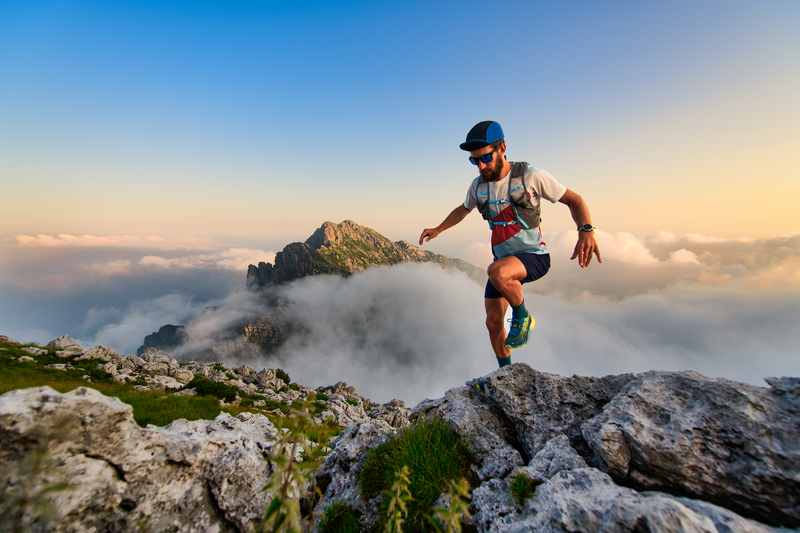
94% of researchers rate our articles as excellent or good
Learn more about the work of our research integrity team to safeguard the quality of each article we publish.
Find out more
REVIEW article
Front. Psychiatry , 20 June 2023
Sec. Social Neuroscience
Volume 14 - 2023 | https://doi.org/10.3389/fpsyt.2023.1205199
This article is part of the Research Topic The Four Streams of the Prefrontal Cortex View all 10 articles
Social interaction is a complex behavior which requires the individual to integrate various internal processes, such as social motivation, social recognition, salience, reward, and emotional state, as well as external cues informing the individual of others’ behavior, emotional state and social rank. This complex phenotype is susceptible to disruption in humans affected by neurodevelopmental and psychiatric disorders, including autism spectrum disorder (ASD). Multiple pieces of convergent evidence collected from studies of humans and rodents suggest that the prefrontal cortex (PFC) plays a pivotal role in social interactions, serving as a hub for motivation, affiliation, empathy, and social hierarchy. Indeed, disruption of the PFC circuitry results in social behavior deficits symptomatic of ASD. Here, we review this evidence and describe various ethologically relevant social behavior tasks which could be employed with rodent models to study the role of the PFC in social interactions. We also discuss the evidence linking the PFC to pathologies associated with ASD. Finally, we address specific questions regarding mechanisms employed by the PFC circuitry that may result in atypical social interactions in rodent models, which future studies should address.
The prefrontal cortex (PFC) is critical for various aspects of mammalian social behavior, including social motivation, recognition, and decision-making (1–3). In humans, the medial PFC (mPFC) is involved in high-order aspects of social interaction, such as self-referential processing, mentalizing, and emotional regulation (4–6). At the same time, deficits in PFC function have been implicated in various neuropsychiatric disorders, including autism spectrum disorder (ASD). Individuals with ASD exhibit atypical social behavior and deficits in social cognition, such as an impaired theory of mind and a lack of social interest (7, 8). Neuroimaging studies have revealed altered PFC activity in individuals with ASD during social tasks (9, 10). As such, understanding the molecular, cellular, and network mechanisms underlying the role of the PFC in social behavior and its dysfunction in ASD may be critical for developing effective treatments for individuals diagnosed with this disorder.
Research using animal models has provided significant insight into the neural circuitry underlying social behavior, including the role of the PFC in social interactions (11–14). Anatomically, the PFC is a complex brain structure with multiple sub-regions, each with a distinct function and connectivity pattern (15, 16). In rodents, most studies have focused on the mPFC, including the prelimbic and infralimbic regions and their downstream projections to the striatum (17), amygdala (18), hypothalamus (19), hippocampus (20) and brainstem (21). These sub-regions were shown to be involved in various aspects of social behavior, including social recognition (22), social approach (23), and aggression (24, 25). Moreover, studies have demonstrated that rodents exhibit complex social behaviors, including social hierarchy (26), empathy (27), and territoriality (28), making them a valuable model for studying the biological mechanisms underlying mammalian social behavior. Accordingly, multiple behavioral tasks have been developed to assess rodent social behavior and the role of the PFC therein, including the three-chamber, social recognition, social habitation/dishabituation, and resident-intruder tests (29–32). Such studies have shown that mPFC lesions or manipulations can lead to deficits in social behavior in rodents (33, 34).
Here, we provide an overview of the role of the PFC in the social behavior of animal models and the implications for understanding possible mechanisms underlying social deficits in ASD. The review discusses anatomical and functional homologies of the PFC in rodents and humans, and its role in various aspects of social interactions. Additionally, current literature on PFC involvement in social behavior deficits that lead to ASD symptoms is highlighted.
Social interactions involve complex information-processing tasks that can broadly be defined as detecting and interpreting social cues and responding appropriately to evolving social contexts (3). By nature, social interactions are multi-faceted and require the integration of external multi-modal sensory information with internal processes. Here, we aim to focus on the following aspects of the process: (1) the motivation for social interaction, which is an internal process; (2) emotional/empathic reactions in response to social cues; and (3) group dynamics, which involve mutual relationship between the subject and others (4, 35–37). These aspects are not mutually exclusive (38) and together affect behavioral decisions. This is exemplified by going out to dinner at a restaurant. This involves interactions with the staff, the degree to which heavily relies on the internal motivation of the subject to interact. The subject’s satisfaction with the food and the staff performance, as well as the subject’s perception of their emotions. Will lead the subject to either compliment or complain about the staff. Moreover, verbal and emotional communication between the dining partners during dinner will depend on whether the environment is friendly or professional. Thus, social motivation, emotional perception of self and others, group dynamics, and the social context all integrate to determine social behavior.
Social motivation, or the willingness to pursue social interactions, is a fundamental aspect of the decision-making process in a social context. Such motivation and subsequent rewarding experiences require the subject to approach social partners and engage them (35). Accordingly, approaching a conspecific is a highly conserved phenotype in multiple species (38, 39). This aspect of social behavior and cognition emerges early in development, with young infants tending to recognize and initiate interactions with their parents (40, 41). Infants must thus recognize familiar faces for proper decision-making in their social contexts from a very early age (42, 43). Hence, social motivation serves as the developmental and evolutionary foundation for complex social behaviors.
The ability to interpret others’ intentions and mental states heavily governs social interactions in any social context. Emotional comprehension, like evaluating social motivation, recognizing body language and facial cues, as well as interpreting implicit and explicit biases of others, are essential to any social interaction. This social cognition process, termed “theory of mind” (44, 45), heavily influences individual social decision-making (46).
Social interactions require effective group dynamics, allowing individuals to develop healthy and essential group relationships (47). Hierarchical, territorial, cooperative, and interdependent social behavior are observed in multiple species. Studies have highlighted the role of social hierarchy in individual well-being, leading to better availability of resources essential to survival, such as food, space, and mating partners (48, 49). Investing in a territorial or hierarchical structure is also an essential decision-making process in which individuals gauge their metabolic energy before involving themselves in conflicts related to group social structure (48). Moreover, the social context of a conflict weighs heavily on an individual’s role in the group dynamics, with an effective change in this role relying on a correct decision-making process.
Social decision-making involves multi-faceted processes, Thus, multiple malfunctions can lead to the atypical social behavior characterizing multiple neuropsychiatric disorders, such as autism spectrum disorder (ASD). Impaired recognition of familiar faces or reduced motivation for social interactions have been reported in ASD (7, 50). Indeed, infants lacking social interest are likely to develop social cognition deficits (51), such as the impaired theory of mind (52, 53). Maladaptive social decision-making capabilities are prevalent in ASD and serve as predictors of overall mortality due to the effects of poor interpersonal relationships on mental and physical health (54). Neuroimaging studies subsequently revealed the involvement of many interconnected brain regions during social decision-making (55). Assigning the process to functionally relevant brain entities is critical for explaining their roles in the atypical behaviors exhibited by individuals diagnosed with ASD.
The PFC has been linked to various aspects of cognition and behavior, such as working memory, decision-making, goal-directed conduct, and social behavior (32, 56, 57). The PFC presents significant yet variable connections to both cortical and sub-cortical areas of the brain, including the hippocampus, amygdala, hypothalamus, and nucleus accumbens, as well as areas associated with sensory-motor functions (18, 58). Many of these areas were shown to be involved in social decision-making (59, 60). Thus, the PFC contributes to all aspects of social interactions, in collaboration with other cortical and sub-cortical regions.
Various regions of the PFC also process distinct aspects of social information (57, 61). Regions that process social motivation play inherent roles in reward, valence, and affiliation and include the orbitofrontal and perigenual anterior cingulate cortices (ventro-medial prefrontal cortex; vmPFC: BA 10,11,12, 25, and 32; orbito-frontal cortex; OFC: BA 10 and 11; and anterior cingulate cortex; ACC: BA 25 and 32) (61). Multiple studies have reported a role for the vmPFC in social motivation and reward. Humans with vmPFC lesions exhibit impairments in emotional recognition and making moral decisions (62). They also failed to learn from recent reward history in a pro-social game (63). Other studies concluded that the OFC plays a role in decision-making based on the valence of the stimuli (64, 65). Additionally, the vmPFC is active when subjects feel socially accepted and comprehend rewarding social cues (66). Interestingly, specific impairments in the tendency of ASD patients to find social stimuli incentivizing or motivating are similar to those seen in humans with vmPFC lesions (67).
Social interactions that necessitate knowledge of oneself and others are consistently associated with activation within the PFC (specifically, the medial and dorso-medial prefrontal cortex; dmPFC). The mPFC is effectively activated while comprehending self-bias and those of others (in line with the theory of mind), beliefs, moral decisions, and emotional states while empathizing with others’ pain and during cooperation [(68, 4);]. Functional magnetic resonance imaging (fMRI) studies showed this region to be active during cooperative tasks among humans, tasks in which ASD patients perform poorly due to lower attention to social cues (69–71). Evidence of decreased activity and connectivity in the mPFC of ASD patients has been reported and are likely to significantly contribute to the social and behavioral deficits presented by these individuals. Studies also demonstrated that ASD patients lack adaptive control in comprehending and adapting their behavior according to an unfair social context or their partner’s emotional expressions (72, 73). Moreover, the infant mPFC is responsive to social cues, like a parent’s face and gaze (74). Furthermore, in contrast to patients who sustained damage to their mPFC as adults, patients who sustained damage to this region as children demonstrate anti-social behavior and poor moral decision-making in adulthood (62). Together, these studies point to the mPFC as serving a crucial role in the forming of proper social cognition in humans from early development stages.
There have been attempts to define the role of distinct PFC sub-regions in separating internal from external social reasoning. The mPFC has been reported to be involved in tasks that involve processing of internal states of self and others, such as empathy, self-reflection, and vicarious moral reasoning (75–77). In contrast, the lateral PFC (lPFC) is part of a network activated by externally guided information processing in the social domain, such as imitation, abstract social reasoning, and internal conflict resolution (78, 79). In addition to ASD, there is strong evidence that patients with other neuropsychiatric disorders, like schizophrenia (SCZ), display hypo-activity in the dorsal lPFC during social interactions (80, 81). Recent works using transcranial direct current stimulation with SCZ and ASD patients described improved social and emotional behavior (82, 83). Yet, despite the apparent improvement in patient behavior following treatment, there was a lack of mechanistic links and specific definitions of such interventions for comorbidities like depression and anxiety. Thus, the particular sections of the PFC that implicitly and explicitly affect individual emotional comprehension remain elusive, although solid evidence points toward the mPFC and lPFC.
The third aspect of social interaction, group dynamics, combines social motivation and emotional comprehension of the social context. fMRI studies found neural correlates of social hierarchy and group dynamics to occur in the PFC (84, 85), as well as in sub-cortical regions, like the amygdala and ventral striatum, that demarcate distress from rewarding social experiences (86, 87). lPFC bias to the superior as opposed to the inferior player in a monetary reward task was only observed in a social context, i.e., with other players, implying that involvement of the lPFC in processing hierarchical information is specifically social in nature (88, 89). Patients with dorsal and lateral PFC lesions do not understand changes in social hierarchy and fail to learn them (67, 90). Thus, activity in the PFC and sub-cortical regions coordinates proper behavioral responses when the social hierarchy is changing, with such knowledge having to be constantly updated in these regions.
In summary, the PFC and its connections to sub-cortical brain regions regulate and encode various aspects of human social interactions (Figure 1). PFC sub-divisions contribute to social motivation, reward, cooperation, and mentalizing of self and others’ socio-emotional states. In the following sections, we compare the above evidence supporting the role for the PFC in human social interactions with what occurs in rodents.
Figure 1. Anatomy of prefrontal cortex in human and mouse the human prefrontal cortex (A) includes Broca areas (BA) 6, 8, 9, and 24 (comprising the anterior cingulate, AC), 10, 11, 12, 25, and 32. The colored regions define PFC sub-divisions in humans and the corresponding homologous regions in mice (91–93) (B).
Non-human primates (NHPs) present rich social behaviors, such that studies on these models may directly inform on clinical interventions for neuropsychiatric disorders such as ASD. Relevant studies are, however, restricted by small sample size, lack of effective circuit-specific manipulation tools, and the general difficulty and slowness of experimentation. Furthermore, limitations in specific genetic lines that mimic mutations found in ASD patients hinder efforts aimed at mechanistic understanding of modifications in NHP social interactions. At the same time, rodent models represent effective and valuable systems for addressing specific questions regarding biological mechanisms and brain circuits involved in social behavior and their alterations by ASD-associated genetic mutations.
From rodents to primates and humans, social interactions and their underlying neural processes have been remarkably conserved. Nevertheless, the neurobiological mechanisms and brain circuits contributing to rodent social interactions remain elusive and have been only partially explained to date. The following section highlights the anatomical correlates of rodent social interactions that align with the human PFC.
Historically, anatomical similarities between the human and rodent PFC gave rise to multiple controversies (91, 93–96). Studies of functional correlates indicated the rodent PFC as being involved in non-social behavior, like working memory (97), impulse control (98), attention, and goal-directed behavior (99, 100). The rodent prelimbic cortex (Figure 1) seems homologous to human BA 32 that is part of the dorsal and ventral PFC, including the lateral PFC (96, 101). At the same time, the rodent infralimbic cortex is considered to be homologous to BA 25, a part of the ventromedial PFC in humans. The rodent medial OFC and ACC share homologies with the human OFC and dorsomedial PFC, respectively. The granular cortical structure of the rodent PFC does not entirely match its human counterpart [(see 93) for detailed comparisons]. Unlike the human PFC, the rodent PFC receives and projects extensively to other cortical and sub-cortical brain regions, specifically, limbic and midline thalamic regions that densely innervate the PFC.
Previous efforts indicated the existence of a dichotomy between sub-regions of the rodent PFC in processing social interactions (102, 103). Because of this, it is crucial to employ behavioral paradigms that are ethologically appropriate and take advantage of typical rodent actions that are involved in social interactions. In the following section, we discuss how the rodent PFC regulates social interactions in ethologically relevant tasks of social behavior and how specific pre-clinical models of ASD may highlight the role of the PFC in such pathologies. We also review the extensive literature on rodent PFC involvement in numerous social behavior tasks by concentrating on three distinct aspects of social interactions and on studies that specifically explore these aspects.
Multiple tasks have been developed to gauge rodent social motivation (31). It should be noted that the parameters quantified in these tasks, such as the time spent near social stimuli, reflect traits that are vastly different from those humans employ during social interactions (104, 105). Furthermore, rodents predominantly utilize the olfactory sensory system during social interactions (106), in contrast to predominant dependence of human social interactions on visual and auditory cues (107).
Multiple tasks have been developed to assess the recognition of conspecifics (social recognition) and the motivation to orient and approach them (see (32) for a detailed list of behavior tasks used to test rodents). The earliest social recognition task, the social habituation/dishabituation test, relied on a series of encounters with the same conspecific (social stimulus) and finally, with an unfamiliar one (108). Such assays reveal that in general, subjects gradually lose the motivation to interact when encountering the same (familiar) social stimulus in subsequent trials (the habituation phase), indicative of recognition of the familiar stimulus. A subject’s interaction time returns to the level of the first trial when exposed to an unfamiliar stimulus (dishabituation), thus controlling for changes in general social motivation. This task effectively reports on short-term and long-term memory in rodents, despite exposing confounds of internal state and novelty that cannot be controlled (109).
Social discrimination tasks were devised to probe the motivation to interact with specific stimuli while using appropriate controls that account for the novelty of a stimulus. For example, the social novelty preference task considers the time spent investigating (i.e., sniffing) a novel stimulus as opposed to a familiar cage-mate or a recently encountered conspecific to control for aggression due to male pheromones and general social motivation (110). These discrimination tasks provide information on different behavioral dynamics (111), which cannot be analyzed in the habituation/dishabituation task. Another variation of social recognition task specifically designed for a monogamous species of voles is the partner preference test. These monogamous rodents preferably interact with their partner after pair-bonding, relative to a stranger (112).
Some of the earliest proof of emotional cognition appears in works where rats (i.e., observers), trained to receive food rewards in lever press tasks, reduced the amount of lever pressing as they observed another rat (i.e., a demonstrator) being exposed to foot shocks. The study reflected the transmission of emotional state between observer and demonstrator rats (113). Similarly, mice and rats demonstrated the social transmission of pain and analgesia (114, 115), fear (116, 117) (Figure 2A), and food preference (118).
Figure 2. Tasks to test affective emotional, empathic and group dynamics in rodents. (A) Social transfer of fear in a rodent observing a demonstrator in pain due to acetic acid injection. (B) Social transfer of fear in a rodent observing a demonstrator experiencing foot-shock-induced pain. (C) Affective emotional state preference of conspecifics experiencing positive emotions, such as relief from thirst or (D) negative emotions, such as stress due to being restrained for a while, over neutral conspecifics. (E) Pro-social empathic behavior of rats freeing a captive conspecific. (F) Semi-natural social box for studying rodent group dynamics.
Several tasks indicate that rodents display emotions, specifically fear, and thus enable social transmission of emotional information. Rodents, moreover, respond to emotional states of other individuals. In transfer learning procedures, such as fear conditioning by proxy, a rat exposed to a novel tone while in the presence of a cage-mate who was previously fear-conditioned to that tone will freeze (119) (Figure 2B). In another procedure, known as social harm aversion, rats avoid a specific task (like lever pressing) if it causes harm to others (120). This behavior is affected by the outcome. For instance, positive outcome behavior occurs more often than does a decrease in negative outcome-related behavior (121).
Recent studies have tested the capability of rodents to recognize and discriminate emotional states of conspecifics (122). In the positive mode of a relevant task, which uses the setup of social discrimination tasks, one of two presented social stimuli is associated with deprivation of water in the home cage for the preceding 23 h and a quenching of thirst for an hour before the experiment. This manipulation of water availability in the home cage induces a “relieved” state in the social stimulus, drawing more attention from the subject than a control stimulus, which remains in neutral conditions (Figure 2C). On the other hand, the negative mode of this test probes discrimination of a negative emotional state, induced in a social stimulus by foot shocks or a short period in a restrainer, as compared to a neutral stimulus. In both positive and negative conditions, the subject mouse prefers interacting more with the arousing stimuli.
An behavioral task in which rats persistently try to free a captive conspecific, despite the temptation to instead consume a highly palatable food presented in the same arena, demonstrates empathy in these animals (123) (Figure 2D). Food-sharing tasks also reveal rats to be pro-social and empathic toward cage-mates. For example, Norway rats shared more palatable food with a partner who provided them with a piece of banana than with a partner who provided a less preferred piece of carrot (124). Rats also displayed pro-social behavior by providing food rewards to their cage-mates, even when they did not benefit from the decision to share food (125). In a consolation test of monogamous voles that quantifies the amount of allogrooming of a familiar, as compared to a stranger demonstrator, when the demonstrator was exposed to mild foot-shocks as stress, these rodents performed allogrooming of their stressed familiar partners so as to reduce their stress. The test thus differentiates empathic responses of a vicarious nature from general stress-coping behavior (126). In summary, these studies open ample avenues to study neural mechanisms of emotional recognition and empathy in rodents.
Social hierarchies emerge in mice when they live in densely populated conditions, where competition for territory, housing, mates, and food plays an essential role in the survival of the individual. Introducing pairs of cage-mates from opposing ends of a tube that does not allow sufficient space for a mouse to turn around or for both mice to pass each other offers one way to measure social dominance (127). Alternatively, semi-natural home cages (Figure 2E) that mimic large mouse colonies have been used to study dominance and hierarchical behavior (128, 129). Affective cooperation and altruistic behavior, investigated in rodents using lever pressing tasks, were shown to be influenced by the hierarchal stature of an animal in the group (130).
Animal models support literature implicating the human PFC in social motivation, in conjunction with sub-cortical areas, such as the nucleus accumbens (NAc) and ventral tegmental area (VTA), which mediate the rewarding aspects of social interaction (131, 132). Although lesion studies have provided evidence for the crucial role of the PFC in social motivation (133, 134), such non-specific manipulation may damage nearby regions and axonal projections around the lesioned areas. Still, a comprehensive study examining murine whole-brain c-Fos expression in a social context revealed that social interaction strongly activates the mouse PFC (135).
PFC circuitry is precisely arranged, presenting an array of interneurons that inhibit circuit activity, as well as neuromodulator inputs that rely on acetylcholine, dopamine and oxytocin. In mice, PFC circuitry is characterized by the canonical flow of excitation between cortical layers (Figure 3A), such as thalamo-recipient pyramidal neurons in layer 3 which send excitatory inputs to layer 2 pyramidal neurons. These layer 2 cells descend in turn to layer 5 pyramidal neurons (136). GABAergic interneurons (i.e., parvalbumin (PV+) and somatostatin (SST+) neurons) strongly control the excitatory drive of long-range and local intercortical-projecting pyramidal neurons in the PFC. These PFC interneurons display remarkable selectivity for connections with pyramidal neurons. In superficial layers, the PV+ and SST+ cells preferentially target layer 2 cortico-amygdalar and cortico-striatal pyramidal neurons (137, 138), whereas deeper in the cortex, the interneurons synapse solely with pyramidal neurons that target other pyramidal neurons (136, 139, 140). Many studies of pre-clinical animal models of ASD have reported decreased inhibitory neurotransmission in the PFC (141, 142), leading to low sociability, vocalization, and reciprocal social interactions (143). Excitatory/inhibitory (E/I) balance changes during development are linked to a critical period of plasticity in the PFC (144, 145). Post-mortem studies in ASD patients (146) extensively indicate reduced GABA receptors expression (147–149), increased Glutamatergic receptors expression (150, 151), and a low number of PV+ neurons in prefrontal cortex (152) which could result in the E/I imbalance. ASD patients show decreased gamma oscillation power, indicative of fast-spiking neurons firing at lower rates (153, 154). Studies in ASD patients showed higher numbers of dendritic spines, overall increased within-region connectivity, and a reduction in long-range connections of the PFC (155–157). Moreover, fMRI studies reported hypoactivation of the ACC to social reward in ASD compared to typically developing controls (158, 159), which indicate that these patient process socially rewarding and motivating cues abnormally (160). Therefore, investigating how alterations in the PFC circuitry affect social motivation and behavior may be essential for exposing the underlying mechanism of social deficits seen in ASD (161). Below, we further review the evidence that modified PFC circuitry interferes with social motivation.
Figure 3. Prefrontal circuit’s specific components and their role in social interaction. (A) PFC circuitry and neuronal cell types driving inter and intra cortical excitatory drive. Specifically, the interneurons PV+ and SST+ inhibitory control over layer 2 (L2) as well as layer 5 (L5) pyramidal neurons. (B) Pyramidal neurons firing rate in PFC is modulated by PV+ and SST+ inhibition to the cell body and apical dendrites, respectively. Higher firing rate of the pyramidal neurons correspond to increased social motivation in mice. While the SST+ neurons are reported to be modulated through oxytocin and corticotrophin releasing hormone, specifically during social motivation and novelty preference behavior in rodents. While cholinergic projections into pyramidal neurons regulate social motivation and memory through nicotinic acetylcholine receptors. Further, low NMDA NR1 in PV+ neurons reduce social investigation. Excitatory synapses are specifically affected by structural protein Shank3 deficiency along with disruption actin formation, which cause low social motivation. Similarly, reduced excitatory post-synaptic currents due to low NMDA and AMPA receptors cause significant imbalance in prefrontal circuit E/I imbalance.
Direct intervention in the E/I balance within the PFC circuitry profoundly affects the social motivation of adult mice. In seminal work, researchers optogenetically manipulated the neural activity of specific PFC neuronal populations during reciprocal interaction with juvenile conspecifics and in the three-chamber sociability task (162). Increasing excitatory activity by stimulating pyramidal neurons disrupted social exploration in the unrestricted interaction test and social preference in the three-chamber test. These deficits were brought down by activating inhibitory PV+ interneurons simultaneously with pyramidal cells, emphasizing the crucial role of an appropriate E/I ratio in the PFC for proper social motivation in mice.
Multiple synaptic or circuit-level factors establish and tightly regulate neuronal E/I balance (163). The balance between excitatory and inhibitory synapses in the brain is maintained through a complex interplay of several factors. These include the development and functioning of these synapses and the signaling pathways and mechanisms that regulate their plasticity. Homeostatic synaptic plasticity and intrinsic neuronal excitability also play roles in this delicate balance (164). At a higher level, E/I balance is regulated by the activity of different circuits, such as local circuits that involve distinct types of interneurons. These interneurons play a crucial role in regulating the activity of pyramidal neurons and modulating long-range connections (165, 166).
In the context of genetic risk factors for ASD, multiple studies have examined the E/I balance and its disruption in the PFC. Malfunctions of alpha-amino-3-hydroxy-5-methyl-4-isoxazole propionic acid (AMPA), N-methyl-D-aspartate (NMDA), and metabotropic glutamate receptors were found to affect the E/I balance in parallel to social behavior. For instance, Gandal et al. (167) showed that mice expressing low levels of the NMDA receptor NR1 subunit in the PFC display low social motivation, decreased ultrasonic vocalizations, and abnormal gamma synchrony. Studies using genetic pre-clinical models linked reduced interneuronal markers in pre-frontal regions to imbalances in the E/I ratio due to a low level or lack of inhibitory control of pyramidal neuron excitability (168, 169). The maladaptive developmental trajectory of inhibitory interneurons and their role in later dysfunction of the PFC circuit have been widely studied (152, 170–172). While the impact of these deficits is global and affects multiple nodes of the social decision-making network that involves social motivation, the PFC is particularly susceptible. For instance, Shank3-deficient mice have been shown to lack social motivation and exhibit specific deficits in PFC circuitry, such as reduced NMDA-based excitatory post-synaptic currents (EPSCs) and a low number of F-actin filaments. These were rescued upon depolymerization of the actin filaments following systemic or focal treatment (173). Recent work involving circuit-specific mutation of Shank3 in PFC-to-basolateral amygdala-projecting neurons recapitulated social motivation deficits and synaptic hypoactivity (174). In addition, chemogenetic activation of pyramidal neurons in the PFC of these mice rescued social interactions in the three-chamber task, as well as NMDA receptor-dependent EPSCs (175). Thus, PFC circuit dysfunction, especially of excitatory neurons projecting to the amygdala, directs social motivation deficits, at least in Shank3-deficient mice. However, mutations of the NMDA receptor NR1 subunit in the PFC and hippocampus of adult mice did not decrease social novelty preference and sociability in the three-chamber task (176). Taken together, the development and early childhood susceptibility of interneurons may play a significant role in the PFC circuit and E/I balance abnormalities (Figure 3B) seen in ASD models (177, 178).
In addition to excitatory glutamatergic and inhibitory GABAergic activity, many neuromodulators alter PFC activity. Specific lesions of cholinergic projections into the PFC reduced rat social interactions in an open field arena (179). Distinct cholinergic inputs from the basal forebrain seemed to regulate different aspects of social interactions, namely social motivation and memory (180). Moreover, cholinergic signaling through nicotinic receptors in the PFC promoted the exploration of novel social stimuli (133). Oxytocin increased pair bonding and pro-social behavior (181–183) through contributions from sub-cortical regions and perhaps via their projections to the PFC (39). Social recognition memory is regulated by oxytocin-mediated modulation of prefrontal cortex plasticity, which is impaired when juvenile rats eat a high-fat diet (184). Moreover, oxytocin receptor (OTr)-expressing SST+ neurons in the murine PFC present sex-specific responses to oxytocin (185). These neurons regulate female motivation to interact with males during the estrus phase, yet do not affect interactions with other females. In another study, chronic activation of pyramidal neurons of rat PFC reduced social motivation to interact with novel stimuli in a three-chamber task (186). These motivation deficits were ameliorated by systemic OTr agonist injections. Recently, Riad et al. (187) showed that corticotrophin-releasing hormone (CRH)-expressing neurons inhibit OTr-positive neurons and layer 2/3 in the mPFC when stimulated in vitro at low frequency. When activated chemo-genetically, these CRH neurons increase novelty preference in male but not female mice. Moreover, a recent study showed that PFC infralimbic CRH+ neurons that project to the lateral septum modulate social novelty preference (188). In summary, more detailed studies on the effects of PFC neuromodulators are required to reveal the intricate mechanisms through which they modulate E/I balance and circuitry in this brain region and regulate its activity during specific social behaviors (Figure 3B).
Social recognition and memory of socially relevant events are essential to social interactions. Early social recognition is impaired in children with ASD (189, 190). A study in rats reported that lesions in the ACC reduced social recognition, while OFC lesions did not affect this behavior (134). Activation of pyramidal neurons in Cntnap2 knockout mice [corresponding to a pre-clinical model of cortical dysplasia focal epilepsy syndrome, a type of ASD (191)] balanced the E/I ratio and alleviated deficits in social recognition of novel juveniles (192). Mice that lack Fgf17, a signaling molecule essential for rostral forebrain development (193), show difficulties in social recognition and low c-Fos activity in the PFC during exploration of opposite sex conspecifics (194).
As discussed above, NMDA receptor hypo-function is a characteristic feature of many ASD mouse models. These deficits in glutamatergic synaptic activity also cause a loss of social recognition and memory. Moreover, acute systemic administration of the NMDA receptor antagonist MK801 reduces recognition of novel juvenile stimulus (195). Specifically, mice with NR1 subunit-deficient GABAergic neurons in the PFC do not distinguish a novel stimulus over a familiar one in a short-term social memory test (196). Collectively, PFC NMDA receptor synaptic activity contributes to social recognition and memory.
Works on empathy behavior in rats, which preferred to rescue a restrained conspecific over getting more food rewards, indicated a role of ACC projections to the Nac shell in regulating such behavior (197). Works from the Hong group (198) showed that dmPFC neuronal activity is related to the sex of the conspecific during social exploration. Furthermore, recent works exploring recognition of emotionally affected conspecifics indicated multi-faceted regulation by the PFC (122, 199). In addition, in mice fear-conditioned to avoid specific social stimuli, SST+ neurons inhibited PV+ neurons in the mPFC, thus causing disinhibition of excitatory projections from the region. These results suggest that the PFC regulates social fear conditioning or affective avoidance by increasing the excitatory drive in the circuit (200).
We are rapidly enhancing our understanding of the neural mechanisms underlying social interactions. Here, we considered an ever-growing body of evidence showing that the prefrontal cortex is a hub in this process. Social interactions involve multiple processes, like decision-making, valence, and perception of the emotions of self and others. It is thus no wonder that such high-order and complex social behavior is affected by disorders like ASD and other neuropsychological comorbidities. We accordingly addressed evidence that the prefrontal circuitry is susceptible to synaptic, cellular, and molecular modifications in ASD. Such modifications bring about a myriad of social deficits, despite the majority of the current literature only reporting on deficits in sociability, social recognition, and vocalization. We suggest that studying social deficits through tasks that address affective emotions, empathic behavior, and even group dynamics will enrich our understanding of the causes of ASD in rodent models. Taken together with studies of the mechanisms and roles of various neuromodulators and transmitters in the PFC during social interactions, such explorations can better guide interventions of clinical value.
AM and SW: conceptualization and writing—review and editing. SW: funding, resources, and supervision. AM: writing—original draft. All authors contributed to the article and approved the submitted version.
This study was supported by ISF-NSFC joint research program (grant No. 3459/20 to SW), the Israel Science Foundation (grants No. 1361/17 and 2220/22 to SW), the Ministry of Science, Technology and Space of Israel (Grant No. 3-12068 to SW), the Ministry of Health of Israel (ERA-NET 2022 grant No. 3-18380), and the United States-Israel Binational Science Foundation (grant No. 2019186 to SW).
The authors thank Shai Netser and Renad Jabarin for their help in gathering literature for this review article.
The authors declare that the research was conducted in the absence of any commercial or financial relationships that could be construed as a potential conflict of interest.
All claims expressed in this article are solely those of the authors and do not necessarily represent those of their affiliated organizations, or those of the publisher, the editors and the reviewers. Any product that may be evaluated in this article, or claim that may be made by its manufacturer, is not guaranteed or endorsed by the publisher.
1. Adolphs, R, Tranel, D, and Damasio, AR. Neural systems subserving emotion; lesion studies of the amygdala, somatosensory cortices, and ventromedial prefrontal cortices In: Handbook of neuropsychology. (eds Gainotti, G.). 2nd Ed., Elsevier Science Amsterdam: (2001). 89–110.
2. Kieckhaefer, C, Schilbach, L, and Bzdok, D. Social belonging: brain structure and function is linked to membership in sports teams, religious groups, and social clubs. Cereb Cortex. (2023) 33:4405–20. doi: 10.1093/cercor/bhac351
3. Millan, MJ, and Bales, KL. Towards improved animal models for evaluating social cognition and its disruption in schizophrenia: the CNTRICS initiative. Neurosci Biobehav Rev. (2013) 37:2166–80. doi: 10.1016/j.neubiorev.2013.09.012
4. Amodio, DM, and Frith, CD. Meeting of minds: the medial frontal cortex and social cognition. Nat Rev Neurosci. (2006) 7:268–77. doi: 10.1038/nrn1884
5. Bickart, KC, Hollenbeck, MC, Barrett, LF, and Dickerson, BC. Intrinsic amygdala–cortical functional connectivity predicts social network size in humans. J Neurosci. (2012) 32:14729–41. doi: 10.1523/JNEUROSCI.1599-12.2012
6. Nieves, N, and von Schroder, C. Roles of medial prefrontal cortex activity in human and animal social learning. In: Vonk, J., Shackelford, T.K. (eds) Encycl Anim Cogn Behav. Springer Cham. (2022):6113–20. doi: 10.1007/978-3-319-55065-7_1298
7. Dubey, I, Ropar, D, and Hamilton, AF. Measuring the value of social engagement in adults with and without autism. Mol Autism. (2015) 6:31. doi: 10.1186/s13229-015-0031-2
8. Morrison, KE, DeBrabander, KM, Jones, DR, Ackerman, RA, and Sasson, NJ. Social cognition, social skill, and social motivation minimally predict social interaction outcomes for autistic and non-autistic adults. Front Psychol. (2020) 11:591100. doi: 10.3389/fpsyg.2020.591100
9. Assaf, M, Jagannathan, K, Calhoun, VD, Miller, L, Stevens, MC, Sahl, R, et al. Abnormal functional connectivity of default mode sub-networks in autism Spectrum disorder patients. NeuroImage. (2010) 53:247–56. doi: 10.1016/j.neuroimage.2010.05.067
10. Sumiya, M, Okamoto, Y, Koike, T, Tanigawa, T, Okazawa, H, Kosaka, H, et al. Attenuated activation of the anterior rostral medial prefrontal cortex on self-relevant social reward processing in individuals with autism Spectrum disorder. NeuroImage Clin. (2020) 26:102249. doi: 10.1016/j.nicl.2020.102249
11. Franklin, TB, Silva, BA, Perova, Z, Marrone, L, Masferrer, ME, Zhan, Y, et al. Prefrontal cortical control of a brainstem social behavior circuit. Nat Neurosci. (2017) 20:260–70. doi: 10.1038/nn.4470
12. Frost, NA, Haggart, A, and Sohal, VS. Dynamic patterns of correlated activity in the prefrontal cortex encode information about social behavior. PLoS Biol. (2021) 19:e3001235. doi: 10.1371/journal.pbio.3001235
13. Lee, E, Rhim, I, Lee, JW, Ghim, J-W, Lee, S, Kim, E, et al. Enhanced neuronal activity in the medial prefrontal cortex during social approach behavior. J Neurosci. (2016) 36:6926–36. doi: 10.1523/JNEUROSCI.0307-16.2016
14. Sakamoto, T, and Yashima, J. Prefrontal cortex is necessary for Long-term social recognition memory in mice. Behav Brain Res. (2022) 435:114051. doi: 10.1016/j.bbr.2022.114051
15. Haber, SN, Liu, H, Seidlitz, J, and Bullmore, E. Prefrontal Connectomics: from anatomy to human imaging. Neuropsychopharmacology. (2022) 47:20–40. doi: 10.1038/s41386-021-01156-6
16. Ko, J. Neuroanatomical substrates of rodent social behavior: the medial prefrontal cortex and its projection patterns. Front Neural Circuits. (2017) 11:41. doi: 10.3389/fncir.2017.00041
17. Park, G, Ryu, C, Kim, S, Jeong, SJ, Koo, JW, Lee, Y-S, et al. Social isolation impairs the prefrontal-nucleus Accumbens circuit subserving social recognition in mice. Cell Rep. (2021) 35:109104. doi: 10.1016/j.celrep.2021.109104
18. Gangopadhyay, P, Chawla, M, Dal Monte, O, and Chang, SWC. Prefrontal–amygdala circuits in social decision-making. Nat Neurosci. (2021) 24:5–18. doi: 10.1038/s41593-020-00738-9
19. Padilla-Coreano, N, Batra, K, Patarino, M, Chen, Z, Rock, RR, Zhang, R, et al. Cortical ensembles orchestrate social competition through hypothalamic outputs. Nature. (2022) 603:667–71. doi: 10.1038/s41586-022-04507-5
20. Phillips, ML, Robinson, HA, and Pozzo-Miller, L. Ventral hippocampal projections to the medial prefrontal cortex regulate social memory. elife. (2019) 8:e44182. doi: 10.7554/eLife.44182
21. Zhang, H, Chaudhury, D, Ma, SM, Montgomery, S, Cao, J-L, and Han, M-H. A key noradrenergic brainstem-mesolimbic circuit: resilience to social stress. Chronic Stress. (2019) 3:247054701985018. doi: 10.1177/2470547019850186
22. Tan, Y, Singhal, SM, Harden, SW, Cahill, KM, Nguyen, D-TM, Colon-Perez, LM, et al. Oxytocin receptors are expressed by glutamatergic prefrontal cortical neurons that selectively modulate social recognition. J Neurosci. (2019) 39:3249–63. doi: 10.1523/JNEUROSCI.2944-18.2019
23. He, L-N, Chen, S, Yang, Q, Wu, Z, Lao, Z-K, Tang, C-F, et al. EphB2-dependent prefrontal cortex activation promotes Long-range social approach and partner responsiveness. Proc Natl Acad Sci. (2023) 120:e2219952120. doi: 10.1073/pnas.2219952120
24. Takahashi, A, Nagayasu, K, Nishitani, N, Kaneko, S, and Koide, T. Control of Intermale aggression by medial prefrontal cortex activation in the mouse. PLoS One. (2014) 9:e94657. doi: 10.1371/journal.pone.0094657
25. Zhang, Z, Zhang, Y, Yuwen, T, Huo, J, Zheng, E, Zhang, W, et al. Hyper-excitability of Corticothalamic PT neurons in MPFC promotes irritability in the mouse model of Alzheimer’s disease. Cell Rep. (2022) 41:111577. doi: 10.1016/j.celrep.2022.111577
26. Williamson, CM, Lee, W, Romeo, RD, and Curley, JP. Social context-dependent relationships between mouse dominance rank and plasma hormone levels. Physiol Behav. (2017) 171:110–9. doi: 10.1016/j.physbeh.2016.12.038
27. du, R, Luo, W-J, Geng, K-W, Li, C-L, Yu, Y, Wei, N, et al. Empathic contagious pain and consolation in laboratory rodents: species and sex comparisons. Neurosci Bull. (2020) 36:649–53. doi: 10.1007/s12264-020-00465-y
28. Lee, W, Khan, A, and Curley, JP. Major urinary protein levels are associated with social status and context in mouse social hierarchies. Proc R Soc B Biol Sci. (2017) 284:20171570. doi: 10.1098/rspb.2017.1570
29. Clipperton-Allen, AE, and Page, DT. Social behavior testing in mice: social interest, recognition, and aggression. Neuromethods. (2023) 190:14. doi: 10.1007/978-1-0716-2748-8_14
30. Clipperton-Allen, AE, and Page, DT. Social behavior testing in mice: social interest, recognition, and aggression In: Psychiatric vulnerability, mood, and anxiety disorders: eds. J. Harro Neuromethods, Humana, New York: NY (2023). 190:279–307.
31. Jabarin, R, Netser, S, and Wagner, S. Beyond the three-chamber test: toward a multimodal and objective assessment of social behavior in rodents. Mol Autism. (2022) 13:41. doi: 10.1186/s13229-022-00521-6
32. Kietzman, HW, and Gourley, SL. How social information impacts action in rodents and humans: the role of the prefrontal cortex and its connections. Neurosci Biobehav Rev. (2023) 147:105075. doi: 10.1016/j.neubiorev.2023.105075
33. Adhikari, A, Topiwala, MA, and Gordon, JA. Article single units in the medial prefrontal cortex with anxiety-related firing patterns are preferentially influenced by ventral hippocampal activity. Neuron. (2011) 71:898–910. doi: 10.1016/j.neuron.2011.07.027
34. Bicks, LK, Koike, H, Akbarian, S, and Morishita, H. Prefrontal cortex and social cognition in mouse and man. Front Psychol. (2015) 6:1805. doi: 10.3389/fpsyg.2015.01805
35. Kohls, G, Chevallier, C, Troiani, V, and Schultz, RT. Social ‘wanting’ dysfunction in autism: neurobiological underpinnings and treatment implications. J Neurodev Disord. (2012). doi: 10.1186/1866-1955-4-10
36. Mitchell, JP, Neil Macrae, C, and Banaji, MR. Dissociable medial prefrontal contributions to judgments of similar and dissimilar others. Neuron. (2006) 50:40. doi: 10.1016/j.neuron.2006.03.040
37. Rees, G, Kreiman, G, and Koch, C. Neural correlates of. Brain. (2002) 3:1–10. doi: 10.1093/brain/awf181
38. Green, MF, Horan, WP, and Lee, J. Social cognition in schizophrenia. Nat Rev Neurosci. (2015) 16:620–31. doi: 10.1038/nrn4005
39. Insel, TR, and Young, LJ. Neuropeptides and the evolution of social behavior. Curr Opin Neurobiol. (2000) 10:784–9. doi: 10.1016/S0959-4388(00)00146-X
40. Carver, LJ, and Dawson, G. Development and neural bases of face recognition in autism. Mol Psychiatry. (2002) 7:1168. doi: 10.1038/sj.mp.4001168
41. Nelson, CA. The development and neural bases of face recognition. Infant Child Dev. (2001) 10:1–2. doi: 10.1002/icd.239
42. Sakuta, Y, Kanazawa, S, and Yamaguchi, MK. Infants prefer a trustworthy person: an early sign of social cognition in infants. PLoS One. (2018) 13:3541. doi: 10.1371/journal.pone.0203541
43. Southgate, V. Are infants Altercentric? The other and the self in early social cognition. Psychol Rev. (2020) 127:182. doi: 10.1037/rev0000182
44. Mier, D, Lis, S, Neuthe, K, Sauer, C, Esslinger, C, Gallhofer, B, et al. The involvement of emotion recognition in affective theory of mind. Psychophysiology. (2010) 47:1031. doi: 10.1111/j.1469-8986.2010.01031.x
45. Mitchell, RLC, and Phillips, LH. The overlapping relationship between emotion perception and theory of mind. Neuropsychologia. (2015) 70:1–10. doi: 10.1016/j.neuropsychologia.2015.02.018
46. Kumaran, D, Banino, A, Blundell, C, Hassabis, D, and Dayan, P. Computations underlying social hierarchy learning: distinct neural mechanisms for updating and representing self-relevant information. Neuron. (2016) 92:52. doi: 10.1016/j.neuron.2016.10.052
47. Wang, F, Kessels, HW, and Hailan, H. The mouse that roared: neural mechanisms of social hierarchy. Trends Neurosci. (2014) 37:674–82. doi: 10.1016/j.tins.2014.07.005
48. Qu, C, Ligneul, R, Van, JB, Henst, D, and Dreher, JC. An integrative interdisciplinary perspective on social dominance hierarchies. Trends Cogn Sci. (2017) 21:893–908. doi: 10.1016/j.tics.2017.08.004
49. Sapolsky, RM. The influence of social hierarchy on primate health. Science. (2005) 308:648–652 doi: 10.1126/science.1106477
50. Dawson, G, Meltzoff, AN, Osterling, J, Rinaldi, J, and Brown, E. Children with autism fail to orient to naturally occurring social stimuli. J Autism Dev Disord. (1998) 28:479–85. doi: 10.1023/A:1026043926488
51. Rosello, B, Berenguer, C, Baixauli, I, García, R, and Miranda, A. Theory of mind profiles in children with autism Spectrum disorder: adaptive/social skills and pragmatic competence. Front Psychol. (2020) 11:7401. doi: 10.3389/fpsyg.2020.567401
52. Brewer, N, Young, RL, and Barnett, E. Measuring theory of mind in adults with autism Spectrum disorder. J Autism Dev Disord. (2017) 47:3080. doi: 10.1007/s10803-017-3080-x
53. Senju, A. Spontaneous theory of mind and its absence in autism Spectrum disorders. Neuroscientist. (2012) 18:108–13. doi: 10.1177/1073858410397208
54. Dempsey, EE, Moore, C, Johnson, SA, Stewart, SH, and Smith, IM. Morality in autism Spectrum disorder: a systematic review. Dev Psychopathol. (2020) 32:3. doi: 10.1017/S0954579419001160
55. Assaf, M, Hyatt, CJ, Wong, CG, Johnson, MR, Schultz, RT, Hendler, T, et al. Mentalizing and motivation neural function during social interactions in autism Spectrum disorders. NeuroImage Clin. (2013) 3:5. doi: 10.1016/j.nicl.2013.09.005
56. Friedman, NP, and Robbins, TW. The role of prefrontal cortex in cognitive control and executive function. Neuropsychopharmacology. (2022) 47:72–89. doi: 10.1038/s41386-021-01132-0
57. Wood, JN, and Grafman, J. Human prefrontal cortex: processing and representational perspectives. Nat Rev Neurosci. (2003) 4:1033. doi: 10.1038/nrn1033
58. Hiser, J, and Koenigs, M. The multifaceted role of the ventromedial prefrontal cortex in emotion, decision making, social cognition, and psychopathology. Biol Psychiatry. (2018) 83:638–47. doi: 10.1016/j.biopsych.2017.10.030
59. Arioli, M, Crespi, C, and Canessa, N. Social cognition through the Lens of cognitive and clinical neuroscience. Biomed Res Int. (2018) 2018:4283427. doi: 10.1155/2018/4283427
60. Martin-Cortecero, J, and Nuñez, A. Sensory responses in the medial prefrontal cortex of anesthetized rats. Implications for sensory processing. Neuroscience. (2016) 339:45. doi: 10.1016/j.neuroscience.2016.09.045
61. Merritt, CC, Maccormack, JK, Stein, AG, Lindquist, KA, and Muscatell, KA. The neural underpinnings of intergroup social cognition: an FMRI Meta-analysis. Soc Cogn Affect Neurosci. (2021) 16:34. doi: 10.1093/scan/nsab034
62. Anderson, SW, Bechara, A, Damasio, H, Tranel, D, and Damasio, AR. Impairment of social and moral behavior related to early damage in human prefrontal cortex. Nat Neurosci. (1999) 2:1032–7. doi: 10.1038/14833
63. Bechara, A, Damasio, H, Tranel, D, and Damasio, AR. The Iowa gambling task and the somatic marker hypothesis: some questions and answers. Trends Cogn Sci. (2005) 9:159–62. doi: 10.1016/j.tics.2005.02.002
64. Camille, N, Tsuchida, A, and Fellows, LK. Double dissociation of stimulus-value and action-value learning in humans with orbitofrontal or anterior cingulate cortex damage. J Neurosci. (2011) 31:2011. doi: 10.1523/JNEUROSCI.3164-11.2011
65. Kennerley, SW, Behrens, TEJ, and Wallis, JD. Double dissociation of value computations in orbitofrontal and anterior cingulate neurons. Nat Neurosci. (2011) 14:2961. doi: 10.1038/nn.2961
66. Grossman, M, Eslinger, PJ, Troiani, V, Anderson, C, Avants, B, Gee, JC, et al. The role of ventral medial prefrontal cortex in social decisions: converging evidence from FMRI and frontotemporal lobar degeneration. Neuropsychologia. (2010) 48:3505–12. doi: 10.1016/j.neuropsychologia.2010.07.036
67. Lieberman, MD, Straccia, MA, Meyer, ML, du, M, and Tan, KM. Social, self,(situational), and affective processes in medial prefrontal cortex (MPFC): causal, multivariate, and reverse inference evidence. Neurosci Biobehav Rev. (2019) 99:311–28. doi: 10.1016/j.neubiorev.2018.12.021
68. Chris, M, and Bird,. The impact of extensive medial frontal lobe damage on ‘Theory of Mind’ and cognition. Brain. (2004) 127:914–928. doi: 10.1093/brain/awh108
69. Bloch, C, Tepest, R, Jording, M, Vogeley, K, and Falter-Wagner, CM. Intrapersonal synchrony analysis reveals a weaker temporal coherence between gaze and gestures in adults with autism Spectrum disorder. Sci Rep. (2022) 12:20417. doi: 10.1038/s41598-022-24605-8
70. Redcay, E, Dodell-Feder, D, Mavros, PL, Kleiner, M, Pearrow, MJ, Triantafyllou, C, et al. Atypical brain activation patterns during a face-to-face joint attention game in adults with autism Spectrum disorder. Hum Brain Mapp. (2013) 34:22086. doi: 10.1002/hbm.22086
71. Rilling, JK, Glenn, AL, Jairam, MR, Pagnoni, G, Goldsmith, DR, Elfenbein, HA, et al. Neural correlates of social cooperation and non-cooperation as a function of psychopathy. Biol Psychiatry. (2007) 61:21. doi: 10.1016/j.biopsych.2006.07.021
72. Edmiston, EK, Merkle, K, and Corbett, BA. Neural and cortisol responses during play with human and Computer Partners in Children with autism. Soc Cogn Affect Neurosci. (2014) 10:159. doi: 10.1093/scan/nsu159
73. Yang, L, Li, P, Mao, H, Wang, H, Shu, C, Bliksted, V, et al. Theory of mind deficits partly mediate impaired social decision-making in schizophrenia. BMC Psychiatry. (2017) 17:1313. doi: 10.1186/s12888-017-1313-3
74. Jessen, S, and Grossmann, T. The developmental origins of subliminal face processing. Neurosci Biobehav Rev. (2020) 116:454–60. doi: 10.1016/j.neubiorev.2020.07.003
75. Lieberman, MD. Social cognitive neuroscience: a review of Core processes. Annu Rev Psychol. (2007) 58:259–89. doi: 10.1146/annurev.psych.58.110405.085654
76. Luyten, P, Campbell, C, Allison, E, and Fonagy, P. The Mentalizing approach to psychopathology: state of the art and future directions. Annu Rev Clin Psychol. (2020) 16:297–325. doi: 10.1146/annurev-clinpsy-071919-015355
77. Shamay-Tsoory, SG, Aharon-Peretz, J, and Perry, D. Two Systems for Empathy: a double dissociation between emotional and cognitive empathy in inferior frontal gyrus versus ventromedial prefrontal lesions. Brain. (2009) 132:279. doi: 10.1093/brain/awn279
78. Abdallah, M, Zanitti, GE, Iovene, V, and Wassermann, D. Functional gradients in the human lateral prefrontal cortex revealed by a comprehensive coordinate-based Meta-analysis. elife. (2022) 11:76926. doi: 10.7554/eLife.76926
79. Koechlin, Etienne. The cognitive architecture of the human lateral prefrontal cortex. Sensorimotor foundations of higher cognition. ed. Haggard: Patrick, Oxford, 1993; online edn, Oxford Academic, 22 Mar. (2012). 482–509.
80. Cardinale, RC, Shih, P, Fishman, I, Ford, LM, and Müller, R-A. Pervasive rightward asymmetry shifts of functional networks in autism Spectrum disorder. JAMA Psychiat. (2013) 70:975–82. doi: 10.1001/jamapsychiatry.2013.382
81. Shin, JE, Choi, SH, Lee, H, Shin, YS, Jang, DP, and Kim, JJ. Involvement of the dorsolateral prefrontal cortex and superior temporal sulcus in impaired social perception in schizophrenia. Prog Neuro-Psychopharmacol Biol Psychiatry. (2015) 58:6. doi: 10.1016/j.pnpbp.2014.12.006
82. Osório, AAC, and Brunoni, AR. Transcranial direct current stimulation in children with autism Spectrum disorder: a systematic scoping review. Dev Med Child Neurol. (2019) 61:298–304 doi: 10.1111/dmcn.14104
83. Rassovsky, Y, Dunn, W, Wynn, J, Wu, AD, Iacoboni, M, Hellemann, G, et al. The effect of transcranial direct current stimulation on social cognition in schizophrenia: a preliminary study. Schizophr Res. (2015) 165:2–3. doi: 10.1016/j.schres.2015.04.016
84. Lau, T, Gershman, SJ, and Cikara, M. Social structure learning in human anterior insula. elife. (2020) 9:53162. doi: 10.7554/eLife.53162
85. Park, SA, Miller, DS, and Boorman, ED. Inferences on a multidimensional social hierarchy use a grid-like code. Nat Neurosci. (2021) 24:916. doi: 10.1038/s41593-021-00916-3
86. McCrory, E, Foulkes, L, and Viding, E. The lancet psychiatry. Lancet Psychiatry. (2022) 9:828, 202–37. doi: 10.1016/S2215-0366(22)00202-4
87. Tabibnia, G. An affective neuroscience model of boosting resilience in adults. Neurosci Biobehav Rev. (2020) 115:321–350 doi: 10.1016/j.neubiorev.2020.05.005
88. Farrow, TFD, Jones, SC, Kaylor-Hughes, CJ, Wilkinson, ID, Woodruff, PWR, Hunter, MD, et al. Higher or lower? The functional anatomy of perceived Allocentric social hierarchies. NeuroImage. (2011) 57:1552–60. doi: 10.1016/j.neuroimage.2011.05.069
89. Zink, CF, Tong, Y, Chen, Q, Bassett, DS, Stein, JL, and Meyer-Lindenberg, A. Know your place: neural processing of social hierarchy in humans. Neuron. (2008) 58:273–83. doi: 10.1016/j.neuron.2008.01.025
90. Karafin, MS, Tranel, D, and Adolphs, R. Dominance attributions following damage to the ventromedial prefrontal cortex. J Cogn Neurosci. (2004) 16:1796–804. doi: 10.1162/0898929042947856
91. Carlén, M. What constitutes the prefrontal cortex? Science. (2017) 358:478–82. doi: 10.1126/science.aan8868
92. Chafee, MV, and Heilbronner, SR. Prefrontal cortex. Curr Biol. (2022) 32:R346–51. doi: 10.1016/j.cub.2022.02.071
93. Le, MP, Ährlund-Richter, S, and Carlén, M. The mouse prefrontal cortex: unity in diversity. Neuron. (2021) 109:1925–44. doi: 10.1016/j.neuron.2021.03.035
94. Laubach, M, Amarante, LM, Swanson, K, and White, SR. What, if anything, is rodent prefrontal cortex? ENeuro. (2018) 5:–2018. doi: 10.1523/ENEURO.0315-18.2018
95. Leonard, CM. Finding prefrontal cortex in the rat. Brain Res. (2016):1645, 1–3. doi: 10.1016/j.brainres.2016.02.002
96. Uylings, HBM, Groenewegen, HJ, and Kolb, B. Do rats have a prefrontal cortex? Behav Brain Res. (2003) 146:3–17 doi: 10.1016/j.bbr.2003.09.028
97. Yang, ST, Gu, E, Lu, X, Wang, J-H, and Li, B-M. Essential role of axonal VGSC inactivation in time-dependent deceleration and unreliability of spike propagation at cerebellar Purkinje cells. Mol Brain. (2014) 7:1–13. doi: 10.1186/1756-6606-7-1
98. Tamura, M, Spellman, TJ, Rosen, AM, Gogos, JA, and Gordon, JA. Hippocampal-prefrontal Theta-gamma coupling during performance of a spatial working memory task. Nat Commun. (2017) 8:2108. doi: 10.1038/s41467-017-02108-9
99. Mederos, S, Sánchez-Puelles, C, Esparza, J, Valero, M, Ponomarenko, A, and Perea, G. GABAergic signaling to astrocytes in the prefrontal cortex sustains goal-directed behaviors. Nat Neurosci. (2021) 24:752. doi: 10.1038/s41593-020-00752-x
100. Le Merre, P, Esmaeili, V, Charrière, E, Galan, K, Salin, PA, Petersen, CCH, et al. Reward-based learning drives rapid sensory signals in medial prefrontal cortex and dorsal Hippocampus necessary for goal-directed behavior. Neuron. (2018) 97:31. doi: 10.1016/j.neuron.2017.11.031
101. Wise, SP. Forward frontal fields: phylogeny and fundamental function. Trends Neurosci. (2008) 31:8. doi: 10.1016/j.tins.2008.08.008
102. Etkin, A, Egner, T, and Kalisch, R. Emotional processing in anterior cingulate and medial prefrontal cortex. Trends Cogn Sci. (2011) 15:85–93. doi: 10.1016/j.tics.2010.11.004
103. Kesner, RP. Subregional analysis of mnemonic functions of the prefrontal cortex in the rat. Psychobiology. (2000) 28:219–28. doi: 10.3758/bf03331980
104. Lewkowicz, DJ, and Bremner, AJ. The development of multisensory processes for perceiving the environment and the self In: Multisensory perception. eds. K Sathian, V.S. Ramachandran. Academic Press. (2020). 89–112.
105. Young, LJ. The neurobiology of social recognition, approach, and avoidance. Biol Psychiatry. (2002) 51:18–26. doi: 10.1016/S0006-3223(01)01268-9
106. Noack, J, Richter, K, Laube, G, Haghgoo, HA, Veh, RW, and Engelmann, M. Different importance of the volatile and non-volatile fractions of an olfactory signature for individual social recognition in rats versus mice and short-term versus Long-term memory. Neurobiol Learn Mem. (2010) 94:568–75. doi: 10.1016/j.nlm.2010.09.013
107. Frith, C. Role of facial expressions in social interactions. Philos Trans R Soc B. (2009) 364:3453–8. doi: 10.1098/rstb.2009.0142
108. Thor, DH, and Holloway, WR. Social memory of the male laboratory rat. J Comp Physiol Psychol. (1982) 96:1000–6. doi: 10.1037/0735-7036.96.6.1000
109. Yang, M, and Crawley, JN. Simple behavioral assessment of mouse olfaction. Curr Protoc Neurosci. (2009) 48:8–24. doi: 10.1002/0471142301.ns0824s48
110. Moy, SS, Nadler, JJ, Perez, A, Barbaro, RP, Johns, JM, Magnuson, TR, et al. Sociability and preference for social novelty in five inbred strains: an approach to assess autistic-like behavior in mice. Genes Brain Behav. (2004) 3:287–302. doi: 10.1111/j.1601-1848.2004.00076.x
111. Netser, S, Meyer, A, Magalnik, H, Zylbertal, A, de la Zerda, SH, Briller, M, et al. Distinct dynamics of social motivation drive differential social behavior in laboratory rat and mouse strains. Nat Commun 11. 5908 (2020). doi: 10.1038/s41467-020-19569-0
112. Insel, TR, and Hulihan, TJ. A gender-specific mechanism for pair bonding: oxytocin and partner preference formation in monogamous voles. Behav Neurosci. (1995) 109:782–9. doi: 10.1037/0735-7044.109.4.782
113. Church, RM. Emotional reactions of rats to the pain of others. J Comp Physiol Psychol. (1959) 52:132–4. doi: 10.1037/h0043531
114. Langford, DJ, Crager, SE, Shehzad, Z, Smith, SB, Sotocinal, SG, Levenstadt, JS, et al. Social modulation of pain as evidence for empathy in mice. Science. (2006) 312:1967–70. doi: 10.1126/science.1128322
115. Toyoshima, M, Okuda, E, Hasegawa, N, Kaseda, K, and Yamada, K. Socially transferred stress experience modulates social affective behaviors in rats. Neuroscience. (2022) 502:68–76. doi: 10.1016/j.neuroscience.2022.08.022
116. Jones, CE, Riha, PD, Gore, AC, and Monfils, M-H. Social transmission of Pavlovian fear: fear-conditioning by-proxy in related female rats. Anim Cogn. (2014) 17:827–34. doi: 10.1007/s10071-013-0711-2
117. Kim, S, Mátyás, F, Lee, S, Acsády, L, and Shin, H-S. Lateralization of observational fear learning at the cortical but not thalamic level in mice. Proc Natl Acad Sci. (2012) 109:15497–501. doi: 10.1073/pnas.1213903109
118. Choleris, E, Guo, C, Liu, H, Mainardi, M, and Valsecchi, P. The effect of demonstrator age and number on duration of socially-induced food preferences in house mouse (Mus Domesticus). Behav Process. (1997) 41:69–77. doi: 10.1016/S0376-6357(97)00029-6
119. Bruchey, AK, Jones, CE, and Monfils, M-H. Fear conditioning by-proxy: social transmission of fear during memory retrieval. Behav Brain Res. (2010) 214:80–4. doi: 10.1016/j.bbr.2010.04.047
120. Hernandez-Lallement, J, Attah, AT, Soyman, E, Pinhal, CM, Gazzola, V, and Keysers, C. Harm to others acts as a negative Reinforcer in rats. Curr Biol. (2020) 30:949–961.e7. doi: 10.1016/j.cub.2020.01.017
121. Hernandez-Lallement, J, Van Wingerden, M, Marx, C, Srejic, M, and Kalenscher, T. Rats prefer mutual rewards in a prosocial choice task. Front Neurosci. (2015) 8:443. doi: 10.3389/fnins.2014.00443
122. Scheggia, D, Managò, F, Maltese, F, Bruni, S, Nigro, M, Dautan, D, et al. Somatostatin interneurons in the prefrontal cortex control affective state discrimination in mice. Nat Neurosci. (2020) 23:47–60. doi: 10.1038/s41593-019-0551-8
123. Bartal, IB-A, Decety, J, and Mason, P. Empathy and pro-social behavior in rats. Science. (2011) 334:1427–30. doi: 10.1126/science.1210789
124. Dolivo, V, and Taborsky, M. Norway rats reciprocate help according to the quality of help they received. Biol Lett. (2015) 11:20140959. doi: 10.1098/rsbl.2014.0959
125. Márquez, C, Rennie, SM, Costa, DF, and Moita, MA. Prosocial choice in rats depends on food-seeking behavior displayed by recipients. Curr Biol. (2015) 25:1736–45. doi: 10.1016/j.cub.2015.05.018
126. Burkett, JP, Andari, E, Johnson, ZV, Curry, DC, de Waal, FBM, and Young, LJ. Oxytocin-dependent consolation behavior in rodents. Science. (2016) 351:375–8. doi: 10.1126/science.aac4785
127. Lindzey, G, Manosevitz, M, and Winston, H. Social dominance in the mouse. Psychon Sci. (1966) 5:451–2. doi: 10.3758/BF03331044
128. Shemesh, Y, Sztainberg, Y, Forkosh, O, Shlapobersky, T, Chen, A, and Schneidman, E. High-order social interactions in groups of mice. elife. (2013) 2:e00759. doi: 10.7554/eLife.00759
129. Weissbrod, A, Shapiro, A, Vasserman, G, Edry, L, Dayan, M, Yitzhaky, A, et al. Automated Long-term tracking and social Behavioural phenotyping of animal colonies within a semi-natural environment. Nat Commun. (2013) 4:2018. doi: 10.1038/ncomms3018
130. Scheggia, D, la Greca, F, Maltese, F, Chiacchierini, G, Italia, M, Molent, C, et al. Reciprocal Cortico-amygdala connections regulate prosocial and selfish choices in mice. Nat Neurosci. (2022) 25:1505–18. doi: 10.1038/s41593-022-01179-2
131. Gunaydin, LA, Grosenick, L, Finkelstein, JC, Kauvar, IV, Fenno, LE, Adhikari, A, et al. Natural neural projection dynamics underlying social behavior. Cells. (2014) 157:1535–51. doi: 10.1016/j.cell.2014.05.017
132. Kas, MJ, Modi, ME, Saxe, MD, and Smith, DG. Advancing the discovery of medications for autism Spectrum disorder using new technologies to reveal social brain circuitry in rodents. Psychopharmacology. (2014) 231:1147–65. doi: 10.1007/s00213-014-3464-y
133. Avale, ME, Chabout, J, Pons, S, Serreau, P, De Chaumont, F, Olivo-Marin, J-C, et al. Prefrontal nicotinic receptors control novel social interaction between mice. FASEB J. (2011) 25:2145–55. doi: 10.1096/fj.10-178558
134. Rudebeck, PH, Walton, ME, Millette, BHP, Shirley, E, Rushworth, MFS, and Bannerman, DM. Distinct contributions of frontal areas to emotion and social behaviour in the rat. Eur J Neurosci. (2007) 26:2315–26. doi: 10.1111/j.1460-9568.2007.05844.x
135. Kim, Y, Venkataraju, KU, Pradhan, K, Mende, C, Taranda, J, Turaga, SC, et al. Mapping social behavior-induced brain activation at cellular resolution in the mouse. Cell Rep. (2015) 10:292–305. doi: 10.1016/j.celrep.2014.12.014
136. Collins, DP, Anastasiades, PG, Marlin, JJ, and Carter, AG. Reciprocal circuits linking the prefrontal cortex with dorsal and ventral thalamic nuclei. Neuron. (2018) 98:366–379.e4. doi: 10.1016/j.neuron.2018.03.024
137. Lu, J, Tucciarone, J, Padilla-Coreano, N, He, M, Gordon, JA, and Josh Huang, Z. Selective inhibitory control of pyramidal neuron ensembles and cortical subnetworks by chandelier cells. Nat Neurosci. (2017) 20:1377–83. doi: 10.1038/nn.4624
138. McGarry, LM, and Carter, AG. Inhibitory gating of basolateral amygdala inputs to the prefrontal cortex. J Neurosci. (2016) 36:9391–406. doi: 10.1523/JNEUROSCI.0874-16.2016
139. Anastasiades, PG, Marlin, JJ, and Carter, AG. Cell-type specificity of Callosally evoked excitation and feedforward inhibition in the prefrontal cortex. Cell Rep. (2018) 22:679–92. doi: 10.1016/j.celrep.2017.12.073
140. Lee, AT, Gee, SM, Vogt, D, Patel, T, Rubenstein, JL, and Sohal, VS. Pyramidal neurons in prefrontal cortex receive subtype-specific forms of excitation and inhibition. Neuron. (2014) 81:61–8. doi: 10.1016/j.neuron.2013.10.031
141. Gogolla, N, LeBlanc, JJ, Quast, KB, Südhof, TC, Fagiolini, M, and Hensch, TK. Common circuit defect of excitatory-inhibitory balance in mouse models of autism. J Neurodev Disord. (2009) 1:172–81. doi: 10.1007/s11689-009-9023-x
142. Gonçalves, J, Violante, IR, Sereno, J, Leitão, RA, Cai, Y, Abrunhosa, A, et al. Testing the excitation/inhibition imbalance hypothesis in a mouse model of the autism Spectrum disorder: in vivo Neurospectroscopy and molecular evidence for regional phenotypes. Mol Autism. (2017) 8:1–8. doi: 10.1186/s13229-017-0166-4
143. Sacai, H, Sakoori, K, Konno, K, Nagahama, K, Suzuki, H, Watanabe, T, et al. Autism Spectrum disorder-like behavior caused by reduced excitatory synaptic transmission in pyramidal neurons of mouse prefrontal cortex. Nat Commun. (2020) 11:5140. doi: 10.1038/s41467-020-18861-3
144. Hensch, TK. Critical period plasticity in local cortical circuits. Nat Rev Neurosci. (2005) 6:877–88. doi: 10.1038/nrn1787
145. Nelson, SB, and Valakh, V. Excitatory/inhibitory balance and circuit homeostasis in autism Spectrum disorders. Neuron. (2015) 87:684–98. doi: 10.1016/j.neuron.2015.07.033
146. Fetit, R, Hillary, RF, Price, DJ, and Lawrie, SM. The neuropathology of autism: a systematic review of post-mortem studies of autism and related disorders. Neurosci Biobehav Rev. (2021) 129:35–62. doi: 10.1016/j.neubiorev.2021.07.014
147. Fatemi, SH, Folsom, TD, Kneeland, RE, and Liesch, SB. Metabotropic glutamate receptor 5 upregulation in children with autism is associated with Underexpression of both fragile X mental retardation protein and GABAA receptor Beta 3 in adults with autism. Anat Rec. (2011) 294:1635–45. doi: 10.1002/ar.21299
148. Fatemi, SH, Reutiman, TJ, Folsom, TD, and Thuras, PD. GABA a receptor downregulation in brains of subjects with autism. J Autism Dev Disord. (2009) 39:223–30. doi: 10.1007/s10803-008-0646-7
149. Oblak, A, Gibbs, TT, and Blatt, GJ. Decreased GABAA receptors and benzodiazepine binding sites in the anterior cingulate cortex in autism. Autism Res. (2009) 2:205–19. doi: 10.1002/aur.88
150. Kolodny, T, Schallmo, M-P, Gerdts, J, Edden, RAE, Bernier, RA, and Murray, SO. Concentrations of cortical GABA and glutamate in young adults with autism spectrum disorder. Autism Res. (2020) 13:1111–29. doi: 10.1002/aur.2300
151. Lohith, TG, Osterweil, EK, Fujita, M, Jenko, KJ, Bear, MF, and Innis, RB. Is metabotropic glutamate receptor 5 upregulated in prefrontal cortex in fragile X syndrome? Mol Autism. (2013) 4:15–8. doi: 10.1186/2040-2392-4-15
152. Hashemi, E, Ariza, J, Rogers, H, Noctor, SC, and Martínez-Cerdeño, V. The number of Parvalbumin-expressing interneurons is decreased in the prefrontal cortex in autism. Cereb Cortex. (2017) 27:1931–43. doi: 10.1093/cercor/bhw021
153. Bartos, M, Vida, I, and Jonas, P. Synaptic mechanisms of synchronized gamma oscillations in inhibitory interneuron networks. Nat Rev Neurosci. (2007) 8:45–56. doi: 10.1038/nrn2044
154. Sohal, VS, and Rubenstein, JLR. Excitation-inhibition balance as a framework for investigating mechanisms in neuropsychiatric disorders. Mol Psychiatry. (2019) 24:1248–57. doi: 10.1038/s41380-019-0426-0
155. O’Reilly, C, Lewis, JD, and Elsabbagh, M. Is functional brain connectivity atypical in autism? A systematic review of EEG and MEG studies. PLoS One. (2017) 12:e0175870. doi: 10.1371/journal.pone.0175870
156. Wass, S. Distortions and disconnections: disrupted brain connectivity in autism. Brain Cogn. (2011) 75:18–28. doi: 10.1016/j.bandc.2010.10.005
157. Zikopoulos, B, and Barbas, H. Altered neural connectivity in excitatory and inhibitory cortical circuits in autism. Front Hum Neurosci. (2013) 7:609. doi: 10.3389/fnhum.2013.00609
158. Carlisi, CO, Norman, L, Murphy, CM, Christakou, A, Chantiluke, VG, Giampietro, V, et al. Comparison of neural substrates of temporal discounting between youth with autism Spectrum disorder and with obsessive-compulsive disorder. Psychol Med. (2017) 47:2513–27. doi: 10.1017/S0033291717001088
159. Kohls, G, Antezana, L, Mosner, MG, Schultz, RT, and Yerys, BE. Altered reward system reactivity for personalized circumscribed interests in autism. Mol Autism. (2018) 9:1–12. doi: 10.1186/s13229-018-0195-7
160. Clements, CC, Zoltowski, AR, Yankowitz, LD, Yerys, BE, Schultz, RT, and Herrington, JD. Evaluation of the social motivation hypothesis of autism: a systematic review and Meta-analysis. JAMA Psychiat. (2018) 75:797–808. doi: 10.1001/jamapsychiatry.2018.1100
161. Ferguson, BR, and Gao, W-J. PV interneurons: critical regulators of E/I balance for prefrontal cortex-dependent behavior and psychiatric disorders. Front Neural Circuits. (2018) 12:37. doi: 10.3389/fncir.2018.00037
162. Yizhar, O, Fenno, LE, Prigge, M, Schneider, F, Davidson, TJ, O’Shea, DJ, et al. Neocortical excitation/inhibition balance in information processing and social dysfunction. Nature. (2011) 477:171–8. doi: 10.1038/nature10360
163. Wu, J, Aton, SJ, Booth, V, and Zochowski, M. Network and cellular mechanisms underlying heterogeneous excitatory/inhibitory balanced states. Eur J Neurosci. (2020) 51:1624–41. doi: 10.1111/ejn.14669
164. Chen, L, Li, X, Tjia, M, and Thapliyal, S. Homeostatic plasticity and excitation-inhibition balance: the good, the bad, and the ugly. Curr Opin Neurobiol. (2022) 75:102553. doi: 10.1016/j.conb.2022.102553
165. Isaacson, JS, and Scanziani, M. How inhibition shapes cortical activity. Neuron. (2011) 72:231–43. doi: 10.1016/j.neuron.2011.09.027
166. Tremblay, R, Lee, S, and Rudy, B. GABAergic interneurons in the neocortex: from cellular properties to circuits. Neuron. (2016) 91:260–92. doi: 10.1016/j.neuron.2016.06.033
167. Gandal, MJ, Anderson, RL, Billingslea, EN, Carlson, GC, Roberts, TPL, and Siegel, SJ. Mice with reduced NMDA receptor expression: more consistent with autism than schizophrenia? Genes Brain Behav. (2012) 11:740–50. doi: 10.1111/j.1601-183X.2012.00816.x
168. Contractor, A, Ethell, IM, and Portera-Cailliau, C. Cortical interneurons in autism. Nat Neurosci. (2021) 24:1648–59. doi: 10.1038/s41593-021-00967-6
169. Vogt, D, Cho, KKA, Lee, AT, Sohal, VS, and Rubenstein, JLR. The Parvalbumin/somatostatin ratio is increased in Pten mutant mice and by human PTEN ASD alleles. Cell Rep. (2015) 11:944–56. doi: 10.1016/j.celrep.2015.04.019
170. Filice, F, Vörckel, KJ, Sungur, AÖ, Wöhr, M, and Schwaller, B. Reduction in Parvalbumin expression not loss of the Parvalbumin-expressing GABA interneuron subpopulation in genetic Parvalbumin and shank mouse models of autism. Mol Brain. (2016) 9:1–17. doi: 10.1186/s13041-016-0192-8
171. Lauber, E, Filice, F, and Schwaller, B. Dysregulation of Parvalbumin expression in the Cntnap2−/− mouse model of autism Spectrum disorder. Front Mol Neurosci. (2018) 11:262. doi: 10.3389/fnmol.2018.00262
172. Nomura, T, Musial, TF, Marshall, JJ, Zhu, Y, Remmers, CL, Xu, J, et al. Delayed maturation of fast-spiking interneurons is rectified by activation of the TrkB receptor in the mouse model of fragile X syndrome. J Neurosci. (2017) 37:11298–310. doi: 10.1523/JNEUROSCI.2893-16.2017
173. Duffney, LJ, Zhong, P, Wei, J, Matas, E, Cheng, J, Qin, L, et al. Autism-like deficits in Shank3-deficient mice are rescued by targeting actin regulators. Cell Rep. (2015) 11:1400–13. doi: 10.1016/j.celrep.2015.04.064
174. Kim, S, Kim, Y-E, Song, I, Ujihara, Y, Kim, N, Jiang, Y-H, et al. Neural circuit pathology driven by Shank3 mutation disrupts social behaviors. Cell Rep. (2022) 39:110906. doi: 10.1016/j.celrep.2022.110906
175. Qin, L, Ma, K, and Yan, Z. Chemogenetic activation of prefrontal cortex in Shank3-deficient mice ameliorates social deficits, NMDAR hypofunction, and Sgk2 downregulation. IScience. (2019) 17:24–35. doi: 10.1016/j.isci.2019.06.014
176. Finlay, JM, Dunham, GA, Isherwood, AM, Newton, CJ, Nguyen, TV, Reppar, PC, et al. Effects of prefrontal cortex and hippocampal NMDA NR1-subunit deletion on complex cognitive and social behaviors. Brain Res. (2015) 1600:70–83. doi: 10.1016/j.brainres.2014.10.037
177. Robertson, CE, Ratai, E-M, and Kanwisher, N. Reduced GABAergic action in the autistic brain. Curr Biol. (2016) 26:80–5. doi: 10.1016/j.cub.2015.11.019
178. Ueda, S, Niwa, M, Hioki, H, Sohn, J, Kaneko, T, Sawa, A, et al. Sequence of molecular events during the maturation of the developing mouse prefrontal cortex. Complex Psychiatry. (2015) 1:94–104. doi: 10.1159/000430095
179. Savage, S, Kehr, J, Olson, L, and Mattsson, A. Impaired social interaction and enhanced sensitivity to phencyclidine-induced deficits in novel object recognition in rats with cortical cholinergic denervation. Neuroscience. (2011) 195:60–9. doi: 10.1016/j.neuroscience.2011.08.027
180. Okada, K, Nishizawa, K, Kobayashi, T, Sakata, S, Hashimoto, K, and Kobayashi, K. Different cholinergic cell groups in the basal forebrain regulate social interaction and social recognition memory. Sci Rep. (2021) 11:13589. doi: 10.1038/s41598-021-93045-7
181. Froemke, RC, and Young, LJ. Oxytocin, neural plasticity, and social behavior. Annu Rev Neurosci. (2021) 44:359–81. doi: 10.1146/annurev-neuro-102320-102847
182. Oettl, LL, Ravi, N, Schneider, M, Scheller, MF, Schneider, P, Mitre, M, et al. Oxytocin enhances social recognition by modulating cortical control of early olfactory processing. Neuron. (2016) 90:609–21. doi: 10.1016/j.neuron.2016.03.033
183. Smeltzer, MD, Curtis, JT, Aragona, BJ, and Wang, Z. Dopamine, oxytocin, and vasopressin receptor binding in the medial prefrontal cortex of monogamous and promiscuous voles. Neurosci Lett. (2006) 394:146–51. doi: 10.1016/j.neulet.2005.10.019
184. Yaseen, A, Shrivastava, K, Zuri, Z, Hatoum, OA, and Maroun, M. Prefrontal oxytocin is involved in impairments in prefrontal plasticity and social memory following acute exposure to high fat diet in juvenile animals. Cereb Cortex. (2019) 29:1900–9. doi: 10.1093/cercor/bhy070
185. Nakajima, M, Görlich, A, and Heintz, N. Oxytocin modulates female Sociosexual behavior through a specific class of prefrontal cortical interneurons. Cells. (2014) 159:295–305. doi: 10.1016/j.cell.2014.09.020
186. Janz, P, Knoflach, F, Bleicher, K, Belli, S, Biemans, B, Schnider, P, et al. Selective oxytocin receptor activation prevents prefrontal circuit dysfunction and social behavioral alterations in response to chronic prefrontal cortex activation in rats. BioRxiv. (2022). doi: 10.1101/2022.11.08.515590
187. Riad, MH, Park, K, Ibañez-Tallon, I, and Heintz, N. Local production of Corticotropin-releasing hormone in prefrontal cortex modulates male-specific novelty exploration. Proc Natl Acad Sci. (2022) 119:e2211454119. doi: 10.1073/pnas.2211454119
188. Reyes, L, de, NS, Diaz, PAS, Manas, RN, Ruiz-Pino, A, Nomura, Y, et al. Corticotropin-releasing hormone signaling from prefrontal cortex to lateral septum supports social novelty preference. BioRxiv. (2022). doi: 10.1101/2022.03.15.484224
189. Eussen, MLJM, Louwerse, A, Herba, CM, Van Gool, AR, Verheij, F, Verhulst, FC, et al. Childhood facial recognition predicts adolescent symptom severity in autism Spectrum disorder. Autism Res. (2015) 8:261–71. doi: 10.1002/aur.1443
190. Webb, SJ, Neuhaus, E, and Faja, S. Face perception and learning in autism spectrum disorders. Q J Exp Psychol. (2017) 70:970–86. doi: 10.1080/17470218.2016.1151059
191. Strauss, KA, Puffenberger, EG, Huentelman, MJ, Gottlieb, S, Dobrin, SE, Parod, JM, et al. Recessive symptomatic focal epilepsy and mutant Contactin-associated protein-like 2. N Engl J Med. (2006) 354:1370–7. doi: 10.1056/NEJMoa052773
192. Selimbeyoglu, A, Kim, CK, Inoue, M, Lee, SY, Hong, ASOO, Kauvar, I, et al. Modulation of prefrontal cortex excitation/inhibition balance rescues social behavior in CNTNAP2-deficient mice. Sci Transl Med. (2017) 9:eaah6733. doi: 10.1126/scitranslmed.aah6733
193. Cholfin, JA, and Rubenstein, JLR. Patterning of frontal cortex subdivisions by Fgf17. Proc Natl Acad Sci. (2007) 104:7652–7. doi: 10.1073/pnas.0702225104
194. Scearce-Levie, K, Roberson, ED, Gerstein, H, Cholfin, JA, Mandiyan, VS, Shah, NM, et al. Abnormal social behaviors in mice lacking Fgf17. Genes Brain Behav. (2008) 7:344–54. doi: 10.1111/j.1601-183X.2007.00357.x
195. Zou, H, Zhang, C, Xie, Q, Zhang, M, Shi, J, Jin, M, et al. Low dose MK-801 reduces social investigation in mice. Pharmacol Biochem Behav. (2008) 90:753–7. doi: 10.1016/j.pbb.2008.06.002
196. Belforte, JE, Zsiros, V, Sklar, ER, Jiang, Z, Yu, G, Li, Y, et al. Postnatal NMDA receptor ablation in Corticolimbic interneurons confers schizophrenia-like phenotypes. Nat Neurosci. (2010) 13:76–83. doi: 10.1038/nn.2447
197. Ben-Ami Bartal, I, Breton, JM, Sheng, H, Long, KLP, Chen, S, Halliday, A, et al. Neural correlates of Ingroup Bias for Prosociality in rats. elife. (2021) 10:e65582. doi: 10.7554/eLife.65582
198. Kingsbury, L, Huang, S, Raam, T, Ye, LS, Wei, D, Hu, RK, et al. Cortical representations of conspecific sex shape social behavior. Neuron. (2020) 107:941–953.e7. doi: 10.1016/j.neuron.2020.06.020
199. Levy, DR, Tamir, T, Kaufman, M, Parabucki, A, Weissbrod, A, Schneidman, E, et al. Dynamics of social representation in the mouse prefrontal cortex. Nat Neurosci. (2019) 22:2013–22. doi: 10.1038/s41593-019-0531-z
Keywords: autism, prefrontal cortex, rodent models, social interaction, social behavior
Citation: Mohapatra AN and Wagner S (2023) The role of the prefrontal cortex in social interactions of animal models and the implications for autism spectrum disorder. Front. Psychiatry. 14:1205199. doi: 10.3389/fpsyt.2023.1205199
Received: 16 April 2023; Accepted: 26 May 2023;
Published: 20 June 2023.
Edited by:
Lei Xu, Sichuan Normal University, ChinaReviewed by:
Xiaoxiao Zheng, Chinese Academy of Sciences, ChinaCopyright © 2023 Mohapatra and Wagner. This is an open-access article distributed under the terms of the Creative Commons Attribution License (CC BY). The use, distribution or reproduction in other forums is permitted, provided the original author(s) and the copyright owner(s) are credited and that the original publication in this journal is cited, in accordance with accepted academic practice. No use, distribution or reproduction is permitted which does not comply with these terms.
*Correspondence: Alok Nath Mohapatra, dGhpbmthbG9rQGdtYWlsLmNvbQ==
Disclaimer: All claims expressed in this article are solely those of the authors and do not necessarily represent those of their affiliated organizations, or those of the publisher, the editors and the reviewers. Any product that may be evaluated in this article or claim that may be made by its manufacturer is not guaranteed or endorsed by the publisher.
Research integrity at Frontiers
Learn more about the work of our research integrity team to safeguard the quality of each article we publish.