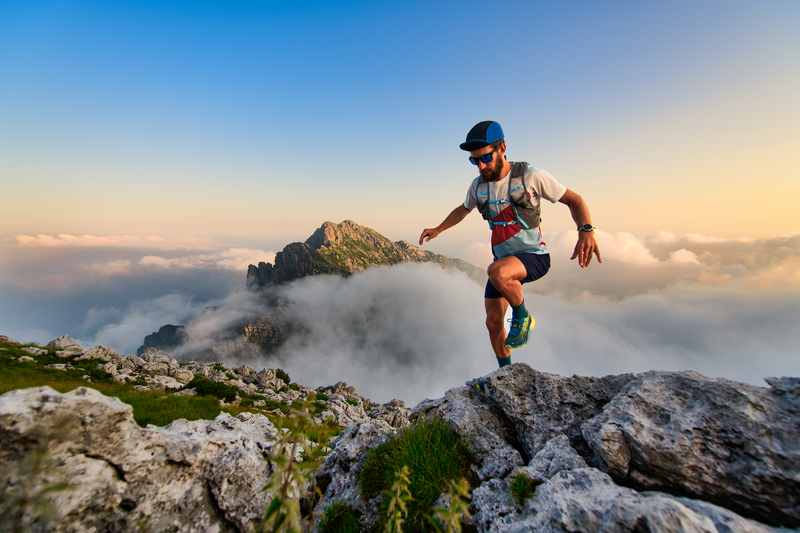
95% of researchers rate our articles as excellent or good
Learn more about the work of our research integrity team to safeguard the quality of each article we publish.
Find out more
MINI REVIEW article
Front. Psychiatry , 30 November 2023
Sec. Sleep Disorders
Volume 14 - 2023 | https://doi.org/10.3389/fpsyt.2023.1289367
This article is part of the Research Topic The Complex Relationship Between Sleep, Rhythms, and Mood Disorders: Volume II View all 8 articles
Obstructive sleep apnea is one of the most common sleep disorders with a high estimated global prevalence and a large number of associated comorbidities in general as well as specific neuropsychiatric complications such as cognitive impairment. The complex pathogenesis and effects of the disorder including chronic intermittent hypoxia and sleep fragmentation may lead to enhanced neuronal damage, thereby contributing to neuropsychiatric pathologies. Obstructive sleep apnea has been described as an independent risk factor for several neurodegenerative diseases, including Alzheimer's disease and all-cause dementia. The influence of obstructive sleep apnea on cognitive deficits is still a topic of recent debate, and several mechanisms, including neurodegeneration and depression-related cognitive dysfunction, underlying this correlation are taken into consideration. The differentiation between both pathomechanisms of cognitive impairment in obstructive sleep apnea is a complex clinical issue, requiring the use of multiple and costly diagnostic methods. The studies conducted on neuroprotection biomarkers, such as brain-derived neurotrophic factors and neurofilaments, are recently gaining ground in the topic of cognition assessment in obstructive sleep apnea patients. Neurofilaments as neuron-specific cytoskeletal proteins could be useful non-invasive indicators of brain conditions and neurodegeneration, which already are observed in many neurological diseases leading to cognitive deficits. Additionally, neurofilaments play an important role as a biomarker in other sleep disorders such as insomnia. Thus, this review summarizes the current knowledge on the involvement of neurofilaments in cognitive decline and neurodegeneration in obstructive sleep apnea patients as well as discusses its possible role as a biomarker of these changes.
Obstructive sleep apnea (OSA) refers to a common sleep-related breathing disorder characterized by recurrent episodes of apneas and hypopneas, resulting from repetitive upper-airway collapse (1). The estimated global prevalence of OSA ranges from 9 to 38%, and most cases remain undiagnosed due to non-specific manifestations, such as snoring, drowsiness, or excessive daytime sleepiness (2). Chronic intermittent hypoxia (CIH) and sleep fragmentation, as the main consequences of OSA, lead to enhanced neuronal damage and a decrease in synaptic plasticity, thereby contributing to neurological pathologies (3). The untreated disorder is an independent risk factor for (4, 5) any neurocognitive disorder, Alzheimer's disease, and Parkinson's disease (6). Since not every patient experiences these disorders, the potential influence of compensatory mechanisms among OSA patients was reported (7). Continuous positive airway pressure (CPAP) as the first-line treatment for OSA patients has been considered an efficacious method for preserving cognitive function in some articles (8, 9); however, it should be noted that not all patients can benefit from the proposed change and the available data are not conclusive enough to support its effectiveness (10).
Cognitive functioning refers to a collection of mental processes (e.g., perception, attention, memory, reasoning, and language skills) that are essential in comprehending the surrounding environment and acquiring knowledge. Cognitive performance declines with normal aging due to the accumulation of damage—neuronal structure alterations, synapse loss, and neuronal network dysfunction; however, it can be accelerated by some disorders (11). As a hallmark of OSA, CIH triggers cerebral hypoperfusion, endothelial dysfunction, and neuroinflammation, leading to blood-brain barrier dysfunction and cerebral small vessel disease; sleep fragmentation enhances the negative influence by increasing the production of oxygen species, coagulation, and sympathetic activity (12). Patients with OSA are more likely to exhibit deficits in attention and vigilance, long-term memory impairment, visuospatial dysgnosia, and executive dysfunction (13). The most prominent brain damage occurs in the prefrontal cortex, which is in charge of executive functions, and the greatest loss of gray matter localizes in the right basolateral amygdala/hippocampus (14, 15) (as shown in Figure 1).
Figure 1. The summary of pathophysiological pathways leading to cognitive impairment in obstructive sleep apnea (OSA) patients. Two main hallmarks of OSA—chronic intermittent hypoxia (CIH) and sleep fragmentation—may influence cognitive decline through several mechanisms. The main hypotheses regarding the involvement of CIH in this process include neuronal cytoskeletal destabilization, synaptic disruption, neuroinflammation, and cerebral hypoperfusion, which possibly lead to neuronal injury and a decline in cognitive functions. Sleep fragmentation in OSA patients may evoke neurodegenerative changes including amyloid-beta aggregation as well as microglia activation. Additionally, it may lead to impaired brain energy metabolism and neuroinflammation, which are the possible risk factors of cognitive decline. The changes in neurofilament levels may be a possible biomarker of pathomechanisms underlined in red color.
Opinions are divided on whether all cognitive deficits in OSA are associated with neuronal damage, pointing at pseudodementia as a culprit of abnormalities (16). Patients with OSA due to general deterioration in life quality are more likely to suffer from depressive symptoms which affect the cognitive performance. The differentiation between the early signs of neurodegeneration and depression-related cognitive dysfunction can be challenging and expensive to identify. The clinical symptoms associated with this condition are often indistinguishable, making the diagnosis a time-consuming and expensive process that may involve magnetic resonance imaging. Currently, the optimal diagnostic method has not yet been developed; however, detecting peripheral blood or cerebrospinal fluid biomarkers associated with the neuronal condition can be promising as a non-invasive and cost-effective solution for identifying cognitive decline even in the earliest stages, tracking deficits' progression, and predicting reversibility chances. Biomarkers of neuroprotection, for e.g., brain-derived neurotrophic factor (BDNF), insulin-like growth factor 1, and neurofilaments (NFs), as well as Alzheimer's disease-related biomarkers, for e.g., amyloid-β 42, phosphorylated tau, and total tau, are gaining ground (17–24). The determination of especially NFs (possible from CSF and/or plasma/serum), due to their specificity and ease of measurement, along with cognitive testing appears to be prospective in cognition assessment.
We conducted a review of current literature regarding biomarkers of neuroprotection and neurodegeneration, specifically on neurofilaments. Additionally, we examined the involvement of neurofilaments in sleep disorders with a particular emphasis on OSA and cognitive disorders associated with it. We searched the following databases using the following search queries: PubMed (search queries: “neurofilaments,” “sleep disorders and neurofilaments,” “OSA and neurofilaments,” “BDNF and OSA,” “neurodegeneration and OSA,” and “neurodegeneration and neurofilaments”); Google Scholar (search queries: “OSA and neurofilaments,” “sleep disorders and neurofilaments,” “insomnia and neurofilaments,” “neurofilaments and neurodegeneration,” “neurofilaments and OSA and cognition and biomarker,” “OSA and BDNF,” and “neurofilaments and sleep architecture”).
In this study, we aim to summarize the recent data regarding the involvement of NFs in the neurodegenerative processes in OSA. Furthermore, it is important to review the existing literature describing the usefulness of NF plasma concentration as a possible biomarker of cognitive decline in OSA patients.
The neurofilaments in the mature nervous system belong to a type IV intermediate filament family composed of quadruplet proteins—heavy, medium, light chain neurofilaments (abbreviated as NfH, NfM, and NfL, and encoded by NEFH, NEFM, and NEFL genes), and internexin (25). They are neuron-specific scaffolding subunits highly expressed in axons, providing structural stability vital to the outgrowth of axons (26). In addition, the NF structure consists of a highly conserved central domain composed of 310 amino acids specific to all intermediate filaments, providing a coiled-coil structure, a C-terminus with multiple lysine-serine-proline (KSP) motifs, and an N-terminus (27). The NF synthesis occurs constantly in the neurons' soma and the subunits combine to form 10-nm-diameter cytoplasmatic polymers, which are transported along axons by slow axonal transport (28). After reaching their destination, NFs undergo phosphorylation and glycosylation. The most extensive modifications occur in KSP repeats located in the tail. Both transport defects and phosphorylation disturbances were reported to cause neurodegeneration (29).
Impaired glucose metabolism was reported to trigger hyperphosphorylation of NFs, although there was no significant difference in overall NF levels; the change was accompanied by decreased protein O-GlcNAcylation in the cerebrum and tau hyperphosphorylation (30). The reciprocal relationship between O-GlcNAcylation and the level of NF phosphorylation at the head and tail domains containing KSP repeat motifs indicated the influence of post-transcriptional modification on the protein structure, resulting in shorter NFs with less assemblage, accumulating in somas rather than axons. In addition, improving O-GlcNAcylation as a target of treatment may result in fewer hyperphosphorylated NFs (31). Furthermore, phosphatase and tensin homolog/phosphorylated protein kinase B pathway dysregulation with cyclin-dependent kinase 5 and extracellular signal-regulated kinase involvement were connected with overall tau and NF hyperphosphorylation (32). Notably, the activation of extracellular signal-regulated kinase contributes to oxidative stress-triggered NF damage, which is found to be increased among OSA patients (33–35). Increased levels of non-phosphorylated NFs can alter homeostasis and contribute to neuronal degeneration; however, the exact etiology remains to be elucidated (36). The dysregulation of heat shock protein Hsp27 and alphaB-crystallin were reported to possibly take part in NFs' structure disturbances via kinase and phosphatase activity imbalance, leading to enhanced aggregation (37).
Among other cytoskeletal proteins (such as microtubules, actin filaments, and associated proteins), NFs are only specific to neurons, thereby making them useful indicators for non-invasive assessment of the brain condition by analogy with troponins in heart damage. NfL is the most abundant and soluble subunit of the neurofilament family, and therefore, it is the most studied. Neuro-axonal damage causes a release of NFs into the cerebrospinal fluid (CSF) and subsequently into the bloodstream. This may occur either passively or using exosomes (38). Compared to other brain injury biomarkers, such as BDNF or tau protein, NFs are not produced in the periphery, which can easily reflect the protein brain levels without errors (39). As we age, it is inevitable that thousands of neurons degenerate and are lost every day under normal circumstances Therefore, CSF/serum NFs maintain a relatively low constant level; however, pathologic conditions resulting in enhanced neuronal death increase the biomarker levels. NfL elevated levels have been observed in various neurological conditions, including Alzheimer's disease, mild cognitive impairment, frontotemporal dementia, amyotrophic lateral sclerosis, and multiple sclerosis (40–44).
Healthy sleep possesses neuroprotective effects in healthy individuals by participating in proper waste clearance, regaining neuronal sensitivity, and preventing reactive oxygen species overproduction (45). There is limited data available on the impact of sleep quality and related sleep disturbances on NFs. However, sleep disorders were reported to increase the risk of Alzheimer's disease, vascular dementia, and all-cause dementia (46). Sleep disturbances can be one of the first symptoms of ongoing brain damage, without other diagnostics (47).
Sleepiness can be associated with increased NfL levels, indicating a self-perpetuating cycle. The dependency may arise from the initial neuronal disruption that occurred independently from a sleep disorder and caused sleepiness through related neural network damage. Another explanation can start from exogenous stimuli, such as sleep depletion, which in consequence injure neurons and induce a release of NFs from cells (48). In general, sleep loss results in a depletion of global cognitive capacities, particularly reflected in attention and psychomotor vigilance impairment (49). In one study, one-night sleep loss among healthy subjects did not alter the levels of NfL, glial fibrillary acidic protein (GFAP), β-amyloid (Aβ) 40, and Aβ42; however, tau protein blood level was found to be increased (50). Another study indicated that serum levels of NfL in healthy subjects were significantly higher following one night of total sleep deprivation in a laboratory setting. Although the cognitive assessment did not statistically differ depending on the weight, obese subjects had increased pTau-181 levels, a biomarker of neurodegeneration (51). Apart from OSA participants described in this report, patients with chronic insomnia disorder exhibited higher serum levels of NfL, NfH, and NSE, which decreased after 6 months of treatment, indicating a possibility of reversal of the changes; the S100B protein level remained increased without significant alteration. The free therapy group of patients had lower cognitive performance scores measured by the Montreal Cognitive Assessment (MoCA) test compared to controls and those who underwent therapy (52). A different study found that levels of serum NfL and leukocyte telomere shortening (used as an aging marker) had been associated with female chronic insomnia patients' total sleep time, sleep efficiency, and sleep quality, measured by the Pittsburgh Sleep Quality Index (53). Contrarily, NF levels were not informative in narcolepsy type I, and sleep quality assessment, which was measured by the Medical Outcomes Study Sleep Scale, was altered in patients with Alzheimer's disease, suggesting an important role of sleep loss in NF alternations (54, 55).
NREM sleep loss was reported to trigger an increase in CSF and plasma NfL, while REM deprivation alone did not produce the change (56). It can be due to the disruption of NREM synaptic renormalization and cellular maintenance derived from the remodeling of cortical plasticity within slow-wave NREM sleep (57). The loss of N3 sleep can cause accelerated brain aging, resulting in lower cortical and subcortical brain volumes as observed in MRI (58). In patients with mild to moderate Alzheimer's disease, CSF NfL levels exhibited a positive correlation with stage 1 NREM sleep length and a negative correlation with stage 3 NREM sleep length (59). Patients with mild traumatic brain injury who experience poorer sleep quality were at a greater risk of developing OSA, measured by STOP-BANG scores, compared to subjects with better sleep quality. Additionally, poor sleepers had both increased peripheral NfL levels and lower cognitive performance (60). As OSA patients are likely to exhibit NREM sleep disruption in accordance to the scheme—stage N1 elongation and N3 diminution with an enhancement of alpha and beta wave frequency correlated with AHI—it may entail increase in extracellular NF levels (61–63). This pattern does not provide an adequate amount of slow-wave activity and transition into the REM phase, whereas light sleep expands and can cause more nocturnal awakenings, which, therefore, explains the increased tendency to daytime sleepiness complaints (64). In general, NREM sleep improves memory consolidation, reconsolidation, neurocognitive functions, and toxic waste removal, which is why being devoid of an appropriate amount of it can be reflected in CSF/blood NFs levels (see Figure 2).
Figure 2. Pathomechanisms of obstructive sleep apnea (OSA) leading to alterations in neurofilament homeostasis. Intermittent hypoxia in OSA is the main factor involved in neuronal cytoskeletal destabilization that leads to decreased neuronal excitability and neuronal injury. Sleep fragmentation in OSA contributes to neurodegeneration through neuroinflammation and loss of the neuroprotective function of healthy sleep. The characteristic disruption of sleep architecture in OSA patients, especially non-rapid eye movement (NREM) sleep disruption, has also been described to be involved in the process of neurodegeneration. The cytoskeletal changes in neurons and neuronal injury lead to a release of neurofilaments, as a main cytoskeletal protein providing neuronal structural stability, to cerebrospinal fluid and peripheral blood.
OSA through its main features, including CIH, sleep fragmentation, abnormal sympathetic activation, and low-grade inflammation, independently affects brain cells leading to cell stress and damage; however, CIH is the foremost triggering factor (see Figure 1). The latest data suggest nocturnal hypoxia is more associated with an increase in beta-amyloid 42 in the CSF fluid and exacerbated brain glucose uptake than with just sleep fragmentation (65, 66). Another influence of OSA on the central nervous system can be through disruption in the serotonergic system, as impaired serotonin neuromodulation affects both brainstem cardiorespiratory circuits and neurocognitive networks apart from its role in ventilatory stimulation (67–71). CIH and nitric oxide have been reported as inhibiting factors of tryptophan hydroxylase 1, one of the enzymes involved in serotonin synthesis from L-tryptophan (68). Thus, a decrease in serotonin synthesis observed among OSA patients can affect NFs' biology, but the potential connection hasn't been described yet.
It was reported that OSA may trigger neuroaxonal injury, particularly in the hippocampus as a hypoxia-sensitive area, resulting in ~6% gray matter loss during T1 signal MRI scan compared to healthy individuals (72). In addition, a 7-fold increase of apoptosis in the CA1 hippocampal region and an 8-fold increase in the adjacent cortex were detected in mice brains after 2 days of CIH exposure. Although the levels gradually decreased in 14 days, they were still elevated compared to controls (73). Cognitive decline is significantly influenced by the impact of CIH on cellular and chemical neuronal homeostasis (15, 74). In the brain of the intermittent hypoxia neonatal mouse model, the transcription of all NF subunits declined as follows: 20%—NfL and NfM, 30%—NfH (75). The degree of phosphorylation decreased in NfM and NfH, pointing to developed synaptic disruption as the effect of CIH on the neuronal network. Obtained cytoskeletal destabilization has put the NFs at a risk of proteolysis. The decreased neuronal excitability and impaired impulse conduction had been detected up to several weeks after exposure to CIH (75). In adult rats, CIH application during sleep resulted in spatial memory impairment, but not sensorimotor disturbances. Neurogenesis in the hippocampus measured by newly generated neurons immunohistochemical detection double labeling 5-bromo-2′-deoxyuridine connected with NFs decreased after early exposure and increased after 14 days of CIH, pointing to the presence of adaptive changes contributing to subsequent partial cognitive function improvement; however, the overall observation lasted only 30 days and the presumptive development of compensatory mechanisms in response to CIH exposure was not reflected by cognitive impairment amelioration (76). As untreated OSA is an independent risk factor for cognitive decline, it is conceivable that newly generated neurons degenerate within a few weeks of long-term CIH exposure, not alleviating neuronal damage (see Figure 1). Among humans, there was not any study directly measuring the condition of neuroplastic change; however, as brain imaging or behavioral tasks show, OSA patients do not experience improvement in the long term despite compensatory mechanisms, which may, in most cases remain insufficient without implemented treatment (77).
Sleep fragmentation has been reported as a factor directly contributing to not only subjective cognitive decline but also neurodegenerative disease development (78). Animal studies on mice established sleep fragmentation dysregulates endosome-autophagy-lysosome protein degradation pathways and induces neuroinflammation on neural networks. The mice subjected to chronic sleep fragmentation showed an increase in amyloid-beta aggregation in the prefrontal cortex and hippocampus compared to controls; in tissues, there was an enlargement of lysosomes and increase in Ras-related proteins Rab5/Rab7, indicating enhanced endosome activity. In addition, microglia activation reflected by IBA1/CD68 levels participated in changes similar to early-stage Alzheimer's disease development. The study group showed lower scores in cognitive testing and had increased anxiety (79). In addition, fluorodeoxyglucose positron emission tomography exposed impaired brain energy metabolism as an increase of glucose uptake in various parts of the limbic system: the hypothalamus, the hippocampus, and the amygdala. In the hippocampus, hyperphosphorylated tau clusters and gliosis indicating susceptibility to Alzheimer's disease development were detected. However, the differential gene expression pattern in the hippocampus did not correlate with the Alzheimer's disease model, normal aging model, and APOE4 mutation model, but correlated with the stress model (80). In the Alzheimer's disease mice model, sleep fragmentation aggravated the disease severity with an increase in hippocampal beta-amyloid and brain neuroinflammation. Interestingly, there was minimal difference in tau and phosphorylated tau levels in mice with developed Alzheimer's disease and concomitant sleep fragmentation compared to those without. That is why the influence of sleep disruption on tau protein may be important only in the initial development and early stages of neurodegenerative diseases (81).
The evaluation of serum NfL applicability as a general biomarker of brain condition measured as cognitive performance in OSA subjects was investigated only in the children's group. A retrospective study was conducted, wherein the group with both adenotonsillar hypertrophy and OSA exhibited higher NfL levels (31.68 pg/ml) compared to a group with adenotonsillar hypertrophy but without OSA (19.3 pg/ml) (82). The diagnostic discriminatory ability of the biomarker within the context of OSA was assessed as good with the area under the curve of 0.816 and with the use of a cutoff value of NfL of 18.75 pg/ml, resulting in 89.3% sensitivity and 61.2% specificity. NfL levels correlated with polysomnography parameters including Apnea-Hypopnea Index (AHI), Respiratory Arousal Index, and Oxygen Saturation Index, indicating a dependence between biomarker and OSA severity parameters. Cognitive status established using the Wechsler Intelligence Scale for Children (verbal, performance, and full-scale intelligence quotient measured) showed a significant correlation between serum levels of NfL and OSA-associated cognitive deficits (see Figure 2). In addition, intelligence parameters were associated with Oxygen Saturation Index and Respiratory Arousal Index, pointing to the fact that nocturnal severe hypoxemia and sleep fragmentation might be involved in substantial cognitive morbidity observed in OSA more than the number of apneas/hypopneas. Serum brain-derived tau protein as a neurodegenerative biomarker was measured as well; however, there was no significant difference between the group with and without OSA. Among adult OSA patients, NfL levels were correlated with the Oxygen Desaturation Index, but not with the AHI, indicating increasing hypoxia as a trigger of neuroaxonal damage; however, no neurocognitive assessment were performed (83). In another study, NfL levels of cognitively normal individuals with OSA did not correlate with the severity of the disease defined by the AHI values; it should be mentioned that the mean age of participants was 68.1, which makes the study not reliable in light of the current information that individuals with over 60 years are considered as having unstable biomarker levels due to occurring physiological aging (84, 85). Another research of cognitively normal middle-aged (median age 60.4 years) untreated OSA patients exhibited elevated NfL levels, associated with greater white matter hyperintensities quantified by magnetic resonance imaging and lower oxygen saturation—the AHI value was not related to NfL (86).
Research suggests that CPAP therapy among OSA patients can improve cognitive performance and have positive effects on both cardiovascular and metabolic risks (87–89). These changes can occur after reducing the occurrence of apneas/hypopneas and normalizing the sleep structure through sleep-dependent synaptic plasticity observed as brain volume increase (90). Brain imaging studies showed metabolite concentration markers change positively in response to CPAP treatment in mice, but not in humans (91, 92). The positive effect on the brain activity reflected by the task-positive network and default mode network was noted after 2 months of CPAP treatment among OSA patients (93). It is difficult to determine the level of reversibility and stability as there is a paucity of studies comprehensively focusing on brain plasticity and cognitive condition in response to CPAP in the short and long term. As observed, just one night without CPAP resulted in a decline of cognitive performance (94). It was observed whether CPAP therapy can impact serum levels of NfL, and the overnight change in biomarker levels was noted, whereas CPAP withdrawal resulted in an NfL increase, indicating the importance of early detection and institution of treatment (95).
As described above, the indication of peripheral NF levels among OSA patients can support traditional cognitive performance testing, extending the scope of knowledge about a patient's neuropsychological condition. Neurofilament biology and measurement of the ease of levels predispose them to be examined for utility as cognitive biomarkers. Although plenty of potential biomarkers have been tested so far (with the most significant including amyloid-β 42, phosphorylated tau, and total tau), they are not precise and thereby not clinically useful. What is important is that NFs directly reflect the current neural condition as they are part of the neuronal structure. They can easily diffuse through the blood-brain barrier to peripheral blood, which makes them easy and cheap to determine reliable biomarkers. Despite many hypothetical profits, the method has its limitations. First, there is only one study comparing the NF levels and cognitive performance among OSA patients; thus, in the case of this disease, the topic is not explored enough (see Table 1). Additionally, the study was carried out on children, not on adult OSA samples. Other studies comparing NF levels among OSA patients focused more on general dependencies between NF levels and polysomnographic features or the response to CPAP treatment. There was not any research comparing both cognitive performance and NF levels before and after CPAP application, but there were only indirect connections between them. Second, the indication of serum NF levels requires taking a blood sample and laboratory testing. Although the levels of NFs measured in peripheral blood are close to those in the cerebrospinal fluid, the differences can impact reasoning and comparing levels in cognitive change. In addition, the assessment of NF levels should not be the only method used in the cognition measurement; the biomarker can be the most useful while checking the progress of the disease and the influence of the instituted treatment. Therefore, the concept of using NFs as cognitive biomarkers should support traditional cognitive testing, providing a more precise description of the patient's cognitive performance.
Table 1. Studies concerning serum neurofilament light (NFL) levels in obstructive sleep apnea (OSA).
OSA and pathological changes associated with it may contribute to the neurodegenerative processes, making it an independent risk factor for cognitive decline. It has been previously described that both CIH and sleep fragmentation have a significant impact on neuronal homeostasis and cognition. CIH is thought to be responsible for neuroaxonal injury and apoptosis in hippocampal regions responsible for cognitive functioning through cytoskeletal destabilization. On the other hand, sleep fragmentation may induce neuroinflammation leading to neurodegeneration and cognition impairment. Both processes could possibly trigger the increase in the concentration of neurospecific biomarkers of neurodegeneration, such as NFs in plasma and CSF, which could serve as a predictor of cognitive decline. The recent studies on the correlation of NFs and OSA show that NF plasma concentration is positively correlated with the polysomnographic parameters of OSA severity, although there is only one study in the pediatric population showing the dependence of NF concentration and the significant decline in cognition in OSA patients. The emerging data suggest that CPAP therapy may also influence the NF concentration and lead to the improvement of cognitive functions in OSA patients. To our knowledge, this study is the first review to focus on the role of NFs in the neurodegenerative processes in OSA and its possible role as a biomarker of cognitive decline in OSA patients, comprehensively describing most recent studies. In light of these observations, it is important to further investigate NFs as the possible early biomarkers of neurodegeneration and cognitive impairment in OSA patients to properly monitor, adjust, and implement accurate therapy and its efficiency. From a future perspective, exploring the neurofilaments could provide an objective assessment of cognitive function decline; however, more studies are needed to be performed. New directions of research include comparisons between cognitive testing tools and NF levels, measured both in peripheral blood and CSF in OSA patients before and after CPAP treatment. Additionally, the disruption of NF gene expression might play a role in cognitive impairment development, which needs to be examined.
JJ: Conceptualization, Investigation, Writing – original draft. PK: Investigation, Writing – original draft, Visualization. DS: Writing – review & editing. MS: Writing – review & editing. PB: Writing – review & editing. AG: Conceptualization, Writing – review & editing.
The author(s) declare financial support was received for the research, authorship, and/or publication of this article. The study was funded by the Ministry of Education and Science (Poland) SKN/SP/571907/2023 and Medical University of Lodz, grant number 564/1-000-00/564-20-066 to JJ.
The authors declare that the research was conducted in the absence of any commercial or financial relationships that could be construed as a potential conflict of interest.
All claims expressed in this article are solely those of the authors and do not necessarily represent those of their affiliated organizations, or those of the publisher, the editors and the reviewers. Any product that may be evaluated in this article, or claim that may be made by its manufacturer, is not guaranteed or endorsed by the publisher.
1. Arnold J, Sunilkumar M, Krishna V, Yoganand S, Kumar M, Shanmugapriyan D. Obstructive sleep apnea. J Pharm Bioallied Sci. (2017) 9:S26. doi: 10.4103/jpbs.JPBS_155_17
2. Benjafield A V, Ayas NT, Eastwood PR, Heinzer R, Ip MSM, Morrell MJ, et al. Estimation of the global prevalence and burden of obstructive sleep apnoea: a literature-based analysis. Lancet Respir Med. (2019) 7:687–98. doi: 10.1016/S2213-2600(19)30198-5
3. Baril AA, Martineau-Dussault MÈ, Sanchez E, André C, Thompson C, Legault J, et al. Obstructive sleep apnea and the brain: a focus on gray and white matter structure. Curr Neurol Neurosci Rep. (2021) 21:1–10. doi: 10.1007/s11910-021-01094-2
4. Lutsey PL, Misialek JR, Mosley TH, Gottesman RF, Punjabi NM, Shahar E, et al. Sleep characteristics and risk of dementia and Alzheimer's disease: the Atherosclerosis Risk in Communities Study. Alzheimers Dement. (2018) 14:157–66. doi: 10.1016/j.jalz.2017.06.2269
5. Beaudin AE, Raneri JK, Ayas NT, Skomro RP, Fox N, Allen AJMH, et al. Cognitive function in a sleep clinic cohort of patients with obstructive sleep apnea. Ann Am Thorac Soc. (2021) 18:865–75. doi: 10.1513/AnnalsATS.202004-313OC
6. Guay-Gagnon M, Vat S, Forget MF, Tremblay-Gravel M, Ducharme S, Nguyen QD, et al. Sleep apnea and the risk of dementia: a systematic review and meta-analysis. J Sleep Res. (2022) 31:e13589. doi: 10.1111/jsr.13589
7. Teh JZ, Grummitt L, Haroutonian C, Cross NE, Skinner B, Bartlett DJ, et al. Overnight declarative memory consolidation and non-rapid eye movement sleep electroencephalographic oscillations in older adults with obstructive sleep apnea. Sleep. (2023) 46: doi: 10.1093/sleep/zsad087
8. Gabryelska A, Sochal M, Wasik B, Szczepanowski P, Białasiewicz P. Factors affecting long-term compliance of CPAP treatment-A single centre experience. J Clin Med. (2021) 11. doi: 10.3390/jcm11010139
9. Wang G, Goebel JR Li C, Hallman HG, Gilford TM Li W. Therapeutic effects of CPAP on cognitive impairments associated with OSA. J Neurol. (2020) 267:2823–8. doi: 10.1007/s00415-019-09381-2
10. Labarca G, Saavedra D, Dreyse J, Jorquera J, Barbe F. Efficacy of CPAP for improvements in sleepiness, cognition, mood, and quality of life in elderly patients with OSA: systematic review and meta-analysis of randomized controlled trials. Chest. (2020) 158:751–64. doi: 10.1016/j.chest.2020.03.049
11. Murman DL. The impact of age on cognition. Semin Hear. (2015) 36:111–21. doi: 10.1055/s-0035-1555115
12. Kerner NA, Roose SP. Obstructive sleep apnea is linked to depression and cognitive impairment: evidence and potential mechanisms. Am J Geriatr Psychiatry. (2016) 24:496–508. doi: 10.1016/j.jagp.2016.01.134
13. Olaithe M, Bucks RS, Hillman DR, Eastwood PR. Cognitive deficits in obstructive sleep apnea: Insights from a meta-review and comparison with deficits observed in COPD, insomnia, and sleep deprivation. Sleep Med Rev. (2018) 38:39–49. doi: 10.1016/j.smrv.2017.03.005
14. Tahmasian M, Rosenzweig I, Eickhoff SB, Sepehry AA, Laird AR, Fox PT, et al. Structural and functional neural adaptations in obstructive sleep apnea: an activation likelihood estimation meta-analysis. Neurosci Biobehav Rev. (2016) 65:142. doi: 10.1016/j.neubiorev.2016.03.026
15. Beebe DW, Gozal D. Obstructive sleep apnea and the prefrontal cortex: towards a comprehensive model linking nocturnal upper airway obstruction to daytime cognitive and behavioral deficits. J Sleep Res. (2002) 11:1–16. doi: 10.1046/j.1365-2869.2002.00289.x
16. Devita M, Montemurro S, Ramponi S, Marvisi M, Villani D, Raimondi MC, et al. Obstructive sleep apnea and its controversial effects on cognition. J Clin Exp Neuropsychol. (2017) 39:659–69. doi: 10.1080/13803395.2016.1253668
17. Siuda J, Patalong-Ogiewa M, Zmuda W, Targosz-Gajniak M, Niewiadomska E, Matuszek I, et al. Cognitive impairment and BDNF serum levels. Neurol Neurochir Pol. (2017) 51:24–32. doi: 10.1016/j.pjnns.2016.10.001
18. Gabryelska A, Turkiewicz S, Ditmer M, Karuga FF, Strzelecki D, Białasiewicz P, et al. BDNF and proBDNF serum protein levels in obstructive sleep apnea patients and their involvement in insomnia and depression symptoms. J Clin Med. (2022) 11. doi: 10.3390/jcm11237135
19. Gabryelska A, Sochal M. Evaluation of HIF-1 Involvement in the BDNF and probdnf signaling pathways among obstructive sleep apnea patients. Int J Mol Sci. (2022) 23. doi: 10.3390/ijms232314876
20. Gabryelska A, Turkiewicz S, Ditmer M, Sochal M. Neurotrophins in the neuropathophysiology, course, and complications of obstructive sleep apnea-a narrative review. Int J Mol Sci. (2023) 24. doi: 10.3390/ijms24031808
21. Paulsen AJ, Schubert CR, Pinto A, Carlsson CM, Chappell RJ, Fischer ME, et al. Neuroprotective biomarkers and cognitive function in a long-term prospective population-based study of aging US adults. Alzheimer Dis Assoc Disord. (2020) 34:31–9. doi: 10.1097/WAD.0000000000000341
22. Travica N, Berk M, Marx W. Neurofilament light protein as a biomarker in depression and cognitive function. Curr Opin Psychiatry. (2022) 35:30–7. doi: 10.1097/YCO.0000000000000756
23. Chen H, Gu S, Liu X, Xie A, Wang C. Association of blood amyloid beta-protein 1-42 with poststroke cognitive impairment: a systematic review and meta-analysis. Biomed Res Int. (2022) 2022:655278. doi: 10.1155/2022/6552781
24. Tondelli M, Salemme S, Vinceti G, Bedin R, Trenti T, Molinari MA, et al. Predictive value of phospho-tau/total-tau ratio in amyloid-negative Mild Cognitive Impairment. Neurosci Lett. (2022) 787:136811. doi: 10.1016/j.neulet.2022.136811
25. Yuan A, Rao MV, Sasaki T, Chen Y, Kumar A, Veeranna L, et al. Alpha-internexin is structurally and functionally associated with the neurofilament triplet proteins in the mature. CNS J Neurosci. (2006) 26:10006–19. doi: 10.1523/JNEUROSCI.2580-06.2006
26. Julien JP, Mushynski WE. Neurofilaments in health and disease. Prog Nucleic Acid Res Mol Biol. (1998) 61:1–23. doi: 10.1016/S0079-6603(08)60823-5
27. Gafson AR, Barthélemy NR, Bomont P, Carare RO, Durham HD, Julien JP, et al. Neurofilaments: neurobiological foundations for biomarker applications. Brain. (2020) 143:1975–98. doi: 10.1093/brain/awaa098
28. Yan Y, Brown A. Neurofilament polymer transport in axons. J Neurosci. (2005) 25:7014–21. doi: 10.1523/JNEUROSCI.2001-05.2005
29. Grant P, Pant HC. Neurofilament protein synthesis and phosphorylation. J Neurocytol. (2000) 29:843–72. doi: 10.1023/A:1010999509251
30. Deng Y, Li B, Liu Y, Iqbal K, Grundke-Iqbal I, Gong CX. Dysregulation of insulin signaling, glucose transporters, O-GlcNAcylation, and phosphorylation of tau and neurofilaments in the brain: Implication for Alzheimer's disease. Am J Pathol. (2009) 175:2089–98. doi: 10.2353/ajpath.2009.090157
31. Peng P, Wang J, Ding N, Zhou M, Gu Z, Shi Y, et al. Alteration of O-GlcNAcylation affects assembly and axonal transport of neurofilament via phosphorylation. Neurosci Lett. (2019) 698:97–104. doi: 10.1016/j.neulet.2018.11.001
32. Nayeem N, Kerr F, Naumann H, Linehan J, Lovestone S, Brandner S. Hyperphosphorylation of tau and neurofilaments and activation of CDK5 and ERK1/2 in PTEN-deficient cerebella. Mol Cell Neurosci. (2007) 34:400–8. doi: 10.1016/j.mcn.2006.11.014
33. Perry G, Roder H, Nunomura A, Takeda A, Friedlich AL, Zhu X, et al. Activation of neuronal extracellular receptor kinase (ERK) in Alzheimer disease links oxidative stress to abnormal phosphorylation. Neuroreport. (1999) 10:2411–5. doi: 10.1097/00001756-199908020-00035
34. Turkiewicz S, Ditmer M, Sochal M, Białasiewicz P, Strzelecki D, Gabryelska A. Obstructive sleep apnea as an acceleration trigger of cellular senescence processes through telomere shortening. Int J Mol Sci. (2021) 22. doi: 10.3390/ijms222212536
35. Turkiewicz S, Sochal M, Ditmer M, Gabryelska A. Evaluation of oxidative stress in obstructive sleep apnea and its comorbidities using serum concentrations of oxidized guanine species. Pol Arch Intern Med. (2023). doi: 10.20452/pamw.16542
36. Kreutzer M, Seehusen F, Kreutzer R, Pringproa K, Kummerfeld M, Claus P, et al. Axonopathy is associated with complex axonal transport defects in a model of multiple sclerosis. Brain Pathol. (2012) 22:454–71. doi: 10.1111/j.1750-3639.2011.00541.x
37. Björkdahl C, Sjögren MJ, Zhou X, Concha H, Avila J, Winblad B, et al. Small heat shock proteins Hsp27 or alphaB-crystallin and the protein components of neurofibrillary tangles: tau and neurofilaments. J Neurosci Res. (2008) 86:1343–52. doi: 10.1002/jnr.21589
38. Lachenal G, Pernet-Gallay K, Chivet M, Hemming FJ, Belly A, Bodon G, et al. Release of exosomes from differentiated neurons and its regulation by synaptic glutamatergic activity. Mol Cell Neurosci. (2011) 46:409–18. doi: 10.1016/j.mcn.2010.11.004
39. Sochal M, Ditmer M, Gabryelska A, Białasiewicz P. The role of brain-derived neurotrophic factor in immune-related diseases: a narrative review. J Clin Med. (2022) 11. doi: 10.3390/jcm11206023
40. Koychev I, Jansen K, Dette A, Shi L, Holling H. Blood-based ATN biomarkers of Alzheimer's disease: a meta-analysis. J Alzheimers Dis. (2021) 79:177–95. doi: 10.3233/JAD-200900
41. Zhang J, Cheng H, Liu W, Li H, Song Y, Jia L. Neurofilament light chain in cerebrospinal fluid or blood as a biomarker for mild cognitive impairment: a systematic review and meta-analysis. Medicine. (2022) 101:E28932. doi: 10.1097/MD.0000000000028932
42. Karantali E, Kazis D, Chatzikonstantinou S, Petridis F, Mavroudis I. The role of neurofilament light chain in frontotemporal dementia: a meta-analysis. Aging Clin Exp Res. (2021) 33:869–81. doi: 10.1007/s40520-020-01554-8
43. Sferruzza G, Bosco L, Falzone YM, Russo T, Domi T, Quattrini A, et al. Neurofilament light chain as a biological marker for amyotrophic lateral sclerosis: a meta-analysis study. Amyotroph Lateral Scler Frontotemporal Degener. (2022) 23:446–57. doi: 10.1080/21678421.2021.2007952
44. Ning L, Wang B. Neurofilament light chain in blood as a diagnostic and predictive biomarker for multiple sclerosis: A systematic review and meta-analysis. PLoS ONE. (2022) 17:e0274565. doi: 10.1371/journal.pone.0274565
46. Shi L, Chen SJ, Ma MY, Bao YP, Han Y, Wang YM, et al. Sleep disturbances increase the risk of dementia: a systematic review and meta-analysis. Sleep Med Rev. (2018) 40:4–16. doi: 10.1016/j.smrv.2017.06.010
47. Guarnieri B, Adorni F, Musicco M, Appollonio I, Bonanni E, Caffarra P, et al. Prevalence of sleep disturbances in mild cognitive impairment and dementing disorders: a multicenter Italian clinical cross-sectional study on 431 patients. Dement Geriatr Cogn Disord. (2012) 33:50–8. doi: 10.1159/000335363
48. Carvalho DZ, St. Louis EK, Przybelski SA, Morgenthaler TI, Machulda MM, Boeve BF, et al. Sleepiness in cognitively unimpaired older adults is associated with CSF biomarkers of inflammation and axonal integrity. Front Aging Neurosci. (2022) 14:930315. doi: 10.3389/fnagi.2022.930315
49. Killgore WDS. Effects of sleep deprivation on cognition. Prog Brain Res. (2010) 185:105–29. doi: 10.1016/B978-0-444-53702-7.00007-5
50. Benedict C, Blennow K, Zetterberg H, Cedernaes J. Effects of acute sleep loss on diurnal plasma dynamics of CNS health biomarkers in young men. Neurology. (2020) 94:e1181–9. doi: 10.1212/WNL.0000000000008866
51. van Egmond LT, Bukhari S, Benedet AL, Ashton NJ, Meth EMS, Boukas A, et al. Acute sleep loss increases CNS health biomarkers and compromises the ability to stay awake in a sex-and weight-specific manner. Transl Psychiatry. (2022) 12. doi: 10.1038/s41398-022-02146-y
52. Zhang P, Tan CW, Chen GH, Ge YJ, Xu J, Xia L, et al. Patients with chronic insomnia disorder have increased serum levels of neurofilaments, neuron-specific enolase and S100B: does organic brain damage exist? Sleep Med. (2018) 48:163–71. doi: 10.1016/j.sleep.2017.12.012
53. Ren CY, Liu PP Li J, Li YQ, Zhang LJ, Chen GH, Dong FY, et al. Changes in telomere length and serum neurofilament light chain levels in female patients with chronic insomnia disorder. J Clin Sleep Med. (2022) 18:383–92. doi: 10.5664/jcsm.9574
54. Baiardi S, Pizza F, Polischi B, Moresco M, Abu-Rumeileh S, Plazzi G, Parchi P. Cerebrospinal fluid biomarkers of neurodegeneration in narcolepsy type 1. Sleep. (2020) 43:zsz215 doi: 10.1093/sleep/zsz215
55. Sprecher KE, Koscik RL, Carlsson CM, Zetterberg H, Blennow K, Okonkwo OC, et al. Poor sleep is associated with CSF biomarkers of amyloid pathology in cognitively normal adults. Neurology. (2017) 89:445–53. doi: 10.1212/WNL.0000000000004171
56. Liu S, Zhang Z, Shi S, Meng Y, Zhang X, Lei Q, et al. NREM sleep loss increases neurofilament light chain levels in APP/PS1 and C57BL/6 J mice. Sleep Breath. (2023) 27. doi: 10.1007/s11325-022-02719-7
57. Nissen C, Piosczyk H, Holz J, Maier JG, Frase L, Sterr A, et al. Sleep is more than rest for plasticity in the human cortex. Sleep. (2021) 44:zsaa216. doi: 10.1093/sleep/zsaa216
58. Baril AA, Beiser AS, Mysliwiec V, Sanchez E, Decarli CS, Redline S, et al. Slow-wave sleep and MRI markers of brain aging in a community-based sample. Neurology. (2021) 96:e1462–9. doi: 10.1212/WNL.0000000000011377
59. Targa A, Dakterzada F, Benítez I, López R, Pujol M, Dalmases M, et al. Decrease in sleep depth is associated with higher cerebrospinal fluid neurofilament light levels in patients with Alzheimer's disease. Sleep. (2021) 44:zsaa147. doi: 10.1093/sleep/zsaa147
60. Werner JK, Shahim P, Pucci JU, Lai C, Raiciulescu S, Gill JM, et al. Poor sleep correlates with biomarkers of neurodegeneration in mild traumatic brain injury patients: a CENC study. Sleep. (2021) 44:1–8. doi: 10.1093/sleep/zsaa272
61. Appleton SL, Vakulin A, D'Rozario A, Vincent AD, Teare A, Martin SA, et al. Quantitative electroencephalography measures in rapid eye movement and nonrapid eye movement sleep are associated with apnea-hypopnea index and nocturnal hypoxemia in men. Sleep. (2019) 42:zsz092. doi: 10.1093/sleep/zsz092
62. Guilleminault C, Do Kim Y, Chowdhuri S, Horita M, Ohayon M, Kushida C. Sleep and daytime sleepiness in upper airway resistance syndrome compared to obstructive sleep apnoea syndrome. Eur Respir J. (2001) 17:838–47. doi: 10.1183/09031936.01.17508380
63. Vakulin A, D'Rozario A, Kim JW, Watson B, Cross N, Wang D, et al. Quantitative sleep EEG and polysomnographic predictors of driving simulator performance in obstructive sleep apnea. Clin Neurophysiol. (2016) 127:1428–35. doi: 10.1016/j.clinph.2015.09.004
64. Patel AK, Reddy V, Shumway KR, Araujo JF. Physiology, Sleep Stages. StatPearls (2022). Available online at: https://www.ncbi.nlm.nih.gov/books/NBK526132/ (accessed September 4, 2023).
65. Kaczmarski P, Karuga FF, Szmyd B, Sochal M, Białasiewicz P, Strzelecki D, et al. The role of inflammation, hypoxia, and opioid receptor expression in pain modulation in patients suffering from obstructive sleep apnea. Int J Mol Sci. (2022) 23. doi: 10.3390/ijms23169080
66. Fernandes M, Chiaravalloti A, Manfredi N, Placidi F, Nuccetelli M, Izzi F, et al. Nocturnal hypoxia and sleep fragmentation may drive neurodegenerative processes: the compared effects of obstructive sleep apnea syndrome and periodic limb movement disorder on Alzheimer's disease biomarkers. J Alzheimers Dis. (2022) 88:127–39. doi: 10.3233/JAD-215734
67. Wieckiewicz M, Martynowicz H, Lavigne G, Lobbezoo F, Kato T, Winocur E, et al. An exploratory study on the association between serotonin and sleep breathing disorders. Sci Rep. (2023) 13:1–9. doi: 10.1038/s41598-023-38842-y
68. Hilaire G, Voituron N, Menuet C, Ichiyama RM, Subramanian HH, Dutschmann M. The role of serotonin in respiratory function and dysfunction. Respir Physiol Neurobiol. (2010) 174:76–88. doi: 10.1016/j.resp.2010.08.017
69. Tangen Ä, Veldman ER, Svensson J, Tiger M, Nord M, Sorjonen K, et al. Associations between cognition and serotonin 1b receptor availability in healthy volunteers: a [11C]AZ10419369 positron emission tomography study. Int J Neuropsychopharmacol. (2023) 26:241–8. doi: 10.1093/ijnp/pyac084
70. Deza-Araujo YI, Baez-Lugo S, Vuilleumier P, Chocat A, Chételat G, Poisnel G, et al. Whole blood serotonin levels in healthy elderly are negatively associated with the functional activity of emotion-related brain regions. Biol Psychol. (2021) 160:108051. doi: 10.1016/j.biopsycho.2021.108051
71. Tripathi A, Bagchi S, Singh J, Tripathi S, Gupta NK, Arora V. Incidence of obstructive sleep apnea in elderly edentulous patients and the possible correlation of serum serotonin and apnea-hypopnea index. J Prosthodont. (2019) 28:e843–8. doi: 10.1111/jopr.12654
72. Morrell MJ, McRobbie DW, Quest RA, Cummin ARC, Ghiassi R, Corfield DR. Changes in brain morphology associated with obstructive sleep apnea. Sleep Med. (2003) 4:451–4. doi: 10.1016/S1389-9457(03)00159-X
73. Gozal D, Daniel JM, Dohanich GP. Behavioral and anatomical correlates of chronic episodic hypoxia during sleep in the rat. J Neurosci. (2001) 21:2442–50. doi: 10.1523/JNEUROSCI.21-07-02442.2001
74. Kaczmarski P, Sochal M, Strzelecki D, Białasiewicz P, Gabryelska A. Influence of glutamatergic and GABAergic neurotransmission on obstructive sleep apnea. Front Neurosci. (2023) 17:1213971. doi: 10.3389/fnins.2023.1213971
75. Cai J, Tuong CM, Zhang Y, Shields CB, Guo G, Fu H, et al. Mouse intermittent hypoxia mimicking apnoea of prematurity: effects on myelinogenesis and axonal maturation. J Pathol. (2012) 226:495–508. doi: 10.1002/path.2980
76. Gozal D, Row BW, Gozal E, Kheirandish L, Neville JJ, Brittian KR, et al. Temporal aspects of spatial task performance during intermittent hypoxia in the rat: evidence for neurogenesis. Eur J Neurosci. (2003) 18:2335–42. doi: 10.1046/j.1460-9568.2003.02947.x
77. Kim H, Joo EY, Suh S, Kim JH, Kim ST, Hong SB. Effects of long-term treatment on brain volume in patients with obstructive sleep apnea syndrome. Hum Brain Mapp. (2016) 37:395–409. doi: 10.1002/hbm.23038
78. Manousakis JE, Scovelle AJ, Rajaratnam SMW, Naismith SL, Anderson C. Advanced circadian timing and sleep fragmentation differentially impact on memory complaint subtype in subjective cognitive decline. J Alzheimers Dis. (2018) 66:565–77. doi: 10.3233/JAD-180612
79. Xie Y, Ba L, Wang M, Deng SY, Chen SM, Huang LF, et al. Chronic sleep fragmentation shares similar pathogenesis with neurodegenerative diseases: endosome-autophagosome-lysosome pathway dysfunction and microglia-mediated neuroinflammation. CNS Neurosci Ther. (2020) 26:215–27. doi: 10.1111/cns.13218
80. Ba L, Huang L, He Z, Deng S, Xie Y, Zhang M, et al. Does chronic sleep fragmentation lead to Alzheimer's disease in young wild-type mice? Front Aging Neurosci. (2021) 13:759983. doi: 10.3389/fnagi.2021.759983
81. Duncan MJ, Guerriero LE, Kohler K, Beechem LE, Gillis BD, Salisbury F, et al. Chronic fragmentation of the daily sleep-wake rhythm increases amyloid-beta levels and neuroinflammation in the 3xTg-AD mouse model of Alzheimer's disease. Neuroscience. (2022) 481:111–22. doi: 10.1016/j.neuroscience.2021.11.042
82. Shi Y, Feng Y, Chen X, Ma L, Cao Z, Shang L, et al. Serum neurofilament light reflects cognitive dysfunctions in children with obstructive sleep apnea. BMC Pediatr. (2022) 22. doi: 10.1186/s12887-022-03514-9
83. Arslan B, Semsi R, Iriz A, Sepici Dinçel A. The evaluation of serum brain-derived neurotrophic factor and neurofilament light chain levels in patients with obstructive sleep apnea syndrome. Laryngoscope Investig Otolaryngol. (2021) 6:1466–73. doi: 10.1002/lio2.683
84. Khalil M, Pirpamer L, Hofer E, Voortman MM, Barro C, Leppert D, et al. Serum neurofilament light levels in normal aging and their association with morphologic brain changes. Nat Commun. (2020) 11. doi: 10.1038/s41467-020-14612-6
85. Michael O, Bubu M, Queen Umasabor-Bubu O, Williams ET, Turner AD, Williams N, et al. Interaction of obstructive sleep apnea severity and amyloid burden on novel plasma biomarkers of tau and neurofilament light protein in community-dwelling cognitively normal older-adults. Alzheimers Dement. (2021) 17:e055491. doi: 10.1002/alz.055491
86. Sprecher K, Derynda B, Riedner B, Zetterberg H, Blennow K, Carlsson C, et al. 0282 White matter damage and axonal degeneration are related to hypoxia in untreated obstructive sleep apnea. Sleep. (2017) 40:A104–A104. doi: 10.1093/sleepj/zsx050.281
87. Li J, Yan W, Yi M, Lin R, Huang Z, Zhang Y. Efficacy of CPAP duration and adherence for cognitive improvement in patients with obstructive sleep apnea: a meta-analysis of randomized controlled trials. Sleep Breath. (2023) 27:973–82. doi: 10.1007/s11325-022-02687-y
88. Malicki M, Karuga FF, Szmyd B, Sochal M, Gabryelska A. Obstructive sleep apnea, circadian clock disruption, and metabolic consequences. Metabolites. (2022) 13. doi: 10.3390/metabo13010060
89. Karuga FF, Jaromirska J, Malicki M, Sochal M, Szmyd B, Białasiewicz P, et al. The role of microRNAs in pathophysiology and diagnostics of metabolic complications in obstructive sleep apnea patients. Front Mol Neurosci. (2023) 16:1208886. doi: 10.3389/fnmol.2023.1208886
90. Canessa N, Castronovo V, Cappa SF, Aloia MS, Marelli S, Falini A, et al. Obstructive sleep apnea: brain structural changes and neurocognitive function before and after treatment. Am J Respir Crit Care Med. (2011) 183:1419–26. doi: 10.1164/rccm.201005-0693OC
91. Douglas RM, Miyasaka N, Takahashi K, Latuszek-Barrantes A, Haddad GG, Hetherington HP. Chronic intermittent but not constant hypoxia decreases NAA/Cr ratios in neonatal mouse hippocampus and thalamus. Am J Physiol Regul Integr Comp Physiol. (2007) 292. doi: 10.1152/ajpregu.00404.2006
92. O'Donoghue FJ, Wellard RM, Rochford PD, Dawson A, Barnes M, Ruehland WR, et al. Magnetic resonance spectroscopy and neurocognitive dysfunction in obstructive sleep apnea before and after CPAP treatment. Sleep. (2012) 35:41–8. doi: 10.5665/sleep.1582
93. Prilipko O, Huynh N, Schwartz S, Tantrakul V, Kushida C, Paiva T, et al. The effects of CPAP treatment on task positive and default mode networks in obstructive sleep apnea patients: an fMRI study. PLoS ONE. (2012) 7:e0047433. doi: 10.1371/journal.pone.0047433
94. Kribbs NB, Pack AI, Kline LR, Getsy JE, Schuett JS, Henry JN, et al. Effects of one night without nasal CPAP treatment on sleep and sleepiness in patients with obstructive sleep apnea. Am Rev Respir Dis. (1993) 147:1162–8. doi: 10.1164/ajrccm/147.5.1162
Keywords: obstructive sleep apnea, chronic intermittent hypoxia, neurofilaments, cognitive impairment, biomarkers, neurodegeneration
Citation: Jaromirska J, Kaczmarski P, Strzelecki D, Sochal M, Białasiewicz P and Gabryelska A (2023) Shedding light on neurofilament involvement in cognitive decline in obstructive sleep apnea and its possible role as a biomarker. Front. Psychiatry 14:1289367. doi: 10.3389/fpsyt.2023.1289367
Received: 05 September 2023; Accepted: 30 October 2023;
Published: 30 November 2023.
Edited by:
Helena Martynowicz, Wroclaw Medical University, PolandReviewed by:
Mieszko Wieckiewicz, Wroclaw Medical University, PolandCopyright © 2023 Jaromirska, Kaczmarski, Strzelecki, Sochal, Białasiewicz and Gabryelska. This is an open-access article distributed under the terms of the Creative Commons Attribution License (CC BY). The use, distribution or reproduction in other forums is permitted, provided the original author(s) and the copyright owner(s) are credited and that the original publication in this journal is cited, in accordance with accepted academic practice. No use, distribution or reproduction is permitted which does not comply with these terms.
*Correspondence: Agata Gabryelska, YWdhdGEuZ2FicnllbHNrYUBnbWFpbC5jb20=
Disclaimer: All claims expressed in this article are solely those of the authors and do not necessarily represent those of their affiliated organizations, or those of the publisher, the editors and the reviewers. Any product that may be evaluated in this article or claim that may be made by its manufacturer is not guaranteed or endorsed by the publisher.
Research integrity at Frontiers
Learn more about the work of our research integrity team to safeguard the quality of each article we publish.