- 1Institute of Neuroscience and Medicine (INM-2), Forschungszentrum Jülich GmbH, Jülich, Germany
- 2Department of Psychiatry, Psychotherapy and Psychosomatics, Medical Faculty, RWTH Aachen, Aachen, Germany
- 3Multimodal Neuroimaging Group, Department of Nuclear Medicine, University Hospital Cologne, Cologne, Germany
- 4University Hospital, Heinrich Heine University Düsseldorf, Düsseldorf, Germany
Introduction: Sleep deprivation and electroconvulsive therapy (ECT) effectively ameliorate symptoms in major depressive disorder (MDD). In rodents, both are associated with an enhancement of cerebral adenosine levels, which in turn likely influence adenosinergic receptor expression. The aim of the current study was to investigate cerebral A1 adenosine receptor (A1AR) availability in patients with MDD as a potential mediating factor of antidepressant effects of ECT using [18F]CPFPX and positron emission tomography (PET).
Methods: Regional A1AR availability was determined before and after a series of ECT applications (mean number ± SD 10.4 ± 1.2) in 14 subjects (4 males, mean age 49.5 ± 11.8 years). Clinical outcome, measured by neuropsychological testing, and ECT parameters were correlated with changes in A1AR availability.
Results: ECT had a strong antidepressive effect (p < 0.01) while on average cerebral A1AR availability remained unaltered between pre-and post-ECT conditions (F = 0.65, p = 0.42, mean difference ± SD 3.93% ± 22.7%). There was no correlation between changes in clinical outcome parameters and regional A1AR availability, although individual patients showed striking bidirectional alterations of up to 30–40% in A1AR availability after ECT. Solely, for the mean seizure quality index of the applied ECTs a significant association with changes in A1AR availability was found (rs = −0.6, p = 0.02).
Discussion: In the present study, therapeutically effective ECT treatment did not result in coherent changes of A1AR availability after a series of ECT treatments. These findings do not exclude a potential role for cerebral A1ARs in ECT, but shift attention to rather short-termed and adaptive mechanisms during ECT-related convulsive effects.
Introduction
Major depressive disorder (MDD) severely influences life quality and is one of the major causes of suicides. Currently, over 320 million people are affected by MDD worldwide (1). The neurobiological understanding of mood disorders has shifted its focus some 20 years ago from monoaminergic transmission to neural plasticity and resilience (2, 3). Besides classical pharma-and psychotherapy, electroconvulsive therapy (ECT) has been established as effective treatment for MDD putatively via increase of adenosine concentrations (4).
Adenosine, acting as a neuromodulator, binds to four subtypes of G-protein-coupled receptors, A1, A2A, A2B and A3. Of these, the inhibitory A1 adenosine receptor (A1AR) shows highest affinity toward adenosine (5). In the rodent and human brain, A1ARs are ubiquitously expressed (6, 7) in neurons on somato-dendritic and axonal pre-and post-synaptic sites as well as in glia cells. Activation of presynaptic A1ARs mainly restrains the release of excitatory transmitters like glutamate from synaptic terminals, while the postsynaptic activation ultimately hyperpolarizes the membrane and inhibits signal transduction (8). With these mechanisms adenosine exerts homeostatic control of brain function, e.g., in epileptogenesis (9) and sleep–wake cycle (10), regulates synaptic plasticity, and supports neuroprotection in ischemic, hypoxic and oxidative stress events (11, 12).
Adenosine as the nucleoside precursor of the adenine-nucleotides AMP, ADP, and ATP stands in dynamic equilibrium with these compounds and is crucial for the energy metabolism of living organisms. In the extracellular space of the brain, adenosine either derives from the metabolism of adenosine-triphosphate (ATP) or is released from cells via bidirectional nucleoside transporters (5). Therefore, its concentration is closely related to energy consumption and sensitively indicates the balance between energy demand and supply on the synaptic level.
Specifically, adenosine has been identified as an endogenous anticonvulsant (9, 13). The high ATP breakdown during generalized seizures generates an excessive amount of adenosine with a transient increase of extracellular adenosine concentrations of more than 20-fold. In turn, consecutive activation of inhibitory A1ARs induces seizure arrest and postictal refractoriness (14). However, data on chronic regulation of A1AR in epilepsy remains inconclusive with observed up-and downregulations of the receptor in humans (15, 16) and rodent models (17–19). But, series of ECS in rats, artificially inducing seizures and mimicking human ECT, led to an increased expression of A1ARs in the cortex and other regions of the rat brain (20, 21).
In humans, therapeutic initiation of seizures with a subsequent surge of adenosine in terms of ECT is a potent therapeutic option in either severe or medication resistant depression (22–24). The effect of ECT seems to be rather associated with the intensity of generalized seizures than with the electric current (25). However, the molecular mechanisms of the antidepressant effects are not completely understood. Enhanced A1AR signaling either via transgenic knock-in or via injection of an A1AR agonist in wild-type mice was associated with a distinct resilience toward depressive-like behavior, whereas A1AR knockout induced opposite effects (26). Thus, adenosine and an upregulation of its A1AR might be involved in the persistent antidepressant efficacy of ECT particularly after repeated applications. Moreover, A1AR knockout mice showed a resistance toward antidepressant effects of SD, another well-established therapeutic option of MDD (27).
Altogether, there is evidence that adenosine and central inhibitory A1ARs might be crucial for antidepressant efficacy of primary non-pharmacological therapeutic interventions like ECT in MDD. As there is an urgent need of fast and reliably acting antidepressant therapies, especially in suicidal patients, it is essential to understand the molecular base of non-medical treatment options to refine therapeutic interventions.
The main aim of the current study was to investigate cerebral A1AR availability as a potential neurochemical basis for sustained ECT effects in patients with medication resistant severe MDD as originally proposed by van Calkar and Biber (28). A1AR availability was determined by the A1AR antagonist 8-cyclopentyl-3-(3-[18F]fluoropropyl)-1-propylxanthine ([18F]CPFPX) and positron emission tomography (PET) (29–31) before and after a series of ECT applications. As a secondary outcome, results were additionally correlated to clinical ratings of therapeutic effects, cognitive side effects as well as to inherent parameters of ECT like stimulus charge and seizure quality.
Material and methods
Subjects and study design
All study participants were inpatients of the Department of Psychiatry, Psychotherapy and Psychosomatics of the University Hospital, Aachen, Germany. Fifteen subjects (mean age 49.5 ± 11.8 years, range 26.2–63.9 years, 11 females) were included in the study after obtaining written informed consent. Except for one female, all subjects were right-handed. One of the females was excluded during data analysis due to cortical atrophy. For demographic parameters of the remaining 14 subjects, see Table 1.
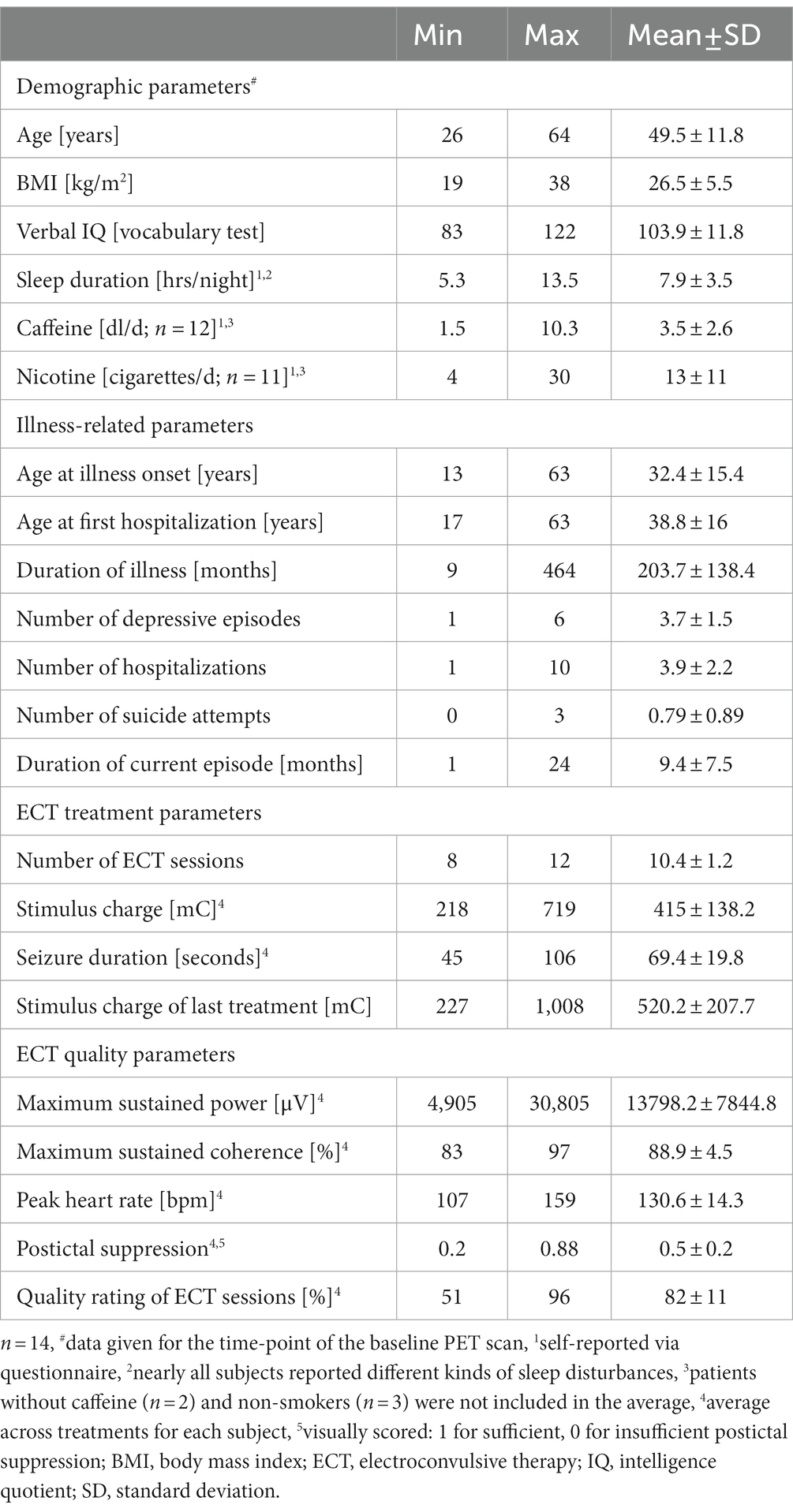
Table 1. Demographic and illness-related data as well as ECT treatment and quality parameters of study subjects.
Subjects had been diagnosed with recurrent moderate or severe major depressive episodes according to ICD-10 criteria (German edition, F33.1 (n = 1), F33.2 (n = 9) that were accompanied by psychotic symptoms (F33.3) in three subjects). One subject suffered from a single severe depressive episode with psychotic symptoms (F32.3). Psychiatric comorbidities were present in two subjects (personality disorder (F61, one male) and somatic symptom disorder (F45.0, one female)). All participants were classified as medication-resistant and had undergone at least two adequate antidepressant medications with different mechanisms of action. For further illness-related parameters, see Table 1.
At the time of study investigations all subjects were free of comorbidities or medication with a known influence on adenosine receptor expression. Further information on given medication can be found in Supplementary material and methods; Supplementary Table S1.
Subjects underwent baseline neuropsychological examination (4.1 ± 2.4 days before the PET investigation) and subsequently received the first baseline (BL) PET scan at the PET laboratory of the Institute of Neuroscience and Medicine, Forschungszentrum Jülich. ECT treatments started after a time interval of 1.36 ± 0.84 days after BL PET. After 10.4 ± 1.2 (range 8–12) ECT sessions, the second PET scan (follow-up, mean time lag between BL and follow-up PET: 43 ± 6.4 days) was scheduled with a time lag of 5.71 ± 2.7 (range 2–13) days after the last ECT session to minimize interference of acute effects with more persistent alterations of A1AR availability. Further neuropsychological ratings were scheduled 2.5 ± 4 days after the second PET scan. Prior to both PET scans, subjects refrained from any caffeine consumption for at least 36 h to preclude influences on PET pharmacology (32). All procedures were carried out on accordance with the Declaration of Helsinki and were approved by the Ethics Committees of the University Hospitals Düsseldorf and Aachen as well as by the German Federal Office for Radiation Protection.
Neuropsychological ratings
Neuropsychological test batteries for both ratings consisted of Beck Depression Inventory-2 (BDI-2), Global Assessment of Functioning (GAF), Montgomery-Åsberg Depression Rating Scale (MADRS), and Hamilton Depression Scale (HAMD-21). Mini Mental State Examination (MMSE), Verbal Learning and Memory Test (a modified version of Rey Auditory Verbal Learning Test, RAVLT), Wechsler Memory Scale (Working Memory (WMS WM) and Short Term Memory (WMS STM)) were supposed to measure potential cognitive side effects of ECT treatment. Intelligence Quotient (IQ) was estimated by Wortschatztest (WST), a German test for passive vocabulary. All ratings were conducted by psychologists of the neuropsychological team of the Department of Psychiatry, Psychotherapy and Psychosomatics of the University Hospital Aachen.
ECT treatments
Treatments were started with right unilateral (RUL) electrode position, a pulse width of 0.5 ms and a charge according to the age of the patient (33). Etomidate was applied as a narcotic (average dose 24.4 ± 5.6 mg) and succinylcholine as a muscle relaxant (average dose 113.7 ± 19.1 mg). Due to a strong anticonvulsive reaction, the narcotic was changed to S-ketamine (average dose 91.7 ± 28.9 mg) in three subjects during the course of the treatments.
The regular treatment frequency was twice a week with occasional reduction to one session in case of strong short-term cognitive side effects or required cardiovascular diagnostic assessments. In five patients, the electrode position was changed from RUL to left-anterior-right-temporal (LART) after at least six treatments due to unsatisfying clinical improvement. Re-Stimulation was performed in 1 to 4 ECT sessions of five patients with insufficient seizures. Further details on ECT treatment parameters are given in Table 1. All treatments were performed with the Thymatron System IV (SOMATICS, INC, Lake Bluff, IL, USA) in accordance with routinely used procedures (34–37).
Subsequent to the treatment, seizure quality was rated by analysis of the two frontal EEG and the ECG recordings according to the following criteria: EEG seizure duration >20 s, midictal amplitude >180 μV, maximal sustained coherence >85%, maximum heart rate > 110/min, and level of postictal suppression (see Table 1). Since the automatic evaluation of the postictal suppression index was often distorted by technical problems, it was qualitatively assigned to the categories ‘sufficient, insufficient or not applicable’ based on visual inspection of the investigating physician. All other parameters were based on the output of the Thymatron System. Seizure quality index for each session was given as the percentage of all applicable and fulfilled criteria ranging from zero to 100%. To compensate for the anticonvulsive effect, the stimulus charge was increased by 100 mC when the seizure quality turned out low.
Imaging procedures and data analysis
PET imaging was performed in supine position in quite ambiance with an ECAT EXACT HR+ Scanner (Siemens CTI). Scan duration was 90 min and started simultaneously with radiotracer injection via bolus-infusion with a Kbol of 63 min. [18F]CPFPX was produced in-house as previously described (31). Arterialized venous blood samples were collected 1, 5, and 10 min after scan start with subsequent sampling every 10 min.
Determination of radioactivity in whole blood and plasma as well as plasma metabolite analyses were performed as described previously (38). A1AR availability was determined as the tissue-to-plasma ratio (TPR) under equilibrium conditions from minutes 50–90. Under these conditions, the TPR equals the total distribution volume (VT) and can be expressed as the ratio of activity concentration in the respective region of interest (CROI) and the metabolite-corrected plasma (CP) input function: VT = CROI/CP. Further information on image processing is given in the Supplementary material and methods.
Statistical analyses
All data was first analyzed with regard to normal distribution via Shapiro–Wilk test. Differences in general parameters of PET scans as well as therapeutic impact of ECT and its potential side effects, determined via neuropsychological testing, were analyzed by two-tailed paired t-tests of the baseline and follow-up parameters. Interferences of regional A1AR availability by ECT treatment were further investigated with linear mixed model analysis with brain region and time as fixed parameters and random intercepts attributed to patients.
To examine relationships of an A1AR related molecular basis and antidepressive effects of ECT treatment, the average differences in A1AR availability across brain regions of each patient were correlated with individual neuropsychological rating scale changes. The same procedure was applied to determine influences of study design related parameters, anticonvulsive effects of ECT treatment, individual case history, and ECT quality indexes on changes in distinct A1AR availability. Pearson’s correlation coefficient (r) or Spearman’s rho (rs) were determined in dependency of normal distribution of underlying data. Level of significance was set to α < 0.05. All calculations were performed using SPSS Statistics (version 24.0, IBM Corp., Armonk, NY, USA).
Results
Baseline and follow-up PET scans were not significantly different regarding daytime of scan, injected dose, molar activity at time of injection, injected amount of substance, and mean rate of change of parent compound in plasma as an indicator for quality of equilibrium (see Supplementary Table S2).
Subject-specific parameters like BMI, sleep duration per night, caffeine intake and nicotine consumption did not differ between both scans. In some subjects, medication had to be modified due to patients complaining about side effects or to improve seizure quality.
Treatment of subjects with ECT resulted in a strong decline of the depressive symptoms as shown in Table 2 (upper part). None of the cognitive tests yielded significant differences before and after ECT treatment (Table 2, lower part). Regional A1AR availability as measured before and after ECT treatments is shown in Figure 1 (for absolute values see Supplementary Table S3). Distribution of cerebral A1ARs is heterogeneous as depicted in representative PET images of individual subjects for both the baseline as well as the follow-up condition (see Figure 2A).
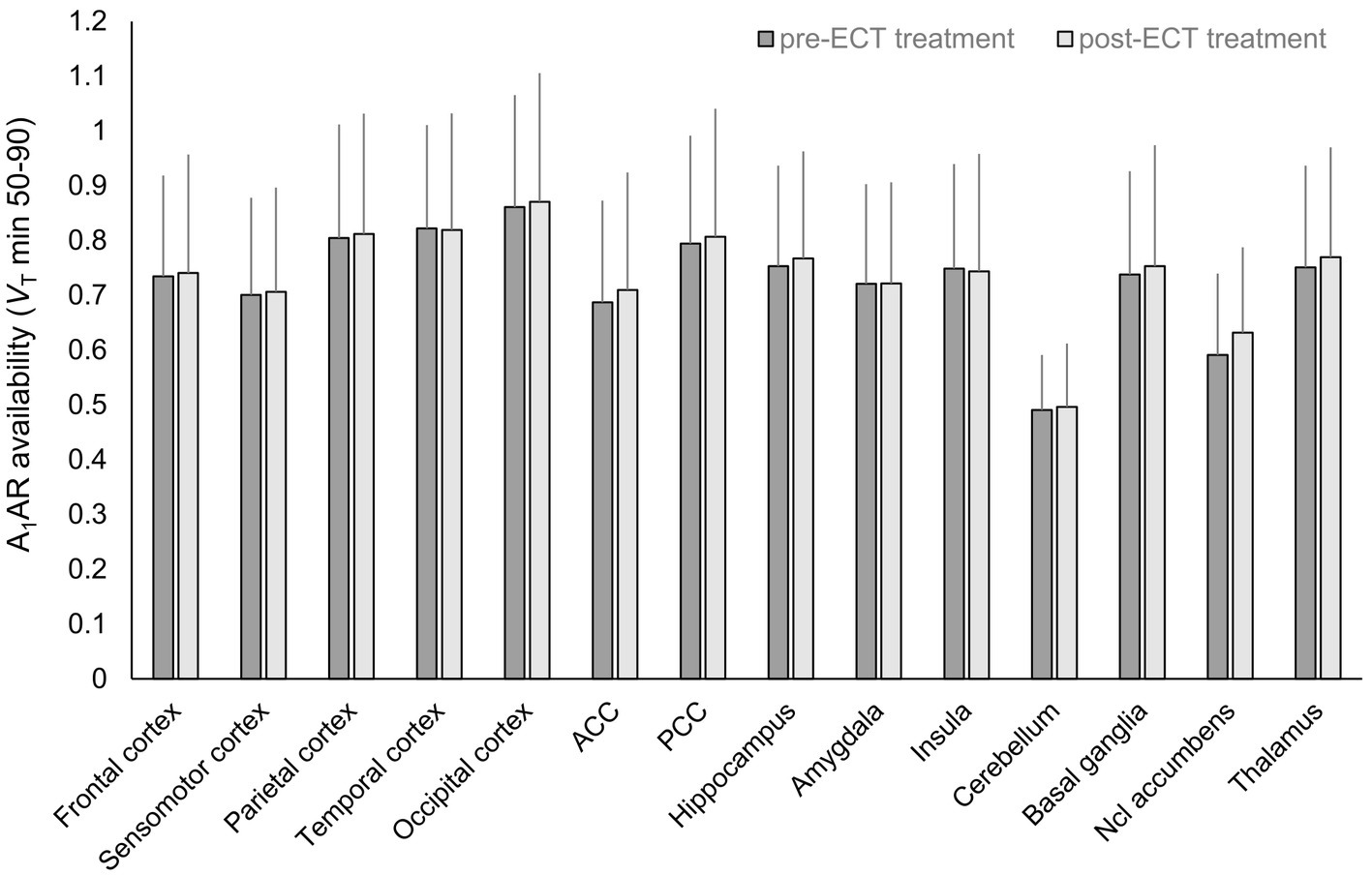
Figure 1. Regional A1AR availability before and after ECT treatment. n = 14, mean ± standard deviation, A1AR, A1 adenosine receptor; ACC, anterior cingulate cortex; ECT, electroconvulsive therapy; Ncl, nucleus; PCC, posterior cingulate cortex; VT, distribution volume in tissue.
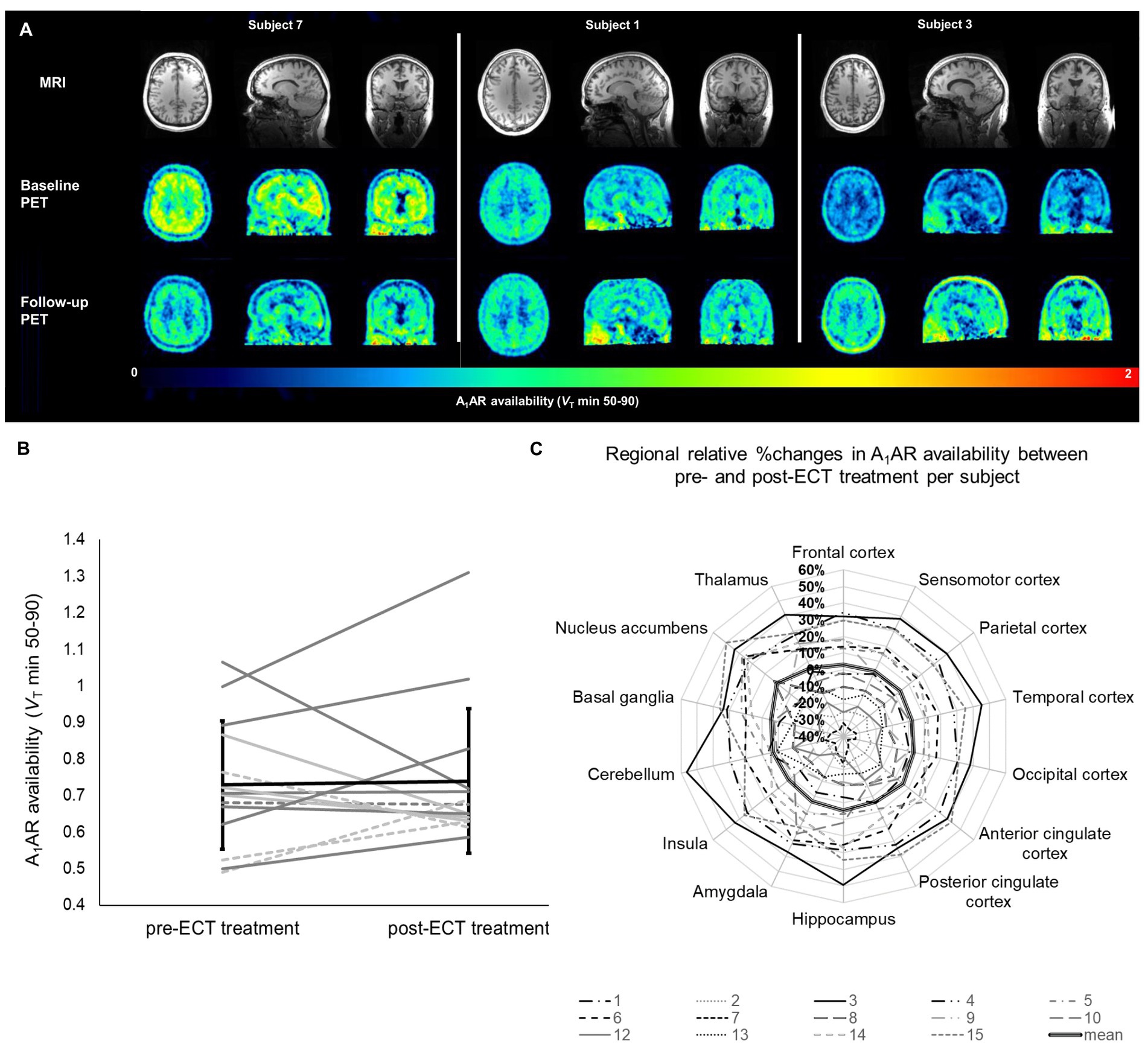
Figure 2. Individual changes in A1AR availability in relation to ECT treatment. (A) Parametric PET images demonstrating A1AR availability of three subjects representing the highly individual A1AR responses to ECT treatment. Given are transversal (left), sagittal (middle), and coronal (right) planes. Upper row shows individual MRI images used as anatomical references. (B) Mean A1AR availability per subject averaged over all investigated regions. Each line represents one subject, dotted lines depict male subjects, patients diagnosed other than F33.2 (including psychiatric comorbidities) are marked in light gray. Mean ± standard deviation for all subjects is given in black. (C) Regional relative changes given in % of pre-ECT A1AR availability. Each line depicts one subject. Bold line represents the mean of all subjects (n = 14). A1AR, A1 adenosine receptor availability; ECT, electroconvulsive therapy; MRI, magnetic resonance imaging; PET, positron emission tomography; VT, distribution volume in tissue.
In mixed model analysis the variable brain region significantly influenced A1AR availability (F = 12.8, p < 0.01), but not the variable time-point of measurement (F = 0.65, p = 0.42). As shown in Figure 2B alterations in A1AR availability across regions between pre-and post-ECT at group levels were marginal (mean ± SD average difference 3.93% ± 22.7%). Mean relative difference in A1AR availability per region (see Figure 2C; Supplementary Table S3) ranged from 2.03% ± 24.5% (insula) to 10.93% ± 27.85% (nucleus accumbens). Moreover, there was no interaction between brain region and time-point of measurement (F = 0.06, p = 1).
Noticeably, at the individual level subjects showed highly variable responses ranging from inclines of A1AR availability across regions after ECT treatment of 40% to declines of 32% (see Figure 2B). Nevertheless, at the level of specific brain regions, each subject showed high coherence in A1AR response over all investigated regions after ECT treatment (see Figure 2C) with a low within-subject share related to the 14 investigated brain regions of around 8% of the total variance of the differences in A1AR availability. According to the small contribution of the variable brain region to total variance, for further analysis the relative differences in A1AR availability between baseline and follow-up PET were averaged across all brain regions for each patient and then correlated with the individual clinical outcome determined via repeated neuropsychological ratings. Neither a relationship between A1AR variations and clinical responses nor a link to potential cognitive side effects of ECT treatment were found (see Supplementary Table S4). In line with this, an exploratory linear mixed model analysis with inclusion of the fixed factor response to ECT treatment (defined as a reduction of HAM-D scores of more than 50%) revealed no influence of clinical response on A1AR availability in relation to ECT treatment (F = 0.51, p = 0.49).
Furthermore, neither parameters related to study design like the number of applied ECT treatments (r = 0.35, p = 0.22) nor the time interval between the last ECT treatment and the follow-up PET (rs = −0.18, p = 0.54) nor individual case history with regard to total duration of depression (r = −0.17, p = 0.57) and number of depressive episodes (r = −0.42, p = 0.13) influenced A1AR responses in the investigated subjects. Stimulus charges of the last ECT prior to the follow-up PET (r = 0.44, p = 0.12, see Figure 3A) as well as the relative difference in stimulus intensities related to the initial charge of the first treatment session (r = 0.12, p = 0.7) as indicators of the anticonvulsive impact of ECT did not reveal any significant links to individual A1AR changes either.
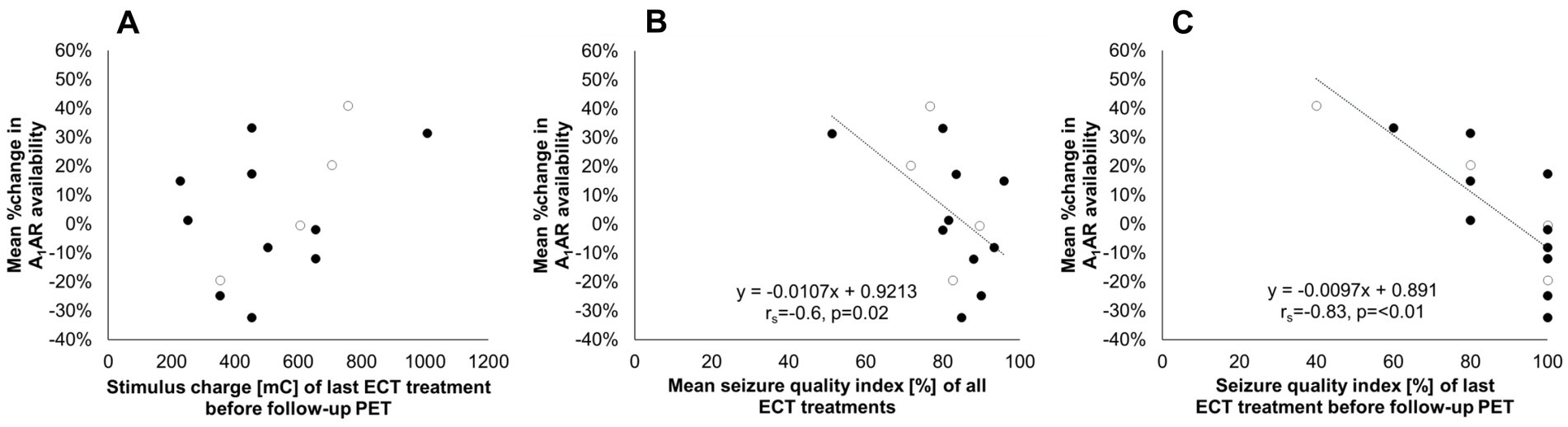
Figure 3. Changes in A1AR availability between pre-and post-ECT treatment in relation to treatment parameters. The vertical axis indicates the relative change in A1AR availability averaged across investigated regions per subject related to the respective baseline value before treatment. The horizontal axis indicates (A) Stimulus charge of the last ECT treatment before follow-up PET. (B) Seizure quality index as a mean of all ECT treatments. (C) Seizure quality index of the last ECT treatment before follow-up PET. Regression line and parameters for significant correlations are depicted in the graphs (B,C). Open circles represent male subjects. n = 14. A1AR, A1 adenosine receptor; ECT, electroconvulsive therapy; PET, positron emission tomography; rs, Spearman’s rho.
However, for the mean seizure quality index of the applied ECT treatments a significant association with average differences in A1AR availability across investigated regions per subject was found (rs = −0.6, p = 0.02, see Figure 3B), linking strong increases of receptor availability to low stimulus quality. This link was even more pronounced when correlating changes in A1AR availability with the seizure quality index of the last ECT treatment before follow-up PET (rs = −0.83, p = <0.01, see Figure 3C). Explorative analysis revealed that the maximal sustained coherence as part of the seizure quality index seems to be the main responsible parameter for the observed relationship.
Discussion
The present longitudinal in vivo study is to the best of our knowledge the first examining the relationship of cerebral A1AR availability, ECT treatment and clinical outcome parameters in medication-resistant depressive patients. Our main findings reveal that A1AR availability did not differ before and 5.7 days (2–11 days) after ECT treatment in any of the evaluated brain regions on the group level of investigated subjects. Meanwhile ECT proved to be clinically effective in all subjects with a considerable decrease of depressive symptoms. Although no group effects were detected, courses of A1AR availability were highly variable across patients, with inclines and declines of up to 30–40%. Interestingly, individual changes in A1AR availability were highly concordant across all investigated regions which points to stable individual reactions to ECT. However, these did not correspond to individual clinical outcomes and most treatment features. Solely mean seizure quality indexes showed correlations with individual changes in A1AR availability over time, a relationship which was even stronger when only the last ECT treatment before the follow-up PET was considered.
Previous preclinical studies indicated significant effects of adenosine (39) and the cerebral A1AR (26) on depressive behavior. In naïve mice, Kaster et al. (39) demonstrated effects of intraperitoneal adenosine injections on immobility in the forced swim as well as tail suspension test, being widely considered as a sign for depressive-like behavior. Serchov et al. (26) showed in the chronic behavioral despair mouse-model that increasing transgenic A1AR expression ameliorated typical depressive-like behaviors in paradigms like the forced swim test, tail suspension test and sucrose preference test. Given these observations as well as the fact that ECS resulted in an increased expression of A1ARs in rodents (20, 21), it was rather unexpected that on average A1AR availability in humans remains constant after a series of ECT applications. However, respective effects were observed in animal models and it is an ongoing debate to what extend rodent models comprehensively resemble the complex human condition of MDD. Current models of depression should meet criteria such as face validity (similarity of symptoms), predictive validity and construct validity and it is unambiguously hard to create models achieving high scores in all of these parameters (40). Especially it is at least challenging to separate the pathologies of chronic stress and depression in animal models. Thus, although indicators of behavioral despair in chronic unpredictable stress models rated high in specificity of depressive-like symptoms (41) it has to be critically reflected if repeated chronic swim stress as a rather severe stressor in mice might predominantly provoke stress responses at behavioral and molecular levels. In this context, Crema et al. (42) revealed an upregulation of the A1AR in the chronic unpredictable mild stress model and the chronic restraint stress model, both being used as preclinical models of depression. However, only animals in the chronic unpredictable mild stress group showed depressive-like behavior indicating that the observed regulation of the A1AR was primarily triggered by chronic stress. Behavioral readouts in rodent models of depression are critically discussed in the literature because of primarily assessing immobility, e.g., in the forced swim and tail suspension test, and thus coping with stressful situations rather than depression-like behavior (40, 43). Complexity of symptoms in human depression and related difficulties to model and translate subjective human emotions to specific rodent behaviors should be taken into account when interpreting and translating preclinical data to the clinical situation.
In humans, therapeutic ECT is performed in a conservative manner including muscle relaxation, carefully selected stimulation paradigms with limited total charge, continuous monitoring of the vital signs and a treatment frequency of 2–3 per week to increase tolerability. In particular, especially the hyper-oxygenation prior to the treatment and the maintenance of a constant oxygen saturation of 100% might be a crucial difference between human ECT regimes and rodent ECS studies for which no such precautions are described. In a cell model of smooth muscle cells chronic hypoxia thus led to a 3.5 fold increase in maximum specific binding of the A1AR antagonist [3H]DPCPX (44). Moreover, under transient hypoxic conditions, cultured astrocytes react with increasing extracellular adenosine levels to maintain brain homeostasis via reduction of presynaptic excitatory transmitter release in an A1AR-dependant mechanism which ultimately downregulates synaptic activity during seizures (45). In summary, preclinical investigations on ECS cannot be directly compared with the human condition – neither with regard to the strength of the seizure and the consecutive level of adenosine nor in view of other physiological parameters having an potential impact on energy metabolism and adenosine signaling. These discrepancies might result in longer-lasting and more intense regulations of the A1AR in rodent models due to stronger stimuli.
Altogether, the previously observed increases in A1ARs are more likely an adaptive mechanism than reflecting the molecular base of the long-lasting antidepressant efficacy of ECS/ECT. Indeed, adenosine and the A1AR act in an anticonvulsive manner ultimately terminating pathological seizures [for review (see 46)]. Adenosine surges and an altered A1AR expression might cause insufficient therapeutic seizure generation, short seizure duration as a component of seizure quality, as well as the need to adapt the stimulus charge during the course of ECTs. Diminished A1AR availability post-ECT in subjects with higher seizure quality may thus reflect limited compensatory mechanisms in response to induced convulsions in these subjects. Such A1AR mediated coping mechanisms were already observed by Elmenhorst et al. (47) in the context of SD and alcohol intake with subjects showing more pronounced increases in A1AR availability after intervention performed better in the psychomotor vigilance task. The dynamic reserve of the A1AR, i.e., the potential to increase A1AR availability upon stimulus, may thus reflect resilience to convulsions, alcohol and SD. Interestingly, some subjects showed a downregulation of A1AR availability after ECT treatment in the current study. This observation is in line with previously observed inconclusive results on regulation of A1AR availability in epilepsy as some studies indicated a decrease in receptor availability in both human disease and rodent epilepsy models (16–19). However, reasons for this putative individual vulnerability to convulsions remain unclear and warrants further investigation.
Although no consistent long-term effects of ECT on A1AR availability were determined, a more uniform, transient upregulation of the receptor after ECT cannot be excluded as the follow-up PET scan was performed with an average time lag of approximately 6 days after the last ECT treatment in the present study, thus avoiding interferences of acute ECT effects and A1AR quantification. In previous preclinical investigations on A1AR availability after ECS (20, 21) short-term effects in the immediate postictal phase might have been mimicked by a competition of massively increased endogenous adenosine and the utilized agonistic radioligand.
SD, as another antidepressant therapy induces such short-term upregulations of the A1AR (48) but just a single night of recovery sleep after 52 h of SD was able to completely restore A1AR availability to baseline levels (49). In line, recovery sleep in patients with MDD lead to a relapse of depressive symptoms in most SD-responders (50). Although SD elicits short-term antidepressive actions and the underlying molecular mechanisms are not completely understood it seems to be at least partly mediated by the A1AR (51). Despite the putative short-term antidepressive efficacy, these studies indicate that the turnover of components of the adenosinergic system is at least faster than 2–11 days in humans. This finding supports the hypothesis of a rapid adaption of this system in response to ECT induced adenosine surges but with only short-term antidepressive effects.
A theoretical study design to address short-term interactions of adenosine and the A1AR would employ an agonistic radiotracer and continuous PET acquisition during a running ECT treatment. By this, effects of repetitive ECT on adenosine and its binding to A1ARs could be investigated. Moreover, as high adenosine surges occurred during ECT and several hints point toward an involvement of the purinergic system in mood disorders, other targets like the adenosine A2A adenosine receptor (A2AAR), the ATP receptor P2X7 as well as interactions of the A1AR/A2AAR and the adenosinergic and dopaminergic system warrant further investigations. Recently, it was shown, that ketamin-induced increases in axon terminal density, measured via determination of synaptic vesicle protein 2 and PET, might be crucial for reduction of depressive symptoms in subgroups of depressed patients with specific molecular characteristics (52). Keeping this in mind, future research on pathophysiological mechanism of MDD should also focus on interactions of the neuromodulator adenosine and restoration of synaptic connections. Furthermore, larger and more homogenous samples would offer the opportunity to classify patients in subgroups on the basis of their molecular and behavioral traits to further elucidate mechanisms of treatment response with the ultimate goal of a more individualized therapy of MDD.
Our rather heterogeneous sample closely depicts the common clinical situation of therapy-resistant severely depressed patients being treated with ECT. However, besides age, heterogeneous treatment parameters as well as individual history of disease, medication and psychiatric diagnosis will surely introduce a relevant variability. In the present sample the coefficient of variation averaged over all patients and investigated regions amounted to 24% before and 27% after the treatment. This is only slightly higher than in our previous studies on effects of SD in which the coefficient of variation was approximately 20% (averaged across brain regions and subjects (48)). Though, taken into consideration that the current sample consists of severely depressed patients of different gender, age, smoker status and medication, interindividual variance of data seems proportional in comparison to the male, young, non-smoking and non-medicated group of healthy volunteers measured in our previous SD studies. Apart from one subject, all patients showed remarkable improvement of symptoms after ECT treatment. Response rates between 50 and 86% (depending on the applied neuropsychological test and cut off) in the current sample are in the expected range (53), further highlighting that the sample is representative despite the relatively small sample size which is however in the range of other clinical PET studies performed in severely diseased patients (54–58). In such investigations especially with observations over longer time periods, oftentimes, individual medication and therapy regimes had to be adapted to the actual status and burden of the patient which automatically resulted in slightly varying treatments over the course of several weeks. Notwithstanding, in the present sample neither individual history of disease nor specific parameters of ECT treatments, like, e.g., number of treatment sessions, time intervals between the last ECT treatment and follow-up PET investigation, electrode position, and changes in stimulus charges had an influence on individual changes of A1AR availability. Moreover, usage of S-ketamine in three subjects for anesthesia during ECT did not influence antidepressant effects of ECT treatment in the current sample as relative changes in, e.g., HAM-D in two of these subjects were less than the average change across the sample. In addition, a direct effect of S-ketamine on A1AR availability is not known up to now which is mirrored in the current sample with heterogeneous responses of A1AR availability after ECT treatment in the respective patients. Nevertheless, one of the main limitations of the current study is the missing control group. However, longitudinal PET imaging with [18F]CPFPX was proven reliable with a moderately high test–retest stability as determined on consecutive days (30). Comparisons of test–retest parameters in rodents for different interscan intervals (59, 60) moreover indicates that A1AR receptor availability as determined via [18F]CPFPX-PET is stable over periods of up to more than 3 months which is longer than the interscan intervals of the current study. Intraindividual changes of A1AR availability between baseline and follow-up PET observed in the current study were of greater magnitude as previously determined in the human test–retest study (30). Thus, percentage A1AR variability across regions between the two scans of the current study were approximately elevated by half in comparison to standard test–retest conditions (~19% vs. ~13%). Furthermore, coefficients of variation for determined A1AR variability between both time points across regions and subjects were additionally increased within the same range (~63% vs. ~40%). In the context of the rather marginal increase of absolute variability in A1AR distribution volume measured in patients with MDD in comparison to healthy subjects (see above) and further PET-specific methodological considerations (see Supplementary Discussion), it seems likely, that more patient-centered factors mainly determine the high variability of A1AR responses to ECT treatment. Although these factors are not known in detail yet, it appears as they mirror more robust individual characteristics presumably in form of an anticonvulsive response mechanism.
In conclusion, our present study shows that the cerebral A1AR does not exhibit long-term changes that could directly explain the antidepressant effects of ECT. Short-term antidepressive efficacy of A1AR regulation can, however, not be excluded based on the current study design. Highly individual A1AR responses to ECT treatment showed coherence across brain regions within the same subjects but no correlation with treatment outcomes. Future studies on effects of ECT treatment warrant bigger sample sizes to further elucidate the complex interplay of molecular and behavioral changes with putative individual trademarks in the depressive brain.
Data availability statement
The raw data supporting the conclusions of this article will be made available by the authors, without undue reservation.
Ethics statement
The studies involving human participants were reviewed and approved by Ethics Committees of the University Hospitals Düsseldorf and Aachen. The patients/participants provided their written informed consent to participate in this study.
Author contributions
TK, MG, and AB conceptualized and designed the study. MG recruited patients. TK, MG, AM, and AN acquired the data, while TK, MG, DE, and AB analyzed and interpreted data. TK provided a first draft of the manuscript. MG, AM, DE, AN, FS, and AB subsequently revised the manuscript. All authors contributed to the article and approved the submitted version.
Acknowledgments
The excellent technical assistance of Sylvia Köhler-Dibowski, Stephanie Krause, Dorothe Krug Magdalene Vögeling, Susanne Schaden, Silke Frensch, and Lutz Tellmann (Forschungszentrum Jülich) is gratefully acknowledged. We thank Maria Klingebiel and Alina Treichel for valuable support in data processing. Further, we thank the Department of Radiochemistry (INM-5) of Forschungszentrum Jülich for supplying the radioligand.
Conflict of interest
The authors declare that the research was conducted in the absence of any commercial or financial relationships that could be construed as a potential conflict of interest.
Publisher’s note
All claims expressed in this article are solely those of the authors and do not necessarily represent those of their affiliated organizations, or those of the publisher, the editors and the reviewers. Any product that may be evaluated in this article, or claim that may be made by its manufacturer, is not guaranteed or endorsed by the publisher.
Supplementary material
The Supplementary material for this article can be found online at: https://www.frontiersin.org/articles/10.3389/fpsyt.2023.1228438/full#supplementary-material
References
1. World Health Organisation. Depression and other common mental disorders: Global Health estimates. (2017). Contract No.: C BY-NC-SA 3.0 IGO. World Health Organization.
2. Coyle, JT, and Duman, RS. Finding the intracellular signaling pathways affected by mood disorder treatments. Neuron. (2003) 38:157–60. doi: 10.1016/S0896-6273(03)00195-8
3. Manji, HK, Drevets, WC, and Charney, DS. The cellular neurobiology of depression. Nat Med. (2001) 7:541–7. doi: 10.1038/87865
4. Sadek, AR, Knight, GE, and Burnstock, G. Electroconvulsive therapy: a novel hypothesis for the involvement of purinergic signalling. Purinergic Signal. (2011) 7:447–52. doi: 10.1007/s11302-011-9242-y
5. Borea, PA, Gessi, S, Merighi, S, Vincenzi, F, and Varani, K. Pharmacology of adenosine receptors: the state of the art. Physiol Rev. (2018) 98:1591–625. doi: 10.1152/physrev.00049.2017
6. Fastbom, J, Pazos, A, and Palacios, JM. The distribution of adenosine A1 receptors and 5′-nucleotidase in the brain of some commonly used experimental animals. Neuroscience. (1987) 22:813–26. doi: 10.1016/0306-4522(87)92961-7
7. Fastbom, J, Pazos, A, Probst, A, and Palacios, JM. Adenosine A1 receptors in the human brain: a quantitative autoradiographic study. Neuroscience. (1987) 22:827–39. doi: 10.1016/0306-4522(87)92962-9
8. Sebastião, AM, and Ribeiro, JA. Adenosine receptors and the central nervous system In: CN Wilson and SJ Mustafa, editors. Adenosine receptors in health and disease. Berlin, Heidelberg: Springer Berlin Heidelberg (2009). 471–534.
9. Boison, D, Sandau, US, Ruskin, DN, Kawamura, M Jr, and Masino, SA. Homeostatic control of brain function - new approaches to understand epileptogenesis. Front Cell Neurosci. (2013) 7:109. doi: 10.3389/fncel.2013.00109
10. Porkka-Heiskanen, T, and Kalinchuk, AV. Adenosine, energy metabolism and sleep homeostasis. Sleep Med Rev. (2011) 15:123–35. doi: 10.1016/j.smrv.2010.06.005
11. Cunha, RA. Adenosine as a neuromodulator and as a homeostatic regulator in the nervous system: different roles, different sources and different receptors. Neurochem Int. (2001) 38:107–25. doi: 10.1016/S0197-0186(00)00034-6
12. Sheth, S, Brito, R, Mukherjea, D, Rybak, LP, and Ramkumar, V. Adenosine receptors: expression, function and regulation. Int J Mol Sci. (2014) 15:2024–52. doi: 10.3390/ijms15022024
13. Van Gompel, JJ, Bower, MR, Worrell, GA, Stead, M, Chang, SY, Goerss, SJ, et al. Increased cortical extracellular adenosine correlates with seizure termination. Epilepsia. (2014) 55:233–44. doi: 10.1111/epi.12511
14. During, MJ, and Spencer, DD. Adenosine: a potential mediator of seizure arrest and postictal refractoriness. Ann Neurol. (1992) 32:618–24. doi: 10.1002/ana.410320504
15. Angelatou, F, Pagonopoulou, O, Maraziotis, T, Olivier, A, Villemeure, JG, Avoli, M, et al. Upregulation of A1 adenosine receptors in human temporal lobe epilepsy: a quantitative autoradiographic study. Neurosci Lett. (1993) 163:11–4. doi: 10.1016/0304-3940(93)90217-9
16. Glass, M, Faull, RL, Bullock, JY, Jansen, K, Mee, EW, Walker, EB, et al. Loss of A1 adenosine receptors in human temporal lobe epilepsy. Brain Res. (1996) 710:56–68. doi: 10.1016/0006-8993(95)01313-X
17. Ekonomou, A, Sperk, G, Kostopoulos, G, and Angelatou, F. Reduction of A1 adenosine receptors in rat hippocampus after kainic acid-induced limbic seizures. Neurosci Lett. (2000) 284:49–52. doi: 10.1016/S0304-3940(00)00954-X
18. Ochiishi, T, Takita, M, Ikemoto, M, Nakata, H, and Suzuki, SS. Immunohistochemical analysis on the role of adenosine A1 receptors in epilepsy. Neuroreport. (1999) 10:3535–41. doi: 10.1097/00001756-199911260-00014
19. Rebola, N, Coelho, JE, Costenla, AR, Lopes, LV, Parada, A, Oliveira, CR, et al. Decrease of adenosine A1 receptor density and of adenosine neuromodulation in the hippocampus of kindled rats. Eur J Neurosci. (2003) 18:820–8. doi: 10.1046/j.1460-9568.2003.02815.x
20. Gleiter, CH, Deckert, J, Nutt, DJ, and Marangos, PJ. Electroconvulsive shock (ECS) and the adenosine neuromodulatory system: effect of single and repeated ECS on the adenosine A1 and A2 receptors, adenylate cyclase, and the adenosine uptake site. J Neurochem. (1989) 52:641–6. doi: 10.1111/j.1471-4159.1989.tb09168.x
21. Newman, M, Zohar, J, Kalian, M, and Belmaker, RH. The effects of chronic lithium and ECT on A1 and A2 adenosine receptor systems in rat brain. Brain Res. (1984) 291:188–92. doi: 10.1016/0006-8993(84)90670-X
22. Grözinger, M, Smith, ES, and Conca, A. On the significance of electroconvulsive therapy in the treatment of severe mental diseases. Wien Klin Wochenschr. (2015) 127:297–302. doi: 10.1007/s00508-015-0749-z
23. Kellner, CH, Obbels, J, and Sienaert, P. When to consider electroconvulsive therapy (ECT). Acta Psychiatr Scand. (2020) 141:304–15. doi: 10.1111/acps.13134
24. Socci, C, Medda, P, Toni, C, Lattanzi, L, Tripodi, B, Vannucchi, G, et al. Electroconvulsive therapy and age: age-related clinical features and effectiveness in treatment resistant major depressive episode. J Affect Disord. (2018) 227:627–32. doi: 10.1016/j.jad.2017.11.064
25. Kirstein, L, and Ottosson, JO. Experimental studies of electroencephalographic changes following electroconvulsive therapy: the role of the electrical stimulation and of the seizure studied by variation of stimulus intensity and modification by lidocaine of seizure discharge. Acta Psychiatr Scand Suppl. (1960) 35:49–67.
26. Serchov, T, Clement, HW, Schwarz, MK, Iasevoli, F, Tosh, DK, Idzko, M, et al. Increased Signaling via adenosine A1 receptors, sleep deprivation, imipramine, and ketamine inhibit depressive-like behavior via induction of Homer1a. Neuron. (2015) 87:549–62. doi: 10.1016/j.neuron.2015.07.010
27. Ioannou, M, Wartenberg, C, Greenbrook, JTV, Larson, T, Magnusson, K, Schmitz, L, et al. Sleep deprivation as treatment for depression: systematic review and meta-analysis. Acta Psychiatr Scand. (2021) 143:22–35. doi: 10.1111/acps.13253
28. van Calker, D, and Biber, K. The role of glial adenosine receptors in neural resilience and the neurobiology of mood disorders. Neurochem Res. (2005) 30:1205–17. doi: 10.1007/s11064-005-8792-1
29. Bauer, A, Holschbach, MH, Meyer, PT, Boy, C, Herzog, H, Olsson, RA, et al. In vivo imaging of adenosine A1 receptors in the human brain with [18F]CPFPX and positron emission tomography. NeuroImage. (2003) 19:1760–9. doi: 10.1016/S1053-8119(03)00241-6
30. Elmenhorst, D, Meyer, PT, Matusch, A, Winz, OH, Zilles, K, and Bauer, A. Test-retest stability of cerebral A1 adenosine receptor quantification using [18F]CPFPX and PET. Eur J Nucl Med Mol Imaging. (2007) 34:1061–70. doi: 10.1007/s00259-006-0309-x
31. Holschbach, MH, Olsson, RA, Bier, D, Wutz, W, Sihver, W, Schüller, M, et al. Synthesis and evaluation of no-carrier-added 8-cyclopentyl-3-(3-[(18)F]fluoropropyl)-1-propylxanthine ([(18)F]CPFPX): a potent and selective A(1)-adenosine receptor antagonist for in vivo imaging. J Med Chem. (2002) 45:5150–6. doi: 10.1021/jm020905i
32. Elmenhorst, D, Meyer, PT, Matusch, A, Winz, OH, and Bauer, A. Caffeine occupancy of human cerebral A1 adenosine receptors: in vivo quantification with 18F-CPFPX and PET. J Nucl Med. (2012) 53:1723–9. doi: 10.2967/jnumed.112.105114
33. Loh, N, Nickl-Jockschat, T, Sheldrick, AJ, and Grözinger, M. Accessibility, standards and challenges of electroconvulsive therapy in Western industrialized countries: a German example. World J Biol Psychiatry. (2013) 14:432–40. doi: 10.3109/15622975.2012.665176
34. Brunner, I, and Grözinger, M. Brief vs. ultrabrief pulse ECT: focus on seizure quality. Eur Arch Psychiatry Clin Neurosci. (2018) 268:831–8. doi: 10.1007/s00406-018-0925-z
35. Kranaster, L, Aksay, SS, Bumb, JM, Hoyer, C, Jennen-Steinmetz, C, and Sartorius, A. A novel seizure quality index based on ictal parameters for optimizing clinical decision making in electroconvulsive therapy. Part 1: development. Eur Arch Psychiatry Clin Neurosci. (2018) 268:819–30. doi: 10.1007/s00406-018-0910-6
36. Minelli, A, Abate, M, Zampieri, E, Gainelli, G, Trabucchi, L, Segala, M, et al. Seizure adequacy markers and the prediction of electroconvulsive therapy response. J ECT. (2016) 32:88–92. doi: 10.1097/YCT.0000000000000274
37. Taylor, R, Wark, H, Leyden, J, Simpson, B, McGoldrick, J, Hadzi-Pavlovic, D, et al. Effects of the anaesthetic-ECT time interval and ventilation rate on seizure quality in electroconvulsive therapy: a prospective randomised trial. Brain Stimul. (2020) 13:450–6. doi: 10.1016/j.brs.2019.12.012
38. Meyer, PT, Elmenhorst, D, Bier, D, Holschbach, MH, Matusch, A, Coenen, HH, et al. Quantification of cerebral A1 adenosine receptors in humans using [18F]CPFPX and PET: an equilibrium approach. NeuroImage. (2005) 24:1192–204. doi: 10.1016/j.neuroimage.2004.10.029
39. Kaster, MP, Rosa, AO, Rosso, MM, Goulart, EC, Santos, AR, and Rodrigues, AL. Adenosine administration produces an antidepressant-like effect in mice: evidence for the involvement of A1 and A2A receptors. Neurosci Lett. (2004) 355:21–4. doi: 10.1016/j.neulet.2003.10.040
40. Harro, J. Animal models of depression: pros and cons. Cell Tissue Res. (2019) 377:5–20. doi: 10.1007/s00441-018-2973-0
41. Cryan, JF, Markou, A, and Lucki, I. Assessing antidepressant activity in rodents: recent developments and future needs. Trends Pharmacol Sci. (2002) 23:238–45. doi: 10.1016/S0165-6147(02)02017-5
42. Crema, LM, Pettenuzzo, LF, Schlabitz, M, Diehl, L, Hoppe, J, Mestriner, R, et al. The effect of unpredictable chronic mild stress on depressive-like behavior and on hippocampal A1 and striatal A2A adenosine receptors. Physiol Behav. (2013) 109:1–7. doi: 10.1016/j.physbeh.2012.11.001
43. Commons, KG, Cholanians, AB, Babb, JA, and Ehlinger, DG. The rodent forced swim test measures stress-coping strategy. Not Depression Like Behavior ACS Chem Neurosci. (2017) 8:955–60. doi: 10.1021/acschemneuro.7b00042
44. Hammond, LC, Bonnet, C, Kemp, PJ, Yates, MS, and Bowmer, CJ. Chronic hypoxia up-regulates expression of adenosine A1 receptors in DDT1-MF2 cells. Biochem Pharmacol. (2004) 67:421–6. doi: 10.1016/j.bcp.2003.09.003
45. Martín, ED, Fernández, M, Perea, G, Pascual, O, Haydon, PG, Araque, A, et al. Adenosine released by astrocytes contributes to hypoxia-induced modulation of synaptic transmission. Glia. (2007) 55:36–45. doi: 10.1002/glia.20431
46. Weltha, L, Reemmer, J, and Boison, D. The role of adenosine in epilepsy. Brain Res Bull. (2019) 151:46–54. doi: 10.1016/j.brainresbull.2018.11.008
47. Elmenhorst, EM, Elmenhorst, D, Benderoth, S, Kroll, T, Bauer, A, and Aeschbach, D. Cognitive impairments by alcohol and sleep deprivation indicate trait characteristics and a potential role for adenosine A(1) receptors. Proc Natl Acad Sci U S A. (2018) 115:8009–14. doi: 10.1073/pnas.1803770115
48. Elmenhorst, D, Meyer, PT, Winz, OH, Matusch, A, Ermert, J, Coenen, HH, et al. Sleep deprivation increases A1 adenosine receptor binding in the human brain: a positron emission tomography study. J Neurosci. (2007) 27:2410–5. doi: 10.1523/JNEUROSCI.5066-06.2007
49. Elmenhorst, D, Elmenhorst, EM, Hennecke, E, Kroll, T, Matusch, A, Aeschbach, D, et al. Recovery sleep after extended wakefulness restores elevated A(1) adenosine receptor availability in the human brain. Proc Natl Acad Sci U S A. (2017) 114:4243–8. doi: 10.1073/pnas.1614677114
50. Giedke, H, and Schwärzler, F. Therapeutic use of sleep deprivation in depression. Sleep Med Rev. (2002) 6:361–77. doi: 10.1053/smrv.2002.0235
51. Hines, DJ, Schmitt, LI, Hines, RM, Moss, SJ, and Haydon, PG. Antidepressant effects of sleep deprivation require astrocyte-dependent adenosine mediated signaling. Transl Psychiatry. (2013) 3:e212. doi: 10.1038/tp.2012.136
52. Holmes, SE, Finnema, SJ, Naganawa, M, DellaGioia, N, Holden, D, Fowles, K, et al. Imaging the effect of ketamine on synaptic density (SV2A) in the living brain. Mol Psychiatry. (2022). 4:2273–2281. doi: 10.1038/s41380-022-01465-2
53. Cusin, C, and Dougherty, DD. Somatic therapies for treatment-resistant depression: ECT, TMS, VNS. DBS Biol Mood Anxiety Disord. (2012) 2:14. doi: 10.1186/2045-5380-2-14
54. Baldinger-Melich, P, Gryglewski, G, Philippe, C, James, GM, Vraka, C, Silberbauer, L, et al. The effect of electroconvulsive therapy on cerebral monoamine oxidase a expression in treatment-resistant depression investigated using positron emission tomography. Brain Stimul. (2019) 12:714–23. doi: 10.1016/j.brs.2018.12.976
55. Gilbert, TM, Zürcher, NR, Wu, CJ, Bhanot, A, Hightower, BG, Kim, M, et al. PET neuroimaging reveals histone deacetylase dysregulation in schizophrenia. J Clin Invest. (2019) 129:364–72. doi: 10.1172/JCI123743
56. Ishibashi, K, Miura, Y, Wagatsuma, K, Toyohara, J, Ishiwata, K, and Ishii, K. Occupancy of adenosine A(2A) receptors by istradefylline in patients with Parkinson's disease using (11)C-preladenant PET. Neuropharmacology. (2018) 143:106–12. doi: 10.1016/j.neuropharm.2018.09.036
57. Ossenkoppele, R, Tolboom, N, Foster-Dingley, JC, Adriaanse, SF, Boellaard, R, Yaqub, M, et al. Longitudinal imaging of Alzheimer pathology using [11C]PIB, [18F]FDDNP and [18F]FDG PET. Eur J Nucl Med Mol Imaging. (2012) 39:990–1000. doi: 10.1007/s00259-012-2102-3
58. Rissanen, E, Virta, JR, Paavilainen, T, Tuisku, J, Helin, S, Luoto, P, et al. Adenosine A2A receptors in secondary progressive multiple sclerosis: a [(11)C]TMSX brain PET study. J Cereb Blood Flow Metab. (2013) 33:1394–401. doi: 10.1038/jcbfm.2013.85
59. Kroll, T, Elmenhorst, D, Weisshaupt, A, Beer, S, and Bauer, A. Reproducibility of non-invasive a1 adenosine receptor quantification in the rat brain using [(18)F]CPFPX and positron emission tomography. Mol Imaging Biol. (2014) 16:699–709. doi: 10.1007/s11307-014-0729-0
Keywords: A1 adenosine receptor, electroconvulsive therapy, positron emission tomography, major depressive disorder, seizure quality
Citation: Kroll T, Grözinger M, Matusch A, Elmenhorst D, Novakovic A, Schneider F and Bauer A (2023) Effects of electroconvulsive therapy on cerebral A1 adenosine receptor availability: a PET study in patients suffering from treatment-resistant major depressive disorder. Front. Psychiatry. 14:1228438. doi: 10.3389/fpsyt.2023.1228438
Edited by:
Po-Han Chou, China Medical University, TaiwanReviewed by:
Chun-Hung Chang, China Medical University Hospital, TaiwanShaw-Ji Chen, Taitung MacKay Memorial Hospital, Taiwan
Copyright © 2023 Kroll, Grözinger, Matusch, Elmenhorst, Novakovic, Schneider and Bauer. This is an open-access article distributed under the terms of the Creative Commons Attribution License (CC BY). The use, distribution or reproduction in other forums is permitted, provided the original author(s) and the copyright owner(s) are credited and that the original publication in this journal is cited, in accordance with accepted academic practice. No use, distribution or reproduction is permitted which does not comply with these terms.
*Correspondence: Tina Kroll, t.kroll@fz-juelich.de
†These authors have contributed equally to this work