- Department of Chemistry, University of Pittsburgh, Pittsburgh, PA, United States
In contrast to well established psychedelics such as lysergic acid diethylamide (LSD) and psilocybin, ergot alkaloids of the clavine subclass have not been thoroughly investigated, in spite of their broad occurrence in nature and their well-established potent physiological effects. This study presents the current knowledge on the biological properties of clavine alkaloids, draws comparisons to the pharmacology of ergolines and related psychedelics, and demonstrates opportunities to develop novel structure–activity relationship (SAR) profiles. The latter could usher in a new stage of medicinal chemistry studies that enable an expansion of the currently structurally limited portfolio of psychedelic therapeutics.
1 Introduction
Psychedelic compounds have been used by a variety of cultures for centuries due to their ability to elicit an altered state of consciousness, evoke transcendence, reopen the social reward learning critical period, and to heal a variety of health disorders (1). Traditionally used by communities in South America and Africa for religious ceremonies and rituals, these compounds share a potential for substance abuse but are now re-emerging as potential therapeutics in North America and Europe (2). The demand for psychedelic therapy (3) and psychedelic wellness retreats (4, 5) has seen tremendous growth in recent years, in part driven by microdosing applications and the combination with traditional psychotherapeutic counseling. Users experience an altered state that involves changes in sensory perception and mood and may undergo dissociation or a loss of self-identity (6–10). An ability to reset a social reward learning mechanism might be a shared feature across psychedelic drugs (11). Interestingly, some users claim that a psychedelic experience permanently changed their outlook on life and/or improved their quality of life long after the experience (12). Although these claims are subjective, there is increasing evidence that suggests psychedelics can be used to treat psychiatric diseases, including anxiety and major depressive disorders (13, 14).
Historically, scientific research into psychedelics and their therapeutic benefits has been slow and controversial. Classic psychedelics including lysergic acid diethylamide (LSD), psilocybin, dimethyltryptamine (DMT), and mescaline were used in the 1950s and 60s to treat alcoholism, addiction, anxiety, and trauma (Figure 1) (15, 16). LSD was also used to obtain a model for psychosis and schizophrenia due to its ability to elicit symptoms of these disorders at high doses (17–19). Unfortunately, there were growing concerns of abuse regarding psychedelics and these compounds became associated with counterculture. As a result, the Drug Abuse Control Amendments of 1965 and the Controlled Substances Act of 1970 were passed. These policies severely limited the availability of psychedelic compounds for researchers and clinical investigations into these substances were stalled. Twenty years later, many seminal and novel studies on psychedelic compounds emerged. Among the earliest examples are Rick Strassman’s studies on the dose dependent effects of DMT in humans (20, 21). These promising psychiatric and clinical investigations of psychedelics have brought these compounds out of the dark ages (22). Indeed, this new wave of vibrant research into psychedelic compounds has ushered in a “psychedelic renaissance.” (23).
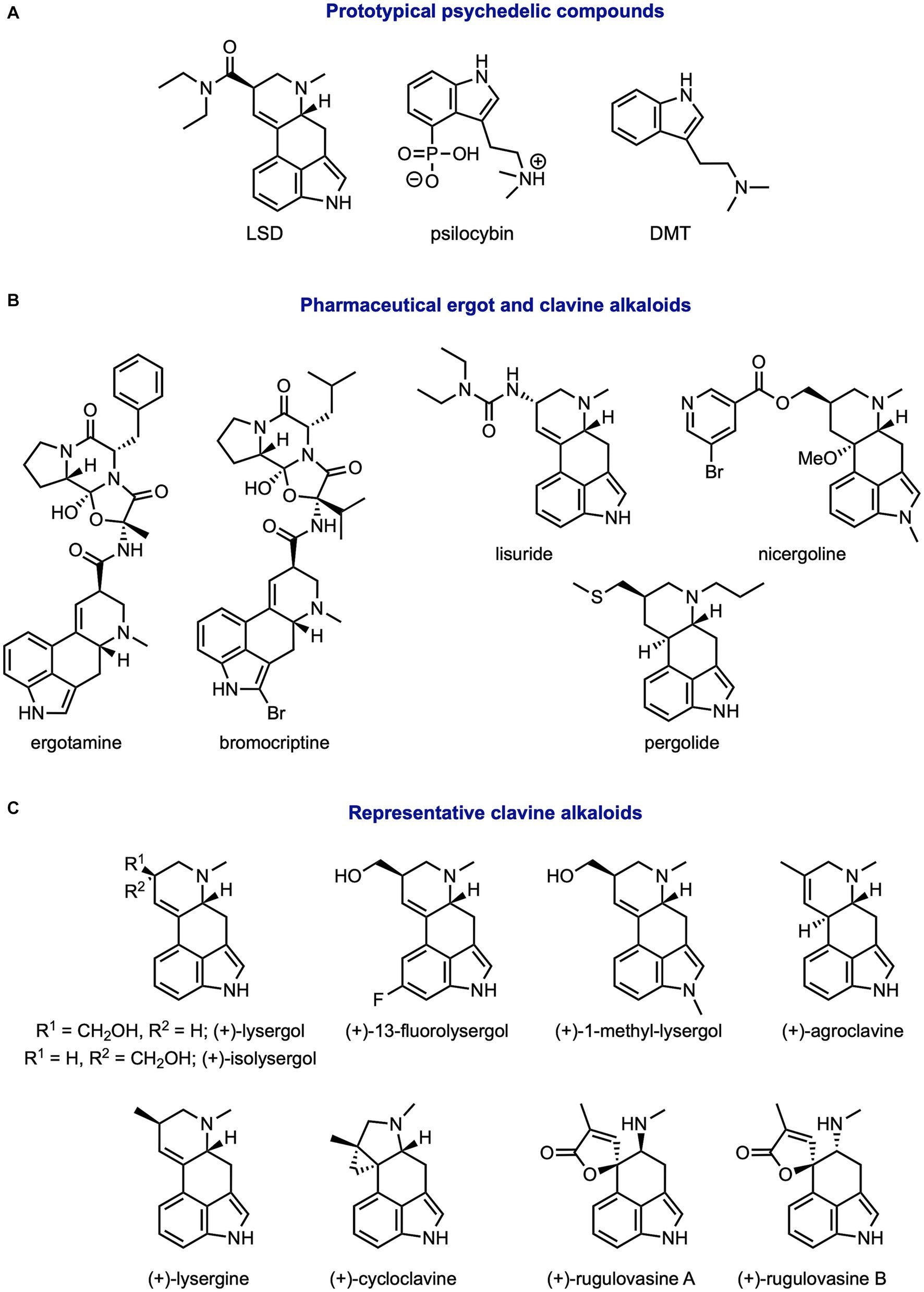
Figure 1. Structures of common ergot and clavine alkaloids. (A) Prototypical psychedelics, (B) pharmaceutical alkaloids, and (C) other studied clavine alkaloids.
Considerable efforts have been expended to elucidate how psychedelics and structurally related compounds elicit their unique biological responses. LSD, an ergot alkaloid and a prototypical psychedelic, has strong interactions with aminergic G-protein-coupled receptors (GPCRs), including serotonin, dopamine, histamine, adrenergic and muscarinic receptors (24–30). Recently, Lewis et al. screened LSD at 33 human aminergic GPCRs using a G protein dissociation BRET-based assay as part of a pharmacological profiling of a non-hallucinogenic LSD analog (vide infra) (31). The receptors were ranked by calculating a relative activity (log EMAX/EC50), and the top-ranked GPCRs included all five of the 5-HT1 and the three 5-HT2 subtypes, 5-HT6, and the α2C adrenergic receptor. Dopamine receptors D2–4 and the human serotonin receptors 5-HT5A and 5-HT7A were among the top 15 hits. LSD showed pan-agonism at 5-HT1 (EC50 = 0.62–2.38 nM) and 5-HT2 receptors (EC50 = 0.30–0.66 nM). At the remaining serotonin receptors, LSD demonstrated potent agonism at 5-HT6 (EC50 = 0.13 nM), antagonism at 5-HT7A, and only partial agonism at 5-HT5A (EC50 = 2.33 nM, EMAX = 11% relative to 5-HT). LSD was an agonist at the D2, and D4 receptors (EC50 = 2.17 and 4.03 nM, respectively) and a partial agonist at D3 (EC50 = 7.57 nM, EMAX = 75% relative to dopamine). Finally, LSD showed partial agonism at α2C (EC50 = 0.56 nM, EMAX = 80% relative to norepinephrine). Ergotamine is a natural ergot alkaloid and a precursor of LSD with a similar pharmacological profile to LSD but is not generally considered as psychoactive. Ergotamine differs from LSD by its cyclic peptide moiety appended to the amide sidechain. Peng et al. profiled ergotamine’s pharmacology and discovered that ergotamine has a good affinity (Ki < 10 μM) for ~70% of all human aminergic GPCRs (32). Furthermore, 15 of these receptors show low or sub-nanomolar affinity based on a radioligand competition binding assay. While ergotamine displayed agonism at 5-HT2A–C and most 5-HT1 receptors, it did not display agonist activity at the D3, α1B, α1D, or β2 receptors despite high affinities, suggesting it acts as an antagonist at these receptors. Finally, ergotamine was an inverse agonist at 5-HT7, and further profiling showed that it also has opioid receptor agonist activity.
Most targets of LSD and/or ergotamine have been implicated in numerous diseases and disorders. Many 5-HT1 receptors show promise for the treatment of anxiety, depression, PTSD, and migraines (33–38). 5-HT2A activation promotes neuronal growth and dendritic arbor complexity, and these outcomes are linked to improvements in mood and addiction disorders (39–41). The 5-HT2C receptor contributes to mood regulation and is a common target for treating obsessive compulsive disorder (OCD) and the mode of action of antipsychotics (42–46). This mechanism has also been implicated in treating addiction and obesity (47). Targeting 5-HT6 has been shown to improve learning and memory and may also treat cognitive impairment (48–50). Additionally, 5-HT7 receptors regulate inflammation across the body, and agonists are associated with anti-inflammatory and neuroprotective effects (51–53). Finally, dopamine agonists are commonly used for treating involuntary movement disorders (54–56). However, activation of 5-HT2A is also believed to be a primary mechanism in which psychedelics produce their psychoactive effects (57–63). In contrast, activation of 5-HT2B is associated with cardiac valvulopathy, and thus targeting this receptor is generally undesirable (64, 65). Therefore, many studies aim to discover psychedelic analogs that are selective for other receptors over 5-HT2A/2B or demonstrate functional selectivity, in which a ligand stabilizes distinct receptor conformations that can selectively activate or deactivate downstream signaling pathways while keeping others unchanged (66, 67).
Biochemical, structural biology, bioengineering, and docking studies have all aided in the pursuit of non-hallucinogenic psychedelic analogs by pinpointing key structural features of compounds that elicit biased agonism. In 2013, Wacker et al. demonstrated that LSD, ergotamine, and other ergolines have functional selectivity for β-arrestin signaling at the 5-HT2B while also being unbiased for the 5-HT1B receptor (68). A comparison of crystal structures of LSD and ergotamine in both receptors revealed that the peptide appended to ergotamine was the cause of ergotamine’s superior biased signaling compared to LSD. An additional analysis of LSD complexed with 5-HT2B by Wacker et al. in 2017, in combination with molecular dynamics simulations, revealed a structural basis for LSD’s slow binding kinetics at 5-HT2A and 5-HT2B (69). This work also revealed that the amide substituents in LSD play a significant role in the position of the ligand within the orthosteric pocket, the molecule’s long residence time, and its ability to promote β-arrestin translation. Similarly, solved crystal structures of ergotamine and ritanserin bound to 5-HT2C by Peng et al. revealed distinctive binding modes for each compound and key receptor–ligand interactions that promote serotonin receptor-subtype selectivity (32). An analysis of serotonin, LSD, psilocin, and lisuride complexed with 5-HT2A by Cao et al. displayed a secondary binding pose for psilocin and serotonin in which their indole cores interact with the extended binding pocket of the receptor (70). Their data suggest that serotonin/psilocin may adopt two different positions, either in the orthosteric binding pocket or the extended binding pocket, and these would produce different biological effects. This group campaigned to synthesize a molecule that would favor the extended binding pocket, with the hypothesis that this would promote β-arrestin recruitment bias at 5-HT2A. They successfully synthesized compounds IHCH-7079 and IHCH-7086 which displayed antidepressant effects without inducing hallucinogenic behavior in mouse models. Docking studies of tetrahydropyridines complexed with 5-HT2A performed by Kaplan et al. led to the synthesis and further testing of 17 molecules (71). Among these, two displayed antagonist activity at 5-HT2B, one displayed antagonist activity at 5-HT2A, and another displayed agonist activity at 5-HT2B. Additional structure-based optimization led to the discovery of a selective 5-HT2A antagonist and two 5-HT2A partial agonists. The two agonists elicited antidepressant behavior in mouse models and low levels of head-twitch response when compared to LSD. Notably, these agonists were also quite selective and showed a 4.6–6.4-fold preference of 5-HT2A over 5-HT2B and a 29–51-fold preference over 5-HT2C. Later, Dong et al. described a fluorescent biosensor which was capable of predicting a compound’s hallucinogenic potential by detecting distinct GPCR conformations induced by hallucinogenic ligands in vivo (72). This biosensor was then used to identify a nonhallucinogenic DMT analog with neuroplasticity-promoting and antidepressant effects. Finally, 2-Br-LSD was demonstrated by Lewis et al. to be a non-hallucinogenic analog of LSD with neuroplasticity-promoting effects (31). Pharmacological profiling showed that 2-Br-LSD is a significantly more selective ligand than LSD and does not show activity at aminergic GPCRs known to affect blood pressure, heart rate, and other autonomic functions (e.g., 5-HT2B). Interestingly, while the compound was non-hallucinogenic, it still displayed agonism at 5-HT2A. However, the authors had shown that while 2-Br-LSD has high potency at 5-HT2A (EC50 = 0.81 nM), it only partially activates the receptor (EMAX = 60%, relative to serotonin); whereas LSD is nearly a full agonist (EMAX = 92%, relative to serotonin). They later tested 2-Br-LSD as an antagonist in 5-HT2A Gq dissociation and β-arrestin2 recruitment assays, and the analog did indeed act as a potent partial antagonist.
Many studies of psychedelics and their analogs focus on lysergic acid amides and dimethyltryptamine derivatives and their biological effects. Clavine alkaloids differ from lysergic acid derivatives by the structure of the quinoline ring system fused onto the indole core and the absence of a carboxylic acid functional group at C8 (Figure 2). They often have a greater conformational flexibility and are therefore more tunable receptor ligands (73, 74). However, clavine alkaloids are still comparatively understudied despite their potential for high bioactivity and superior receptor selectivity. As testimony to their medicinal effectiveness, several semisynthetic ergolines and clavines have reached the market for treating disorders and diseases such as migraines, cancer, Parkinson’s disease, and dementia (75, 76). For example, lisuride is structurally similar to LSD, yet it does not typically produce hallucinogenic effects and has often been used to treat Parkinson’s disease and migraines (77). It also produces antidepressant behavior in mice (78). Lisuride displays nanomolar binding affinity for most aminergic GPCRs including serotoninergic, adrenergic, and especially dopaminergic receptors. Its ability to treat Parkinson’s disease is believed to primarily stem from its agonist activity at the dopamine receptors (79). While lisuride has potent agonist activity at 5-HT1A (80), it is a strong antagonist at 5-HT2B and thus devoid of cardiac valvulopathy side effects (81). At 5-HT2A, lisuride is a G-protein biased agonist and this may be the mechanism responsible for how the medicinal properties of lisuride and potential hallucinogenic side effects are decoupled (78). However, strong activation of 5-HT1A has been shown to block the head-twitch response in mice treated with a 5-HT2A/5-HT2C agonist (82). Dopamine and adrenoreceptors may also play a significant role in modulating head twitch response. Bromocriptine, a 2-brominated derivative of ergocriptine is a medicinally relevant ergot alkaloid that has been used in combination with levodopa in the treatment of Parkinson’s disease (83). Bromocriptine has also been implicated as a potential therapeutic agent for type 2 diabetes (84). Similar to lisuride, it is a potent D2 agonist and has good binding affinity for a number of serotonin receptors. However, bromocriptine is an antagonist at D1, an inverse agonist at D4, and a partial agonist at 5-HT2B (80). It also behaves as an agonist at 5-HT2A and 5-HT2C receptors and, interestingly, acts as an antagonist at the adrenoreceptors α2A, α2B, and α2C. Nicergoline has been used for the treatment of dementia as well as cerebrovascular, peripheral vascular, and balance disorders (85, 86). The compound has high binding affinity for α1 and 5-HT1A receptors and demonstrated antagonism at these receptors (87). While nicergoline only has appreciable affinity for α2 and 5-HT2 receptors, it has low affinity for the dopamine receptors D1 and D2 and the muscarinic receptors M1 and M2. As a final example, ergotamine (vida supra) has been used for the prevention and treatment of migraines (88). It should be noted that the toxicity of these compounds and of all members of the ergot alkaloid class is a potential concern (89–92). Ergotism and ischemia can occur if the levels of ergot alkaloids in the body exceed a certain threshold. This typically occurs if a patient is co-treated with a cytochrome P450 inhibitor and the ergot alkaloid medication is not properly metabolized; therefore, prescribed dosages of ergot alkaloids are typically low (93). Potential toxicity should not discourage the development of these compounds into marketable drugs, but rather demonstrates the need for additional clinical and preclinical studies and investigations of natural and synthetic alkaloids before their full therapeutic potential can be unlocked.
2 Notable advancements
Most ergot alkaloid drugs are produced semi-synthetically from field cultivations or fermentations of ergot fungi (94). The clinically useful natural product precursors for derivatization are elymoclavine, lysergol, and lysergic acid, which can be isolated directly from the fungus or received from global saponification or reduction of the bulk mass of ergopeptines and lysergic acid amides. Recent studies to reconstitute the biosynthetic pathways of ergot alkaloids provide scalable access to single molecules. The biosynthesis of cycloclavine, a complex rearranged clavine alkaloid containing a cyclopropyl ring has been elucidated, and production of cycloclavine through heterologous expression in yeast, delivered titers of >500 mg L−1 (95). Microbial cell factories and cell-free systems, two unrelated systems for the biosynthesis of biomolecules, were combined to overproduce agroclavine in titers of 1,209 mg L−1 (96). The clavine oxidase (CloA) is a putative cytochrome P450 that catalyzes formation of lysergic acid from agroclavine in the ergot alkaloid biosynthetic pathway. A specific region between the F-G helices of CloA was identified as responsible for detecting and directing oxidation of agroclavine. An engineered, chimeric AT5 9Hypo CloA, which contained a clear substrate access channel and an enzyme surface cleft, increased levels of lysergic acid by 15-fold compared to the wildtype enzyme. Further discussion on scalable biosynthetic processes is beyond the scope of this review but remains an active field that holds potential to facilitate clavine and ergot alkaloid diversification. The biological implications of clavine alkaloids, closely related to LSD, have typically been evaluated through derivatizing natural products or investigations of readily derived stereoisomers.
2.1 Natural and substituted clavine alkaloids
Preliminary investigations into the SAR of the clavine alkaloids by Hofmann and Pertz established primary modalities pertinent for activity at CNS receptors. Notably, therapeutic investigations of clavine alkaloid derivatives were pioneered by Hofmann after his historic discovery of LSD (97). (+)-1-Methyl lysergol showed a 13-fold increase in serotonin inhibitory effect compared to naturally occurring (+)-lysergol, but 1-methyl-ergotamine was less potent than ergotamine, indicating this slight modification was mainly relevant to clavine alkaloids. Moreover, (+)-lysergol produces a pyretogenic effect, but the methylated analog initiates an inverse reaction and lowers the body temperature in rabbits. Finally, (+)-1-methyl lysergol (LD50 = 18 mg/kg) was over 50-fold less toxic than (+)-lysergol (LD50 = 0.32 mg/kg).
Furthermore, Pertz et al. investigated eight naturally occurring clavine alkaloids for antagonism/partial agonism at 5-HT2A receptors and antagonism at α1-adrenoreceptors in blood vessels of rat tail arteries and aorta (73). While CNS receptor structure differs between species, they demonstrated that clavine alkaloids can have different modes of action compared to 5-HT. This concept was later supported by cryo-EM structures of LSD-bound 5-HT2A; the binding modes of the semi-synthetic ergot alkaloid differed from the agonist 25CN-NBOH (98). Clavines, with the exception of costaclavine containing the deactivating cis-CD ring junction, show moderate affinity to 5-HT2A receptors and α1-adrenoreceptors. They display partial agonism activity by themselves, and some are antagonists of 5-HT at micromolar concentrations. Moreover, N1-isopropylation can increase affinity and specificity for 5-HT2A vs. α1, which could improve the noxious effects associated with ergot alkaloids. In fact, a similar modification was previously demonstrated by Hofmann (vide supra). In a subsequent report, Pertz et al. investigated cycloalkanecarboxylic esters derived from lysergol, dihydrolysergol-I, and elymoclavine (74). As with the naturally occurring clavines, these derivatives displayed partial agonism and antagonism effects of the contractile properties of 5-HT. Thereby, SAR investigations into acyl group variation and N1-modification demonstrated that the tetracyclic indolo[4,3-fg]quinoline system was the primary pharmacophore, and hydroxy acylation with cycloalkyl groups coupled with N1-alkylation synergistically increased 5-HT2A receptor selectivity over the α1-adrenoreceptor.
In 2017, Luo et al. disclosed an elegant asymmetric synthesis of (+)-lysergol, (+)-isolysergol, (−)-isolysergol, and (+)-13-fluorolysergol featuring a Ciamician-Dennstedt-type rearrangement to form the piperidine C ring and a Rh-carbenoid formal [3 + 2] cycloaddition to construct the ergoline scaffold (99). These analogs were subjected to agonism assays at four serotonin receptors, 5-HT1A,2A–C. CHO-K1 cells expressing each receptor were loaded with a dye and analyzed by FLIPR during addition of compounds or control agonist. All of the compounds displayed a reduced maximal activation for each receptor compared to 5-HT (see Table 1). For instance, while (+)-lysergol activated 5-HT2A with an EC50 value of 1.6 nM, it was limited to 51% of the maximum 5-HT stimulation. Similarly, (+)-lysergol activated 5-HT2C to 43% maximum of 5-HT stimulation. The unnatural substituted analog (+)-13-fluorolysergol, while selective for 5-HT1A and 5-HT2A, only showed 17% maximal activation of 5-HT2A compared to 5-HT. While less effective than the natural ligand, 5-HT, partial agonism and receptor selectivity garnered by ring substitution could lead to effective therapeutic agents without deleterious hallucinogenic effects (vide supra).
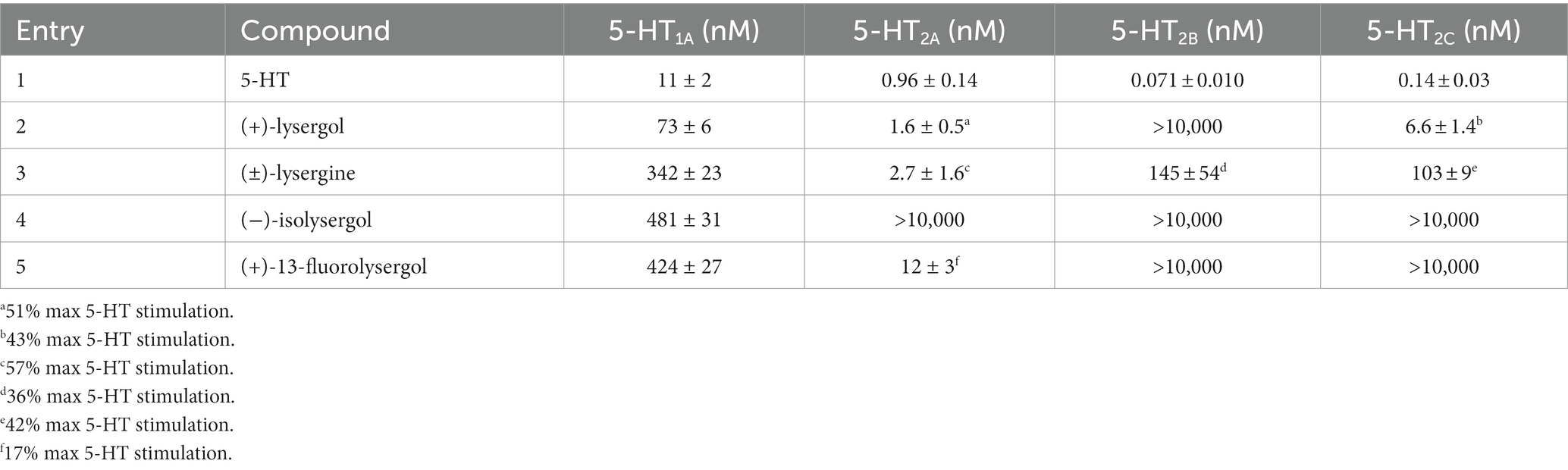
Table 1. Lysergol analog activation of 5-HT receptors reported by Luo et al. (99).
2.2 Clavine alkaloid stereoisomers
Wipf et al. reported the shortest synthesis of racemic lysergols to date, and after chiral resolution of the enantiomers, investigated the effects of all four stereoisomers on several serotonin receptors. Of note, binding data of (−)-lysergol were disclosed for the first time. All but (−)-isolysergol maintained excellent affinity for 5-HT1A,2A–C (100). Agonism effects of (+)-lysergol, (−)-lysergol, and (+)-isolysergol were calculated as a percent of control response to a known reference (Table 2). The agonism activity of (+)-lysergol corroborated previous investigations (100). With 5-fold reduced activity at 5-HT2A, the naturally occurring (+)-lysergol should have reduced psychoactive effects compared to LSD. Moreover, it displayed a similar profile to psilocin with about half of psilocin’s activity at 5-HT2A. Interestingly, (−)-lysergol was a selective agonist for 5-HT2C. Many 5-HT2C activators that are used to regulate mood are poorly selective between 5-HT2B and 5-HT2A, so further investigations and SAR would be justified to elaborate upon these findings. (+)-Isolysergol displayed very similar activation of 5-HT1A compared to (+)-lysergol, but was less active towards 5-HT2C, suggesting the configuration of C(8) plays a more significant role in agonism activity towards 5-HT2C than 5-HT1A.
Interestingly, while (+)-lysergol had a high affinity for 5-HT2B, it produced no agonism effects within the tested range (101). Similarly, (+)-isolysergol showed high affinities for 5-HT2A and 5-HT2B without agonism effects. Since these ligands bind without inducing activation, further studies are required to determine if they act as antagonists, as findings from Pertz (vide supra) (73) suggest these clavines could also produce antagonism effects at other CNS receptors. Of particular note is also the stereospecificity of the binding/agonistic effects of the four lysergol stereoisomers. While not necessarily unexpected for rigid and selective compounds, this nonetheless highlights opportunities for future ligand designs at 5-HTRs.
Recently, investigations into the activation effects of rearranged clavine alkaloids, including (+)- and (−)-cycloclavine and all stereoisomers of rugulovasine have been reported (99, 100, 102, 103). Wipf et al. developed an asymmetric synthesis of cycloclavine and investigated the specific binding and activation of both natural and unnatural enantiomers at several CNS receptors (104). The naturally occurring (+)-cycloclavine was an agonist for 5-HT1A (EC50 = 0.14 μM) and 5-HT2C (EC50 = 0.016 μM). (−)-Cycloclavine displayed comparably much smaller activation effects, and only nominally affected 5-HT2C (EC50 = 3.2 μM). (+)-Cycloclavine displayed a remarkably similar profile to (+)-lysergol, albeit with ~8-fold lower 5-HT2A activation. Since both cycloclavine enantiomers were poor activators of 5-HT2A, they may only exhibit weak hallucinogenic effects, but (+)-cycloclavine could display promising mood-regulating properties.
Piersanti et al. disclosed an asymmetric synthesis of all stereoisomers of rugulovasines and evaluated their CNS receptor activity (103). They were tested for specific binding at serotonin (5-HT1A,2A,2C), adrenergic (α2), and dopamine (D1,2L,3) receptors, and were found to be specific solely for the first. Of the four isomers, (+)-rugulovasine B was the overall best ligand and (−)-rugulovasine B displayed poor affinity for all but 5-HT1A. Moreover, among the targets that were investigated, the rugulovasines were selective for 5-HT receptors. Since all four isomers displayed a strong affinity for 5-HT1A, they were subjected to an activation assay for that target. Each isomer was a strong activator of this receptor with nanomolar potencies. (+)-Rugulovasine was the most potent agonist (<2 nM) and was surprisingly more active than LSD. This significant enantiospecificity and potency translated to other 5-HT receptors. Among the seven other serotonin receptors tested (5-HT1A,2B–C,5A,6,7), (+)-rugulovasine B displayed nanomolar activation for all but 5-HT5A (13.7 μM), in contrast to LSD which only displayed low activation of 5-HT2B (12.0 μM). (+)-Rugulovasine B is a potent activator of 5-HT2A and 5-HT2B, suggesting hallucinogenic, cardiotoxic, and europhoric effects might occur in consumers. Accordingly, further (ant)agonism assays of dopamine and adrenergic receptors of the rugulovasines are required to validate their therapeutic potential. Hopefully, the expedient synthetic approach will enable future investigations of the biologically understudied rugulovasine scaffold.
3 Conclusion
The exploration of clavine alkaloids as therapeutic agents is of continued and significant interest. Early work focused on improving the potency and decreasing the toxicity of natural clavines through minor structural modifications, such as N1-alkylation or O-acylation (73, 74, 97). More recently, efficient total syntheses of clavine alkaloids have enabled systematic investigations of stereoisomers (100, 103, 104) and analogs with significant structural modifications (99). (+)-Lysergol, (+)-isolysergol, and (+)-cycloclavine thus far bear the most potential as non-hallucinogenic “psychedelic” agents. While more CNS receptor profiling and in vivo studies are required, they maintain promising 5-HT receptor profiles, as they poorly activate receptors that cause deleterious effects [5-HT2A (hallucinations) and 5-HT2B (cardiotoxicity)], but strongly activate the mood-regulators 5-HT1A and 5-HT2C. Moreover, (+)-lysergol displays a very similar profile to the clinically studied psychedelic psilocin at the 5-HT1A,2A–C receptors, and derivatization with deep-seated changes achievable through de novo synthesis could provide access to more selective ligands, as demonstrated by Luo et al. (99). The understudied rearranged clavine scaffolds, including cycloclavine and the rugulovasines, also demonstrate unique enantiospecific activation of the CNS receptors, similar to the lysergols. Some clavines such as (+)-rugulovasine B can be potent 5-HT2A activators; however, they are relatively unselective agonists and could produce detrimental side effects. Future SAR campaigns on the clavine alkaloid scaffold are well justified by their known biological profile and are likely to discover a broad range of novel and potentially therapeutic analogs, paving the way to decouple the development of new psychedelics from the study of the known natural product isolates.
Data availability statement
The original contributions presented in the study are included in the article/supplementary material, further inquiries can be directed to the corresponding author.
Author contributions
NT: Writing – original draft, Writing – review & editing. EP: Writing – original draft, Writing – review & editing. PW: Conceptualization, Supervision, Writing – review & editing.
Funding
The author(s) declare financial support was received for the research, authorship, and/or publication of this article. The authors acknowledge funding from the University of Pittsburgh (discretionary funds to PW).
Conflict of interest
The authors declare that the research was conducted in the absence of any commercial or financial relationships that could be construed as a potential conflict of interest.
Publisher’s note
All claims expressed in this article are solely those of the authors and do not necessarily represent those of their affiliated organizations, or those of the publisher, the editors and the reviewers. Any product that may be evaluated in this article, or claim that may be made by its manufacturer, is not guaranteed or endorsed by the publisher.
References
1. Schultes, RE, Hofmann, A, and Rätsch, C. Plants of the gods: their sacred, healing, and hallucinogenic powers. Rochester: Healing Arts Press (2001).
2. Tupper, KW, Wood, E, Yensen, R, and Johnson, MW. Psychedelic medicine: a re-emerging therapeutic paradigm. CMAJ. (2015) 187:1054–9. doi: 10.1503/cmaj.141124
3. Gardner, J, Carter, A, O’Brien, K, and Seear, K. Psychedelic-assisted therapies: the past, and the need to move forward responsibly. Int J Drug Policy. (2019) 70:94–8. doi: 10.1016/j.drugpo.2019.05.019
4. Lowe, H, Toyang, N, Steele, B, Valentine, H, Grant, J, Ali, A, et al. The therapeutic potential of psilocybin. Molecules. (2021) 26:2948. doi: 10.3390/molecules26102948
5. Lutkajtis, A. Four individuals’ experience during and following a psilocybin truffle retreat in the Netherlands. J Psychedelic Stud. (2021) 5:22–32. doi: 10.1556/2054.2021.00162
6. Holze, F, Vizeli, P, Ley, L, Müller, F, Dolder, P, Stocker, M, et al. Acute dose-dependent effects of lysergic acid diethylamide in a double-blind placebo-controlled study in healthy subjects. Neuropsychopharmacology. (2021) 46:537–44. doi: 10.1038/s41386-020-00883-6
7. Vogt, SB, Ley, L, Erne, L, Straumann, I, Becker, AM, Klaiber, A, et al. Acute effects of intravenous DMT in a randomized placebo-controlled study in healthy participants. Transl Psychiatry. (2023) 13:172. doi: 10.1038/s41398-023-02477-4
8. Stoliker, D, Egan, GF, Friston, KJ, and Razi, A. Neural mechanisms and psychology of psychedelic ego dissolution. Pharmacol Rev. (2022) 74:876–917. doi: 10.1124/pharmrev.121.000508
9. Forstmann, M, Yudkin, DA, Prosser, AMB, Heller, SM, and Crockett, MJ. Transformative experience and social connectedness mediate the mood-enhancing effects of psychedelic use in naturalistic settings. Proc Natl Acad Sci U S A. (2020) 117:2338–46. doi: 10.1073/pnas.1918477117
11. Nardou, R, Sawyer, E, Song, YJ, Wilkinson, M, Padovan-Hernandez, Y, de Deus, JL, et al. Psychedelics reopen the social reward learning critical period. Nature. (2023) 618:790–8. doi: 10.1038/s41586-023-06204-3
12. Nikolaidis, A, Lancelotta, R, Gukasyan, N, Griffiths, RR, Barrett, FS, and Davis, AK. Subtypes of the psychedelic experience have reproducible and predictable effects on depression and anxiety symptoms. J Affect Disord. (2023) 324:239–49. doi: 10.1016/j.jad.2022.12.042
13. Plazas, E, and Faraone, N. Indole alkaloids from psychoactive mushrooms: chemical and pharmacological potential as psychotherapeutic agents. Biomedicine. (2023) 11:461. doi: 10.3390/biomedicines11020461
14. Inserra, A, de Gregorio, D, and Gobbi, G. Psychedelics in psychiatry: neuroplastic, immunomodulatory, and neurotransmitter mechanisms. Pharmacol Rev. (2021) 73:202–77. doi: 10.1124/pharmrev.120.000056
15. Dyck, E. LSD: a new treatment emerging from the past. CMAJ. (2015) 187:1079–80. doi: 10.1503/cmaj.141358
16. Calleja-Conde, J, Morales-García, JA, Echeverry-Alzate, BKM, Giné, E, and López-Moreno, JA. Classic psychedelics and alcohol use disorders: a systematic review of human and animal studies. Addict Biol. (2022) 27:e13229. doi: 10.1111/adb.13229
17. Cameron, LP, and Olson, DE. Dark classics in chemical neuroscience: N,N-dimethyltryptamine (DMT). ACS Chem Neurosci. (2018) 9:2344–57. doi: 10.1021/acschemneuro.8b00101
18. Barber, GS, and Aaronson, ST. The emerging field of psychedelic psychotherapy. Curr Psychiatry Rep. (2022) 24:583–90. doi: 10.1007/s11920-022-01363-y
19. Nichols, DE. Dark classics in chemical neuroscience: lysergic acid diethylamide (LSD). ACS Chem Neurosci. (2018) 9:2331–43. doi: 10.1021/acschemneuro.8b00043
20. Strassman, RJ, and Qualls, CR. Dose-response study of N,N-dimethyltryptamine in humans I. Neuroendocrine, autonomic, and aardiovascular effects. Arch Gen Psychiatry. (1994) 51:85–97. doi: 10.1001/archpsyc.1994.03950020009001
21. Strassman, RJ, Qualls, CR, Uhlenhuth, EH, and Kellner, R. Dose-response study of N,N-dimethyltryptamine in humans. II. Subjective effects and preliminary results of a new rating scale. Arch Gen Psychiatry. (1994) 51:98–108. doi: 10.1001/archpsyc.1994.03950020022002
22. Carhart-Harris, RL, and Goodwin, GM. The therapeutic potential of psychedelic drugs: past, present, and future. Neuropsychopharmacology. (2017) 42:2105–13. doi: 10.1038/npp.2017.84
23. Hadar, A, David, J, Shalit, N, Roseman, L, Gross, R, Sessa, B, et al. The psychedelic renaissance in clinical research: a bibliometric analysis of three decades of human studies with psychedelics. J Psychoactive Drugs. (2023) 55:1–10. doi: 10.1080/02791072.2021.2022254
24. Nichols, DE, Frescas, S, Marona-Lewicka, D, and Kurrasch-Orbaugh, DM. Lysergamides of isomeric 2,4-Dimethylazetidines map the binding orientation of the diethylamide moiety in the potent hallucinogenic AgentN,N-Diethyllysergamide (LSD). J Med Chem. (2002) 45:4344. doi: 10.1021/jm020153s
25. Passie, T, Halpern, JH, Stichtenoth, DO, Emrich, HM, and Hintzen, A. The pharmacology of lysergic acid diethylamide: a review. CNS Neurosci Ther. (2008) 14:295–314. doi: 10.1111/j.1755-5949.2008.00059.x
26. Egan, CT, Herrick-Davis, K, Miller, K, Glennon, RA, and Teitler, M. Agonist activity of LSD and lisuride at cloned 5HT2A and 5HT2C receptors. Psychopharmacology. (1998) 136:409–14. doi: 10.1007/s002130050585
27. Watts, VJ, Lawler, CP, Fox, DR, Neve, KA, Nichols, DE, and Mailman, RB. LSD and structural analogs: pharmacological evaluation at D1 dopamine receptors. Psychopharmacology. (1995) 118:401–9. doi: 10.1007/BF02245940
28. Giacomelli, S, Palmerry, M, Romanelli, L, Cheng, CY, and Silvestrini, B. Lysergic acid diethylamide (LSD) is a partial agonist of D2 dopaminergic receptors and it potentiates dopamine-mediated prolactin secretion in lactotrophs in vitro. Life Sci. (1998) 63:215–22. doi: 10.1016/s0024-3205(98)00262-8
29. Rickli, A, Luethi, RJ, Buchy, D, Hoener, MC, and Liechti, ME. Receptor interaction profiles of novel N-2-methoxybenzyl (NBOMe) derivatives of 2,5-dimethoxy-substituted phenethylamines (2C drugs). Neuropharmacology. (2015) 99:546. doi: 10.1016/j.neuropharm.2015.08.034
30. Rickli, A, Moning, OD, Hoener, MC, and Liechti, ME. Receptor interaction profiles of novel psychoactive tryptamines compared with classic hallucinogens. Eur Neuropsychopharmacol. (2016) 26:1327–37. doi: 10.1016/j.euroneuro.2016.05.001
31. Lewis, V, Bonniwell, EM, Lanham, JK, Ghaffari, A, Sheshbaradaran, H, Cao, AB, et al. A non-hallucinogenic LSD analog with therapeutic potential for mood disorders. Cell Rep. (2023) 42:112203. doi: 10.1016/j.celrep.2023.112203
32. Peng, Y, McCorvy, JD, Harpsøe, K, Lansu, K, Yuan, S, Popov, P, et al. 5-HT2C receptor structures reveal the structural basis of GPCR polypharmacology. Cells. (2018) 172:719–730.e14. doi: 10.1016/j.cell.2018.01.001
33. Ramírez Rosas, MB, Labruijere, S, Villalón, CM, and Maassen, VDBA. Activation of 5-hydroxytryptamine 1B/1D/1F receptors as a mechanism of action of antimigraine drugs. Expert Opin Pharmacother. (2013) 14:1599–10. doi: 10.1517/14656566.2013.806487
34. Xiang, M, Jiang, Y, Hu, Z, Yang, Y, Du, X, Botchway, BOA, et al. Serotonin receptors 2A and 1A modulate anxiety-like behavior in post-traumatic stress disordered mice. Am J Transl Res. (2019) 11:2288–303.
35. Garcia-Garcia, A, Newman-Tancredi, A, and Leonardo, ED. 5-HT1A receptors in mood and anxiety: recent insights into autoreceptor versus heteroreceptor function. Psychopharmacology. (2014) 231:623–36. doi: 10.1007/s00213-013-3389-x
36. Cameron, LP, Benson, CJ, Dunlap, LE, and Olson, DE. Effects of N, N-dimethyltryptamine on rat Behaviors relevant to anxiety and depression. ACS Chem Neurosci. (2018) 9:1582–90. doi: 10.1021/acschemneuro.8b00134
37. Tiger, M, Varnäs, K, Okubo, Y, and Lundberg, J. The 5-HT1B receptor – a potential target for antidepressant treatment. Psychopharmacology. (2018) 235:1317–34. doi: 10.1007/s00213-018-4872-1
38. Ruf, BM, and Bhagwager, Z. The 5-HT1B receptor: a novel target for the pathophysiology of depression. Curr Drug Targets. (2009) 10:1118–38. doi: 10.2174/138945009789735192
39. Ly, C, Greb, AC, Cameron, LP, Wong, JM, Barragan, EV, Wilson, PC, et al. Psychedelics promote structural and functional neural plasticity. Cell Rep. (2018) 23:3170–82. doi: 10.1016/j.celrep.2018.05.022
40. Vargas, MV, Dunlap, LE, Dong, C, Carter, SJ, Tombari, RJ, Jami, SA, et al. Psychedelics promote neuroplasticity through the activation of intracellular 5-HT2A receptors. Science. (2023) 379:700–6. doi: 10.1126/science.adf0435
41. Cameron, LP, Tombari, RJ, Lu, J, Pell, AJ, Hurley, ZQ, Ehinger, Y, et al. A non-hallucinogenic psychedelic analogue with therapeutic potential. Nature. (2021) 589:474–9. doi: 10.1038/S41586-020-3008-z
42. Papakosta, VM, Kalogerakou, S, Kontis, D, Anyfandi, E, Theochari, E, Boulougouris, V, et al. 5-HT2C receptor involvement in the control of persistence in the reinforced spatial alternation animal model of obsessive-compulsive disorder. Behav Brain Res. (2013) 243:176–83. doi: 10.1016/j.bbr.2013.01.005
43. Flaisher-Grinberg, S, Klavir, O, and Joel, D. The role of 5-HT2A and 5-HT2C receptors in the signal attenuation rat model of obsessive compulsive disorder. Int J Neuropsychopharmacol. (2008) 11:811–25. doi: 10.1017/s146114570800847x
44. Sard, H, Kumaran, G, Morency, C, Roth, BL, Toth, BA, He, P, et al. SAR of psilocybin analogs: discovery of a selective 5-HT2C agonist. Bioorg Med Chem Lett. (2005) 15:4555–9. doi: 10.1016/j.bmcl.2005.06.104
45. Chagraoui, A, Thibaut, F, Skiba, M, Thuillez, C, and Bourin, M. 5-HT2C receptors in psychiatric disorders: a review. Prog Neuro-Psychopharmacol Biol Psychiatry. (2016) 66:120–35. doi: 10.1016/j.pnpbp.2015.12.006
46. Pogorelov, VM, Rodriguiz, RM, Cheng, J, Huang, M, Schmerberg, CM, Meltzer, HY, et al. 5-HT2C agonists modulate schizophrenia-like behaviors in mice. Neuropsychopharmacology. (2017) 42:2163–77. doi: 10.1038/npp.2017.52
47. Higgins, GA, Sellers, EM, and Fletcher, PJ. From obesity to substance abuse: therapeutic opportunities for 5-HT2C receptor agonists. Trends Pharmacol Sci. (2013) 34:560–70. doi: 10.1016/j.tips.2013.08.001
48. Drop, M, Canale, V, Chaumont-Dubel, S, Kurczab, R, Satała, G, Bantreil, X, et al. 2-phenyl-1H-pyrrole-3-carboxamide as a new scaffold for developing 5-HT6 receptor inverse agonists with cognition-enhancing activity. ACS Chem Neurosci. (2021) 12:1228–40. doi: 10.1021/acschemneuro.1c00061
49. Woods, S, Clarke, NN, Layfield, R, and Fone, KCF. 5-HT(6) receptor agonists and antagonists enhance learning and memory in a conditioned emotion response paradigm by modulation of cholinergic and glutamatergic mechanisms. Br J Pharmacol. (2012) 167:436–49. doi: 10.1111/j.1476-5381.2012.02022.x
50. Kendall, I, Slotten, HA, Codony, X, Burgueño, J, Pauwels, PJ, Vela, JM, et al. E-6801, a 5-HT6 receptor agonist, improves recognition memory by combined modulation of cholinergic and glutamatergic neurotransmission in the rat. Psychopharmacology. (2011) 213:413–30. doi: 10.1007/s00213-010-1854-3
51. Di Pilato, P, Niso, M, Adriani, W, Romano, E, Travaglini, D, Berardi, F, et al. Selective agonists for serotonin 7 (5-HT7) receptor and their applications in preclinical models: an overview. Rev Neurosci. (2014) 25:401–15. doi: 10.1515/revneuro-2014-0009
52. Quintero-Villegas, A, and Valdés-Ferrer, SI. Central nervous system effects of 5-HT7 receptors: a potential target for neurodegenerative diseases. Mol Medicine. (2022) 28:70. doi: 10.1186/s10020-022-00497-2
53. Quintero-Villegas, A, and Valdés-Ferrer, SI. Role of 5-HT7 receptors in the immune system in health and disease. Mol Medicine. (2020) 26:2. doi: 10.1186/s10020-019-0126-x
54. Brooks, DJ. Dopamine agonists: their role in the treatment of Parkinson’s disease. J Neurol Neurosurg Psychiatry. (2000) 68:685. doi: 10.1136/jnnp.68.6.685
55. Poewe, W, Seppi, K, Tanner, CM, Halliday, GM, Brundin, P, Volkmann, J, et al. Parkinson disease. Nat Rev Dis Primers. (2017) 3:17013. doi: 10.1038/nrdp.2017.13
56. Scholz, H, Trenkwalder, C, Kohnen, R, Kriston, L, Riemann, D, and Hornyak, M. Dopamine agonists for the treatment of restless legs syndrome. Cochrane Database Syst Rev. (2011) 2011:CD006009. doi: 10.1002/14651858.cd006009.pub2
57. López-Giménez, JF, and González-Maeso, J. Hallucinogens and serotonin 5-HT2A receptor-mediated signaling pathways. Curr Top Behav Neurosci. (2018) 36:45–73. doi: 10.1007/7854_2017_478
58. Hesselgrave, N, Troppoli, TA, Wulff, AB, Cole, AB, and Thompson, SM. Harnessing psilocybin: antidepressant-like behavioral and synaptic actions of psilocybin are independent of 5-HT2R activation in mice. Proc Natl Acad Sci U S A. (2021) 118:e2022489118. doi: 10.1073/pnas.2022489118
59. Jaster, AM, Elder, H, Marsh, SA, de La Fuente, RM, Negus, SS, and González-Maeso, J. Effects of the 5-HT2A receptor antagonist volinanserin on head-twitch response and intracranial self-stimulation depression induced by different structural classes of psychedelics in rodents. Psychopharmacology. (2022) 239:1665–77. doi: 10.1007/s00213-022-06092-x
60. Glatfelter, GC, Pottie, E, Partilla, JS, Sherwood, AM, Kaylo, K, Pham, DNK, et al. Structure–activity relationships for psilocybin, baeocystin, aeruginascin, and related analogues to produce pharmacological effects in mice. ACS Pharmacol Transl Sci. (2022) 5:1181–96. doi: 10.1021/acsptsci.2c00177
61. González-Maeso, J, Weisstaub, NV, Zhou, M, Chan, P, Ivic, L, Ang, R, et al. Hallucinogens recruit specific cortical 5-HT2A receptor-mediated signaling pathways to affect behavior. Neuron. (2007) 53:439–52. doi: 10.1016/j.neuron.2007.01.008
62. Canal, CE, and Morgan, D. Head-twitch response in rodents induced by the hallucinogen 2,5-dimethoxy-4-iodoamphetamine: a comprehensive history, a re-evaluation of mechanisms, and its utility as a model. Drug Test Anal. (2012) 4:556–76. doi: 10.1002/dta.1333
63. Willins, DL, and Meltzer, HY. Direct injection of 5-HT2A receptor agonists into the medial prefrontal cortex produces a head-twitch response in rats. J Pharmcol Exp Ther. (1997) 282:699–706.
64. Rothman, RB, and Baumann, MH. Serotonergic drugs and valvular heart disease. Expert Opin Drug Saf. (2009) 8:317–29. doi: 10.1517/14740330902931524
65. Hutcheson, JD, Setola, V, Roth, BL, and Merryman, WD. Serotonin receptors and heart valve disease – it was meant 2B. Pharmacol Ther. (2011) 132:146–57. doi: 10.1016/j.pharmthera.2011.03.008
66. Urban, JD, Clarke, WP, von Zastrow, M, Nichols, DE, Kobilka, B, Weinstein, H, et al. Functional selectivity and classical concepts of quantitative pharmacology. J Pharmacol Exp Ther. (2007) 320:1–13. doi: 10.1124/jpet.106.104463
67. Wootten, D, Chrisopoulos, A, Marti-Solano, M, Babu, MM, and Sexton, PM. Mechanisms of signaling and biased agonism in G protein-coupled receptors. Nat Rev Mol Cell Biol. (2018) 19:638–53. doi: 10.1038/s41580-018-0049-3
68. Wacker, D, Wang, C, Katritch, V, Won Han, G, Huang, X-P, Vardy, E, et al. Structural features for functional selectivity at serotonin receptors. Science. (2013) 340:615–9. doi: 10.1126/science.1232808
69. Wacker, D, Wang, S, McCorvy, JD, Betz, RM, Venkatakrishnan, AJ, Levit, A, et al. Crystal structure of an LSD-bound human serotonin receptor. Cells. (2017) 168:377–389.e12. doi: 10.1016/j.cell.2016.12.033
70. Cao, D, Yu, J, Wang, H, Luo, Z, Liu, X, He, L, et al. Structure-based discovery of nonhallucinogenic psychedelic analogs. Science. (2022) 375:403–11. doi: 10.1126/science.abl8615
71. Kaplan, AL, Confer, DN, Kim, K, Barros-Álvarez, X, Rodriguiz, RM, Yang, Y, et al. Bespoke library docking for 5-HT2A receptor agonists with antidepressant activity. Nature. (2022) 610:582–91. doi: 10.1038/s41586-022-05258-z
72. Dong, C, Ly, C, Dunlap, LE, Vargas, MV, Sun, J, Hwang, IW, et al. Psychedelic-inspired drug discovery using an engineered biosensor. Cells. (2021) 184:2779–2792.e18. doi: 10.1016/j.cell.2021.03.043
73. Pertz, H. Naturally occurring clavines: antagonism/partial agonism at 5-HT2A receptors and antagonism at α1-adrenoceptors in blood vessels. Planta Med. (1996) 62:387–92. doi: 10.1055/s-2006-957922
74. Pertz, HH, Milhahn, C, and Eich, E. Cycloalkanecarboxylic esters derived from lysergol, dihydrolysergol-I, and elymoclavine as partial agonists and antagonists at rat 5-HT2A receptors: pharmacological evidence that the indolo[4,3-fg]quinoline system of the ergolines is responsible for high 5-HT2A receptor affinity. J Med Chem. (1999) 42:659–68. doi: 10.1021/jm981092u
75. McCabe, SR, and Wipf, P. Total synthesis, biosynthesis and biological profiles of clavine alkaloids. Org Biomol Chem. (2016) 14:5894–913. doi: 10.1039/c6ob00878j
76. Tasker, NR, and Wipf, P. Biosynthesis, total synthesis, and biological profiles of ergot alkaloids. Alkaloids Chem Biol. (2021) 85:1–112. doi: 10.1016/bs.alkal.2020.08.001
77. Horowski, R, and Löschmann, PA. Classic dopamine agonists. J Neural Transm. (2019) 126:449–54. doi: 10.1007/s00702-019-01989-y
78. Pogorelov, VM, Rodriguiz, RM, Roth, B, and Westel, WC. The G protein biased serotonin 5-HT2A receptor agonist lisuride exerts anti-depressant drug-like activities in mice. Front Mol Biosci. (2023) 10:10. doi: 10.3389/fmolb.2023.1233743
79. McDonald, RJ, and Horowski, R. Lisuride in the treatment of parkinsonism. Eur Neurol. (1983) 22:240–55. doi: 10.1159/000115567
80. Kvernmo, T, Houben, J, and Sylte, I. Receptor-binding and pharmacokinetic properties of dopaminergic agonists. Curr Top Med Chem. (2008) 8:1049–67. doi: 10.2174/156802608785161457
81. Hofman, C, Penner, U, Dorow, R, Pertz, HH, Jähnichen, S, Horowski, R, et al. Lisuride, a dopamine receptor agonist with 5-HT2B receptor antagonist properties: absence of cardiac valvulopathy adverse drug reaction reports supports the concept of a crucial role for 5-HT2B receptor agonism in cardiac valvular fibrosis. Clin Neuropharmacol. (2006) 29:80–6. doi: 10.1097/00002826-200603000-00005
82. Schreiber, R, Brocco, M, Audinot, V, Gobert, A, Veiga, S, and Millan, MJ. (1-(2,5-dimethoxy-4 iodophenyl)-2-aminopropane)-induced head-twitches in the rat are mediated by 5-hydroxytryptamine (5-HT) 2A receptors: modulation by novel 5-HT2A/2C antagonists, D1 antagonists and 5-HT1A agonists. J Pharmacol Exp Ther. (1995) 273:101–12.
83. Zhuo, C, Zhu, X, Jiang, R, Ji, F, Su, Z, Sue, R, et al. Comparison for efficacy and tolerability among ten drugs for treatment of Parkinson’s disease: a network meta-analysis. Sci Rep. (2017) 7:45865. doi: 10.1038/srep45865
84. Cincotta, AH. Brain dopamine–clock interactions regulate cardiometabolic physiology: mechanisms of the observed cardioprotective effects of circadian-timed bromocriptine-QR therapy in type 2 diabetes subjects. Int J Mol Sci. (2023) 24:13255. doi: 10.3390/ijms241713255
85. Winblad, B, Carfagna, N, Bonura, L, Rossini, BM, Wong, EHF, and Battaglia, A. Nicergoline in dementia: a review of its pharmacological properties and therapeutic potential. CNS Drugs. (2000) 14:267–87. doi: 10.2165/00023210-200014040-00003
86. Winblad, B, Fioravanti, M, Dolezal, T, Logina, I, Milanov, IG, Popescu, DC, et al. Therapeutic use of nicergoline. Clin Drug Investig. (2008) 28:533–52. doi: 10.2165/00044011-200828090-00001
87. Zajdel, P, Bednarski, M, Sapa, J, and Nowak, G. Ergotamine and nicergoline – facts and myths. Pharmacol Rep. (2015) 67:360–3. doi: 10.1016/j.pharep.2014.10.010
88. Tfelt-Hansen, P, Saxena, PR, Dahlöf, C, Pascual, J, Láinez, M, Henry, P, et al. Ergotamine in the acute treatment of migraine: a review and European consensus. Brain. (2000) 123:9–18. doi: 10.1093/brain/123.1.9
89. Mulac, D, and Humpf, HU. Cytotoxicity and accumulation of ergot alkaloids in human primary cells. Toxicology. (2011) 282:112–21. doi: 10.1016/j.tox.2011.01.019
90. Agriopoulou, S. Ergot alkaloids mycotoxins in cereals and cereal-derived food products: characteristics, toxicity, prevalence, and control strategies. Agronomy. (2021) 11:931. doi: 10.3390/agronomy11050931
91. Korn, AK, Gross, M, Usleber, E, Thom, N, Köhler, K, and Erhardt, G. Dietary ergot alkaloids as a possible cause of tail necrosis in rabbits. Mycotoxin Res. (2014) 30:241–50. doi: 10.1007/s12550-014-0208-0
92. Finch, SC, Munday, JS, Sprosen, JM, and Bhattarai, S. Toxicity studies of Chanoclavine in mice. Toxins. (2019) 11:249. doi: 10.3390/toxins11050249
93. Fröhlich, G, Kaplan, V, and Amann-Vesti, B. Holy fire in an hiv-positive man: case of 21st-century ergotism. CMAJ. (2010) 182:378–80. doi: 10.1503/cmaj.091362
94. Jakubczyk, D, Caputi, L, Hatsch, A, Nielsen, CAF, Diefenbacher, M, Klein, J, et al. Discovery and reconstitution of the cycloclavine biosynthetic pathway – enzymatic formation of a cyclopropyl group. Angew Chem Int Ed Engl. (2015) 54:5117–21. doi: 10.1002/anie.201410002
95. Yu, ZP, An, C, Yao, Y, Wang, CY, Sun, Z, Cui, C, et al. A combined strategy for the overproduction of complex ergot alkaloid agroclavine. Synth Syst Biotechnol. (2022) 7:1126–32. doi: 10.1016/j.synbio.2022.08.003
96. Lim, LR, Wong, G, Go, MK, and Yew, WS. Increasing lysergic acid levels for ergot alkaloid biosynthesis: directing catalysis via the F-G loop of clavine oxidases. Front Microbiol. (2023) 14:1–10. doi: 10.3389/fmicb.2023.1150937
97. Hofmann, A., and Troxler, F. 1-substituted (+)-lysergol. U.S. patent no 3,232,942 (1966). United States Patent Office
98. Kim, K, Che, T, Panova, O, DiBerto, JF, Lyu, J, Krumm, BE, et al. Structure of a hallucinogen-activated Gq-coupled 5-HT2A serotonin receptor. Cells. (2020) 182:1574–1588.e19. doi: 10.1016/j.cell.2020.08.024
99. Yuan, H, Guo, Z, and Luo, T. Synthesis of (+)-lysergol and its analogues to assess serotonin receptor activity. Org Lett. (2017) 19:624–7. doi: 10.1021/acs.orglett.6b03779
100. Tasker, NR, and Wipf, P. A short synthesis of ergot alkaloids and evaluation of the 5-HT1/2 receptor selectivity of lysergols and isolysergols. Org Lett. (2022) 24:7255–9. doi: 10.1021/acs.orglett.2c02569
101. Tasker, NR. Photoflow preparation of PTP4A3 inhibitors, and concise total syntheses of ergot and clavine alkaloids. PhD Dissertation (2023). Pittsburgh (PA): University of Pittsburgh
102. McCabe, SR, and Wipf, P. Eight-step enantioselective total synthesis of (−)-cycloclavine. Angew Chem Int Ed. (2017) 56:324–7. doi: 10.1002/anie.201608820
103. Bartoccini, F, Regni, A, Retini, M, and Piersanti, G. Asymmetric total synthesis of all rugulovasine stereoisomers and preliminary evaluation of their biological properties. Eur. J Org Chem. (2022) 2022:e202200315. doi: 10.1002/ejoc.202200315
Keywords: psychedelic therapies, neuropsychiatric diseases, anxiety, depression, lysergol, cycloclavine, agroclavine, ergot alkaloids
Citation: Tasker NR, Pazur EJ and Wipf P (2023) Biological studies of clavine alkaloids targeting CNS receptors. Front. Psychiatry. 14:1286941. doi: 10.3389/fpsyt.2023.1286941
Edited by:
Martin L. Williams, Swinburne University of Technology, AustraliaReviewed by:
John McCorvy, Medical College of Wisconsin, United StatesCopyright © 2023 Tasker, Pazur and Wipf. This is an open-access article distributed under the terms of the Creative Commons Attribution License (CC BY). The use, distribution or reproduction in other forums is permitted, provided the original author(s) and the copyright owner(s) are credited and that the original publication in this journal is cited, in accordance with accepted academic practice. No use, distribution or reproduction is permitted which does not comply with these terms.
*Correspondence: Peter Wipf, cHdpcGZAcGl0dC5lZHU=